Tomosyn expression pattern in the mouse hippocampus suggests both presynaptic and postsynaptic functions
- 1 Department of Neurobiology, The George S. Wise Faculty of Life Sciences, Tel-Aviv University, Tel Aviv, Israel
- 2 Department of Molecular and Integrative Physiology, University of Michigan, Ann Arbor, MI, USA
- 3 Laboratory of Neuroscience, National Institute on Aging, National Institutes of Health, Baltimore, MD, USA
- 4 Physiologisches Institut, Universität des Saarlandes, Homburg, Saar, Germany
The protein tomosyn decreases synaptic transmission and release probability of vesicles, and is essential for modulating synaptic transmission in neurons. In this study, we provide a detailed description of the expression and localization patterns of tomosyn1 and tomosyn2 in the subareas of the mouse hippocampus. Using confocal and two-photon high-resolution microscopy we demonstrate that tomosyn colocalizes with several pre- and postsynaptic markers and is found mainly in glutamatergic synapses. Specifically, we show that tomosyn1 is differentially distributed in the mouse hippocampus and concentrated mainly in the hilus and mossy fibers. Surprisingly, we found that tomosyn2 is expressed in the subiculum, CA1 and CA2 pyramidal cell bodies, dendrites and spines, and colocalizes with PSD95, suggesting a postsynaptic role. These results suggest that in addition to the well-characterized presynaptic function of tomosyn in neurotransmitter release, tomosyn2 might have a postsynaptic function, and place tomosyn as a more general regulator of synaptic transmission and plasticity.
Introduction
The mouse hippocampus has been well characterized and is commonly divided into the following major areas: CA1, CA2, CA3, dentate gyrus, and hilus. Together with other essential structures, the hippocampus is involved in mammalian learning and memory (both spatial, recognition, episodic, and long-term declarative memories; Aggleton and Brown, 1999; Mumby et al., 1999; Reilly, 2001), depression and fear (Geinisman, 2000; Geinisman et al., 2000). As a key player in a variety of important processes, the hippocampus has considerable connections to other cortical and subcortical areas, such as the entorhinal cortex, prefrontal cortex, and thalamus. Not surprisingly, it is also the main brain area affected by neurodegenerative diseases, epilepsy, and stress.
In the synapse, synaptic transmission involves several steps, starting with neurotransmitter uptake to the synaptic vesicle, docking of the vesicle to the plasma membrane at active zones, priming of the vesicle [a process believed to represent formation of SNARE (soluble N-ethylamide-sensitive factor attachment protein receptor) protein complexes, which renders the vesicle fusion competent], and calcium-dependent fusion of the vesicle, leading to neurotransmitter release to the synaptic cleft (Richmond and Broadie, 2002; Sudhof, 2004; Becherer and Rettig, 2006; Rizo and Rosenmund, 2008; Verhage and Sorensen, 2008). This exocytotic process is followed by recycling of the vesicle through endocytotic pathways. The protein tomosyn takes part in regulating the priming step of the vesicle cycle by interfering with the formation of fusion-competent SNARE complexes and thereby inhibiting the vesicle’s fusion competence (Fujita et al., 1998; Gracheva et al., 2006; Sakisaka et al., 2008; Ashery et al., 2009). Many proteins define the level of synaptic transmission and plasticity in the hippocampus (such as short and long-term potentiation, STP and LTP, respectively; Castillo et al., 1997, 2002; Breustedt et al., 2010), hence, the specific localization and expression levels of those proteins can change synaptic transmission properties and hippocampus physiology (Powell, 2006). In addition, as every subarea in the hippocampus has its own role and connectivity (e.g., place cells in CA1; Amaral and Witter, 1989), it is of interest to characterize the expression of a key modulator protein such as tomosyn in the subareas of this essential structure.
Tomosyn, also known as a syntaxin-binding protein, is a 130-kDa cytosolic protein that decreases synaptic transmission and release probability of vesicles (Fujita et al., 1998; Yizhar et al., 2004; Baba et al., 2005; Gracheva et al., 2006; Ashery et al., 2009). In mammals, tomosyn is encoded by two genes, tomosyn1 and tomosyn2, that together form seven isoforms: tomosyn1 splice variants s, m, and b, and tomosyn2 splice variants s, m, b, and xb (Groffen et al., 2005).
Tomosyn1 mRNA distribution in the mouse hippocampus suggests a uniform distribution in the granular cell bodies of the dentate gyrus, and slightly lower but uniform distribution in CA3 and CA1 pyramidal cell bodies (Groffen et al., 2005). Tomosyn2 mRNA levels are higher in the CA2 pyramidal cell bodies, and uniformly distributed in the granular cell bodies of the dentate gyrus and CA1 pyramidal cell bodies (Groffen et al., 2005). In this article we expand upon the reported mRNA distribution to reveal a unique distribution pattern of tomosyn proteins in the hippocampal subareas.
For general and high-resolution analyses of tomosyn proteins localization together with a precise quantification of their expression, we used immunofluorescence approaches together with confocal and high-resolution two-photon microscopy. These techniques make it possible to determine both the protein’s localization and extent of expression in specific hippocampal areas. In addition, we employed double immunofluorescence staining to identify colocalization of tomosyn with pre- and postsynaptic marker proteins in order to specify tomosyn’s potential functional role more accurately. Our study aims to provide further information on tomosyn’s localization in one of the most important brain regions, the hippocampus, and to reveal its relationship with several well-defined proteins.
Materials and Methods
Experimental Animals
Six wild-type 10-week-old male C57BL/6J mice and six tomosyn1-knockout 8-week-old male mice (kindly provided by Prof. Y. Takai, Kobe University, Japan; Sakisaka et al., 2008) were used for this study.
Animals were housed in a controlled environment and were provided with food and tap water ad libitum. Room lights were on between 0500 and 1900 h. All experiments were performed in accordance with the Tel-Aviv University Animal Care Committee.
In all animals, the hippocampus was examined in the bregma range of −1.70 to −2.06 mm according to the mouse brain atlas (Franklin and Paxinos, 1997).
Immunofluorescence Staining
Mice were anesthetized with ketamine and xylazine and sacrificed by transcardiac perfusion with 0.9% saline followed by perfusion with 4% paraformaldehyde (PFA) in 0.1 M phosphate buffer, pH 7.4. Brains were removed and fixed in 4% PFA in 0.1 M phosphate buffer overnight at 4°C, and then left in 30% sucrose for two nights at 4°C.
Brains were cut on a freezing microtome at the level of the frontal cortex and cerebellum into a series of eight adjacent 30 μm thick coronal sections and collected into a cryoprotectant solution (30% ethylene glycol, 30% glycerol) in PBS, pH 7.4 and stored at −20°C until use. Free-floating sections were washed in PBS to remove any remnants of cryoprotectant solution. Sections were blocked with 20% normal goat serum (Vector Laboratories, Burlingame, CA, USA) with 0.1% Triton X-100 for 3 h at RT. Then the following relevant primary antibodies were applied for two nights at 4°C: tomosyn (1:100 home-made affinity-purified rabbit anti-tomosyn polyclonal, as was described in (Hatsuzawa et al., 2003)), synaptophysin (1:200 mouse anti-synaptophysin monoclonal, S-5768 clone SVP-38, Sigma, St Louis, MO, USA), MAP2 (1:100 mouse anti-MAP2 monoclonal, HM-2 clone M-4403, Sigma), VGluT1 (1:2000 guinea pig anti-VGluT1 polyclonal, AB-5905, Millipore, Billerica, MA, USA), VGAT (1:200 mouse anti-VGAT monoclonal, Cat. No. 131 011, Synaptic Systems, Goettingen, Germany), GAD67 (1:200 affinity-purified mouse anti-GAD67 monoclonal, MAB5406 clone 1G10.2, Millipore, Billerica, MA, USA). After rinsing with PBST (PBS with 0.1% Triton X-100), the sections were incubated for 1 h at RT at a 1:1000 dilution in 2% normal goat serum in PBST with the following relevant secondary antibodies: goat anti-rabbit 488, goat anti-mouse 568, goat anti-guinea pig 647 (Alexa fluor, Invitrogen, Carlsbad, CA, USA). Following rinses with PBST, the sections were incubated with 4′,6-diamidino-2-phenylindole (DAPI) at a 1:1000 dilution for 5 min, rinsed again with PBST, and mounted on a slide sealed with a coverslip. To minimize variability, sections from all animals were stained and treated simultaneously.
