A Mini-Review on Hydrogen-Rich Syngas Production by Thermo-Catalytic and Bioconversion of Biomass and Its Environmental Implications
- Institute of Energy Policy and Research, Universiti Tenaga Nasional, Kajang, Malaysia
The thermo-catalytic and biochemical conversion of biomass to hydrogen-rich syngas has been widely reported with less emphasis on the environmental implications of the processes. This mini-review presents an overview of different thermo-catalytic route of converting biomass to hydrogen-rich syngas as well as their environmental impact investigated using life cycle assessment methodology. The review revealed that most of the authors employed, biomass gasification, biomass pyrolysis, reforming and fermentative processes for the hydrogen-rich syngas production. Global warming potential was observed as the most significant environmental impact reported in the reviewed articles. The CO2 equivalent emissions were found to varies with each of the processes and the type of feedstock used. Trends from literature shows that both thermo-catalytic and biochemical processes have competitive advantages and potential to compete favorable with the existing technology used for hydrogen production. Nevertheless, it cannot be ascertained that these technologies should be excluded from environmental burdens. This mini-review could be a quick guide to future research interest in environmental impact of hydrogen-rich syngas production by thermo-catalytic and biochemical conversion of biomass.
Introduction
Most of the energy demand globally is sourced from fossil sources such as coal, natural gas, and petroleum (Bölük and Mert, 2014). The utilization of these fossil-based energy often resulted in the emissions of greenhouses gases which are primarily responsible for global warming (Ayodele et al., 2017). Hence, the quest for a more sustainable and cleaner energy source. The challenges encountered using energy derived from fossil source could be reduced by the use of renewable energy source such as biomass as an alternative to fossil fuel (REN21, 2018). Biomass as the fourth most abundant sources of energy are components of everyday life and can be obtained from various sources such as animal wastes, industrial wastes, municipal solid wastes, food processing wood, agricultural residues such as straw, stover, cane trash, and green agricultural waste (Yamakawa et al., 2018). These biomass-based feedstocks have the advantages of being converted directly into more valuable end products in the form of gas, liquid, and solids through different technological routes (Landis et al., 2018). Besides, it is renewable, sustainable, its utilization has less negative environmental impact compared to energy from fossil sources (Baykara, 2018). Biomass are renewable materials that can be used directly as fuel or as feedstock for the production of value- added products (Valle et al., 2018; Barbuzza et al., 2019).
According to Dhillon and von Wuehlisch (2013) biomass as renewable feedstock is a worthwhile substitute to the depleted fossil fuel resources for the production of energy and value-added products. Demirbas (2009) classified biomass feedstock into 9 categories namely forest residues, bio-renewable waste, energy crops, sugar crops, aquatic plants, landfill, food crops, industrial organic waste. Forest residues consists of wood, logging residues, trees, shrubs and wood residues, and sawdust. Forest residues find wide applications as feedstocks for the production of biofuels or intermediates such as bio-syngas (BioSNG), biocrude, methanol, and biodimethylether via thermochemical processes. Bio-renewable waste which include agricultural wastes, crop residues, mill wood wastes, urban wood wastes, urban organic wastes are organic materials from plant and animals in waste form. Energy crops which could be woody or herbaceous are crops grown for the sole purpose of being used as feedstock for production of bioenergy. Energy crops include short rotation woody crops, herbaceous woody crops, grasses, starch crops, and sugar crops. Industrial organic waste are the wastes obtained from industrial activities such as palm oil processing, brewery, and olive oil production. These include palm oil mill effluent, brewery waste, and olive oil mill wastewater. Although, 95% of hydrogen produced commercially was obtained from natural gas reforming which is to a large extent not environmentally friendly and not sustainable (Kaiwen et al., 2017).
Two of the most prominent means of converting biomass is thermo-catalytic and bio-chemical pathways (Damartzis and Zabaniotou, 2011; Tyagi et al., 2014). Thermo-catalytic routes include processes such as gasification, reforming, pyrolysis while the biochemical route involve the fermentative process (Parthasarathy and Narayanan, 2014; Shahirah et al., 2016; Kaiwen et al., 2017; Yamakawa et al., 2018). The product of the various technical pathways produces gases such as, H2, CO, CO2, CH4, and light gaseous hydrocarbon. The use of catalyst coupled with separation technology can help to improve the selectivity of hydrogen in the gaseous stream (Sumrunronnasak et al., 2016). Besides, syngas, a mixture of H2 and CO could be used as a chemical intermediate for the production of gasoline and other oxygenates via Fischer-Tropsch synthesis (Ayodele et al., 2016). Although, research interest has been focused on how the various conversion processes can be improved to maximize productivity, there is an increasing attention on the potential environmental impact of using the various technical pathways for hydrogen-rich syngas production (Bhandari et al., 2014). This mini-review therefore focuses on the overview of the analysis of the thermo-catalytic and the biochemical routes for hydrogen-rich syngas production and their environmental impacts from life cycle assessment studies reported in published literature. There are extensive review papers on hydrogen production by methane decomposition (Abbas and Wan Daud, 2010), agricultural waste by dark fermentation (Guo et al., 2010; Ghimire et al., 2015), chemical and photochemical conversion of hydrogen sulfide (Reverberi et al., 2016), thermochemical conversion of biomass (Arregi et al., 2018; Salam et al., 2018), by chemical looping technology (Luo et al., 2018). None of these reviews addressed the environmental implications of producing hydrogen-rich syngas by thermo-catalytic and biochemical conversion of biomass which is the focus of this mini-review.
