Evaluating the Effect of Non-cellular Bioactive Glass-Containing Scaffolds on Osteogenesis and Angiogenesis in in vivo Animal Bone Defect Models
- 1Cambridge Clinical School, University of Cambridge, Cambridge, United Kingdom
- 2Division of Trauma and Orthopaedics, Department of Surgery, Addenbrooke's Hospital, University of Cambridge, Cambridge, United Kingdom
The use of bone scaffolds to replace injured or diseased bone has many advantages over the currently used autologous and allogeneic options in clinical practice. This systematic review evaluates the current evidence for non-cellular scaffolds containing bioactive glass on osteogenesis and angiogenesis in animal bone defect models. Studies that reported results of osteogenesis via micro-CT and results of angiogenesis via Microfil perfusion or immunohistochemistry were included in the review. A literature search of PubMed, EMBASE and Scopus was carried out in November 2019 from which nine studies met the inclusion and exclusion criteria. Despite the significant heterogeneity in the composition of the scaffolds used in each study, it could be concluded that scaffolds containing bioactive glass improve bone regeneration in these models, both by osteogenic and angiogenic measures. Incorporation of additional elements into the glass network, using additives, and using biochemical factors generally had a beneficial effect. Comparing the different compositions of non-cellular bioactive glass containing scaffolds is however difficult due to the heterogeneity in bioactive glass compositions, fabrication methods and biochemical additives used.
Introduction
Bones are composed of a dense connective tissue and they serve a large variety of functions. These include mechanical functions such as internal organ protection, synthetic functions such as hematopoiesis within the bone marrow and metabolic functions such as acting as reservoirs for minerals (Taichman, 2005). Thus, the repair of bone defects caused by trauma, infection and congenital abnormalities is important (Zhao et al., 2015). Many methods to achieve regeneration have been investigated, including the “gold standard” autologous bone grafting, allograft implantation, or autologous bone transplantation. However, these methods are not without their limitations, especially with regards to cost-effectiveness and even efficacy (Dimitriou et al., 2011). One of the alternative methods proposed is to focus on regenerating the tissue through tissue engineering, rather than replacing it (Langer, 2000). Commonly in tissue engineering, templates or “scaffolds” are used to provide an appropriate environment for this tissue regeneration (O'Brien, 2011). Scaffolds can be seeded with cells and growth factors in order to aid this process.
The ideal properties of a scaffold have been described previously (O'Brien, 2011). Firstly, the scaffold must be biocompatible, allowing for cells to migrate through the scaffold and lay down new matrix. They must elicit a negligible immune reaction to prevent any inflammatory process from reducing healing of the bone. They should also be biodegradable, with degradation products being non-toxic. Having both mechanical properties and sufficient porosity to function adequately from the implantation to remodeling stage is also important. An interconnected pore structure within the scaffold is crucial to facilitate the penetration of cells and diffusion of nutrients. Research suggests that the minimum pore size for bone regeneration is 100 μm (Wagoner Johnson and Herschler, 2011).
Other properties described (Wagoner Johnson and Herschler, 2011) include the ability to serve as a template for bone formation by allowing cells to adhere and proliferate (osteoconductivity). They should also be able to bind with surrounding bone tissue (bioactivity) and ideally have the ability to induce bone formation (osteoinductivity). Furthermore, a strong correlation between decreased vascular function and failed bone healing has been described in the literature previously (Dickson et al., 1994). The importance of re-establishing an adequate blood vessel system has been comprehensively reviewed previously (Stegen et al., 2015). Indeed, the low survival of osteogenic cells after implantation of the scaffold in the early stages of bone regeneration has been attributed to an inadequate rate of blood vessel invasion into the scaffold (Giannoni et al., 2010; Stegen et al., 2015). Thus, scaffolds that have potential to provide better angiogenesis are also sought after (Rouwkema and Khademhosseini, 2016).
A plethora of scaffolds made from different biomaterial groups have been investigated in the field, including but not limited to calcium phosphate bioactive ceramic scaffolds, polymeric scaffolds, composite scaffolds, and metallic scaffolds (Bose et al., 2012). Bioactive glass scaffolds have also recently gained attention. The first bioactive glass introduced was the 45S5 Bioglass® introduced by Hench et al. (1971) and was composed of a quaternary SiO2-CaO–Na2O–P2O5 oxide system. It was “bioactive” in the sense that it could form a hydroxycarbonated apatite (HCA) layer on the glass surface upon contacting solutions mimicking human plasma, allowing for a bonding interface with the bone tissue (Hench, 1991). Many other bioactive glasses have similar oxide compositions to the original 45S5 Bioglass®, but with varying concentrations (Brauer, 2015)—some such compositions reviewed in this article are displayed in Table 1. One of the key features of bioactive glasses are that they release ionic dissolution products which can control osteoblastic gene expression, allowing for their bone regenerative ability (Xynos et al., 2001). Further, bioactive glass is able to release soluble ions (such as Si, Ca, P, Na ions) at the rate required for proliferation and differentiation of cells (Larry and Hench, 2006; El-Rashidy et al., 2017). Previous investigations have established that they are osteoconductive and osteoinductive too (Hench et al., 2014). Recently, bioactive glass has gained traction because of its ability to induce the angiogenesis needed for bone regeneration, as aforementioned (Kargozar et al., 2018a). This could provide a more cost-effective way of promoting neovascularization than the use of growth factors (Rahaman et al., 2011). For example, some bioactive glasses such as S53P4 (53% SiO2, 23% Na2O, 20% CaO, 4% P2O5) have been shown to increase vascular endothelial growth factor (VEGF) secretion to stimulate angiogenesis in bone (Detsch et al., 2014). Authors have highlighted that investigating ways of the promoting of angiogenesis as one of the most important topics in regenerative medicine (Rouwkema and Khademhosseini, 2016). Thus bioactive glasses may provide one such solution (Kargozar et al., 2018a).
There has been a recent trend in adding other components to the bioactive glass in order to aid regeneration. It has been suggested that adding suitable dopants could influence osteoblastic differentiation (Nohra et al., 2014). These include metallic elements such as copper, strontium and borosilicate, biochemical factors such as dimethyloxallyl glycine (DMOG) and organic polymers such as photo-cross linkable gelatin derivative containing gelatin and methacrylicanhydride (GeIMA). Khan et al. found that adding strontium promoted early stage in vivo osteointegration and bone remodeling (Khan et al., 2016). A previous study, for example, showed that DMOG, an inhibitor of the enzyme hypoxia-inducible factor prolyl hydroxylase (HIF-PH), could mimic a hypoxic microenvironment and thereby increase angiogenic capacity and osteogenic differentiation of human bone marrow stromal cells (Wu et al., 2013a). Other studies have indicated that bioactive glass and GeIMA composites could encourage cell attachment and proliferation as well as osteogenic differentiation.
There is a large body of evidence supporting the efficacy of various types of bioactive glass scaffolds to induce angiogenesis and osteogenesis in vitro. For example, Zhang et al. recently found that their bioactive glass functionalized chondroitin sulfate hydrogel had promoted the formation of a vascular network (Zhang et al., 2019). Jia et al. looked at bioactive silicate (13–93) and borosilicate (2B6Sr) glass scaffolds and found that they were able to facilitate cell attachment and promoted formation of VEGF (Jia et al., 2019). They could also help stimulate mineral deposition and osteoblast marker gene expression. Quinlan et al. found that using cobalt bioactive glass with a particular particle size could encourage the formation of VEGF and could also help osteoblast cell proliferation irrespective of particle size in vitro (Quinlan et al., 2015). These in vitro analyses can provide an evaluation of cell differentiation and proliferation, as well as cytotoxicity (Hanks et al., 1996), and avoid the unnecessary use of animals. However, it is well-documented that in vitro studies can only conduct evaluations of toxicity in the short term, due to the short lifespan of cultured cells (Pizzoferrato et al., 1994). Further, in vitro evaluation test more simplified parts of the more complex in vivo mechanisms due to the fact that in vivo cells often interact with another (Pizzoferrato et al., 1994). It is therefore important to evaluate scaffolds in vivo.
It is also important that we have viable methods to assess the efficacy of these scaffolds in vivo to promote angiogenesis and osteogenesis. Advances in micro-CT has allowed studies to assess the mineralization of bone formation spatially and temporally in a non-destructive way (van Lenthe et al., 2007) and can provide information such as bone mineral density (BMD), bone volume fraction and new bone volume. Immunohistochemistry has allowed studies to investigate angiogenesis. A plethora of growth factors have been shown to promote angiogenesis. For example, VEGF has been shown to stimulate endothelial proliferation and migration (Ferrara et al., 1992). Other endothelial specific markers include CD31 and CD34 (Miettinen et al., 1994). Detection of such markers allow for an easy way to identify blood vessels and endothelial cells (Lu et al., 2006). Another way in which this can be assessed is the use of Microfil® perfusion and subsequent micro-CT. This method enables not only the visualization of blood vessels but the quantitative characterization of blood vessels and their branching (Ehling et al., 2014) and so is considered to be superior to angiography or blood vessel casting (Lu et al., 2006).