Briefly, for the PSD95 immunostaining mice were decapitated and their brains were immediately frozen in liquid nitrogen. Then 14 μm thick coronal sections were cut on a cryostat with temperature adjusted to −20°C. After 1 h at room temperature (RT), slices were fixed in absolute methanol precooled to −20°C for 5 min, and washed in PBS. Sections were blocked with 20% normal goat serum, and incubated over a night at 4°C with the primary antibodies tomosyn (1:100 home-made affinity-purified rabbit anti-tomosyn polyclonal, as was described in Hatsuzawa et al., 2003), and PSD95 (1:200 mouse anti-PSD95 monoclonal, P246 clone 7E3-1B8, Sigma, St Louis, MO, USA). Following rinsing with PBST, the sections were incubated for 1h at RT at a 1:1000 dilution in 2% normal goat serum in PBST with the secondary antibodies goat anti-rabbit 488 and goat anti-mouse 568 (Alexa fluor, Invitrogen, Carlsbad, CA, USA), rinsed with PBST and mounted with a coverslip.
The intensities of immunofluorescence staining and the relative densities of the stained cells in the hippocampus subareas were determined by measurements of the integrated optical density (IOD) sum divided by the total area of the manually selected subarea of the hippocampus using the Image-Pro Plus system (version 5.1; Media Cybernetics, Silver Spring, MD, USA). Each subarea of the hippocampus was manually selected using the irregular area-of-interest tool, and its IOD sum and total area were measured under an equal threshold that was used in all subareas. Two coronal sections in the relevant bregma range were analyzed for each animal.
Confocal and Two-Photon Microscopy
Fluorescence was visualized using a confocal laser scanning microscope (LSM 510; Zeiss, Oberkochen, Germany). Images (1024 × 1024 pixels) were obtained with 10×, 40×, and 63× lenses. Our confocal and two-photon microscope settings were optimized to eliminate background staining in both channels collected by calibrating the laser intensity, signal offset, and amplifier gain on slices treated without primary antibody. Other than making moderate adjustments for contrast and brightness, the images were not manipulated.
Colocalization Analysis
Background staining was subtracted from monochromatic images by subtracting the mean intensity of the image from the entire image (Metamorph version 6.1, Universal Imaging Corp.). Colocalization analysis was based on the Intensity Correlation Analysis plugin (ImageJ v1.37a), in which Pearson’s correlation coefficient values as well as the positive PDM (product of the differences from the mean) image were analyzed (for details, see Li et al., 2004). To verify that no random colocalization was measured, one of the images was horizontally flipped and colocalization analysis was performed: doing so, no colocalization was observed and the Pearson’s correlation values were negative. Profile display mode (LSM image browser, Zeiss, Oberkochen, Germany) was used for the intensity profile graphs.
Antibody Specificity
To verify the absence of non-specific immunostaining using the immunofluorescence method, primary antibodies were excluded but the secondary antibody steps were performed to completion. Under these conditions, no cross-reactivity or significant background staining was observed (not shown). In addition, our detailed tomosyn immunostaining pattern was similar to the general pattern shown in other publications (Fujita et al., 1998; Sakisaka et al., 2008).
Western Blot
The specificity of the affinity-purified rabbit anti-tomosyn polyclonal antibody was confirmed by western blot analysis. Lysates from COS-7 cells transfected [using jetPEI transfecting reagent (Polyplus-transfection, New York, NY, USA)] with mouse tomosyn gene 1 and 2 variants were prepared and analyzed by western blot technique (variants were a non-DNA-transfected control, tomosyn1 splice variants s and m, and tomosyn2 splice variants s, m, b, and xb). All genes were expressed from pLP vectors with citrine and were full-length. The samples were quantified for the amount of protein in each lysate. From these lysates, 50 μg samples were prepared and electrophoresed in a 12% Bis–Tris gel, along with the PageRuler Plus prestained protein ladder (Fermentas, Glen Burnie, MD, USA), for 30 min at 60 V, then for 2 h at 100 V on ice. The samples were then transferred to nitrocellulose membranes (Invitrogen, Carlsbad, CA, USA) at 200 mA for 2 h. Membranes were then blocked in 5% non-fat dry milk in TBS with 0.1% Tween-20 overnight at 4°C. The next day, membranes were washed for 5 min three times in TBS with 0.2% Tween-20 at RT and then probed with the primary antibodies: affinity-purified rabbit anti-tomosyn polyclonal antibody, 1:2000, and mouse anti-tubulin, 1:20000, in 0.05% sodium azide and 1% bovine serum albumin (BSA) in TBS with 0.1% Tween-20 at RT for 1 h. Then membranes were washed four times and incubated for 1 h at RT with the appropriate horseradish peroxidase-conjugated secondary antibody. After six washes, protein bands were visualized by ECL (Pierce® Thermo Scientific, Rockford, IL, USA).
Immunocytochemistry
In addition to the western blot, the specificity of the affinity-purified rabbit anti-tomosyn polyclonal antibody was confirmed by immunocytochemistry. COS-7 cells transfected (using jetPEI transfecting reagent) with mouse tomosyn gene 1 and 2 variants were grown on coverslips and fixed with 4% PFA for 20 min, permeabilized for 5 min in 0.1% Triton X-100, and blocked for 1 h in normal goat serum (200 μg/mL), all at RT. Then cells were probed with affinity-purified rabbit anti-tomosyn polyclonal, 1:200, for 1 h at RT and labeled with goat anti-rabbit 546 secondary antibody for 1 h at RT.
Multiple Sequence Alignments
Alignments to compare tomosyn1 and tomosyn2 amino acid sequences were performed using the ClustalW algorithm in combination with the GONNET 250 matrix (Gonnet et al., 1992). The sequence aligned corresponds to residues 985-1051 of rat tomosyn1-m.
Results
We characterized tomosyn’s expression pattern in the mouse hippocampus by examining both of its isoforms, tomosyn1 and tomosyn2, using an affinity-purified anti-tomosyn rabbit polyclonal antibody for immunostaining of the mouse hippocampus. First, we examined the specificity of the antibody for the different splice variants of mouse tomosyn1 and 2 genes. Since our study was based on immunofluorescence labeling of the endogenous tomosyn proteins, we examined the antibody’s immunofluorescent labeling of COS-7 cells transfected with tomosyn1 splice variants examined – s and m, and tomosyn2 splice variants s, m, b, and xb. Tomosyn2 isoforms expression levels as a result of the transfection in COS-7 cells were low (only 10% of the cells were successfully transfected), while transfection with tomosyn1 isoforms resulted with 50% successfully transfected cells. The antibody recognized all expressed isoforms (Figure 1A), while it did not recognize non-transfected cells (not shown). Next, we performed western blot analysis on COS-7 cells transfected with the same tomosyn isoforms. The affinity-purified anti-tomosyn rabbit polyclonal antibody specifically recognized all tomosyn isoforms (Figure 1B), though due to the low expression levels of tomosyn2 isoforms in the transfected COS-7 cells the labeling intensities for these isoforms were lower. In addition, results of the specificity verification concurred with the peptide against which the antibody was developed, which has a high degree of homology between all isoforms (Figure 1C). Hence, our antibody recognized both tomosyn1 and tomosyn2.