Recent Progress and Pathways for Biomass Conversion to Hydrogen-Rich Syngas
Biomass feedstocks can be converted to hydrogen-rich syngas via thermo-catalytic and biochemical pathways as shown in Figure 1. The thermo-catalytic pathways include gasification, reforming, and pyrolysis while the biochemical pathway involve mainly fermentative processes. Aside, pyrolysis which has hydrocarbons in the forms of biooil as one the major products, the main gaseous products of gasification, reforming and fermentative processes are H2, CO, CO2, CH4, and C2H4.
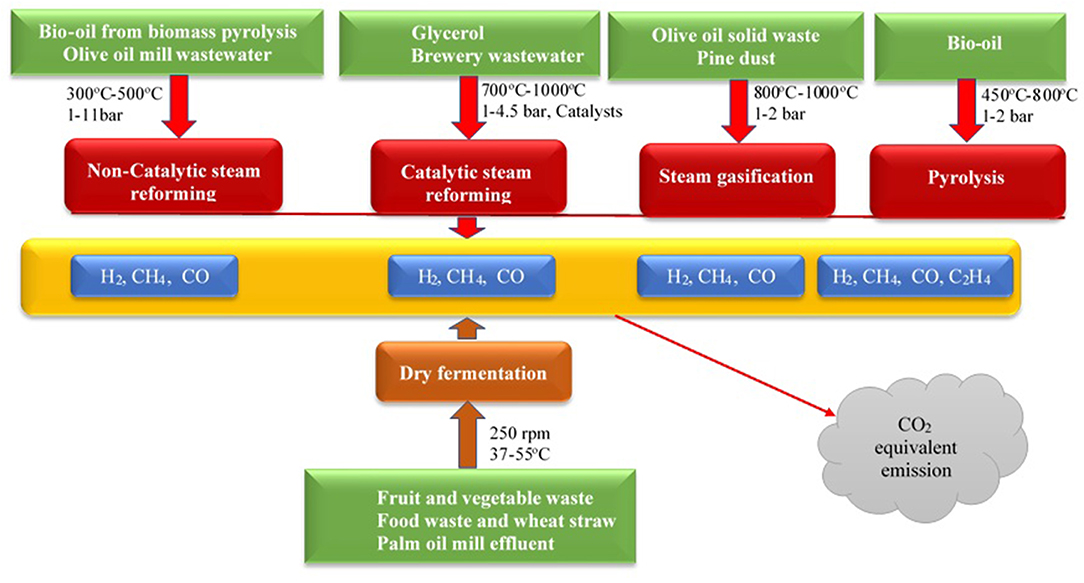
Figure 1. An overview of various thermo-catalytic and fermentative processes used for hydrogen-rich syngas production.
Biomass Gasification
The production of hydrogen by biomass gasification is a well-established technology that employed a controlled process that utilizes heat, steam, and oxygen for the conversion of biomass to hydrogen-rich syngas without the occurrence of combustion (Parthasarathy and Narayanan, 2014; Barbuzza et al., 2019). Biomass gasification often occurs at high temperature (> 700°C). The simplified reaction for biomass gasification in depicted in Equation (1). Equation (1) often occur concurrently with water gas shift reaction where the CO is subsequently converted to H2 as shown in Equation (2) (Abdul Mujeebu, 2016; Zribi et al., 2019).
Hydrogen production by biomass gasification has been investigated by Zribi et al. (2019) using Olive mill solid waste and pine sawdust as feedstock. The gasification of the Olive mill solid waste and pine sawdust were conducted at temperature ranges from 750 to 900°C. using 10–30% steam. The findings show that increase in the gasification temperature and steam partial pressure resulted in a corresponding increase in the hydrogen yield. The major technical constraint of biomass gasification is the formation of tars which are the main sources of impurities to the syngas produced. In addition, the formation of CO2 as one of the gasification products could also have some environmental impact. In view of this, Valderrama Rios et al. (2018) suggested the optimization of the process conditions and tars removal through in situ or post-gasification treatment as plausible means of reducing the challenges of biomass gasification.
