Long Term Feasibility Study of In-field Floating Microbial Fuel Cells for Monitoring Anoxic Wastewater and Energy Harvesting
- 1Ricerca sul Sistema Energetico - RSE S.p.A., Milan, Italy
- 2Bristol Robotics Laboratory, Bristol BioEnergy Centre, University West England, Bristol, United Kingdom
- 3MilanoDepur SpA, WWTP of Milano-Nosedo, Milan, Italy
- 4CISE2007, Milan, Italy
In the present work different prototypes of floating MFCs have been tested in anoxic water environments of wastewater plants in Italy, over a period of 3 years. Several configurations of horizontal (flat) and vertical (tubular) MFCs were assembled, using low-cost and light-weight materials, such as plastic lunch boxes, polystyrene or wood to keep the systems afloat, and ceramics for the MFCs. Untreated carbon cloth or veil was used for both anode and cathode electrodes. Felt (flat MFCs) or clay (tubular MFCs) was used as the cation-exchange separator. Single flat MFCs generated power up to 12 mW/m2 while a 32 cylindrical MFC stack generated up to 18 mW/m2. The testing lasted for more than 2 years and there was no inoculation other than exposing the MFCs to the denitrification environment. The cathodes of the flat MFCs were spontaneously colonized by algae and plants, and this did not affect the stability of the systems. Natural light increased the power output of the flat MFCs which were smaller than 50 × 50 cm. Diurnal oscillation of temperature and periodic water flow did not significantly affect the performance of the MFCs. The largest flat MFC produced the highest absolute power, although in a disrupted way. A new, simple low-energy remote monitoring system, based on LoRa technology was used for data transmission over distances of >500 m. This is a piece of hardware that could potentially be suitable for remote monitoring as part of a network, as it can be directly powered by the deployed MFCs.
Highlights
- Prototypes of floating MFCs producing power for energy harvesting in anoxic wastewater.
- 0.4–3 mW (0.2–0.6 V) was generated by the MFCs connected to a DC/DC converter.
- More than 2 years continuous operation of tubular MFCs set in floaters.
- Large flat MFCs more productive than a set of smaller flat MFC of equivalent electrodes surface.
- Light cycle strongly influences the productivity of small flat MFCs.
- Floating MFCs as energy-autonomous sensors of biodegradation for remote monitoring systems.
Introduction
The biotechnological purification of wastewater is based on complex and strictly interconnected physico-chemical and biological processes. The efficiency of the process is achieved only through careful and correct control of crucial parameters, such as nutrient content, organic loading and aerobic/anaerobic conditions. Therefore, regular measurement of physicochemical parameters such as temperature, water flow, dissolved oxygen, organic load is regularly carried out in wastewater treatment plants (WWTPs). The concentration of biodegradable organic matter is the most important parameter of the process, which would require closer monitoring. The frequency of analysis is governed by the instability of the system operation, which is normally more pronounced in smaller treatment plants where the demand for a greater number of controls is often challenged by the availability of resources (human and technical ones). On-line monitoring devices are often used in modern WWTPs, depending on their dimensions, practice and available resources (Bourgeois et al., 2001), whereas the strict control of the water treatment in emerging economies is often unsustainable, if at all supported. Nevertheless, sensing is becoming increasingly important as a function performed to protect and preserve natural ecosystems, whether in developed or emerging economies.