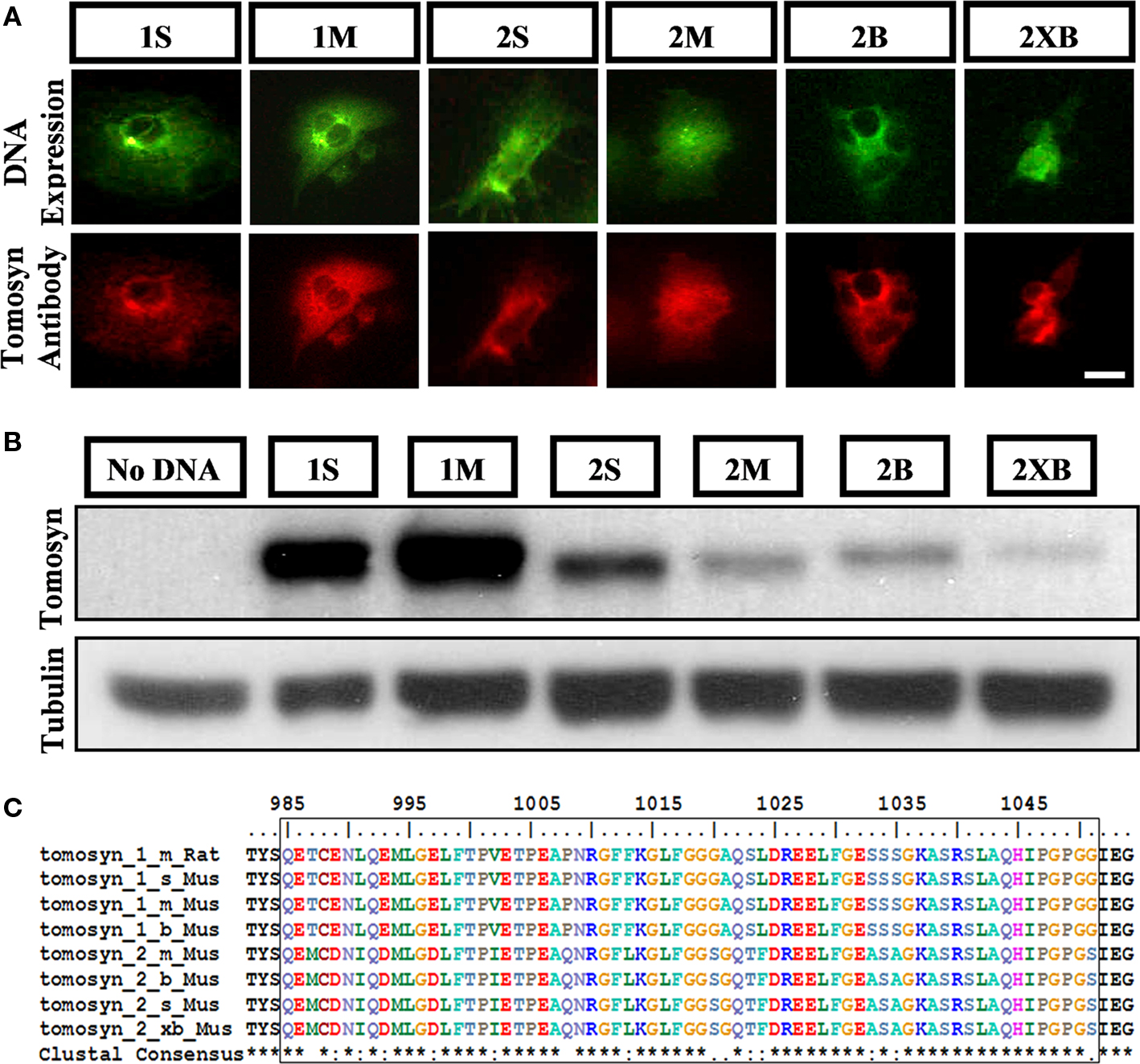
Figure 1. Rabbit anti-tomosyn antibody specifically recognizes all isoforms of tomosyn1 and tomosyn2. Detection of all tomosyn isoforms expressed in COS-7 cells by the affinity-purified anti-tomosyn rabbit polyclonal antibody in (A) immunocytochemistry of cells expressing citrine-tomosyn1 and citrine-tomosyn2 isoforms, labeled with goat anti-rabbit 546 secondary antibody, and in (B) western blot analysis of lysates from COS-7 cells transfected with tomosyn1 and tomosyn2 isoforms, and without DNA as negative control. (C) Multiple sequence alignment of tomosyn1 and tomosyn2 amino acid as aligned to residues 985-1051 of rat tomosyn1-m shows high degree of homology between tomosyn isoforms. The affinity-purified rabbit anti-tomosyn polyclonal antibody was raised against these residues. Scale bar = 5 μm.
Tomosyn Expression Pattern in the Mouse Hippocampus
Tomosyn immunostaining showed heterogeneous expression in the mouse hippocampus (Figure 2): interestingly, whereas in areas CA3 and granular cell bodies of the dentate gyrus, tomosyn immunostaining in the cell body layer was weak (Figure 2A), area CA2 showed very strong cytoplasmic immunostaining (Figures 2D,E). In areas that are well-characterized as synapse subfields, such as the molecular layer of the dentate gyrus, mossy fibers, and the stratum radiatum of CA1 (the dendritic subfield of CA1 pyramidal cells), tomosyn was differentially expressed, with highest expression levels in the mossy fibers and lower levels in the molecular layer of the dentate gyrus and stratum radiatum of CA1 (Figures 2A,C). No sex-based differences were observed – the same expression pattern was resolved in both male and female mice (n = 6 for males and females, not shown). In addition, the expression pattern remained the same in the different coronal hippocampal sections (not shown).
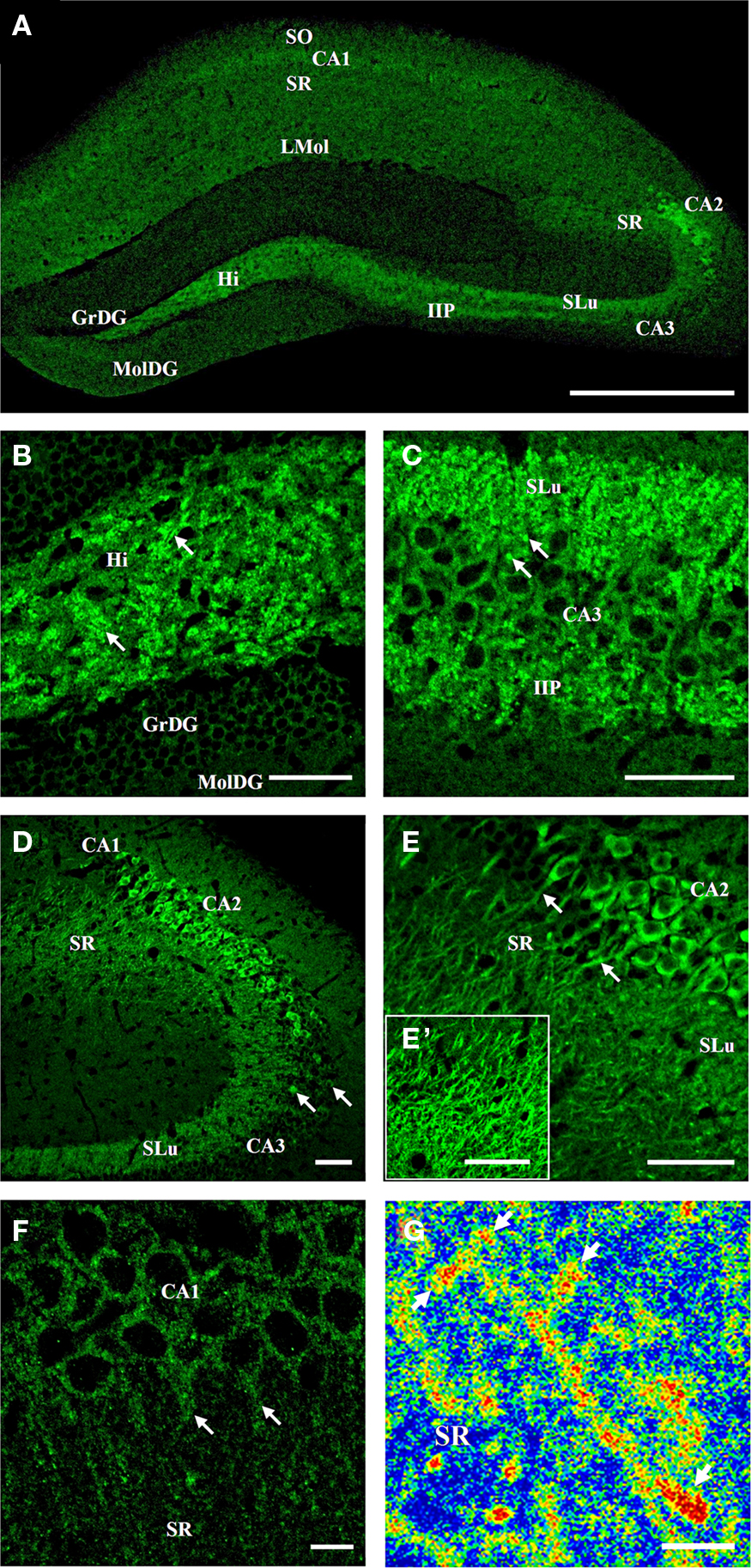
Figure 2. Tomosyn expression pattern in the mouse hippocampus. Immunofluorescence staining of coronal slices of mouse hippocampus. (A) General expression of tomosyn in the hippocampus: high levels can be seen in the hilus, mossy fibers, CA2 and CA1 pyramidal cells. (B) Hilus: high levels in a punctate pattern in the hilus (arrows). (C) Mossy fibers: high levels in the stratum lucidum and intra- and infrapyramidal of the mossy fibers, with mossy fiber terminals labeling (arrows). (D) CA3: low cytoplasmic levels in the CA3 pyramidal cells, with a few strongly labeled cells (arrows). (E) CA2: very high cytoplasmic levels in the CA2 pyramidal cells, with dendrites labeling in the stratum radiatum of CA2 (arrows). (E′) Stratum radiatum of CA2: high levels of tomosyn in dendrites. (F) CA1: low cytoplasmic levels in the CA1 pyramidal cells, with dendrites labeling in the stratum radiatum of CA1 (arrows). (G) High-resolution image taken with two-photon microscopy from the dendrites of CA2 pyramidal cells in the stratum radiatum of CA2 of tomosyn1-knockout mouse. Rainbow mode shows high levels (in red color) of tomosyn2 in spine-like structures along the dendrite (arrows). SO, stratum oriens; SR, stratum radiatum; LMol, stratum lacunosum-moleculare; SLu, stratum lucidum; IIP, intra- and infrapyramidal; Hi, hilus; GrDG, granular layer of dentate gyrus; MolDG, molecular layer of dentate gyrus. Scale bar: (A) = 500 μm, (B–E′) = 50 μm, (F) = 10 μm, (G) = 5 μm.