Biomass Reforming
Liquid-form of biomass such as glycerol, ethanol, methanol, and bio-oil can be employed for the production of hydrogen-rich syngas by reforming process (Arif et al., 2019; Schmitt et al., 2019). Liquid biomass reforming is similar to the process obtained in natural gas reforming which is a mature technology for hydrogen production. The liquid biomass reforming process typically involve the reaction of the biomass-derived liquid with steam, CO2, or O2 at high temperature usually in the presence of a catalyst (Huang et al., 2018; Arif et al., 2019). The reforming reaction often produces hydrogen-rich syngas and some hydrocarbons. The main reaction occurs simultaneously with the water-gas shift reaction which covert the CO produced in the main reaction to H2 and CO2. Several authors have investigated hydrogen production by catalytic reforming of liquid derived from biomass. Silva et al. (2019) investigated steam reforming of biomass-derived glycerol over Rh/Al2O3 catalyst. The steam reforming of the glycerol at 400°C and 4.5 bar resulted in the maximum hydrogen production of 2.6 mol per glycerol fed. Beside using catalyst, the liquid biomass derived reforming can be done in the absence of catalyst as reported by Soria et al. (2019) and Rocha et al. (2017). Soria et al. (2019) reported hydrogen production by non-catalytic steam reforming of bio-oil obtained from biomass pyrolysis at 400°C and 1 bar to obtain H2 yield of 2.5 mol%. In a similar study by Rocha et al. (2017), non-catalytic steam reforming was employed to produce hydrogen from olive oil mill wastewater at temperature range of 300°−500°C and 1–11 bar. The thermodynamic analysis of the process revealed that H2 yield decreases with increase in the pressure and increases with increase in the reaction temperature. Maximum hydrogen yield of 5.5 mol% was obtained at 500°C and 1 bar. In comparison with the biomass gasification process, biomass reforming offers the advantages of producing a purer syngas. However, technical issues such as catalyst deactivation by carbon deposition and sintering, high energy requirement, and lack of understanding of the deactivation mechanisms have been identified as major constraints of the biomass reforming process (Ayodele et al., 2019). Appropriate catalyst design, kinetics of the biomass reforming reaction and optimization of the process conditions have been proposed as means of overcoming these challenges.
Biomass Pyrolysis
Biomass pyrolysis entails the gasification of biomass in the absence of oxygen (Moud et al., 2018). Hydrogen production by biomass pyrolysis is presently receiving research attention as a plausible alternative for hydrogen production from biomass. Although, one of the major products of biomass pyrolysis is biooil, the oil can in turn be pyrolyzed or reformed at temperature range of 450–850°C to produce hydrogen-rich syngas (Chen et al., 2016). Biomass pyrolysis usually resulted in gaseous, liquid and solid products (Gallezot, 2012). During the pyrolytic reaction, the long chains of carbon, hydrogen, and oxygen contained in the biomass are broken into smaller chain of molecules such as gases, tars and bio-oils (Shen et al., 2016). The decomposition rate during biomass pyrolysis is often influenced by process parameters such as the reaction temperature, the heating rate of the biomass, reactor pressure, the composition of the biomass, and the reactor configuration (Oliveiramaia et al., 2018). One main advantage of the biomass pyrolysis is the flexible nature of allowing different types of biomass such as agriculture residues, wood wastes, and municipal solid waste as feedstocks for production of clean energy. Besides, biomass pyrolysis has high efficiency and acceptable environmental performance characteristics (Moud et al., 2018).