There are currently numerous sensing technologies that are being used for monitoring different environmental parameters, many of which can operate for prolonged periods. The cost however, could be disproportionately high for those WWTPs also requiring strict maintenance regimes, high energy and plenty of consumables. In this context, Microbial Fuel Cells (MFCs) are a promising technology with the dual purpose of harvesting energy and sensing aquatic environments, especially but not exclusively wastewater. Several sediment MFCs have been previously investigated (Reimers et al., 2001) and an MFC-powered rowing float (called Row-bot) has also been recently reported for wastewater environments (Philamore et al., 2015), which is based on the EcoBot principle of operation (Ieropoulos and Melhuish, 2005; Ieropoulos et al., 2010). Several other laboratory experiments confirmed the possibility of using MFCs as sensors for biodegradation in the water (Kim et al., 2003; Tront et al., 2008). The challenge, however, remains as one of scale up for self-powering MFC sensors for water quality and establishing real environmental (even remote) monitoring networks, where electricity may not be available. More recently floating systems (Martinucci et al., 2015; Schievano et al., 2017) have also been proposed, with promising results. The low concentration of biodegradable organic matter (10's of mgL−1 COD) dissolved in the water of the anoxic tank strongly limits the power production of this kind of MFC (Martinucci et al., 2015). The scaling up of such simple systems, based on microbial catalysis at anode and cathode, would be needed in order to be performing well-enough for the desired objectives (power and sensing), optimizing the cell geometry and connecting in series parallel several MFC units (Ieropoulos et al., 2008).
In the present study, the performance of floating MFCs suitably designed for anaerobic water environments was tested both for energy harvesting and sensing purposes, in long-term experiments (more than 3 years) in denitrification tanks of WWTPs, as part of a longer term study (Martinucci et al., 2015). A particular focus was the selection of low cost and readily available materials, parts, electronic components and open-source software, for the purpose of wastewater monitoring and for a possible development of monitoring networks, acknowledging the sense of emerging “citizen science” of the industrialized world (Wildschut, 2017). Different designs and geometries of flat and tubular cells were investigated, with electrodes assembled in horizontal and vertical configurations, using plastic lunch boxes or polystyrene to keep the system afloat in contact with the anoxic wastewater. The MFC configuration was based on carbon electrodes avoiding any chemical catalysts or pretreatment, and a simple inert separator (polypropylene or ceramic) between anode and cathode (Figure 1). Experiments were carried out in two Italian WWTP, at the Milano-Nosedo WWTP (2014–2018) and subsequently at the Carimate WWTP (2017–2019). Milano-Nosedo is the largest WWTP serving a population of 1.25 million in the city of Milan, whereas Carimate is one order of magnitude smaller, but with similar aerobic and anoxic treatment processes. Previous work performed with the same flat MFC configuration at the same Milano-Nosedo WWTP demonstrated the possibility to produce energy from such MFC configurations (Martinucci et al., 2015) and higher power densities were achieved reducing the electrode area from 0.125 m2 (40 × 30 cm) to 0.03 m2 (20 × 15 cm). The present study was conducted with the aim of in situ assessing such MFC systems in producing power and sensing, whilst exposed to the elements (diurnal cycle, weather conditions) and water parameters (temperature, flow, organic content, etc.). A new generation of low-energy remote data logging system (LoRa) was also developed and integrated into the electronic circuitry, to utilize the generated power from MFCs and transmit signals over long distances; the near-term vision is that such systems become an integral part of a full MFC sensing package for remote area access and telemetry.