Dentate gyrus
Receiving direct major input from the entorhinal cortex through the perforant path, the dentate gyrus is considered the area in which the initial steps of information processing in the hippocampus take place, and it is well known for its high rate of neurogenesis (van Praag et al., 2002). The granular cells of the dentate gyrus send their axons through the hilus, forming synapses in the hilus area and in the CA3 region through the mossy fiber terminals. Only very weak cytoplasmic staining was noted in the granular cell bodies of the dentate gyrus (Figure 2B). In contrast, strong labeling in a punctate staining pattern was observed in the hilus and mossy fibers areas (Figures 2B,C, arrows, respectively), while in the dendrites area of the dentate gyrus, the molecular layer of the dentate gyrus, only very weak immunostaining was detected (Figures 2A,B).
Mossy fibers
Emerging from the basal portions of the granule cells in the dentate gyrus, the axons bundle connects the dentate gyrus with area CA3 through the mossy fiber terminals (Amaral and Witter, 1989). Mossy fibers innervate the dendrites of CA3 pyramidal cells in the stratum lucidum of the mossy fibers (Amaral and Witter, 1989), and synapse with inhibitory interneurons in the stratum lucidum of the mossy fibers and hilus of the dentate gyrus (Acsady et al., 1998). In the stratum lucidum of the mossy fibers area, very strong immunostaining of the mossy fibers was observed (Figures 2C,D). Strong immunostaining of tomosyn was noted in the intra- and infrapyramidal of the mossy fibers (Figure 2C). Numerous small islands of intense labeling were found in both stratum lucidum and intra- and infrapyramidal of the mossy fibers (Figure 2C, arrows). These data suggest that tomosyn is enriched in a punctate pattern in the stratum lucidum of the mossy fibers, which resembles the pattern of the mossy fiber terminals, and supports tomosyn’s presynaptic localization.
Areas CA2 and CA3
Due to its small dimensions, the CA2 area has unique properties and is highly connected with other subareas in the hippocampus and subregions of the brain (discussed further on). Distinguishing it from CA1 and CA3 areas, in the CA2 area, very strong cytoplasmic labeling of tomosyn was found in the cell bodies of the pyramidal cells, in addition to weak immunostaining in synapses around the CA2 pyramidal cells (Figures 2D,E). Moreover, in addition to the cell bodies, strong immunostaining was found in the dendrites emerging from the pyramidal cells in the stratum radiatum of CA2 (Figures 2E,E′, arrows, Figure 4D), where tomosyn-labeled dendrites were measured up to a distance of about 80 μm from the cell bodies. Importantly, these data strongly suggest tomosyn enrichment in the postsynaptic or dendritic elements of CA2 cells and indicate a possible postsynaptic function for tomosyn. Hence, endogenous tomosyn was observed both presynaptically in the mossy fiber terminals and in the CA2 dendritic tree.
The CA3 is defined as a highly interconnected and excitable network (Amaral and Witter, 1989). In contrast to the intense cytoplasmic staining of tomosyn in the CA2, the CA3 pyramidal cells showed very weak cytoplasmic labeling (Figures 2C,D), though several sparsely distributed cells showed much higher immunostaining, mainly in CA3a (Figure 2D, arrows). Thus, high cytoplasmic levels of tomosyn were detected only in the pyramidal cells of the CA2 area and not in the granular cell bodies of the dentate gyrus, CA1, or CA3 cell bodies.
Area CA1
CA1 pyramidal cells send their axons to the entorhinal cortex and subiculum through the stratum oriens of CA1, designating this area as a major hippocampus-output region. Through their dendrites in the stratum radiatum of CA1, the pyramidal cells of the CA1 receive input from the CA3 subfield, entorhinal cortex layer III, and more (Amaral and Witter, 1989). In CA1, tomosyn was mainly localized to the pyramidal cell cytoplasm, as reflected by weak immunostaining (Figure 2F). In addition, very weak tomosyn immunostaining was observed in the dendrites (stratum radiatum of CA1) and axons (stratum oriens of CA1): examining the stratum radiatum of CA1 under high magnification, immunostaining was localized to the dendrites coming from the CA1 pyramidal cells (Figure 2F, arrows), suggesting a possible postsynaptic role for tomosyn in CA1 as well. Distinct pattern of single-axon labeling was not observed in the axons coming out of the CA1 pyramidal cells to the stratum oriens of CA1, though overall immunostaining of this area was noted. Weak immunostaining was found in the stratum lacunosum-moleculare of CA1 (Figure 2A), whereas strong immunostaining was seen in the fasciola cinereum and subiculum (not shown).
In summary, tomosyn was highly concentrated in mossy fiber terminals and the hilus area, representing presynaptic localization, and in the subiculum, CA1 and CA2 cell bodies and dendrites (Figure 2), possibly representing a postsynaptic or a non-synaptic function of tomosyn.
Tomosyn2 is highly expressed in the CA2 area
To more specifically define the expression pattern of the two tomosyn genes in the mouse hippocampus, we compared immunostaining intensity in the hippocampus subareas of wild-type, tomosyn1-heterozygous, and tomosyn1-knockout mice (Sakisaka et al., 2008) using the same affinity-purified rabbit anti-tomosyn antibody. As expected, tomosyn immunostaining intensity in the hilus, mossy fibers, and CA1 subareas gradually decreased (highest intensity in the wild-type and no tomosyn immunostaining in the tomosyn1-knockout mice), suggesting that tomosyn1 is the main isoform in the mossy fiber synapses and CA1 area (Figure 3). In contrast, in the CA2 area and the stratum radiatum of CA2, the immunostaining intensity in the tomosyn1-knockout mice was similar to the wild-type, suggesting that this area is enriched with tomosyn2 isoforms. In addition, in the CA1 and the subiculum, tomosyn2 was observed in the cell bodies as well as in the dendrites (not shown). To further improve the characterization of tomosyn in the dendrites located in the stratum radiatum of CA2, we used two-photon high-resolution microscopy to examine whether tomosyn is located in the dendritic spines. Examining brain slices derived from tomosyn1-knockout mice, tomosyn2 immunostaining was observed in several dendritic structures that resembled spines along the CA2 pyramidal cells’ dendrites (Figure 2G, arrows). These results agree with the mRNA distribution of tomosyn, which shows high levels of tomosyn2 in the CA2 area (Groffen et al., 2005). However, while tomosyn1 is highly expressed in the presynaptic area, tomosyn2 is highly expressed in the cell bodies and dendrites, suggesting a postsynaptic or non-synaptic-dendritic role for this isoform.
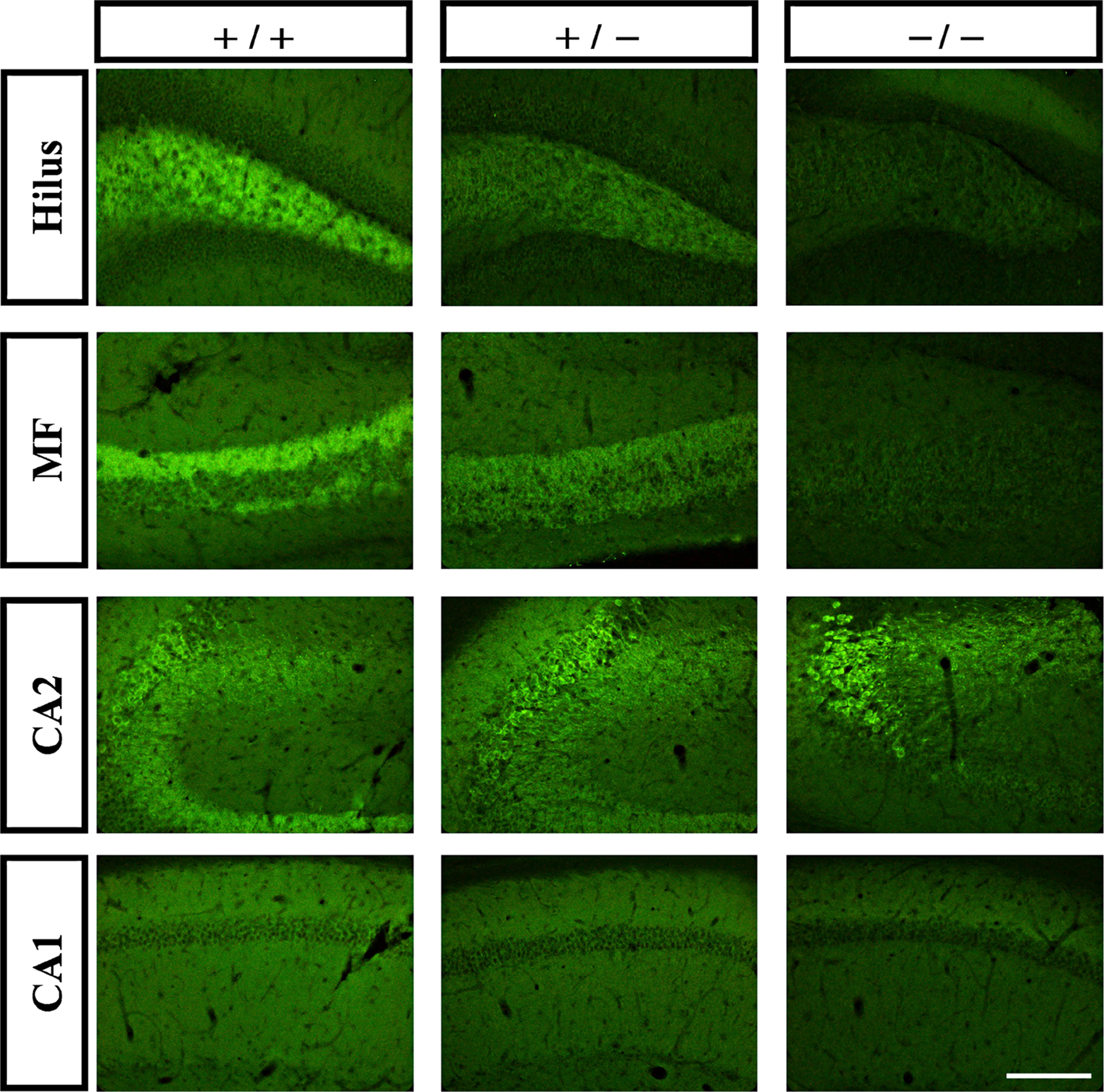
Figure 3. Tomosyn2 is highly expressed in the CA2 area. Immunofluorescence staining of coronal slices of hippocampus subareas of wild-type (+/+), tomosyn1-heterozygous (+/−) and tomosyn1-knockout mice (−/−). Gradual decrease in immunostaining intensity in hilus, mossy fibers and CA1, but not in CA2, suggests high levels of tomosyn1 in mossy fiber terminals and tomosyn2 in subarea CA2. Scale bar = 250 μm.