Due to the aforementioned advantages of these methods, only studies which included an immunohistochemistry or Microfil approach to assess angiogenesis combined with micro-CT analyses for osteogenesis were included in this study. This provides a more robust assessment of scaffolds and their outcomes of angiogenesis and osteogenesis in these in vivo studies. As previously mentioned, several studies in the literature utilize scaffolds which have added biochemical components. Due to the heterogeneity in the components added, few systematic reviews have included such studies. We provide a comprehensive, update on in vivo studies looking at bioactive glass- containing non-cellular scaffolds with or without added biochemical components for osteogenesis and angiogenesis.
Methods
A literature search was performed across three databases, PubMed, Scopus and EMBASE, in November 2019 using the search terms “bioactive glass AND bone AND angiogenesis” from 2015 to present. The inclusion criteria were as follows: (1) in vivo animal studies, using surgically-created bone defects, without any pre-treatment, to assess bone regeneration, (2) published in a peer-reviewed journal, (3) published from 2015 onwards, (4) containing study groups with no additional cells seeded in a bioactive glass-containing scaffold, (5) with the scaffold being implanted directly into the bone defect, (6) assessing both angiogenesis and osteogenesis to establish extent of bone regeneration in model, (7) assessing angiogenesis using either immunohistochemistry or Microfil® perfusion method, and (8) assessing osteogenesis using micro-CT method. Exclusion criteria were: (1) all in vitro studies, (2) conference papers, (3) studies not published in English, and (4) articles for which the full-text version was not freely accessible.
Title and abstract screening was performed by four authors (CKIR, CDSR, JCR, and DS) on the non-duplicate articles retrieved by the initial search against the inclusion criteria. An additional hand search was also performed on pertinent review articles for any articles that might have been missed by the initial search. Full-text articles were retrieved for those chosen for further assessment.
Results
The outline of the search results is displayed in Figure 1. To be included in this review, studies must have used bioactive glass-containing scaffolds and implanted them directly into a bone defect. Those that did not directly insert them into a bone defect are referred to as “ectopic implantation method.” Those that treated the bone to cause osteonecrosis before creating the bone defect were also excluded and are referred to as “uses pre-treatment.” Articles must have reported outcomes for both angiogenesis, in the form of either Microfil® perfusion or immunohistochemistry, and osteogenesis, in the form of micro-CT. Those that did not report a measure of angiogenesis are given as “no measure of angiogenesis.” Articles that did not report using the appropriate outcome measures detailed above are given as “incorrect outcome measure.”
The initial search and hand search for this systematic review returned nine studies. The study characteristics of these nine papers are detailed in Table 1. Table 2 shows the results of the studies included in this review.
Demographic Features of Animal Models
In total, 202 animals were surgically treated to form experimental models with critical-sized bone defects. Of these, 158 subjects were rats whilst Jia et al. (2015) used rabbit models. In 70 (34.7%) animals across three papers, a single defect (Jia et al., 2015; Qi et al., 2017; Jing et al., 2018) was surgically created, whilst the other 132 (65.3%) animals across six papers sustained two defects (Min et al., 2015; Zhao et al., 2015; Qi et al., 2017; Li et al., 2019; Wang et al., 2019; Wu et al., 2019). The length of time for which the scaffold was implanted before termination of the experiment ranged from 8 weeks to 3 months with one paper examining their findings at two end dates (both 4- and 8-weeks post-implantation) (Xin et al., 2017).
Features of the Scaffolds
All studies included in this review used different bioactive glass compositions and/or glass fabrication methods to create them, as shown in Table 1. Eight (Min et al., 2015; Zhao et al., 2015; Qi et al., 2017; Xin et al., 2017; Li et al., 2019; Wu et al., 2019) out of the nine papers included added components to the bioactive glass scaffold. These studies can be divided into 3 broad categories. Firstly, those that incorporated additional elements into the bioactive glass network (Jia et al., 2015; Zhao et al., 2015; Wu et al., 2019). Secondly, those that used additives to the scaffold (Xin et al., 2017; Li et al., 2019; Wang et al., 2019). Finally, those that used biochemical factors (Min et al., 2015; Qi et al., 2017). These findings are summarized in Table 1.
Do the Scaffolds Induce Osteogenesis?
In order to be included in this review, articles must have used micro-CT to evaluate osteogenesis. These results are detailed in Table 2. All nine papers showed evidence of bone regeneration when the bioactive glass was present, which, in the seven experiments where this was applicable (Jia et al., 2015; Min et al., 2015; Zhao et al., 2015; Xin et al., 2017; Jing et al., 2018; Li et al., 2019; Wu et al., 2019) was increased compared to the non-bioactive glass treated control.
Four papers assessed BMD as an indicator of osteogenesis. Both Zhao et al. (2015) and Wu et al. (2019) found that there was significantly increased bone formation seen on micro-CT in animals treated with bioactive glass scaffolds compared to negative controls or animals treated with scaffolds which did not include bioactive glass, respectively. Wang et al. (2019) and Qi et al. (2017) did not include a negative controls, with all experiments using bioactive glass. Both papers showed that BMD was seen to improve across all groups and was significantly increased in bioactive glass scaffolds treated with other substances, this has been further discussed below.
Of the nine papers, only six used micro-CT to assess the bone volume fraction [bone volume/total volume (BV/TV)]. Wang et al. (2019) and Qi et al. (2017) found similar results as with BMD testing; again, the micro-CT assessment of bone volume fraction showed significantly increased osteogenesis in bioactive glass scaffolds treated with other substances. This is demonstrated in Figures 2E,F. The appearance of the calvarial bone defects at 8 weeks after implantation is shown in Figures 2A–D. Both Xin et al. (2017) and Wu et al. (2019) showed that the gels with bioactive glass nanoparticles showed significant increases in bone volume fraction compared to those treated without bioactive glass. Zhao et al. (2015) observed significantly greater bone volume fraction in MBG scaffolds group compared to the negative control group, with the Strontium- supplemented MBG scaffold showing significantly greater improvement than all other scaffolds. Li et al. (2019) found that FTY with bioactive glass and PLGA scaffolds showed the greatest increase in bone volume fraction compared to all other groups including the negative control. The MBG-PLGA group also showed a significantly greater increase bone volume fraction than the PLGA alone group as well as the negative control group. This effect on osteogenesis is demonstrated in Figures 3E,G. The micro-CT appearance of calvarial bone defects at 8 weeks is seen in Figure 3A whilst the blood vessels are seen in Figure 3B. The osteogenesis at week 8 is displayed by staining in Figure 3C. Figure 3D shows sequential fluorescent labeling to display the degree of bone mineralisation in each scaffold. Figure 3F shows quantitatively the degree of osteogenesis using staining and Figure 3H using fluorescent labeling.
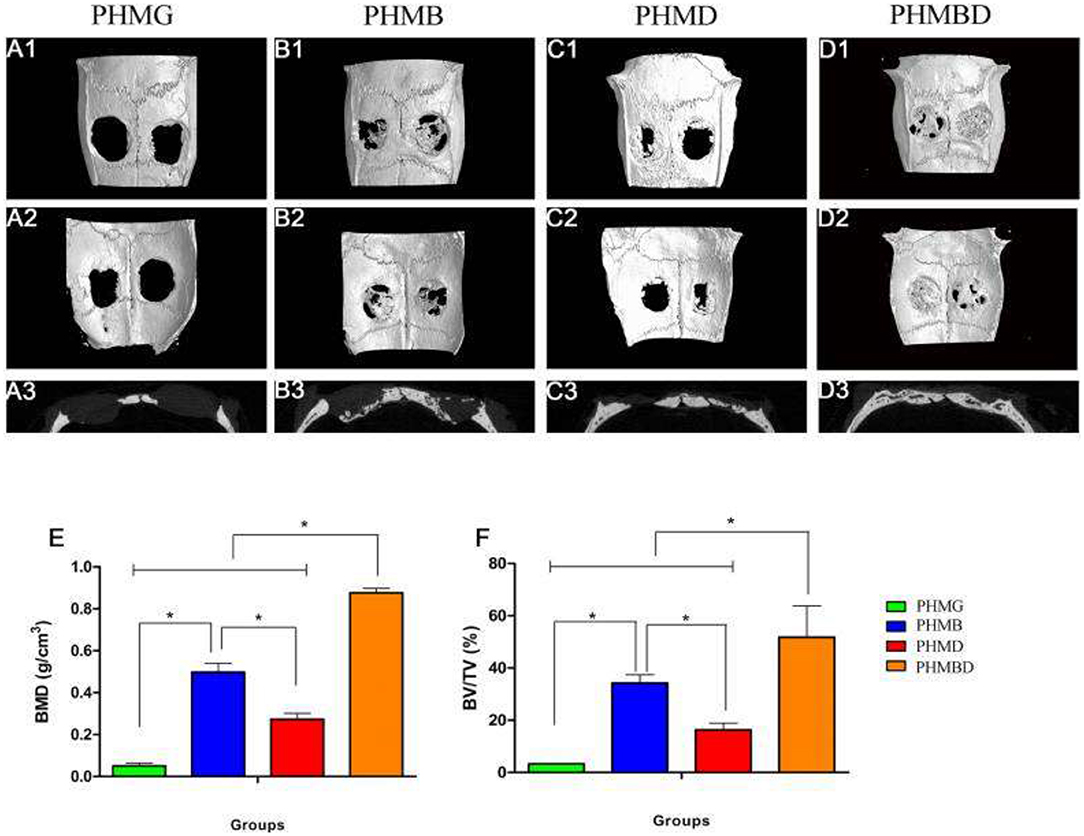
Figure 2. The effect of mesoporous bioactive glass containing groups on osteogenesis. The representative 3D reconstructions of superficial (A1–D1), interior (A2–D2), and sagittal images (A3–D3) of calvarial bone defects taken at 8 weeks after implantation are shown. Morphometric analysis of (E) BMD and (F) BV/TV is also shown as determined by micro-CT for each group at 8 weeks (*p < 0.05). MBG, mesoporous bioactive glasses; PHMB, BMP-2 + MBG-PHBHHx; PHMBD, BMP-2 + DMOG + MBG-PHBHHx; PHMD, DMOG + MBG-PHBHHx; PHMG, pure MBG-PHBHHx. Figure and caption reused from Qi et al. (2017). Used under the Creative Commons License (https://creativecommons.org/licenses/by/4.0/legalcode).