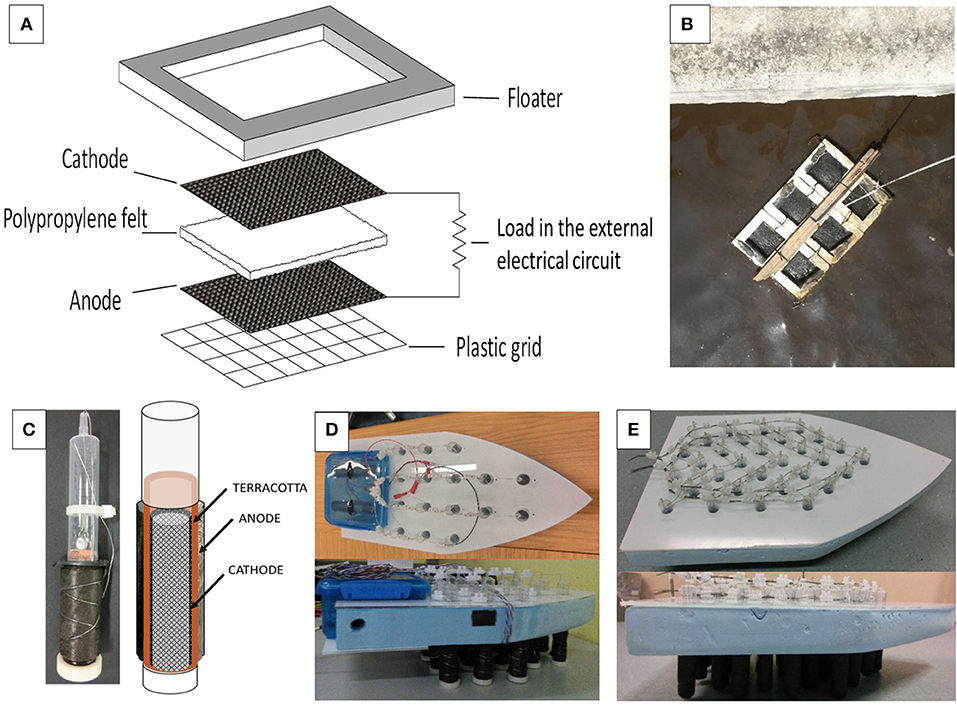
Figure 1. Scheme of a flat MFC (A) and set-up of six MFC elements in a frame ready for the immersion in wastewater (B). Single tubular MFC element and its scheme (C). Tubular MFC elements integrated into a styrene boat: small boat with 16 elements (D); large boat with 32 elements (E).
Materials and Methods
Experiments
Several flat MFCs units of different dimensions were operated in a denitrification anoxic pool at the Milano-Nosedo WWTP during a trial period of more than 3 years (2014–2017). Some flat MFCs units were operated as individual units and the output was monitored through a resistance of 100 Ω while other units, usually the better performing, were occasionally disconnected from the resistance and connected in parallel with other units, to harvest the generated energy (data not shown). A schematic representation alongside photos to illustrate the system are shown in Figures 1A,B. Another long term testing of six flat MFCs with small electrodes and anodes short-circuited all together was operated in the anoxic basin of a different WWTP located at Carimate (Milan, Italy) since June 2017, for about 1 year. The generated electricity from these MFCs was also monitored through a connected 100 Ω electrical resistance.
Several tubular MFCs inserted in a polystyrene frame shaped like a boat were also tested close to the flat ones in the same denitrification anoxic pool at Milano-Nosedo WTTP (Figures 1C–E). The tubular ceramic MFC units shown in the picture and diagram of Figure 1C were connected in two different floating stacks, one with 16 MFC units (Small boat, Figure 1D) and the other with 32 MFC units (Big boat, Figure 1E). All the MFCs units of each boat were electrically connected in parallel using stainless steel wire and terminal blocks and an external resistance of 100 Ω was connected between them. The small boat (16 tubular MFCs) was introduced into the Milano-Nosedo WTTP since 2014, close to the flat MFCs of various geometries and was removed in 2018, after more than 3 years of continuous operation. The big boat (32 tubular MFCs) started to operate at the beginning of March in 2016 and was removed with all the other MFCs in 2018. The different geometries of flat MFCs were operated in the same period.
The trends of power generated in long time experiments by the different flat and tubular MFCs, summarized in Table 1, are discussed in this work. The flat MFC geometries tested at Milano-Nosedo WWTP are named: Flat-large (one, with 50 × 50 cm electrodes), Flat-medium (four, with 18 × 18 cm electrodes) and Flat-small (six, with 10 × 13 cm electrodes). Six more Flat-small MFCs with anodes electrically connected together were the ones tested at the Carimate WWTP.
A detailed description of the Milano-Nosedo WWTP, one of the largest plants in Europe, for 1,250,000 Population equivalent (PE) and water parameters, has been reported previously (Martinucci et al., 2015). In brief, the anoxic water is characterized by an incoming BOD5 of around 170 mgL−1 reduced through biodegradation for 99% (<5 mgL−1 outlet); an incoming COD of about 300 ± 100 mgL−1 and COD <15 mgL−1 in the outlet. The residual COD concentration in the anoxic tank is around 20 mgL−1 measured on a filtrated (0.45 μ) sample, excluding the activated sludge.