Tomosyn Colocalization with Synaptic and Neuronal Markers in the Mouse Hippocampus
Having characterized tomosyn’s localization and expression in the mouse hippocampus, a series of double-labeling experiments were performed with tomosyn and specific synaptic markers (synaptophysin, VGluT1, VGAT, GAD67, PSD95) or the dendritic marker MAP2.
Synaptophysin
Synaptophysin is a vesicular transmembrane protein ubiquitously expressed in all synapses, and is therefore the ideal marker for synapses (Navone et al., 1986). To examine tomosyn localization in synapses in the hippocampus, we examined tomosyn and synaptophysin colocalization. While overall similar marker patterns were resolved in synapse subareas using anti-tomosyn and anti-synaptophysin antibodies, a few subareas showed much stronger colocalization.
In the dentate gyrus, strong colocalization between tomosyn and synaptophysin (Pearson’s correlation 0.758, see Materials and Methods) was observed in the hilus, in a punctate pattern (not shown). Similarly, in the mossy fibers, strong colocalization (Pearson’s correlation 0.781) in a similar punctate pattern was observed in both stratum lucidum (Figures 4A and 5A) and intra- and infrapyramidal of the mossy fibers (not shown). However, whereas in the hilus the punctae were small, those in the mossy fibers were bigger, and localized mainly in proximity to the pyramidal cells of CA3. These punctae represent mossy fiber terminals, demonstrating strong presynaptic localization of tomosyn in these terminals.
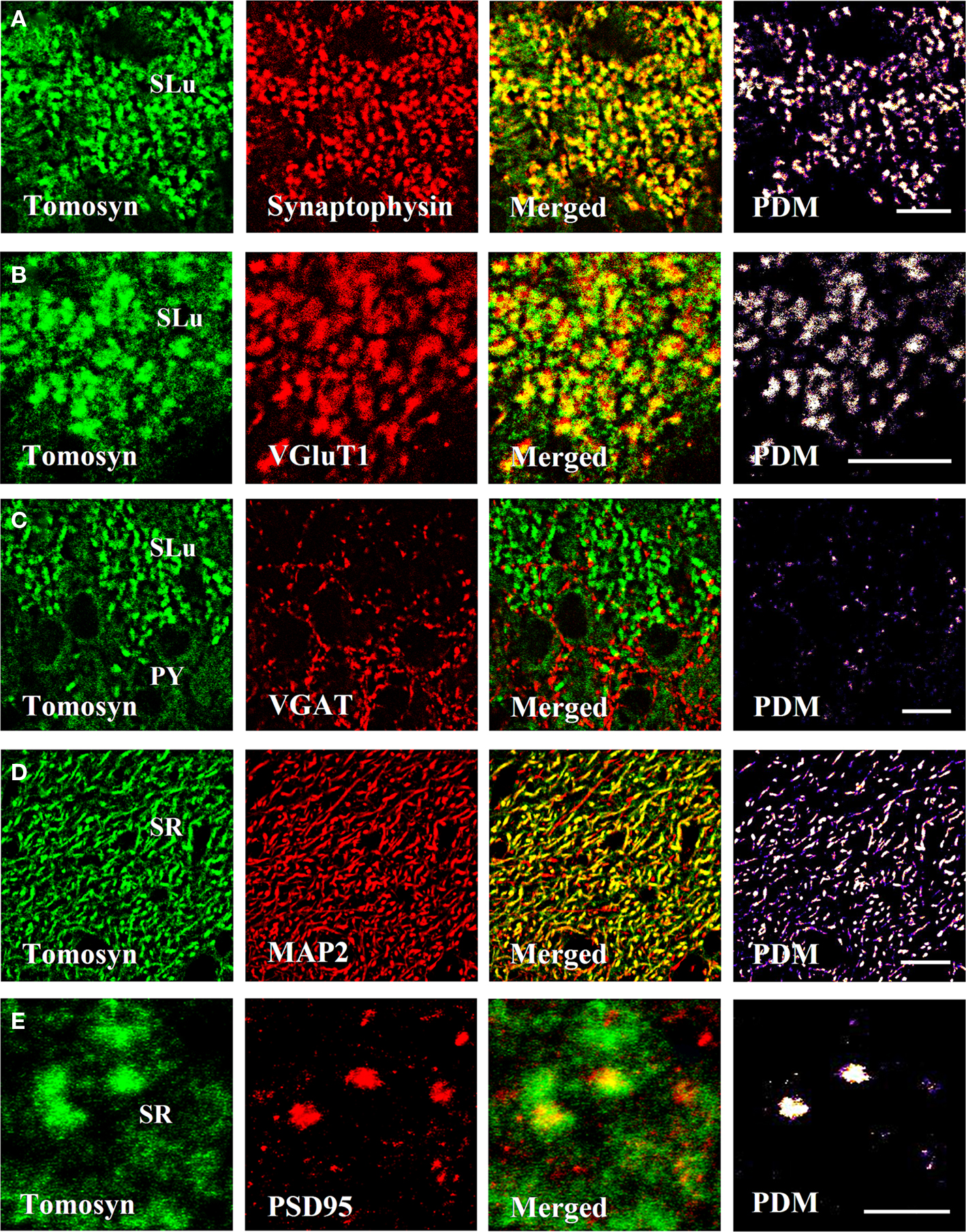
Figure 4. Tomosyn colocalizes with synaptic and dendritic markers in the mouse hippocampus. Immunofluorescence double-labeling of coronal slices of mouse hippocampus. (A) Tomosyn colocalization with synaptophysin in the stratum lucidum of the mossy fibers. (B) Tomosyn colocalization with VGluT1 in the stratum lucidum of the mossy fibers. (C) Tomosyn colocalization with VGAT in the stratum lucidum of the mossy fibers. (D) Tomosyn colocalization with MAP2 in the stratum radiatum of CA2. (E) Tomosyn colocalization with PSD95 in the stratum radiatum of CA2. PDM, positive product of the differences from the mean; SR, stratum radiatum of CA2; SLu, stratum lucidum of the mossy fibers; IIP, intra- and infrapyramidal of the mossy fibers; Hi, hilus; PY, CA3 pyramidal cells. In positive PDM images, bright spots represent colocalization. Scale bar: (A–D) = 10 μm, (E) = 5 μm.