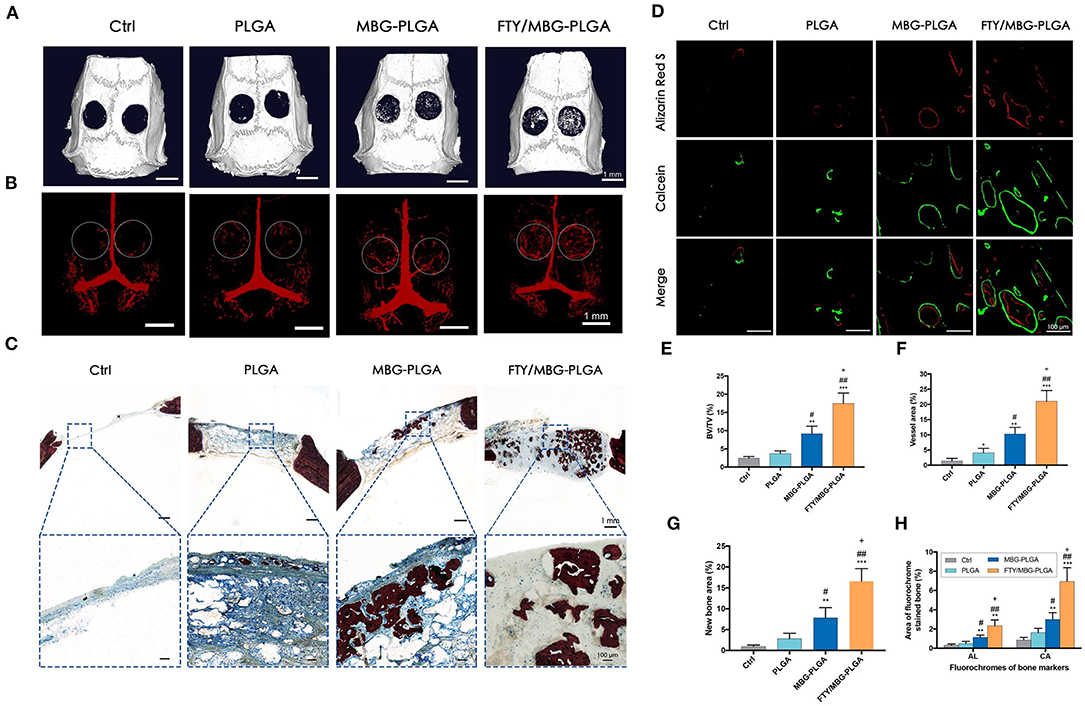
Figure 3. Osteogenesis and aniogenesis in critical sized calvarial bone defects. (A) Micro-CT images for the bone repair in calvarial defects of different groups after 8 weeks of implantation. (B) Micro-CT observation of the newly-formed blood vessels perfused with Microfil in the defect regions (as indicated by the white circle) at week 8. (C) New bone formation observed by Van Gieson's picrofuchsin staining at week 8 (red, new bone; blue, residual material). (D) Sequential fluorescent labeling observation for dynamic bone mineralization, Alizarin Red S (AL, week 4), and Calcein (CA, week 6). Quantitative data (n = 3) from micro-CT analysis of BV/TV, bone volume fractions (E) and blood vessel volume in the defect area (F). The quantitative analysis of the new bone area of Van Gieson's picrofuchsin staining (G) and sequential fluorescent labeling (H). *P < 0.05, **P < 0.01, ***P < 0.001 compared with control group; #P < 0.05, ##P < 0.01 compared with PLGA group; +P < 0.05 compared with MBG-PLGA group. PLGA, poly(lactic-co-glycolic acid); MBG-PLGA, mesoporous bioactive glass and poly(lactic-co-glycolic acid) scaffold; FTY/MBG-PLGA, FTY720 with mesoporous bioactive glass and=poly(lactic-co-glycolic acid) scaffold. Figure and caption reused from Li et al. (2019). Used under the Creative Commons License (https://creativecommons.org/licenses/by-nc-nd/4.0/legalcode).
Jia et al. (2015) found that, if left for 9 months, both the bioactive glass containing scaffolds, namely silicate 13–93 and borosilicate 2B6Sr, induced significantly more new bone formation compared to the negative control.
Do the Scaffolds Induce Angiogenesis?
The studies included in this review assessed angiogenesis using two methods: immunohistochemistry to evaluate new blood vessel formation by staining vascular endothelium or by visualizing neo-vasculature using CT with Microfil® perfusion. These results are detailed in Table 2.
Of the nine papers included in this review, seven used immunohistochemistry to investigate the angiogenesis-promoting qualities of bioactive glass (Jia et al., 2015; Qi et al., 2017; Xin et al., 2017; Jing et al., 2018; Li et al., 2019; Wang et al., 2019; Wu et al., 2019). The majority of the papers used CD31, where three out of five the included papers found that positively staining micro vessels were significantly increased in the experiments which included bioactive glass, in at least one time point (Xin et al., 2017; Jing et al., 2018; Li et al., 2019). This effect, in the study by Li et al. (2019), is demonstrated in Figures 4A,B. Notably, Jia et al. (2015) found that although there was a significant increase in density between the 2B6Sr containing scaffold compared to the 13-93 and ABG groups at 3 months, this difference was not observed at 9 months. Similarly, Qi et al. (2017) observed that although neovascularization and CD31 staining was seen in all bioactive glass treated groups, this was increased in BMP-2+DMOG+MBG-PHBHHx scaffolds and PHMD compared to the PHMB and PHMG, thus suggesting that DMOG helps to increase angiogenesis. Three other stains were also used, each by a single paper. Similar to their CD31 findings, Jing et al. (2018) observed that the number of VEGF-positive blood vessels were significantly higher in bioactive glass treated experiments compared to negative controls, suggesting higher levels of angiogenesis with bioactive glass. In contrast, Wang et al. (2019) found limited CD34 staining in bone defects treated with bioactive glass alone, whilst there was more staining as the concentration of graphene oxide used increased. However, there was no negative control used in this study for comparison. Similarly, Wu et al. (2019) found similar smooth muscle (α-SMA) antigen staining in the bioactive glass containing group (BG/CH/SF/GP) compared to the non-bioactive glass containing group (CH/SF/GP).
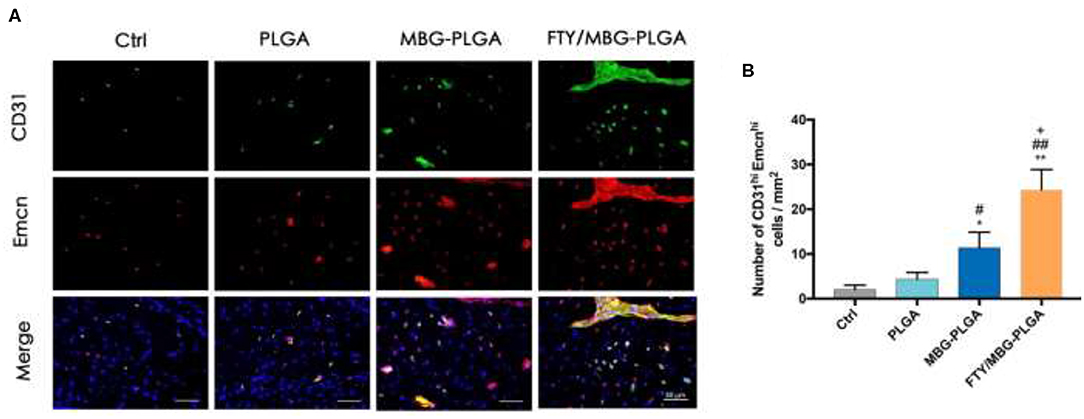
Figure 4. Angiogenesis in critical sized calvarial bone defects, using CD31hi and Emcnhi immunofluorescence staining for calvarial defect sections. (A) Immunofluorescence staining images of CD31 and Emcn for the calvarial defect sections from various groups (green, CD31; red, Emcn; blue, cell nuclei). (B) The quantitative analysis of CD31hiEmcnhi cells per mm2 from the staining results, n = 5. *P < 0.05, **P < 0.01 compared with control group; #P < 0.05, ##P < 0.01 compared with PLGA group; +P < 0.05 compared with MBG-PLGA group. PLGA, poly(lactic-co-glycolic acid); MBG-PLGA, mesoporous bioactive glass and poly(lactic-co-glycolic acid) scaffold; FTY/MBG-PLGA, FTY720 with mesoporous bioactive glass and=poly(lactic-co-glycolic acid) scaffold. Figure and Caption reused from Li et al. (2019). Used under the Creative Commons License (https://creativecommons.org/licenses/by-nc-nd/4.0/legalcode).