Fermentative Process
The fermentative hydrogen production is a type of anaerobic conversions that involves the conversion of biomass feedstocks using bacteria and protozoa which employs enzymes (Azwar et al., 2014). Hydrogen production by fermentative process is strongly dependent on factors such the type of the microorganisms, carbon source, nitrogen source, pH and the reactor temperature (Abubackar et al., 2019). The fermentative processes can be performed either in the presence of light (photo-fermentation) or in the absence of light (Dark-fermentation) (Wang et al., 2014; Abubackar et al., 2019). Several authors have reported hydrogen production by fermentative process using biomass feedstocks such as palm oil mill effluent, fruit and vegetable waste. Keskin et al. (2019) reported the conversion of fruit and vegetable waste to hydrogen by dry fermentative techniques using a dark fermenter. The fermentation of the fruit and vegetable waste performed at 37°C and 250 rpm resulted in the production of 53–68 mL H2/g VS using 2 percolation per day. In a similar study, Abubackar et al. (2019) investigated the production of hydrogen from fruit and vegetable waste using dry fermentation technique. Unlike the work of Keskin et al. (2019) the fruit and vegetable wastes were treated using autoclaved prior to the fermentation process. The fermentation process conducted at 250 rpm, 55°C resulted in 27.19 H2/g VS. It can be seen that there is variation in the values of H2 produced from the work of Abubackar et al. (2019) and Keskin et al. (2019) which could be attributed to the differences in the process conditions such as the fermenter temperature. The production of H2 by dark fermentation has been reported by Maaroff et al. (2019) and Ghimire et al. (2018) using palm oil mill effluent, food waste, and wheat straw. Maaroff et al. (2019) employed two-stage sequencing batch reactor system for the fermentation of the palm oil mill effluent to obtain 10.34 mmol H2/L.h. While Ghimire et al. (2018) performed solid state dark fermentation of the food waste and wheat straw to obtain hydrogen. The authors reported that H2 production was inhibited at total solid content higher than 15%. The main challenges with the fermentative process are the slow production rate and smaller yield of H2 produced compared to the thermo-catalytic processes.
Environmental Implications via Life Cycle Assessment Analysis of the Different Thermo-Catalytic and Biochemical Pathways
The growing concern about environmental pollution from the anthropogenic utilization of energy derived from fossil fuel has raised the concern for alternative, substantiable, and environmentally friendly energy source (Mundaca, 2017). Several studies have revealed that the production of energy from biomass derived feedstock have emerged as plausible means of mitigating greenhouse gas emissions (Bölük and Mert, 2014). To ascertain this clam, life cycle assessment has been adjudged as a significant technique to investigate the different environmental impact of renewable energy production from different feedstocks (Djomo and Blumberga, 2011). Moreover, hydrogen energy has been identified as the cleanest form of energy with zero emissions of greenhouse gases. In line with this several studies have been conducted to investigate the life cycle assessment of the hydrogen-rich syngas production from different biomass derived feedstocks as summarize in S1 (Supplementary Material). Generally, in all of these studies, the system boundary usually employed for the thermo-catalytic conversion process include biomass production, biomass processing and the afterlife of the production processes. In this review, biomass such as short-rotation poplar biomass, pine, eucalyptus, almond pruning, vine pruning, poplar wood chips, corn stovers, sweet potato peels, sweet sorghum stalk, wheat straw, glycerol, poultry tallow, and bioethanol were used as feed stocks for the production of hydrogen-rich syngas (Djomo and Blumberga, 2011; Dufour et al., 2012; Hajjaji, 2014). The output streams of each of these processes were found to contain mainly H2, CO, CO2, CH4, and hydrocarbons in the form of bio-oil.
Environmental impact assessment of each of the processes for hydrogen-rich syngas production differs in accordance with the operating conditions such as reaction temperature, pressure, reactor configuration, heating rates, the nature of microorganism, the types of catalyst and so on. It can be seen that amongst the thermo-catalytic processes for hydrogen-rich syngas production, biomass pyrolysis has the highest CO2 equivalent emissions compared to other processes (Iribarren et al., 2012; Susmozas et al., 2016). Although, there is no unify bases to compare all these process in terms of the environmental impact of the production activities due to the differences in equipment, process conditions and feedstocks. The combinations of water gas shift reaction with reforming process tends to reduce the CO2 equivalent emissions as reported by Dufour and Moreno (2011). Nevertheless, each of the feedstocks was found to have a defined environmental impacted which was reflected from the CO2 equivalent emissions. On the average, the fermentative process of hydrogen rich-syngas production tends to have lesser environmental impact in terms of the CO2 equivalent emissions (Djomo et al., 2008; Djomo and Blumberga, 2011). The study by Djomo and Blumberga (2011) revealed that the LCA analysis of hydrogen production using sweet potato peels, sweet sorghum stalks and wheat straw as feedstocks resulted in CO2 equivalent emissions of 5.18, 5.32 and 5.6, respectively. However, the use of 2-step fermentative process of the potato peels resulted in a lower CO2 equivalent emission 5.11. This implies that an improvement in a production process could enhance the reduction of CO2 equivalent emissions.
Practical Implications of the Study and Future Research Outlook
The conversion of biomass to hydrogen-rich syngas by thermo-catalytic and biological means presents a lot of technical challenges. Besides, these technological pathways used for the production of hydrogen-rich syngas is not entirely free from environmental burdens in terms of equivalent CO2 emission. Therefore, research attention must be focus on redesigning and optimization of the process parameters of the thermo-catalytic and biological conversion of biomass to hydrogen-rich syngas with the main goal of minimizing the CO2 equivalent emission. Moreover, appropriate optimization strategies combine with kinetic and mechanistic studies of the reaction pathways could help in resolving some the key challenges. Besides, a future research outlook could provide a platform for LCA analysis of different thermo-catalytic and biochemical pathway using a single feedstock per term.