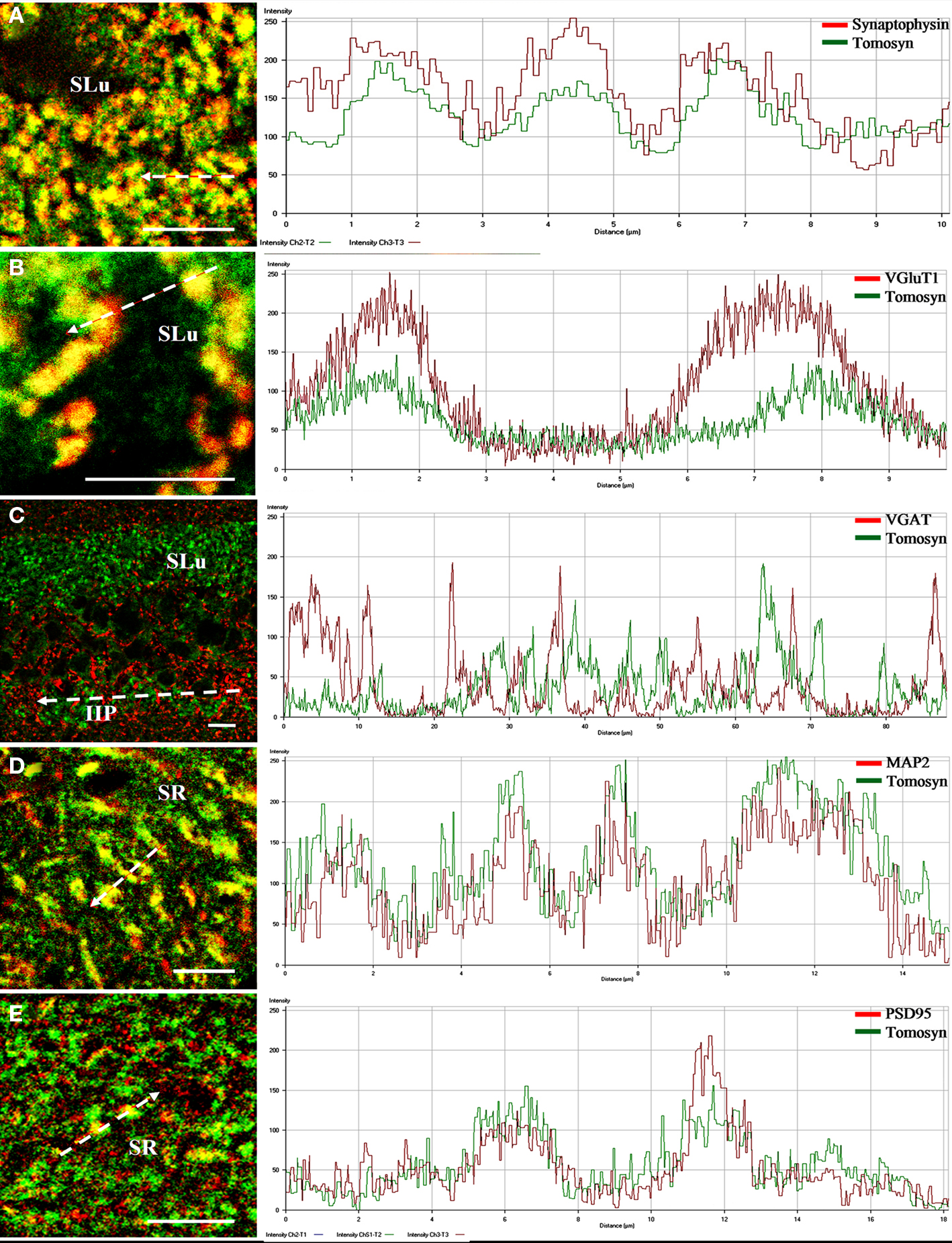
Figure 5. Intensity profile graphs of tomosyn colocalization with synaptic and dendritic markers in the mouse hippocampus. Line scan graphs showing the immunofluorescence intensity along the freely positioned arrow of tomosyn and (A) synaptophysin in the stratum lucidum of the mossy fibers, (B) VGluT1 in the stratum lucidum of the mossy fibers, (C) VGAT in the stratum lucidum and intra- and infrapyramidal of the mossy fibers, (D) MAP2 in the stratum radiatm of CA2, (E) PSD95 in the stratum radiatum of CA2. SR, stratum radiatum of CA2; SLu, stratum lucidum of the mossy fibers; IIP, intra- and infrapyramidal of the mossy fibers. Scale bar = 10 μm.
As tomosyn immunostaining was significantly reduced to background levels in the tomosyn1-knockout mice, we suggest that tomosyn1 is the main tomosyn isoform expressed presynaptically in the mossy fiber terminals, although low levels of tomosyn2 cannot be ruled out. No colocalization was observed in other subareas of the hippocampus. Whereas for tomosyn labeling the cytoplasmic pattern in the CA2 pyramidal cell bodies was very strong, no such pattern was noted when labeling with the synaptophysin antibody, a finding that led us to examine colocalization with dendritic and postsynaptic markers in this area.
Vesicular glutamate transporter 1
Vesicular glutamate transporter 1 (VGluT1) is a glutamate transporter located in the membrane of synaptic vesicles, and it is therefore a marker for nerve terminals of excitatory neurons and excitatory synapses (Ziegler et al., 2002). In the hilus, stratum lucidum and intra- and infrapyramidal of the mossy fibers, very strong colocalization (stratum lucidum of the mossy fibers, Pearson’s correlation 0.71) was observed, showing a punctate pattern at many sites (Figures 4B and 5B). As in the case of colocalization with synaptophysin, punctae in the hilus were small, and those in the mossy fibers were bigger. No colocalization was observed in other subareas of the hippocampus. These data suggest that tomosyn is highly enriched in glutamatergic synapses in subareas of the hippocampus.
Vesicular GABA transporter and GAD67
Vesicular GABA transporter (VGAT) is a GABA transporter located in the membrane of synaptic vesicles, and it is therefore a marker for nerve terminals of inhibitory neurons and inhibitory synapses (Chaudhry et al., 1998). While VGAT exhibited a typical inhibitory staining pattern, no colocalization was detected with tomosyn in any subareas of the mouse hippocampus (stratum lucidum of the mossy fibers, Pearson’s correlation −0.32; Figures 4C and 5C). Moreover, same results were measured with GAD67, an additional marker for inhibitory neurons and synapses (not shown).
Microtubule-associated protein 2
Microtubule-associated protein 2 (MAP2) is a neuron-specific protein involved in stabilizing its microtubule cytoskeleton, and it is enriched in dendrites (Goedert et al., 1991). To examine whether tomosyn is localized in dendrites and has a non-synaptic possible role, we examined tomosyn and MAP2 colocalization. Very strong cytoplasmic colocalization was observed in the pyramidal cells of CA2, as well as in the dendrites in the stratum radiatum of CA2 (Pearson’s correlation 0.701; Figures 4D and 5D). Moderate colocalization (Pearson’s correlation 0.196) was observed in the pyramidal cells of CA1 and their dendrites in the stratum radiatum of CA1, and in the subiculum (not shown). No colocalization was observed in the hilus (Pearson’s correlation −0.036) and the mossy fibers (Pearson’s correlation −0.112). To distinguish between the two tomosyn genes, we examined the colocalization of tomosyn and MAP2 in tomosyn1-knockout mice. The measured pattern and levels were the same as those described above in tomosyn1-knockout mice. These data confirm our findings that tomosyn2 is localized in the dendrites of the pyramidal cells of the subiculum, CA1 and CA2, and indicate an additional neuronal function for tomosyn2 in the hippocampus.
Postsynaptic density protein, 95 kDa
Postsynaptic density protein, 95 kDa (PSD95) is one of the components of excitatory postsynaptic complexes located in the central synapses (Gundelfinger and tom Dieck, 2000). To determine whether tomosyn is also located postsynaptically in excitatory synapses, we examined tomosyn and PSD95 colocalization. Moderate colocalization (Pearson’s correlation 0.281) was observed in the spines in the stratum radiatum of CA2 (Figures 4E and 5E) and of the CA1 and subiculum (not shown). No colocalization was observed in the hilus (Pearson’s correlation −0.042) and the mossy fibers (Pearson’s correlation −0.124). Similar colocalization of tomosyn and PSD95 was also observed in tomosyn1-knockout mice. These data confirm our findings that tomosyn2 is localized in the spines of the pyramidal cells of the subiculum, CA1 and CA2, and indicate a postsynaptic function for tomosyn2 in the hippocampus.
Discussion
A previous report examining the expression pattern of tomosyn1 and tomosyn2 mRNA in the mouse central nervous system showed non-homogenous distribution of tomosyn isoforms in the cell bodies in the hippocampus (Groffen et al., 2005). However, in situ hybridization does not give any indication of the expression levels of the resulting proteins nor their subcellular localization. Despite tomosyn’s essential role in regulating synaptic transmission, its distribution in the mouse hippocampus has never been extensively studied. Here we provide the first detailed description of the localization and expression levels of tomosyn1 and tomosyn2 in the mouse hippocampus. In addition, using subcellular colocalization with several synaptic and neuronal markers, we identify tomosyn gene expression with specific pre- and postsynaptic elements within the hippocampus. This high-resolution characterization of tomosyn distribution is crucial to elucidate its contribution to hippocampus physiology. Using confocal and two-photon high-resolution microscopy, we show that tomosyn is differentially distributed in the mouse hippocampus, with its main concentration in the hilus, mossy fibers, pyramidal cells of CA1, and, with a markedly high cytoplasmic concentration in the pyramidal cells of CA2. We further show that although tomosyn1 is located mostly in presynaptic terminals in the mossy fiber, tomosyn2 is mainly expressed in the CA2 pyramidal cells as well as their dendrites and spines, suggesting an intriguing postsynaptic function for this isoform in regulating synaptic transmission.