In summary, the addition of bioactive glass alone increased angiogenesis marker staining in four of nine papers (Jia et al., 2015; Xin et al., 2017; Jing et al., 2018; Li et al., 2019), but this was not significant in the experiments by Wu et al. (2019) and can't be assessed with Wang et al. (2019) as they did not test the effect of bioactive glass alone. Five out of nine papers included in this review assessed angiogenesis through analyzing Microfil® perfusion studies (Min et al., 2015; Zhao et al., 2015; Qi et al., 2017; Li et al., 2019; Wang et al., 2019). All of these papers showed evidence of angiogenesis and the formation of new blood vessels in the bone defect, although this process could be enhanced by the addition of other substances. Notably, both Li et al. (2019) and Zhao et al. (2015) showed that scaffolds containing bioactive glass induced significantly greater new blood vessel area than those without.
Studies Reporting Relative Increase in Angiogenesis and Osteogenesis
Five out of nine studies (Min et al., 2015; Zhao et al., 2015; Xin et al., 2017; Jing et al., 2018; Li et al., 2019) showed increases in both angiogenesis and osteogenesis in bioactive glass-containing scaffolds compared to scaffolds without bioactive glass (Xin et al., 2017; Li et al., 2019) or negative control groups (Min et al., 2015; Zhao et al., 2015; Jing et al., 2018).
Wu et al. (2019) showed that, although the bioactive glass containing scaffolds showed increased levels of osteogenesis compared to those without bioactive glass, they did not always show an increase in angiogenesis compared to those without. Jia et al. (2015) showed that although both bioactive glass containing scaffolds showed complete more osteogenesis than in the negative control, there was no comment on the extent of angiogenesis in the negative control for comparison. As all groups contained bioactive glass, the results of Qi et al. (2017) and Wang et al. (2019) cannot be commented on in this respect.
Effects of Changing the Composition of the Bioactive Glass Scaffolds
Seven out of the nine papers included in this review studied bioactive glass scaffolds with added non-cellular components as outlined below. These can be broadly divided into components which were incorporated into the bioactive glass network, additives, and finally biochemical factors.
Incorporation of Additional Elements Into Bioactive Glass Network
Ions and inorganic compounds: Three papers investigated the effect of incorporating metals into the bioactive glass network. Jia et al. (2015) added boron trioxide (borosilicate) to the bioactive which made for very successful scaffolds. Increased osteogenesis compared to the negative control was observed for both silicate and borosilicate scaffolds, whilst for angiogenesis, the borosilicate-containing bioactive glass scaffold showed higher levels of CD31 positively stained vasculature initially, but by 9 months there was no difference between the two scaffolds. Zhao et al. (2015) also found that although the addition of mesoporous glass showed more favorable outcomes with both osteogenesis and angiogenesis, however, the addition of strontium improved outcomes in terms of bone growth, bone volume fraction and angiogenesis. Similarly, Wu et al. (2019) found that the addition of copper allowed for a full repair of the bone defect, whilst bioactive glass alone only allowed for partial repair. Furthermore, α smooth muscle (α-SMA) antigen staining was greater in copper treated scaffolds. Micro-CT results further corroborated these results with copper improving osteogenesis as observed on micro-CT, significantly higher BMD and BV/TC.
Use of Additives to the Bioactive Glass Scaffold
Wang et al. (2019) examined the effects of adding graphene oxide to bioactive glass scaffolds. Micro-CT showed markedly increased osteogenesis in groups treated with higher concentrations of graphene oxide in the bioactive glass. Moreover, angiogenesis was also seen to be increased as shown by CD34 staining and Microfil® perfusion experiments in bioactive glass containing graphene oxide compared to those without.
Two papers evaluated the effect of bioactive glass when used in combination with organic polymers to form a scaffold. Xin et al. (2017) found that crossing linking MBGNs with photo-cross-linkable GelMA and then incorporating GelMA increased angiogenesis, as shown by significantly higher levels of CD31 staining at 4 weeks compared to scaffolds just created by chemically modifying mesoporous bioactive glass nanoparticles with photo-cross-linkable GelMA. Furthermore, Li et al. (2019) found that MBG-PLGA composite scaffolds were more effective at inducing osteogenesis when the bioactive lipid, FTY720, was added, as shown by micro-CT study. Microfil® perfusion studies and CD31 staining showed a similar effect on angiogenesis.
Use of Biochemical Factors
Two out of the nine papers included in this paper assessed the effect of the addition of osteoinductive biochemical factors. One of the factors was DMOG. Qi et al. (2017) found that BMD was highest in the bioactive glass group treated with DMOG as well as BMP-2 along with angiogenesis as observed with CD31 staining. In experiments by Min et al. (2015) angiogenesis was observed with bioactive glass with or without the addition of DMOG. However, DMOG loaded scaffolds showed an advantage as they promoted the ingrowth of dense vessels into the center of the defect, whilst unloaded bioactive glass scaffolds encouraged growth around the periphery.
Discussion
The regenerative capacity of bioactive glass scaffolds are dependent on a number of factors including the composition of the bioactive glass scaffolds, the method of fabrication and the microstructure of the scaffold amongst others (El-Rashidy et al., 2017). Although bioactive glass could enhance bone formation, more research is needed to characterize the influencing factors. This review attempts to elucidate the effects of non-cellular bioactive glass-containing scaffolds on osteogenesis and angiogenesis in bone defect by analyzing the in vivo animal literature.
Osteogenesis and Bioactive Glass Scaffolds
Osteogenesis was initially thought to take place through the dissolution of particles (in the original 45S5 Bioglass® causing an HCA layer to form rapidly, the glass degrading and then allowing space for bone ingrowth Hench and Jones, 2015. It was later shown that the dissolution of the bioactive glass particles releases ions that acted as signals into the cells, prompting upregulation of certain genes and subsequent increases in nuclear transcription factors, cell cycle regulators, and growth factors such as insulin-like growth factor II (IGF-II) (Xynos et al., 2000, 2001). Transcription of extracellular matrix (ECM) components and secretion into a mineralized matrix is thought to lead to the formation and growth of bone nodules along with differentiation of the osteocyte phenotype (Hench and Jones, 2015). Individual ions also play a role, with ions such as calcium being important for upregulation of osteogenic genes (Hench, 2009) and silicon important for the formation and calcification of bone tissue (Carlisle, 1981).
Bone Volume Fraction as an Indicator for Osteogenesis
Two studies (Qi et al., 2017; Wang et al., 2019) found that bone volume fraction significantly increased in bioactive glass scaffolds treated with other substances. Xin et al. (2017) and Wu et al. (2019) showed that gels with bioactive glass nanoparticles also had significant increases in bone volume fraction compared to defects treated without bioactive glass. Similarly, Li et al. (2019) showed that the bioactive glass containing groups showed greater increases in the bone volume fraction than those without bioactive glass. Zhao et al. (2015) found a greater bone volume fraction in MBG scaffolds than negative controls. Jia et al. (2015) found that bioactive glass containing scaffolds induced more bone formation than negative controls.
BMD as an Indicator for Osteogenesis
Wang et al. (2019) and Qi et al. (2017) found that bioactive glass scaffolds increased BMD. Zhao et al. (2015) and Wu et al. (2019) found that BMD also increased in animals with bioactive glass scaffolds compared to negative controls or animals with scaffolds without bioactive glass. Thus, these results on the whole do indicate that bioactive glass scaffolds could induce osteogenesis, with no papers showing an insignificant difference between control and bioactive glass containing groups.
Angiogenesis and Bioactive Glass Scaffolds
Angiogenesis is the growth of blood vessels from existing vasculature, occurring throughout life and can be physiological or pathological. The two types of angiogenesis are sprouting and intussusceptive. Intussusceptive angiogenesis is when a vessel is split into two and is more relevant in embryogenesis than in the growth of vascular networks into scaffolds. Sprouting angiogenesis is initiated in hypoxic conditions due to the expression of VEGF-A by parenchymal cells and a tip cell guided by filopodia will follow the VEGF gradient using VEGF receptors. Vacuoles will form and tip cells will fuse forming a continuous lumen for blood to flow through and once oxygen is supplied, VEGF-A levels return to normal. Other signaling pathways such as the delta-notch pathway are also responsible for sprout formation, but even this pathway is reliant on VEGF expression demonstrating the importance of the factor (Adair and Montani, 2010). Markers such as CD31 which is an endothelial cell-cell adhesion molecule have been shown to be an indication of new vessel formation (DeLisser et al., 1997), as well as CD34 which is expressed on tip cells (Siemerink et al., 2012) and a-SMA which is an indication of microvessel density (Tonino and Abreu, 2011).