The Carimate WWTP is a smaller waterworks facility and serves about 70,221 inhabitants in an area close to Milan, producing about 5,000,000 m3/y of wastewater. Part of the treatment is for industrial wastewater, which is about 1,000,000 m3/y and which corresponds to 4,000–5,000 more population equivalent, (as BOD5). The dissolved COD in the anoxic wastewater of this plant is a little higher than in the Milano-Nosedo plant, usually ranging between 10 and 80 mgL−1.
Flat MFCs Fabrication
Schematic diagrams for the flat MFC are presented in Figure 1A. The two electrodes of each MFC had the same area and were placed parallel to each other at a distance of 1 cm, separated by a polypropylene (PPE) felt. The cathode and anode were both made of carbon cloth, which was free of any chemical catalyst (cod. SCCT-8, SAATI, Legnano, Italy). An electrical resistance of 100 Ω was connected in the external circuit of each MFC, between anode(s) and cathode.
Tubular Ceramic MFCs Building
Tubular MFCs were assembled using terracotta cylinders (L = 7 cm, d = 1.5 cm, thickness 0.2 cm) that were sealed at one end with a plastic stopper. The anodes were made from carbon fiber veil (280 cm2 per MFC) that was folded, wrapped around the cylinder and secured with stainless steel wire (Figure 1C). The cathodes made of activated carbon as previously described (Gajda et al., 2015) were inserted into the cylinders and connected with stainless steel crocodile clips to the wire. A 10 mL plastic syringe was attached to the top of the ceramic cylinder in order to fit the MFCs into the floating “boat”-like polystyrene material. In this way, the activated carbon is exposed to air through the open top connected to the syringe in the floating part of the boat.
Data Acquisition and Transmission
For each tested flat MFC, the voltage V through the R (100 Ω) resistance was recorded every 10 min, using a multichannel data logger made with Arduino hardware and open-source software, as described in Supporting Information (Figure S1). The generated power P (V × I), where I is the current flowing through the external resistance R, was calculated using Ohm's low (V = I × R). The data acquired were then transmitted using a LoRa low energy transmission system described in Figures S1, S2, which was set up and tested for data transmission over distances of >500 m.
Results and Discussion
Both the flat MFCs and the tubular MFCs types were immersed in the denitrification tank without prior inoculation. The electrode facing the anoxic water quickly developed a biofilm fueled by the residual organics dissolved into the water in contact with the electrode. In the case of the flat MFCs whose electrodes were separated though a porous PPE felt, without any electrolytic membrane, biofilm grew also on the cathode sustaining the catalysis of the cathodic reaction (biocathode). Previous works on laboratory-based systems reported that the air-breathing biocathode of single chamber and membraneless MFCs using the same anoxic wastewater from the Milano-Nosedo plant quickly become anaerobic developing a biofilm rich of bacteria of the sulfur and nitrogen cycles (Guerrini et al., 2014; Rago et al., 2017). The same microorganisms differently enrich the anode and the cathode, but alone or in synergy with other microorganisms, such as Spirochetes and photosynthetic anaerobic bacteria are able to sustain a good cathodic catalytic activity using atmospheric oxygen just as the end-terminal electron acceptor (Cristiani et al., 2013). This mechanism allows the flat floating MFCs to perform in the anoxic tank. The development of the biofilm at the cathode was inhibited in the case of tubular ceramic MFCs, as the ceramic porosity does not allow the passage of bacteria (Rago et al., 2018), but also due to the fact that the internal cavities of the ceramic cylinders were not directly exposed to the anoxic water. The cathodic operation, in this case, was facilitated by the activated carbon, whose cathodic catalytic properties, which are higher than the plain carbon cloth, have been well-documented (Dong et al., 2012; Wei et al., 2012; Gajda et al., 2014; Santoro et al., 2017).