The main input of the hippocampus comes from the entorhinal cortex through the perforant path to the dentate gyrus, and from the dentate gyrus information is transferred through the mossy fibers to area CA3 (Andersen et al., 1969). In addition, the mossy fiber synapses proximally on dentate gyrus basket cells and pyramidal-like neurons in the hilus (Amaral and Witter, 1989). Given the fact that tomosyn is a modulator of synaptic transmission (Fujita et al., 1998; Yizhar et al., 2004, 2007; Gracheva et al., 2006; Sakisaka et al., 2008; Ashery et al., 2009), and our findings showing high levels of tomosyn1 in the mossy fibers, we suggest that tomosyn1 plays an important role in the physiology of mossy fiber terminals. Mossy fiber terminals are known for their low release probability, high paired-pulse facilitation, and high frequency-dependent facilitation. As tomosyn is known to reduce release probability, the high levels of tomosyn1 in mossy fibers might explain this low release probability. Indeed, tomosyn1-knockout mice exhibit an increase in release probability that is reflected in a decrease in paired-pulse facilitation (Sakisaka et al., 2008). Therefore, tomosyn1 may act as a regulator of release probability in this synapse.
Surprisingly, very high levels of tomosyn2 were found in the CA2 pyramidal cells, where, in addition to its cytoplasmic localization, long segments of dendrites extending to the stratum radiatum of CA2 were labeled as well. Though usually ignored due to its small dimensions and barely detectable borders, the CA2 area has unique characteristics, such as its specific expression of fibroblast growth factor 2 (Williams et al., 1996), as well as its unique resistance to seizure generation (Sloviter, 1983). Worthy of note are the facts that (i) the CA2 region receives projections from the hypothalamic supramammillary nucleus region (Haglund et al., 1984; Vertes and McKenna, 2000); (ii) the CA3 pyramidal cells send their connections to CA1 via schaffer collateral through the CA2 subarea (Sekino et al., 1997); (iii) the CA2 pyramidal cells receive input directly from the entorhinal cortex (Bartesaghi et al., 2006); (iv) it has been suggested that mossy fibers may taper into the CA2 region (Mercer et al., 2007). These data suggest that the CA2 subarea is a major junction for the input and output of information transferred through the hippocampus. Interestingly, we observed high concentrations of tomosyn2 in CA2 pyramidal cells and of tomosyn1 in the mossy fiber terminals innervating this area. Moreover, tomosyn2 colocalizes with MAP2 and PSD95 in the stratum radiatum of CA2, suggesting dendritic and/or postsynaptic roles for tomosyn2 in the CA2 region. As such, tomosyn might regulate synaptic transmission in this important junction both pre- and postsynaptically.
Combining the high levels of tomosyn mRNA that were measured in the granule cell bodies of the dentate gyrus (Groffen et al., 2005) with our finding that tomosyn levels are higher in the hilus and mossy fibers than in the granular cell bodies, we suggest that following synthesis in the granular cell bodies, tomosyn is efficiently transported to the hilus and mossy fiber synapses. In addition, tomosyn’s colocalization with synaptophysin supports the notion that tomosyn is directed to synapse sites in the hilus and the mossy fibers. While strong colocalization of tomosyn and VGluT1 was observed in the hilus and mossy fibers, no colocalization was observed with VGAT or GAD67. These data suggest that tomosyn is highly enriched in glutamatergic synapses in the mouse hippocampus. Moreover, it has been shown that in tomosyn1-knockout mice, synaptic efficacy at the mossy fiber synapses is enhanced (Sakisaka et al., 2008), strengthening our suggestion of tomosyn’s role in the mossy fibers as a synaptic transmission modulator. Since tomosyn1 was observed also in areas showing no overlapping with synaptophysin in the hilus and stratum lucidum of the mossy fibers, it is possible that tomosyn1 has also a non-synaptic role in these areas.
It was previously shown that in the cerebellar molecular layer, tomosyn was equally localized pre- and postsynaptically (Fujita et al., 1998). Nevertheless, in the following years tomosyn was mainly related as a presynaptic regulator of neurotransmission, and no adequate attention was given to its postsynaptic localization in general, and specifically in the hippocampus. Therefore, of particular interest is our novel finding that tomosyn2 might function postsynaptically and have a non-synaptic role in the hippocampus. This interpretation is strengthened by our data demonstrating colocalization of tomosyn2 with MAP2 and PSD95 in several subareas of the hippocampus. Recent publications suggest that insertion of AMPA and NMDA receptors, which are necessary for LTP, is inhibited by a SNARE-dependent mechanism postsynaptically (Lu et al., 2001; Kwon and Castillo, 2008). It is therefore possible that tomosyn2 inhibits LTP postsynaptically in CA1 and CA2 pyramidal cells by inhibiting SNARE-dependent AMPA-R/NMDA-R insertion. Thus a new and exciting function is postulated for tomosyn2 as a postsynaptic regulator of AMPA-R/NMDA-R insertion. However, this possibility needs to be further explored. As was discussed before, in addition to its high cytoplasmic expression, high levels of tomosyn2 were observed in the long segments of dendrites of CA2 pyramidal cells. This is opposite to the expression pattern found in the granular cell bodies of the dentate gyrus and mossy fibers, where tomosyn is highly expressed in the mossy fiber extensions, with low levels in the soma and dendrites of these cells.
These two different patterns suggest that tomosyn isoforms can be differentially transported to different neuronal subareas, depending on the isoform and the type of neuron in which it is expressed.
In general, this study’s findings improve our understanding of tomosyn’s mode of action and expression in subareas of the mouse hippocampus, and provide important information on its functional role in controlling synaptic transmission. According to the role of tomosyn in synaptic transmission regulation, our results show that some subareas of the hippocampus express higher concentrations of tomosyn than others, suggesting a higher degree of regulation in these subareas. Moreover, while tomosyn1 is mainly localized in presynaptic terminals, tomosyn2 is concentrated in postsynaptic elements. Further investigation is therefore warranted to define the expression and localization of different tomosyn isoforms and their involvement in synaptic plasticity processes in the hippocampus.
Conflict of Interest Statement
The authors declare that the research was conducted in the absence of any commercial or financial relationships that could be construed as a potential conflict of interest.
Acknowledgments
We thank Dr. E. F. Indig, Dr. M. Shaharabany and Dr. E. Krause for their technical help. Tomosyn1-knockout mice were kindly provided by Prof. Y. Takai, Kobe University and Prof. J. Miyoshi, Osaka, Japan. This work was supported by grants from the Israel Science Foundation (Grant no. 1211/07; Uri Ashery) and the National Institutes of Health (RO1 NS053978; Edward L. Stuenkel and Uri Ashery).
References
Acsady, L., Kamondi, A., Sik, A., Freund, T., and Buzsaki, G. (1998). GABAergic cells are the major postsynaptic targets of mossy fibers in the rat hippocampus. J. Neurosci. 18, 3386–3403.
Aggleton, J. P., and Brown, M. W. (1999). Episodic memory, amnesia, and the hippocampal-anterior thalamic axis. Behav. Brain Sci. 22, 425–444; discussion 444–489.
Amaral, D. G., and Witter, M. P. (1989). The three-dimensional organization of the hippocampal formation: a review of anatomical data. Neuroscience 31, 571–591.
Andersen, P., Bliss, T. V., Lomo, T., Olsen, L. I., and Skrede, K. K. (1969). Lamellar organization of hippocampal excitatory pathways. Acta Physiol. Scand. 76, 4A–5A.
Ashery, U., Bielopolski, N., Barak, B., and Yizhar, O. (2009). Friends and foes in synaptic transmission: the role of tomosyn in vesicle priming. Trends Neurosci. 32, 275–282.
Baba, T., Sakisaka, T., Mochida, S., and Takai, Y. (2005). PKA-catalyzed phosphorylation of tomosyn and its implication in Ca2+-dependent exocytosis of neurotransmitter. J. Cell Biol. 170, 1113–1125.
Bartesaghi, R., Migliore, M., and Gessi, T. (2006). Input–output relations in the entorhinal cortex-dentate-hippocampal system: evidence for a non-linear transfer of signals. Neuroscience 142, 247–265.
Becherer, U., and Rettig, J. (2006). Vesicle pools, docking, priming, and release. Cell Tissue Res. 326, 393–407.
Breustedt, J., Gundlfinger, A., Varoqueaux, F., Reim, K., Brose, N., and Schmitz, D. (2010). Munc13-2 differentially affects hippocampal synaptic transmission and plasticity. Cereb. Cortex 20, 1109–1120.
Castillo, P. E., Janz, R., Sudhof, T. C., Tzounopoulos, T., Malenka, R. C., and Nicoll, R. A. (1997). Rab3A is essential for mossy fibre long-term potentiation in the hippocampus. Nature 388, 590–593.