One of the indications for the development of bioactive glass scaffolds, was the potential for enhanced angiogenesis. The creation of a vascular network is vital for tissue to successfully regenerate and has been acknowledged as a significant issue in tissue engineering, particularly for larger tissues such as bone. Spontaneous growth of vessels has a slow rate and vascularization of an implant may take weeks, during which time tissue is unevenly supplied and may centrally go hypoxic (Rouwkema et al., 2008). Bioactive glass has been shown to be proangiogenic in vitro, stimulating the expression of VEGF from fibroblasts and proliferation of microvascular endothelial cells (Day, 2005). The ionic dissolution products such as Si, which were shown to affect gene expression in human osteoblasts explaining osteogenic effects (Xynos et al., 2001), have also been shown to be pro-angiogenic (Zhai et al., 2012). Calcium silicate has been shown to stimulate VEGF and VE-cad expression, with silicon ions determined to have a significant role in angiogenesis by dilution experiments and calcium ions supplementing the silicon's effect (Li and Chang, 2013).
The results from papers on the effects on bioactive glass on angiogenesis in vivo are mixed. Four papers showed evidence of bioactive glass alone increasing immunohistochemistry staining indicating angiogenesis e.g., CD31 (Qi et al., 2017; Xin et al., 2017; Jing et al., 2018; Li et al., 2019), VEGF (Jing et al., 2018). Increased Microfil® perfusion was seen by the only four groups who tested Microfil® perfusion for controls or bioactive glass alone as part of their study (Min et al., 2015; Zhao et al., 2015; Qi et al., 2017; Li et al., 2019). Wang et al. (2019) did not compare to control but showed limited staining of CD34 with bioactive glass alone, whilst Wu et al. (2019) saw no difference in a-SMA staining between non-bioactive glass and bioactive glass treated groups. Jia et al. (2015) found no significant difference in CD31 staining between bioactive glass scaffolds (13–93 silicate alone) and autologous bone graft controls at 3M and 9M. This is significant as the three other studies that used CD31 as a marker and compared angiogenesis to a non-bioactive glass control all found an increase (Xin et al., 2017; Jing et al., 2018; Li et al., 2019), but Jia et al. (2015) was also the only paper that used a rabbit femoral defect instead of rat skull. Although the papers tend to indicate that bioactive glass has pro-angiogenetic properties, the results are not as overwhelming as the osteogenic effects and indicate a potential avenue for future improvement, which will be discussed below.
How Does Changing the Composition of Bioactive Glass Scaffolds Impact Their Properties?
Only a single study reviewed focused on the use of bioactive glass without any non-cellular additives (Jing et al., 2018). Innovative scaffolds tend to involve the incorporation of metals, biochemical factors and organic compounds. It is important to acknowledge this variance in composition as the heterogeneity of the scaffolds makes it difficult to directly compare results.
Incorporating Additional Elements (Boron, Strontium, Copper) Into the Bioactive Glass Network
Boron seems to have a physiological role in bone where it accumulates, with recent mechanisms proposed such as simultaneous activation of the NaBC1 ion channel and VEGFR (Moseman, 1994). There is also evidence in other models that boron in bioactive glass allows for enhanced bone formation, potentially due to its impact on angiogenesis (Gorustovich et al., 2006). Jia et al. (2015) found boron improved angiogenesis (assessed by CD31 staining) at 3 months compared to standard silicate bioactive glass, but this difference was not found at 9 months. This suggests that boron can increase angiogenesis early but there is no overall effect by the end of 9 months. Zhao et al. (2015) found strontium both increased BMD and bone volume fraction on micro-CT as well as Microfil® perfusion, therefore having osteogenic and angiogenic benefits. This follows on from evidence that strontium stimulates bone formation and reduces bone resorption (Kyllönen et al., 2015). This could be due to strontium ions' ability to inhibit receptor activator of nuclear factor kappa-B ligand (RANKL) in mesenchymal stem cells, thereby inhibiting osteoclast differentiation (Marie et al., 2011). Strontium also promotes vessel formation by stimulation of VEGF, MMP-2 etc. (Zarins et al., 2017). Copper also increased osteogenic and angiogenic effects of bioactive glass, particularly angiogenesis (a-SMA) which was similar between controls and standard bioactive glass (Wu et al., 2019). A link between copper and bone health has been established previously (Qu et al., 2018) and has been found to have osteostimulation properties, facilitating new bone formation (Wu et al., 2013b). The ion also stimulates angiogenic factor expression such as VEGF and HIF-1 (Du et al., 2018). Copper and silicon ions have also been reported to have synergistic effects on angiogenesis in vitro (Kong et al., 2014).
Using Additives (Graphene Oxide and Organic Polymers) in Bioactive Glass Scaffolds
Wang et al. (2019) used graphene oxide, an inorganic compound, and found that not only did the addition increase osteogenesis and angiogenesis (assessed by both CD34 and Microfil® perfusion), but a high amount had significantly better results than a low amount of graphene in the scaffold. This was particularly evident in the CD34 staining, which was limited in the use of standard bioactive glass scaffolds. Graphene oxide is a conductive material, which are known to enhance bone repair, and it is known to induce osteoblastic differentiation and mineralization (Cheng et al., 2018).
MBG-PLGA (PLGA alone was equivalent to control) composites were evaluated by Li et al. both with and without FTY720, a bioactive lipid. FTY720 is used as an immunosuppressive drug for multiple sclerosis due to a reduction in peripheral lymphocytes and T cell inhibition (Baer et al., 2018) with a developing role in cancer therapy (White et al., 2016). There is evidence local delivery of FTY720 in calvarial defects increases bone volume (Huang et al., 2012), potentially by stimulating sphingosine 1-phosphate receptors and as a result, the local microvascular network (Aronin et al., 2010). Those with FTY had increased bone volume, CD31 staining and Microfil® blood vessel area implying a benefit of this organic compound. Xin et al. (2017) incorporated photo-cross-linked GelMA into a bioactive glass nanoparticles scaffold in which GelMA was part of the composition of the particles. GelMA has good biocompatibility and is similar to the ECM, as a semi-synthetic hydrogel. There was no control without any GelMA at all, but the addition of photo-cross-linked GelMA to the composite nanoparticles did increase the bone volume fraction and CD31 staining. It is important at this point to acknowledge the use of nanoparticles in bioactive glass. Their high surface to volume ratio and ability to incorporate into matrices more evenly helps maximize the yield of the bioactive glass in bone regeneration (Tabia et al., 2019).
The Use of Biochemical Factors (DMOG) in Bioactive Glass Scaffolds
DMOG is an osteoinductive biochemical factor. It is a competitive inhibitor of prolyl hydroxylase enzymes which subsequently leads to less degradation of the angiogenic factor HIF-1a (Zhang et al., 2016). The osteogenic effects of DMOG are not clear as Qi et al. (2017) found BMD was better than bioactive glass alone but for Min et al. (2015) there was no difference. Nevertheless, both papers found a benefit in angiogenesis. Qi et al. (2017) saw CD31 staining and Microfil® perfusion was greatest with both DMOG and bioactive glass, but interestingly DMOG alone had a significantly better effect than bioactive glass alone, which is the opposite to osteogenic results. Min et al. (2015) also found that DMOG-loaded bioactive glass scaffolds showed more vessel ingrowth into the center of the defect compared to bioactive glass alone as opposed to around the peripheries.
Other Bioactive Glass Scaffolds and the Future
For this systematic review, any scaffolds with cells added were excluded due to the potentially drastic impact on bone formation. Nevertheless, scaffolds now frequently incorporate stem cells to enhance the potential for regeneration. Adipose-derived mesenchymal stem cells are commonly the cells used (Handel et al., 2013; Du et al., 2018) due to the relative ease in obtaining them and potential for differentiation into multiple relevant cell types (Strem et al., 2005). Other stem cell types used include those derived from bone and umbilical cords, with benefits in particular seen with bone-marrow derived stem cells for osteogenesis and umbilical cord for angiogenesis on comparison (Kargozar et al., 2018b). UC-derived stem cells may therefore be useful in stimulating angiogenesis when added onto bioactive glass, which as seen from above has a bigger need to be targeted than osteogenesis.