MFC Power Performance
The power output from the flat and tubular MFC systems (boats) floating in anoxic wastewater, over a long period of time, is shown in Figures 2–7. The lack of data in Figure 2 (May 2016) is due to technical issues with the data acquisition system and is not an indication of the MFCs ceasing to work. The data shown represent significant parts of the whole 3-year operational period. After approximately 2 weeks from the initial immersion, all the MFCs reached a maximum voltage, varying between 0.2 and 0.5 V depending on the type. In the case of the small boat, a peak voltage of 0.7 V (about 2 mW of power) was produced within the first month of operation (Figure S1). Output levels suddenly dropped, before stabilizing, possibly due to humidity, which would have caused short-circuiting and the uptake of atmospheric oxygen. The small boat had been generating power in the range of 0.04–0.31 mW, showing power density up to 9.6 mW/m2 especially during the first period of the operation, although not continuously. Performance, however, dropped continuously in the second year, when the Big boat was put side by side (Figure 2), which outperformed the smaller boat. The big boat MFCs swiftly reached a power output of 1.15 mW in November 2015 and reached about 0.9 mW (Figure 2), corresponding to a power density of 14 mW/m2 and continued to generate power with a similar trend during the whole experimental period. The better performance of the big boat, compared to the smaller one, is not simply due to the higher number of MFCs connected in series/parallel (32 instead of 16) alone, but also due to the fact that the MFCs in the small boat had probably undergone cathode clogging due to moisture penetration and sludge accumulation resulting from water splashes, as it was operating for a longer period of time. Hence, the decrease in the oxygen supply to the cathode had probably caused a decrease of MFC performance.
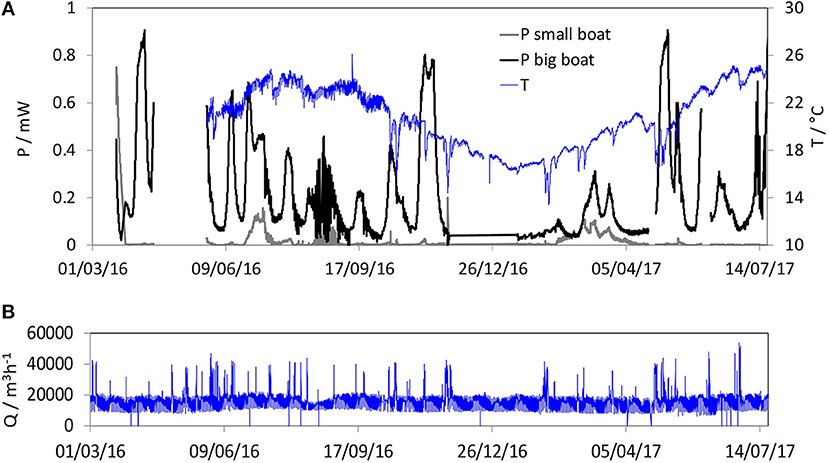
Figure 2. Power trends of the Small boat and Large boat MFCs during more than 1 year (April 2016–July 2017) in an anoxic tank (Milano-Nosedo WWTP) (A); trends of temperature and wastewater flow incoming in the plant is also reported (B).
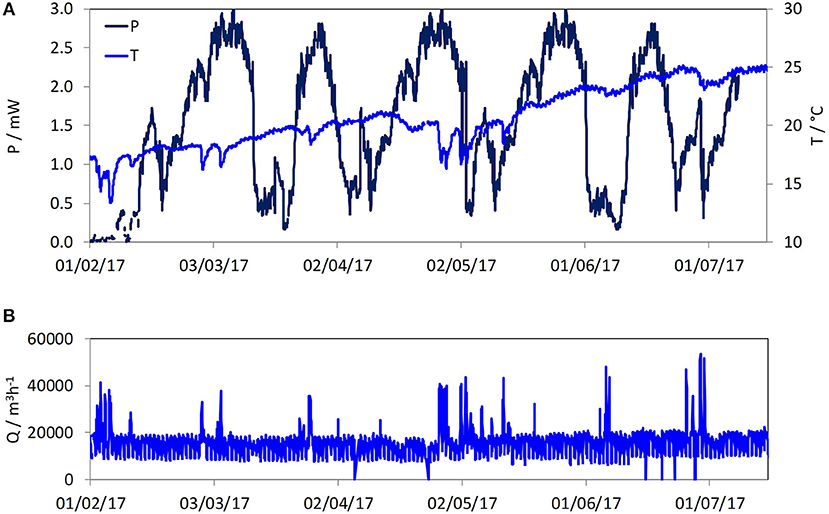
Figure 3. Power trend of the Flat-large MFC (50 × 50 cm) operated from January to July 2017 (Milano-Nosedo WWTP) (A); trends of temperature and incoming wastewater flow in the plant (B).