Conclusion
In this study, a mini-review on hydrogen-rich syngas production from various biomass-derived feedstocks using thermo-catalytic and biological pathways as well as their environmental impacts has been presented. The end products of each of the conversion process is dependent on the types of feedstock, the conversion technology, and process conditions. The analysis of the reviewed papers based on LCA have ascertained that energy production from renewable source such as biomass is not totally free from environmental impacts. Global warming impact was found to be the main environmental concern based on the CO2 equivalent emissions. The CO2 equivalent emissions vary with the type of biomass conversion pathways with the fermentative process having the least environmental impact. The limitations of the LCA analysis of hydrogen-rich syngas production from the different thermo-catalytic route is the lack of defined basis for comparative analysis of the different pathways based on environmental metrics.
Author Contributions
BA developed the concept and wrote the manuscript. SM, TT, and SS modified the concept and proofread the manuscript for language and technicalities.
Funding
The authors would like to acknowledge the financial support of Universiti Tenaga Nasional, Malaysia through BOLD2025 researchers grant (10436494/B2019141).
Conflict of Interest
The authors declare that the research was conducted in the absence of any commercial or financial relationships that could be construed as a potential conflict of interest.
References
Abbas, H. F., and Wan Daud, W. M. A. (2010). Hydrogen production by methane decomposition: a review. Int. J. Hydrogen Energy 35, 1160–1190. doi: 10.1016/j.ijhydene.2009.11.036
Abdul Mujeebu, M. (2016). Hydrogen and syngas production by superadiabatic combustion – a review. Appl. Energy 173, 210–224. doi: 10.1016/j.apenergy.2016.04.018
Abubackar, H. N., Keskin, T., Yazgin, O., Gunay, B., Arslan, K., and Azbar, N. (2019). Biohydrogen production from autoclaved fruit and vegetable wastes by dry fermentation under thermophilic condition. Int. J. Hydrogen Energy 44, 18776–18784. doi: 10.1016/j.ijhydene.2018.12.068
Arif, N. N. M., Abidin, S. Z., Osazuwa, O. U., Vo, D. V. N., Azizan, M. T., and Taufiq-Yap, Y. H. (2019). Hydrogen production via CO2 dry reforming of glycerol over ReNi/CaO catalysts. Int. J. Hydrogen Energy 44, 20857–20871. doi: 10.1016/j.ijhydene.2018.06.084
Arregi, A., Amutio, M., Lopez, G., Bilbao, J., and Olazar, M. (2018). Evaluation of thermochemical routes for hydrogen production from biomass: a review. Energy Convers. Manag. 165, 696–719. doi: 10.1016/j.enconman.2018.03.089
Ayodele, B., Abdullah, B. T. A., Alsaffar, M. A., Mustapa, S. I., and Saleh, S. F. (2019). Recent advances in renewable hydrogen production by thermo-catalytic conversion of biomass-derived glycerol : overview of prospects and challenges. Int. J. Hydrogen Energy (in press). doi: 10.1016/j.ijhydene.2019.08.002
Ayodele, B. V., Hossain, S. S., Lam, S. S., Osazuwa, O. U., Khan, M. R., and Cheng, C. K. (2016). Syngas production from CO2 reforming of methane over neodymium sesquioxide supported cobalt catalyst. J. Nat. Gas Sci. Eng. 34, 873–885. doi: 10.1016/j.jngse.2016.07.059
Ayodele, B. V., Khan, M. R., and Cheng, C. K. (2017). Greenhouse gases abatement by catalytic dry reforming of methane to syngas over samarium oxide-supported cobalt catalyst. Int. J. Environ. Sci. Technol. 14, 2769–2782. doi: 10.1007/s13762-017-1359-2
Azwar, M. Y., Hussain, M. A., and Abdul-Wahab, A. K. (2014). Development of biohydrogen production by photobiological, fermentation and electrochemical processes: a review. Renew. Sustain. Energy Rev. 31, 158–173. doi: 10.1016/j.rser.2013.11.022
Barbuzza, E., Buceti, G., Pozio, A., Santarelli, M., and Tosti, S. (2019). Gasification of wood biomass with renewable hydrogen for the production of synthetic natural gas. Fuel 242, 520–531. doi: 10.1016/j.fuel.2019.01.079