The future of bioactive glass in bone regeneration is vast. The biocompatibility and osteogenic effects make it ideal to encourage the healing of bone defects, as seen both in vitro and reviewed here in vivo. One avenue that is being explored in bioactive glass currently is the controlled release of biomolecules from the scaffold. This could be utilized in bone regeneration with targeted drug delivery. The factors released could include transforming growth factors, bone morphogenetic proteins, stromal cell-derived factors etc. to imitate in vivo signaling for repair, many of which are approved for use in humans and incorporated into implants already (Gothard et al., 2014; Baino et al., 2018). The other key advancement in bioactive glass scaffolds, is the structure of these scaffolds. Mesoporous bioactive glass scaffolds were initially brittle meaning they could not be used in practice. Developments in 3D printing and polymer addition have strengthened the scaffold (Baino et al., 2018), but structure could still be improved, with recent advancements in nanoparticles increasing the surface area exposed to tissue. A randomized controlled clinical trial in humans using bioglass for bone healing after tooth extraction showed preservation of alveolar bone and enhanced bone remodeling (El Shazley et al., 2016). Despite this being a very different situation to critical size bone defects, the successful use of the biomaterial in humans implies promise for bioactive glass in therapeutics.
Limitations
It is important to highlight the limitations of this systematic review. First, only three databases were searched (Pubmed, Scopus, and EMBASE). Additionally, only studies that were in English were looked at, therefore there might have been some relevant studies that may have been overlooked. Nine studies were included in this review, which represents a very small number in the bioactive glass scaffold literature. The studies that were included used a variable type of scaffold and used different animal models limiting any direct comparison between studies. There was also variability in the methodology of the studies, for example some studies using BMD as opposed to bone volume fraction as a marker for osteogenesis, and different immunohistochemistry markers for angiogenesis. Future studies using standardized methods of measuring these properties would help in comparison and making conclusions. Further, some studies did not include a control, making any definitive causal conclusions hard to ascertain, as well as only one study evaluating results at a longer time point of 9 months (Jia et al., 2015). Future studies should aim to take measurements at more than one time point and indeed in the long term, which could be important for clinical translation of such scaffolds from animal defect models to humans.
Conclusion
The data from this systematic review suggests that despite the heterogeneity of the scaffolds and markers assessed, there is consistent evidence that bioactive glass does improve bone regeneration in these models, both by osteogenic and angiogenic measures. This review also highlighted the benefits of changing the composition of the bioactive glass scaffolds using 3 methods. Firstly, incorporation of additional elements into the bioactive glass network such as boron, copper and strontium. Secondly, the use of additives and finally the use of biochemical factors. However, comparisons between the scaffolds are limited by the heterogeneity of study methods. The future of bioactive glass will broaden as advancements in technology are made and the structure, composition and additives for bone scaffolds must be optimized for and trialed in humans, which will take a significant amount of research and time. Increased consistency in composition of scaffold and outcome measures will help in determining the scaffold with optimal results. Nevertheless, the in vivo results do confirm that bioactive glass has a future in bone regeneration.
Author Contributions
CDSR, CKIR, JR, DS, and WK: conceptualization, methodology, and writing—review and editing. CDSR, CKIR, JR, and DS: writing—original draft preparation. WK: supervision.
Conflict of Interest
The authors declare that the research was conducted in the absence of any commercial or financial relationships that could be construed as a potential conflict of interest.
Acknowledgments
The authors gratefully acknowledged the financial support of Versus Arthritis (Formerly Arthritis Research UK) through Versus Arthritis Tissue Engineering and Regenerative Therapies Centre (Grant 21156).
Abbreviations
45S5, glass with 45 wt.% of SiO2 and 5:1 molar ratio of calcium to phosphorous; α-SMA, alpha-smooth muscle actin; BMD, bone mineral density; BMP-2, human bone morphogenetic protein-2; BV/TV, bone volume/total bone volume i.e., bone volume fraction; CD31, cluster of differentiation 31; CD34, cluster of differentiation 34; Cu-BG/CH/SF/GP, copper-doped bioactive glass with chitosan/silk fibroin/glycerophosphate concentration 1; Cu-BG/CH/SF/GP(II), copper-doped bioactive glass with chitosan/silk fibroin/glycerophosphate concentration 2; DMOG, dimethyloxallyl glycine; ECM, extracellular matrix; FTY720, fingolimod; GelMA, gelatin methacryloyl; GelMa-G-MBGNs, MGBN with photo-cross-linkable GelMA which have been further integrated into GelMA; HCA, hydroxycarbonated apatite; HIF-1, hypoxia-inducible factor-1; HIF-PH, hypoxia-inducible factor prolyl hydroxylase; IGF-II, insulin-like growth factor II; M, months; MBG, mesoporous bioactive glass; MBGN, mesoporous bioactive glass nanoparticle; MBG-LGO, MBG-low graphene oxide; MBG-HGO, MBG-high graphene oxide; MBG-PHBHHx, MBG-doped poly(3-hydroxybutyrate-co-3-hydroxyhexanoate); Mm, millimeters; MMP-2, matrix metallopeptidase-2; MPHS, MBG with poly(3-hydroxybutyrate-co-3-hydroxyhexanoate); PHMB, BMP-2 + MBG-PHBHHx; PHMBD, BMP-2 + DMOG + MBG-PHBHHx; PHMD, DMOG + MBG-PHBHHx; PHMG, pure MBG-PHBHHx; PLGA, poly(lactic-co-glycolic acid); RANKL, receptor activator of nuclear factor kappa-B ligand; rhBMP-2, recombinant human bone morphogenetic protein-2; TEOS, tetraethyl orthosilicate; TEP, triethyl phosphate; VEGF, vascular endothelial growth factor; W, weeks; Wt, weight.
References
Adair, T., and Montani, J. (2010). “Angiogenesis,” in Integrated Systems Physiology: From Molecules to Function. (San Rafael, CA: Morgan & Claypool Life Sciences), 1–84.
Aronin, C. E. P., Sefcik, L. S., Tholpady, S. S., Tholpady, A., Sadik, K. W., MacDonald, T. L., et al. (2010). FTY720 promotes local microvascular network formation and regeneration of cranial bone defects. Tissue Eng. Part A. 16, 1801–1809. doi: 10.1089/ten.tea.2009.0539
Baer, A., Colon-Moran, W., and Bhattarai, N. (2018). Characterization of the effects of immunomodulatory drug fingolimod (FTY720) on human T cell receptor signaling pathways. Sci. Rep. 8:10910. doi: 10.1038/s41598-018-29355-0
Baino, F., Hamzehlou, S., and Kargozar, S. (2018). Bioactive glasses: where are we and where are we going? J. Func. Biomater. 9:25. doi: 10.3390/jfb9010025
Bose, S., Roy, M., and Bandyopadhyay, A. (2012). Recent advances in bone tissue engineering scaffolds. Trends Biotechnol. 30, 546–554. doi: 10.1016/j.tibtech.2012.07.005
Brauer, D. S. (2015). Bioactive Glasses-Structure and Properties. Angew. Chem. Int. Ed. 54, 4160–4181. doi: 10.1002/anie.201405310
Carlisle, E. M. (1981). Calcified tissue international silicon: a requirement in bone formation independent of vitamin D 1. Calcif. Tissue Int. 33, 27–34.
Cheng, X., Wan, Q., and Pei, X. (2018). Graphene family materials in bone tissue regeneration: perspectives and challenges. Nanosc. Res. Lett. 13:289. doi: 10.1186/s11671-018-2694-z
Day, R. M. (2005). Bioactive glass stimulates the secretion of angiogenic growth factors and angiogenesis in vitro. Tissue Eng. 11, 768–777. doi: 10.1089/ten.2005.11.768
DeLisser, H. M., Christofidou-Solomidou, M., Strieter, R. M., Burdick, M. D., Robinson, C. S., Wexler, R. S., et al. (1997). Involvement of endothelial PECAM-1/CD31 in angiogenesis. Am. J. Pathol. 151, 671–677.
Detsch, R., Stoor, P., Grünewald, A., Roether, J. A., Lindfors, N. C., and Boccaccini, A. R. (2014). Increase in VEGF secretion from human fibroblast cells by bioactive glass S53P4 to stimulate angiogenesis in bone. J. Biomed. Mater. Res. Part A. 102, 4055–4061. doi: 10.1002/jbm.a.35069
Dickson, K., Katzman, S., Delgado, E., and Contreras, D. (1994). Delayed unions and nonunions of open tibial fractures. Correlation with arteriography results. Clin. Orthopaed. Relat. Res. 302, 189–193.