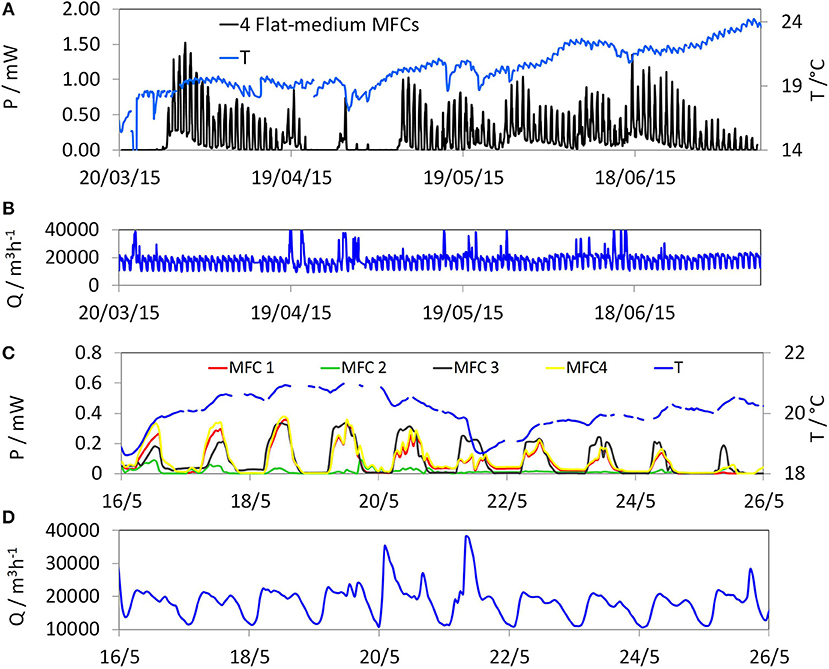
Figure 4. Power trends of the Flat-medium MFCs (18 × 18 cm) operated from April to July 2017 (Milano-Nosedo WWTP) (A); trends of temperature and wastewater flow incoming in the plant (B); zoomed view of the power trends at daily scale (C); zoomed view of temperature and wastewater flow at daily scale (D).
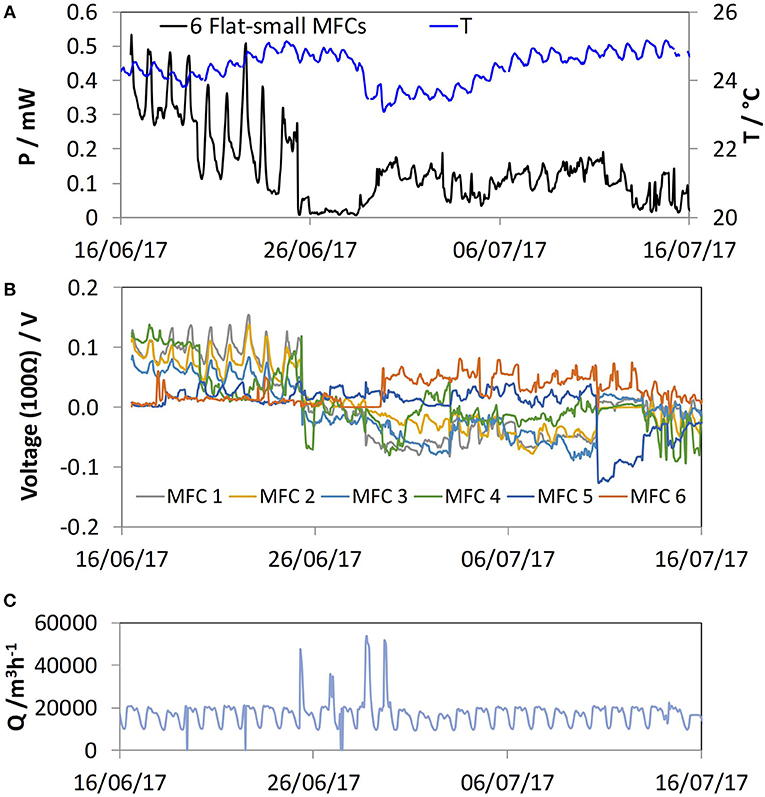
Figure 5. Total power (A) and individual voltage trends (B) produced by six Flat-small MFCs (10 × 13 cm) during 1 month operation (June–July 2017) at Milano-Nosedo WWTP. Wastewater temperature (A) and flow (C).