Baykara, S. Z. (2018). Hydrogen: a brief overview on its sources, production and environmental impact. Int. J. Hydrogen Energy 43, 10605–10614. doi: 10.1016/j.ijhydene.2018.02.022
Bhandari, R., Trudewind, C. A., and Zapp, P. (2014). Life cycle assessment of hydrogen production via electrolysis e a review. J. Clean. Prod. 85, 151–163. doi: 10.1016/j.jclepro.2013.07.048
Bölük, G., and Mert, M. (2014). Fossil & renewable energy consumption, GHGs (greenhouse gases) and economic growth: Evidence from a panel of EU (European Union) countries. Energy 74, 439–446. doi: 10.1016/j.energy.2014.07.008
Chen, F., Wu, C., Dong, L., Vassallo, A., Williams, P. T., and Huang, J. (2016). Characteristics and catalytic properties of Ni/CaAlOx catalyst for hydrogen-enriched syngas production from pyrolysis-steam reforming of biomass sawdust. Appl. Catal. B Environ. 183, 168–175. doi: 10.1016/j.apcatb.2015.10.028
Damartzis, T., and Zabaniotou, A. (2011). Thermochemical conversion of biomass to second generation biofuels through integrated process design—A review. Renew. Sustain. Energy Rev. 15, 366–378. doi: 10.1016/j.rser.2010.08.003
Demirbas, A. (2009). Biorefineries: current activities and future developments. Energy Convers. Manag. 50, 2782–2801. doi: 10.1016/j.enconman.2009.06.035
Dhillon, R. S., and von Wuehlisch, G. (2013). Mitigation of global warming through renewable biomass. Biomass Bioenergy 48, 75–89. doi: 10.1016/j.biombioe.2012.11.005
Djomo, S. N., and Blumberga, D. (2011). Comparative life cycle assessment of three biohydrogen pathways. Bioresour. Technol. 102, 2684–2694. doi: 10.1016/j.biortech.2010.10.139
Djomo, S. N., Humbert, S., and Dagnija, B. (2008). Life cycle assessment of hydrogen produced from potato steam peels. Int. J. Hydrogen Energy 33, 3067–3072. doi: 10.1016/j.ijhydene.2008.02.006
Dufour, J., and Moreno, J. (2011). Life cycle assessment of hydrogen production from biomass gasification. Evaluation of different feedstock. Int. J. Hydrogen Energy 37, 14026–14039. doi: 10.1016/j.ijhydene.2012.06.015
Dufour, J., Serrano, D. P., Gálvez, J. L., González, A., Soria, E., and Fierro, J. L. G. (2012). Life cycle assessment of alternatives for hydrogen production from renewable and fossil sources. Int. J. Hydrogen Energy 37, 1173–1183. doi: 10.1016/j.ijhydene.2011.09.135
Gallezot, P. (2012). Conversion of biomass to selected chemical products. Chem. Soc. Rev. 41, 1538–1558. doi: 10.1039/C1CS15147A
Ghimire, A., Frunzo, L., Pirozzi, F., Trably, E., Escudie, R., Lens, P. N. L., et al. (2015). A review on dark fermentative biohydrogen production from organic biomass: process parameters and use of by-products. Appl. Energy 144, 73–95. doi: 10.1016/j.apenergy.2015.01.045
Ghimire, A., Trably, E., Frunzo, L., Pirozzi, F., Lens, P. N. L., Esposito, G., et al. (2018). Effect of total solids content on biohydrogen production and lactic acid accumulation during dark fermentation of organic waste biomass. Bioresour. Technol. 248, 180–186. doi: 10.1016/j.biortech.2017.07.062
Guo, X. M., Trably, E., Latrille, E., Carrre, H., and Steyer, J. P. (2010). Hydrogen production from agricultural waste by dark fermentation: a review. Int. J. Hydrogen Energy 35, 10660–10673. doi: 10.1016/j.ijhydene.2010.03.008
Hajjaji, N. (2014). Thermodynamic investigation and environment impact assessment of hydrogen production from steam reforming of poultry tallow. Energy Convers. Manag. 79, 171–179. doi: 10.1016/j.enconman.2013.12.018
Huang, C., Xu, C., Wang, B., Hu, X., Li, J., Liu, J., et al. (2018). High production of syngas from catalytic steam reforming of biomass glycerol in the presence of methane. Biomass Bioenergy 119, 173–178. doi: 10.1016/j.biombioe.2018.05.006
Iribarren, D., Peters, J. F., and Dufour, J. (2012). Life cycle assessment of transportation fuels from biomass pyrolysis. Fuel 97, 812–821. doi: 10.1016/j.fuel.2012.02.053