Dimitriou, R., Jones, E., McGonagle, D., and Giannoudis, P. V. (2011). Bone regeneration: current concepts and future directions. BMC Med. 9:66. doi: 10.1186/1741-7015-9-66
Du, J., Xie, P., Lin, S., Wu, Y., Zeng, D., Li, Y., et al. (2018). Time-phase sequential utilization of adipose-derived mesenchymal stem cells on mesoporous bioactive glass for restoration of critical size bone defects. ACS Appl. Mater. Interf. 10, 28340–28350. doi: 10.1021/acsami.8b08563
Ehling, J., Theek, B., Gremse, F., Baetke, S., Möckel, D., Maynard, J., et al. (2014). Micro-CT imaging of tumor angiogenesis: Quantitative measures describing micromorphology and vascularization. Am. J. Pathol. 184, 431–441. doi: 10.1016/j.ajpath.2013.10.014
El Shazley, N., Hamdy, A., El-Eneen, H. A., El Backly, R. M., Saad, M. M., Essam, W., et al. (2016). Bioglass in alveolar bone regeneration in orthodontic patients: randomized controlled clinical trial. JDR Clin. Transl. Res. 1, 244–255. doi: 10.1177/2380084416660672
El-Rashidy, A. A., Roether, J. A., Harhaus, L., Kneser, U., and Boccaccini, A. R. (2017). Regenerating bone with bioactive glass scaffolds: a review of in vivo studies in bone defect models. Acta Biomaterialia, 62, 1–28. doi: 10.1016/j.actbio.2017.08.030
Ferrara, N., Houck, K., Jakeman, L., and Leung, D. W. (1992). Molecular and biological properties of the vascular endothelial growth factor family of proteins. Endocr. Rev. 13, 18–32. doi: 10.1210/edrv-13-1-18
Giannoni, P., Scaglione, S., Daga, A., Ilengo, C., Cilli, M., and Quarto, R. (2010). Short-Time survival and engraftment of bone marrow stromal cells in an ectopic model of bone regeneration. Tissue Eng. Part A 16, 489–499. doi: 10.1089/ten.tea.2009.0041
Gorustovich, A. A., López, J. M. P., Guglielmotti, M. B., and Cabrini, R. L. (2006). Biological performance of boron-modified bioactive glass particles implanted in rat tibia bone marrow. Biomed. Mater. 1, 100–105. doi: 10.1088/1748-6041/1/3/002
Gothard, D., Smith, E. L., Kanczler, J. M., Rashidi, H., Qutachi, O., Henstock, J., et al. (2014). Tissue engineered bone using select growth factors: a comprehensive review of animal studies and clinical translation studies in man. Eur. Cells Mater. 28, 166–208. doi: 10.22203/eCM.v028a13
Handel, M., Hammer, T. R., Nooeaid, P., Boccaccini, A. R., and Hoefer, D. (2013). 45S5-Bioglass(®)-based 3D-scaffolds seeded with human adipose tissue-derived stem cells induce in vivo vascularization in the CAM angiogenesis assay. Tissue Eng. Part A 19, 2703–2712. doi: 10.1089/ten.TEA.2012.0707
Hanks, C. T., Wataha, J. C., and Sun, Z. (1996). In vitro models of biocompatibility: a review. Dental Mater. 12, 186–193. doi: 10.1016/S0109-5641(96)80020-0
Hench, L. L. (1991). Bioceramics: from concept to clinic. J. Am. Ceramic Soc. 74, 1487–1510. doi: 10.1111/j.1151-2916.1991.tb07132.x
Hench, L. L. (2009). Genetic design of bioactive glass. J. Eur. Ceramic Soc. 29, 1257–1265. doi: 10.1016/j.jeurceramsoc.2008.08.002
Hench, L. L., and Jones, J. R. (2015). Bioactive glasses: frontiers and challenges. Front. Bioeng. Biotechnol. 3:194. doi: 10.3389/fbioe.2015.00194
Hench, L. L., Roki, N., and Fenn, M. B. (2014). Bioactive glasses: importance of structure and properties in bone regeneration. J. Mol. Struc. 1073, 24–30. doi: 10.1016/j.molstruc.2014.03.066
Hench, L. L., Splinter, R. J., Allen, W. C., and Greenlee, T. K. (1971). Bonding mechanisms at the interface of ceramic prosthetic materials. J. Biomed. Mater. Res. 5, 117–141. doi: 10.1002/jbm.820050611
Huang, C., Das, A., Barker, D., Tholpady, S., Wang, T., Cui, Q., et al. (2012). Local delivery of FTY720 accelerates cranial allograft incorporation and bone formation. Cell Tissue Res. 347, 553–566. doi: 10.1007/s00441-011-1217-3
Jia, W., Lau, G. Y., Huang, W., Zhang, C., Tomsia, A. P., and Fu, Q. (2015). Bioactive Glass for Large Bone Repair. Adv. Healthcare Mater. 4, 2842–2848. doi: 10.1002/adhm.201500447
Jia, W., Lau, G. Y., Huang, W., Zhang, C., Tomsia, A. P., and Fu, Q. (2019). Cellular response to 3-D printed bioactive silicate and borosilicate glass scaffolds. J. Biomed. Mater. Res. Part B Appl. Biomater. 107, 818–824. doi: 10.1002/jbm.b.34178
Jing, X., Yin, W., Tian, H., Chen, M., Yao, X., Zhu, W., et al. (2018). Icariin doped bioactive glasses seeded with rat adipose-derived stem cells to promote bone repair via enhanced osteogenic and angiogenic activities. Life Sci. 202, 52–60. doi: 10.1016/j.lfs.2018.02.026
Kargozar, S., Baino, F., Hamzehlou, S., Hill, R. G., and Mozafari, M. (2018a). Bioactive glasses: sprouting angiogenesis in tissue engineering. Trends Biotechnol. 36, 430–444. doi: 10.1016/j.tibtech.2017.12.003
Kargozar, S., Mozafari, M., Hashemian, S. J., Brouki Milan, P., Hamzehlou, S., Soleimani, M., et al. (2018b). Osteogenic potential of stem cells-seeded bioactive nanocomposite scaffolds: a comparative study between human mesenchymal stem cells derived from bone, umbilical cord Wharton's jelly, and adipose tissue. J. Biomed. Mater. Res. Part B Appl. Biomater. 106, 61–72. doi: 10.1002/jbm.b.33814
Khan, P. K., Mahato, A., Kundu, B., Nandi, S. K., Mukherjee, P., Datta, S., et al. (2016). Influence of single and binary doping of strontium and lithium on in vivo biological properties of bioactive glass scaffolds. Sc. Rep. 6:32964. doi: 10.1038/srep32964
Kong, N., Lin, K., Li, H., and Chang, J. (2014). Synergy effects of copper and silicon ions on stimulation of vascularization by copper-doped calcium silicate. J. Mater. Chem. B 2, 1100–1110. doi: 10.1039/c3tb21529f
Kyllönen, L., D'Este, M., Alini, M., and Eglin, D. (2015). Local drug delivery for enhancing fracture healing in osteoporotic bone. Acta Biomater. 11, 412–434. doi: 10.1016/j.actbio.2014.09.006
Langer, R. (2000). Biomaterials in drug delivery and tissue engineering: one laboratory's experience. Acc. Chem. Res. 33, 94–101. doi: 10.1021/ar9800993
Larry, R., and Hench, L. (2006). The story of Bioglass. J. Mater. Sci. Mater. Med. 17, 967–978. doi: 10.1007/s10856-006-0432-z
Li, H., and Chang, J. (2013). Bioactive silicate materials stimulate angiogenesis in fibroblast and endothelial cell co-culture system through paracrine effect. Acta Biomater. 9, 6981–6991. doi: 10.1016/j.actbio.2013.02.014
Li, S., Song, C., Yang, S., Yu, W., Zhang, W., Zhang, G., et al. (2019). Supercritical CO2 foamed composite scaffolds incorporating bioactive lipids promote vascularized bone regeneration via Hif-1α upregulation and enhanced type H vessel formation. Acta Biomater, 94, 253–267. doi: 10.1016/j.actbio.2019.05.066
Lu, C., Marcucio, R., and Miclau, T. (2006). Assessing angiogenesis during fracture healing. Iowa Orthopaed. J. 26, 17–26.
Marie, P. J., Felsenberg, D., and Brandi, M. L. (2011). How strontium ranelate, via opposite effects on bone resorption and formation, prevents osteoporosis. Osteopor. Int. 22, 1659–1667. doi: 10.1007/s00198-010-1369-0
Miettinen, M., Lindenmayer, A. E., and Chaubal, A. (1994). Endothelial cell markers CD31, CD34, and BNH9 antibody to H- and Y-antigens–evaluation of their specificity and sensitivity in the diagnosis of vascular tumors and comparison with von Willebrand factor. Modern Pathol. 7, 82–90.