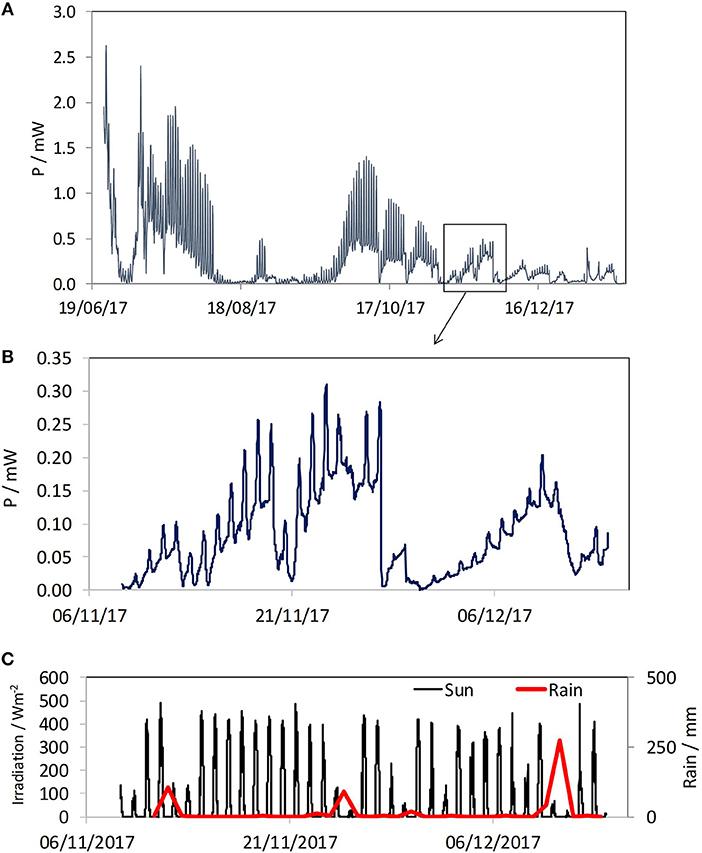
Figure 6. Power trend of six Flat-small MFCs (10 × 13 cm) with shortened anodes (Carimate WWTP) (A). A detail of power trend between November and December 2017 (B); with meteorological measurements: sun irradiation and rain (C).
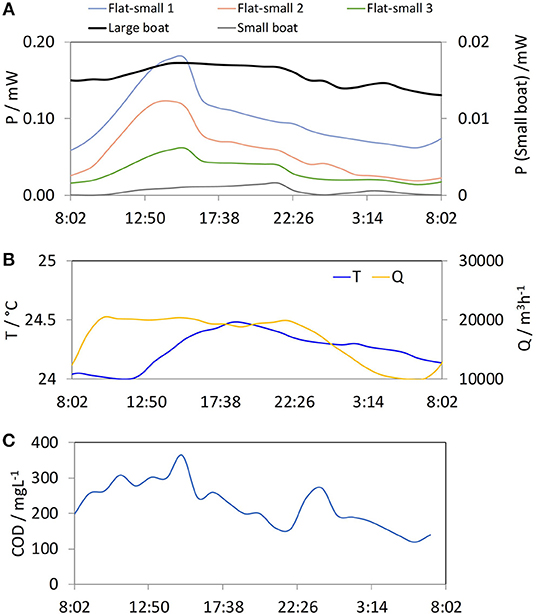
Figure 7. One day (20–21 June 2017) power trends of three Flat-small MFCs and the two boats (A). Temperature, flow (B), and COD (C) concentration in the wastewater sampled at the inlet of the Milano-Nosedo WWTP is also reported.