Kaiwen, L., Bin, Y., and Tao, Z. (2017). Economic analysis of hydrogen production from steam reforming process: a literature review. Energy Sour. Part B Econ. Plan. Policy 13, 109–115. doi: 10.1080/15567249.2017.1387619
Keskin, T., Abubackar, H. N., Yazgin, O., Gunay, B., and Azbar, N. (2019). Effect of percolation frequency on biohydrogen production from fruit and vegetable wastes by dry fermentation. Int. J. Hydrogen Energy. 44, 18767–18775. doi: 10.1016/j.ijhydene.2018.12.099
Landis, D. A., Gratton, C., Jackson, R. D., Gross, K. L., Duncan, D. S., Liang, C., et al. (2018). Biomass and bioenergy biomass and biofuel crop effects on biodiversity and ecosystem services in the North Central US. Biomass Bioenergy 114, 18–29. doi: 10.1016/j.biombioe.2017.02.003
Luo, M., Yi, Y., Wang, S., Wang, Z., Du, M., Pan, J., et al. (2018). Review of hydrogen production using chemical-looping technology. Renew. Sustain. Energy Rev. 81, 3186–3214. doi: 10.1016/j.rser.2017.07.007
Maaroff, R. M., Md Jahim, J., Azahar, A. M., Abdul, P. M., Masdar, M. S., Nordin, D., et al. (2019). Biohydrogen production from palm oil mill effluent (POME) by two stage anaerobic sequencing batch reactor (ASBR) system for better utilization of carbon sources in POME. Int. J. Hydrogen Energy 3395–3406. doi: 10.1016/j.ijhydene.2018.06.013
Moud, P. H., Kantarelis, E., Andersson, K. J., and Engvall, K. (2018). Biomass pyrolysis gas conditioning over an iron-based catalyst for mild deoxygenation and hydrogen production. Fuel 211, 149–158. doi: 10.1016/j.fuel.2017.09.062
Mundaca, G. (2017). How much can CO2emissions be reduced if fossil fuel subsidies are removed? Energy Econ. 64, 91–104. doi: 10.1016/j.eneco.2017.03.014
Oliveiramaia, D., De Martinna, A., Souzachagas, D., Mabel, A., Araújo, D. M., Lemos, F. C. D., et al. (2018). Catalytic pyrolysis of glycerol in the presence of Nickel (II) Schiff base complex supported in SBA-15: kinetic and products (TG-FTIR and PY-CG / MS). Thermochim. Acta 669, 160–168. doi: 10.1016/j.tca.2018.09.005
Parthasarathy, P., and Narayanan, K. S. (2014). Hydrogen production from steam gasification of biomass: influence of process parameters on hydrogen yield - A review. Renew. Energy 66, 570–579. doi: 10.1016/j.renene.2013.12.025
REN21 (2018). Renewables 2018 Global Status Report. Renewable Energy Policy Network for the 21st Century.
Reverberi, A., Pietro Klemeš, J. J., Varbanov, P. S., and Fabiano, B. (2016). A review on hydrogen production from hydrogen sulphide by chemical and photochemical methods. J. Clean. Prod. 136, 72–80. doi: 10.1016/j.jclepro.2016.04.139
Rocha, C., Soria, M. A., and Madeira, L. M. (2017). Steam reforming of olive oil mill wastewater with in situ hydrogen and carbon dioxide separation – Thermodynamic analysis. Fuel 207, 449–460. doi: 10.1016/j.fuel.2017.06.111
Salam, M. A., Ahmed, K., Akter, N., Hossain, T., and Abdullah, B. (2018). A review of hydrogen production via biomass gasification and its prospect in Bangladesh. Int. J. Hydrogen Energy 43, 14944–14973. doi: 10.1016/j.ijhydene.2018.06.043
Schmitt, N., Apfelbacher, A., Jäger, N., Daschner, R., Stenzel, F., and Hornung, A. (2019). Thermo-chemical conversion of biomass and upgrading to biofuel: the Thermo-Catalytic Reforming process – A review. Biofuels Bioprod. Biorefining 13, 822–837. doi: 10.1002/bbb.1980
Shahirah, M. N. N., Ayodele, B. V., Gimbun, J., and Cheng, C. K. (2016). Samarium promoted Ni/Al2O3 catalysts for syngas production from glycerol pyrolysis. Bull. Chem. React. Eng. Catal. 11, 238–244. doi: 10.9767/bcrec.11.2.555.238-244
Shen, Y., Wang, J., Ge, X., and Chen, M. (2016). By-products recycling for syngas cleanup in biomass pyrolysis – An overview. Renew. Sustain. Energy Rev. 59, 1246–1268. doi: 10.1016/j.rser.2016.01.077