Castillo, P. E., Schoch, S., Schmitz, F., Sudhof, T. C., and Malenka, R. C. (2002). RIM1alpha is required for presynaptic long-term potentiation. Nature 415, 327–330.
Chaudhry, F. A., Reimer, R. J., Bellocchio, E. E., Danbolt, N. C., Osen, K. K., Edwards, R. H., and Storm-Mathisen, J. (1998). The vesicular GABA transporter, VGAT, localizes to synaptic vesicles in sets of glycinergic as well as GABAergic neurons. J. Neurosci. 18, 9733–9750.
Franklin, K. B. J., and Paxinos, G. (1997). Mouse Brain in Stereotaxic Coordinates. San Diego: Academic Press.
Fujita, Y., Shirataki, H., Sakisaka, T., Asakura, T., Ohya, T., Kotani, H., Yokoyama, S., Nishioka, H., Matsuura, Y., Mizoguchi, A., Scheller, R. H., and Takai, Y. (1998). Tomosyn: a syntaxin-1-binding protein that forms a novel complex in the neurotransmitter release process. Neuron 20, 905–915.
Geinisman, Y. (2000). Structural synaptic modifications associated with hippocampal LTP and behavioral learning. Cereb. Cortex 10, 952–962.
Geinisman, Y., Disterhoft, J. F., Gundersen, H. J., McEchron, M. D., Persina, I. S., Power, J. M., van der Zee, E. A., and West, M. J. (2000). Remodeling of hippocampal synapses after hippocampus-dependent associative learning. J. Comp. Neurol. 417, 49–59.
Goedert, M., Crowther, R. A., and Garner, C. C. (1991). Molecular characterization of microtubule-associated proteins tau and MAP2. Trends Neurosci. 14, 193–199.
Gonnet, G. H., Cohen, M. A., and Benner, S. A. (1992). Exhaustive matching of the entire protein sequence database. Science 256, 1443–1445.
Gracheva, E. O., Burdina, A. O., Holgado, A. M., Berthelot-Grosjean, M., Ackley, B. D., Hadwiger, G., Nonet, M. L., Weimer, R. M., and Richmond, J. E. (2006). Tomosyn inhibits synaptic vesicle priming in Caenorhabditis elegans. PLoS Biol. 4, e261. doi: 10.1371/journal.pbio.0040261
Groffen, A. J., Jacobsen, L., Schut, D., and Verhage, M. (2005). Two distinct genes drive expression of seven tomosyn isoforms in the mammalian brain, sharing a conserved structure with a unique variable domain. J. Neurochem. 92, 554–568.
Gundelfinger, E. D., and tom Dieck, S. (2000). Molecular organization of excitatory chemical synapses in the mammalian brain. Naturwissenschaften 87, 513–523.
Haglund, L., Swanson, L. W., and Kohler, C. (1984). The projection of the supramammillary nucleus to the hippocampal formation: an immunohistochemical and anterograde transport study with the lectin PHA-L in the rat. J. Comp. Neurol. 229, 171–185.
Hatsuzawa, K., Lang, T., Fasshauer, D., Bruns, D., and Jahn, R. (2003). The R-SNARE motif of tomosyn forms SNARE core complexes with syntaxin 1 and SNAP-25 and down-regulates exocytosis. J. Biol. Chem. 278, 31159–31166.
Kwon, H. B., and Castillo, P. E. (2008). Long-term potentiation selectively expressed by NMDA receptors at hippocampal mossy fiber synapses. Neuron 57, 108–120.
Li, Q., Lau, A., Morris, T. J., Guo, L., Fordyce, C. B., and Stanley, E. F. (2004). A syntaxin 1, Galpha(o), and N-type calcium channel complex at a presynaptic nerve terminal: analysis by quantitative immunocolocalization. J. Neurosci. 24, 4070–4081.
Lu, W., Man, H., Ju, W., Trimble, W. S., MacDonald, J. F., and Wang, Y. T. (2001). Activation of synaptic NMDA receptors induces membrane insertion of new AMPA receptors and LTP in cultured hippocampal neurons. Neuron 29, 243–254.
Mercer, A., Trigg, H. L., and Thomson, A. M. (2007). Characterization of neurons in the CA2 subfield of the adult rat hippocampus. J. Neurosci. 27, 7329–7338.
Mumby, D. G., Astur, R. S., Weisend, M. P., and Sutherland, R. J. (1999). Retrograde amnesia and selective damage to the hippocampal formation: memory for places and object discriminations. Behav. Brain Res. 106, 97–107.
Navone, F., Jahn, R., Di Gioia, G., Stukenbrok, H., Greengard, P., and De Camilli, P. (1986). Protein p38: an integral membrane protein specific for small vesicles of neurons and neuroendocrine cells. J. Cell Biol. 103, 2511–2527.
Powell, C. M. (2006). Gene targeting of presynaptic proteins in synaptic plasticity and memory: across the great divide. Neurobiol. Learn. Mem. 85, 2–15.
Reilly, C. E. (2001). Hippocampus selectively supports episodic memory retrieval. J. Neurol. 248, 1014–1015.
Richmond, J. E., and Broadie, K. S. (2002). The synaptic vesicle cycle: exocytosis and endocytosis in Drosophila and C. elegans. Curr. Opin. Neurobiol. 12, 499–507.
Sakisaka, T., Yamamoto, Y., Mochida, S., Nakamura, M., Nishikawa, K., Ishizaki, H., Okamoto-Tanaka, M., Miyoshi, J., Fujiyoshi, Y., Manabe, T., and Takai, Y. (2008). Dual inhibition of SNARE complex formation by tomosyn ensures controlled neurotransmitter release. J. Cell Biol. 183, 323–337.
Sekino, Y., Obata, K., Tanifuji, M., Mizuno, M., and Murayama, J. (1997). Delayed signal propagation via CA2 in rat hippocampal slices revealed by optical recording. J. Neurophysiol. 78, 1662–1668.
Sloviter, R. S. (1983). “Epileptic” brain damage in rats induced by sustained electrical stimulation of the perforant path. I. Acute electrophysiological and light microscopic studies. Brain Res. Bull. 10, 675–697.
van Praag, H., Schinder, A. F., Christie, B. R., Toni, N., Palmer, T. D., and Gage, F. H. (2002). Functional neurogenesis in the adult hippocampus. Nature 415, 1030–1034.
Verhage, M., and Sorensen, J. B. (2008). Vesicle docking in regulated exocytosis. Traffic 9, 1414–1424.
Vertes, R. P., and McKenna, J. T. (2000). Collateral projections from the supramammillary nucleus to the medial septum and hippocampus. Synapse 38, 281–293.
Williams, T. E., Meshul, C. K., Cherry, N. J., Tiffany, N. M., Eckenstein, F. P., and Woodward, W. R. (1996). Characterization and distribution of basic fibroblast growth factor-containing cells in the rat hippocampus. J. Comp. Neurol. 370, 147–158.
Yizhar, O., Lipstein, N., Gladycheva, S. E., Matti, U., Ernst, S. A., Rettig, J., Stuenkel, E. L., and Ashery, U. (2007). Multiple functional domains are involved in tomosyn regulation of exocytosis. J. Neurochem. 103, 604–616.
Yizhar, O., Matti, U., Melamed, R., Hagalili, Y., Bruns, D., Rettig, J., and Ashery, U. (2004). Tomosyn inhibits priming of large dense-core vesicles in a calcium-dependent manner. Proc. Natl. Acad. Sci. U.S.A. 101, 2578–2583.
Keywords: tomosyn isoforms, hippocampus, mossy fiber terminals, CA2 area, synaptic protein
Citation: Barak B, Williams A, Bielopolski N, Gottfried I, Okun E, Brown MA, Matti U, Rettig J, Stuenkel EL and Ashery U (2010) Tomosyn expression pattern in the mouse hippocampus suggests both presynaptic and postsynaptic functions. Front. Neuroanat. 4:149. doi: 10.3389/fnana.2010.00149
Received: 16 September 2010;
Accepted: 30 November 2010;
Published online: 20 December 2010.
Edited by:
Alfonso Fairén, University Miguel Hernandez, SpainReviewed by:
Janet E. Richmond, University of Illinois-Chicago, USAAlan Morgan, University of Liverpool, UK
Copyright: © 2010 Barak, Williams, Bielopolski, Gottfried, Okun, Brown, Matti, Rettig, Stuenkel and Ashery. This is an open-access article subject to an exclusive license agreement between the authors and the Frontiers Research Foundation, which permits unrestricted use, distribution, and reproduction in any medium, provided the original authors and source are credited.
*Correspondence: Uri Ashery, Department of Neurobiology, The George S. Wise Faculty of Life Sciences, Tel-Aviv University, Sherman Building Room 719, Tel Aviv 69978, Israel. e-mail: uria@post.tau.ac.il