Min, Z., Shichang, Z., Chen, X., Yufang, Z., and Changqing, Z. (2015). 3D-printed dimethyloxallyl glycine delivery scaffolds to improve angiogenesis and osteogenesis. Biomater. Sci. 3, 1236–1244. doi: 10.1039/c5bm00132c
Moseman, R. F. (1994). Chemical disposition of boron in animals and humans. Environ. Health Perspec. 102(Suppl. 7), 113–117. doi: 10.1289/ehp.94102s7113
Nohra, J., Lao, J., Nedelec, J.-M., and Clermont, S. (2014). Effects of strontium-doped bioactive glass on the differentiation of cultured osteogenic cells. Eur. Cell Mater. 21, 130–143. doi: 10.22203/eCM.v021a11
O'Brien, F. J. (2011). Biomaterials & scaffolds for tissue engineering. Mater. Today 14, 88–95. doi: 10.1016/S1369-7021(11)70058-X
Pizzoferrato, A., Ciapetti, G., Stea, S., Cenni, E., Arciola, C. R., Granchi, D., et al. (1994). Cell culture methods for testing biocompatibility. Clin. Mater. 15, 173–190. doi: 10.1016/0267-6605(94)90081-7
Qi, X., Liu, Y., Ding, Z. Y., Cao, J. Q., Huang, J. H., Zhang, J. Y., et al. (2017). Synergistic effects of dimethyloxallyl glycine and recombinant human bone morphogenetic protein-2 on repair of critical-sized bone defects in rats. Sci. Rep. 7:42820. doi: 10.1038/srep42820
Qu, X., He, Z., Qiao, H., Zhai, Z., Mao, Z., Yu, Z., et al. (2018). Serum copper levels are associated with bone mineral density and total fracture. J. Orthopaed. Transl. 14, 34–44. doi: 10.1016/j.jot.2018.05.001
Quinlan, E., Partap, S., Azevedo, M. M., Jell, G., Stevens, M. M., and O'Brien, F. J. (2015). Hypoxia-mimicking bioactive glass/collagen glycosaminoglycan composite scaffolds to enhance angiogenesis and bone repair. Biomaterials 52, 358–366. doi: 10.1016/j.biomaterials.2015.02.006
Rahaman, M. N., Day, D. E., Sonny Bal, B., Fu, Q., Jung, S. B., Bonewald, L. F., et al. (2011). Bioactive glass in tissue engineering. Acta Biomater. 7, 2355–2373. doi: 10.1016/j.actbio.2011.03.016
Rouwkema, J., and Khademhosseini, A. (2016). Vascularization and angiogenesis in tissue engineering: beyond creating static networks. Trends Biotechnol. 34, 733–745. doi: 10.1016/j.tibtech.2016.03.002
Rouwkema, J., Rivron, N. C., and van Blitterswijk, C. A. (2008). Vascularization in tissue engineering. Trends Biotechnol. 26, 434–441. doi: 10.1016/j.tibtech.2008.04.009
Siemerink, M. J., Klaassen, I., Vogels, I. M. C., Griffioen, A. W., Van Noorden, C. J. F., and Schlingemann, R. O. (2012). CD34 marks angiogenic tip cells in human vascular endothelial cell cultures. Angiogenesis 15, 151–163. doi: 10.1007/s10456-011-9251-z
Stegen, S., van Gastel, N., and Carmeliet, G. (2015). Bringing new life to damaged bone: the importance of angiogenesis in bone repair and regeneration. Bone 70, 19–27. doi: 10.1016/j.bone.2014.09.017
Strem, B. M., Hicok, K. C., Zhu, M., Wulur, I., Alfonso, Z., Schreiber, R. E., et al. (2005). Multipotential differentiation of adipose tissue-derived stem cells. Keio J. Med. 54, 132–141. doi: 10.2302/kjm.54.132
Tabia, Z., El Mabrouk, K., Bricha, M., and Nouneh, K. (2019). Mesoporous bioactive glass nanoparticles doped with magnesium: drug delivery and acellular: In vitro bioactivity. RSC Adv. 9, 12232–12246. doi: 10.1039/c9ra01133a
Taichman, R. S. (2005). Blood and bone: Two tissues whose fates are intertwined to create the hematopoietic stem-cell niche. Blood 105, 2631–2639. doi: 10.1182/blood-2004-06-2480
Tonino, P., and Abreu, C. (2011). Microvessel density is associated with VEGF and α-SMA expression in different regions of human gastrointestinal carcinomas. Cancers 3, 3405–3418. doi: 10.3390/cancers3033405
van Lenthe, G. H., Hagenmüller, H., Bohner, M., Hollister, S. J., Meinel, L., and Müller, R. (2007). Nondestructive micro-computed tomography for biological imaging and quantification of scaffold-bone interaction in vivo. Biomaterials 28, 2479–2490. doi: 10.1016/j.biomaterials.2007.01.017
Wagoner Johnson, A. J., and Herschler, B. A. (2011). A review of the mechanical behavior of CaP and CaP/polymer composites for applications in bone replacement and repair. Acta Biomater. 7, 16–30. doi: 10.1016/j.actbio.2010.07.012
Wang, W., Liu, Y., Yang, C., Qi, X., Li, S., Liu, C., et al. (2019). Mesoporous bioactive glass combined with graphene oxide scaffolds for bone repair. Int. J. Biol. Sci. 15, 2156–2169. doi: 10.7150/ijbs.35670
White, C., Alshaker, H., Cooper, C., Winkler, M., and Pchejetski, D. (2016). The emerging role of FTY720 (Fingolimod) in cancer treatment. Oncotarget 7, 23106–23127. doi: 10.18632/oncotarget.7145
Wu, C., Zhou, Y., Chang, J., and Xiao, Y. (2013a). Delivery of dimethyloxallyl glycine in mesoporous bioactive glass scaffolds to improve angiogenesis and osteogenesis of human bone marrow stromal cells. Acta Biomater. 9, 9159–9168. doi: 10.1016/j.actbio.2013.06.026
Wu, C., Zhou, Y., Xu, M., Han, P., Chen, L., Chang, J., et al. (2013b). Copper-containing mesoporous bioactive glass scaffolds with multifunctional properties of angiogenesis capacity, osteostimulation and antibacterial activity. Biomaterials 34, 422–433. doi: 10.1016/j.biomaterials.2012.09.066
Wu, J., Zheng, K., Huang, X., Liu, J., Liu, H., Boccaccini, A. R., et al. (2019). Thermally triggered injectable chitosan/silk fibroin/bioactive glass nanoparticle hydrogels for in-situ bone formation in rat calvarial bone defects. Acta Biomater. 91, 60–71. doi: 10.1016/j.actbio.2019.04.023
Xin, T., Gu, Y., Cheng, R., Tang, J., Sun, Z., Cui, W., et al. (2017). Inorganic strengthened hydrogel membrane as regenerative periosteum. ACS Appl. Mater. Interf. 9, 41168–41180. doi: 10.1021/acsami.7b13167
Xynos, I. D., Edgar, A. J., Buttery, L. D. K., Hench, L. L., and Polak, J. M. (2001). Gene-expression profiling of human osteoblasts following treatment with the ionic products of Bioglass- 45S5 dissolution. J. Biomed. Mater. Res. 55, 151–157. doi: 10.102/1097-4636(200105)55:2<151::AID-JBM1001>3.0.CO;2-D
Xynos, I. D., Hukkanen, M. V. J., Batten, J. J., Buttery, L. D., Hench, L. L., and Polak, J. M. (2000). Bioglass ®45S5 stimulates osteoblast turnover and enhances bone formation in vitro: Implications and applications for bone tissue engineering. Calcif. Tissue Int. 67, 321–329. doi: 10.1007/s002230001134
Zarins, J., Pilmane, M., Sidhoma, E., and Salma, I. (2017). Does local application of strontium increase osteogenesis and biomaterial osteointegration in osteoporotic and other bone tissue conditions: review of literature. Acta Chirurgica Lat. 16, 17–23. doi: 10.1515/chilat-2017-0004
Zhai, W., Lu, H., Chen, L., Lin, X., Huang, Y., Dai, K., et al. (2012). Silicate bioceramics induce angiogenesis during bone regeneration. Acta Biomater. 8, 341–349. doi: 10.1016/j.actbio.2011.09.008
Zhang, F., Zhou, L., Zhou, Z., Dai, C., Fan, L., Li, C., et al. (2019). Bioactive glass functionalized chondroitin sulfate hydrogel with proangiogenic properties. Biopolymers 110:e23328. doi: 10.1002/bip.23328
Zhang, J., Guan, J., Qi, X., Ding, H., Yuan, H., Xie, Z., et al. (2016). Dimethyloxaloylglycine promotes the angiogenic activity of mesenchymal stem cells derived from iPSCs via activation of the PI3K/Akt pathway for bone regeneration. Int. J. Biol. Sci. 12, 639–652. doi: 10.7150/ijbs.14025
Keywords: scaffolds, bone, bioactive glass, osteogenesis, angiogenesis
Citation: Ranmuthu CDS, Ranmuthu CKI, Russell JC, Singhania D and Khan WS (2020) Evaluating the Effect of Non-cellular Bioactive Glass-Containing Scaffolds on Osteogenesis and Angiogenesis in in vivo Animal Bone Defect Models. Front. Bioeng. Biotechnol. 8:430. doi: 10.3389/fbioe.2020.00430
Received: 09 December 2019; Accepted: 15 April 2020;
Published: 14 May 2020.
Edited by:
Francesco Baino, Politecnico di Torino, ItalyReviewed by:
Anuj Kumar, Yeungnam University, South KoreaSaeid Kargozar, Mashhad University of Medical Sciences, Iran
Emanuel Ionescu, Darmstadt University of Technology, Germany
Copyright © 2020 Ranmuthu, Ranmuthu, Russell, Singhania and Khan. This is an open-access article distributed under the terms of the Creative Commons Attribution License (CC BY). The use, distribution or reproduction in other forums is permitted, provided the original author(s) and the copyright owner(s) are credited and that the original publication in this journal is cited, in accordance with accepted academic practice. No use, distribution or reproduction is permitted which does not comply with these terms.
*Correspondence: Wasim S. Khan, wk280@cam.ac.uk