Silva, J. M., Ribeiro, L. S., Órfão, J. J. M., Soria, M. A., and Madeira, L. M. (2019). Low temperature glycerol steam reforming over a Rh-based catalyst combined with oxidative regeneration. Int. J. Hydrogen Energy 44, 2461–2473. doi: 10.1016/j.ijhydene.2018.11.234
Soria, M. A., Barros, D., and Madeira, L. M. (2019). Hydrogen production through steam reforming of bio-oils derived from biomass pyrolysis: thermodynamic analysis including in situ CO2 and/or H2 separation. Fuel 244, 184–195. doi: 10.1016/j.fuel.2019.01.156
Sumrunronnasak, S., Tantayanon, S., Kiatgamolchai, S., and Sukonket, T. (2016). Improved hydrogen production from dry reforming reaction using a catalytic packed-bed membrane reactor with Ni-based catalyst and dense PdAgCu alloy membrane. Int. J. Hydrogen Energy. 41, 2621–2630. doi: 10.1016/j.ijhydene.2015.10.129
Susmozas, A., Iribarren, D., Zapp, P., Linβen, J., and Dufour, J. (2016). Life-cycle performance of hydrogen production via indirect biomass gasification with CO2 capture. Int. J. Hydrogen Energy 41, 19484–19491. doi: 10.1016/j.ijhydene.2016.02.053
Tyagi, V. K., Angériz Campoy, R., Álvarez-Gallego, C. J., and Romero García, L. I. (2014). Enhancement in hydrogen production by thermophilic anaerobic co-digestion of organic fraction of municipal solid waste and sewage sludge–optimization of treatment conditions. Bioresour. Technol. 164, 408–15. doi: 10.1016/j.biortech.2014.05.013
Valderrama Rios, M. L., González, A. M., Lora, E. E. S., and Almazán del Olmo, O. A. (2018). Reduction of tar generated during biomass gasification: a review. Biomass Bioenergy 108, 345–370. doi: 10.1016/j.biombioe.2017.12.002
Valle, B., Aramburu, B., Benito, P. L., Bilbao, J., and Gayubo, A. G. (2018). Biomass to hydrogen-rich gas via steam reforming of raw bio-oil over Ni/La2O3-Al2O3 catalyst: effect of space-time and steam-to-carbon ratio. Fuel 216, 445–455. doi: 10.1016/j.fuel.2017.11.151
Wang, R., Cui, C., Jin, Y., Liu, B., Xing, D., Xie, G., et al. (2014). Photo-fermentative hydrogen production from mixed substrate by mixed bacteria. Int. J. Hydrogen Energy 39, 13396–13400. doi: 10.1016/j.ijhydene.2014.04.097
Yamakawa, C. K., Qin, F., and Mussatto, S. I. (2018). Advances and opportunities in biomass conversion technologies and biore fi neries for the development of a bio-based economy. Biomass Bioenergy 119, 54–60. doi: 10.1016/j.biombioe.2018.09.007
Zribi, M., Lajili, M., and Escudero-Sanz, F. J. (2019). Hydrogen enriched syngas production via gasification of biofuels pellets/powders blended from olive mill solid wastes and pine sawdust under different water steam/nitrogen atmospheres. Int. J. Hydrogen Energy 44, 11280–11288. doi: 10.1016/j.ijhydene.2018.10.021
Keywords: biomass, biochemical conversion, life cycle assessment, hydrogen-rich syngas, thermo-catalytic conversion
Citation: Ayodele BV, Mustapa SI, Tuan Abdullah TARB and Salleh SF (2019) A Mini-Review on Hydrogen-Rich Syngas Production by Thermo-Catalytic and Bioconversion of Biomass and Its Environmental Implications. Front. Energy Res. 7:118. doi: 10.3389/fenrg.2019.00118
Received: 26 June 2019; Accepted: 07 October 2019;
Published: 25 October 2019.
Edited by:
Mohammad Rehan, King Abdulaziz University, Saudi ArabiaReviewed by:
Abdul-Sattar Nizami, King Abdulaziz University, Saudi ArabiaEldon R. Rene, IHE Delft Institute for Water Education, Netherlands
Copyright © 2019 Ayodele, Mustapa, Tuan Abdullah and Salleh. This is an open-access article distributed under the terms of the Creative Commons Attribution License (CC BY). The use, distribution or reproduction in other forums is permitted, provided the original author(s) and the copyright owner(s) are credited and that the original publication in this journal is cited, in accordance with accepted academic practice. No use, distribution or reproduction is permitted which does not comply with these terms.
*Correspondence: Bamidele Victor Ayodele, ayodelebv@gmail.com