The flat MFCs required slightly longer periods to start power generation, but all reached a peak in <1 month from the immersion. The Flat-large MFC produced higher absolute power, with peaks of 3 mW (Figure 3) corresponding to a density of 12 mW/m2, which were more evident over weekly rather than daily periods. Nevertheless, a minimum of ~0.4 mW was consistently generated. Oscillation trends were already shown in previous experiments (Martinucci et al., 2015), and it was particularly highlighted in smaller flat MFCs (Figures 4–7).
Figure 4 shows the trend of power produced by four identical flat-medium MFCs during about 4 months of continuous operation. The maximum peak power (1.5 mW) was almost half compared to Flat-large MFC with a similar power density (12 mWm2), since the total electrode area (1,296 cm2) was approximately half. On average, the performance was generally inferior. The inferior performance, more marked in the case of the Flat-small MFCs, can be attributed to high variability of the power produced by the single MFCs (Figure S2). Indeed, the performance between the four MFCs was inconsistent with some producing less power than others, in this way negatively affecting the power density. The power trend produced from six of this MFC type is shown in Figure 5. The power produced peaked at 0.5 mW, corresponding to a power density of 6.4 mWm2, which was inferior to the larger ones.
Figure 6 shows three (out of six) of the better performing Flat-small MFCs, which produced 13.6, 9.4, and 4.6 mWm2, respectively. These results point to a severe concern regarding the achievement of a stable power from the smaller MFCs for energy harvesting. In fact, the problem of spatial distribution and stability affected these flat MFC more than the others, especially in cases of storms and pronounced changes in water flow. The increased rate between perimeter and area characterizing the electrodes of the smallest flat MFCs resulted in the anaerobic conditions becoming rate-limiting for the anode, which operated next to the water surface. Consequently, the possibility of polarity reversal of the cells has to be taken into account for these MFCs, causing the decay of produced power. A phenomenon such as this is reported in Figure 6, documented in the power trends of the six Flat small MFCs operating in the Summer of 2017.
MFC Output vs. Temperature and Water Flow
The generated power by the tubular MFCs on the boat and the relationship with temperature and water flow (Q) measured at the plant inlet during the same period of time is reported in Figure 2. The variation of power appears to be affected by the large variation of flow rate that was consistent with the adverse weather events (mainly rainfall and storms) which diluted the incoming wastewater whilst increasing the flow causing temporary decreases. No direct correlation of power with the water temperature is noticeable, neither for the diurnal cycle nor for the whole experimental period. Nevertheless, the MFC performance decreased to very low levels during wintertime, when the environment temperature generally dropped below 15°C impacting the temperature of the first layer of water. This result is consistent with mesophilic bacterial metabolism, which has an optimum between 20 and 35°C, in the context of the Arrhenius law of temperature. The daily oscillation of power from both Small and Big boats were negligible, like in the case of the flat MFC with much larger cathodes (Figure 3). In fact, cylindrical cathodes of the boats were not exposed to light since they are positioned inside the terracotta cylinder and immersed in water. The observed increases in power can be consequently, correlated with the diurnal cycle and also with the concentration of organic substance through the anodes. Also, the power oscillations of Flat-large MFC are only marginally dependent on the temperature and water flow as for MFC boats (Figure 3). In fact, the decay in performance was mainly coinciding with periods of intense rain and other events that significantly diluted the dissolved organic substance, changing the organic load in the stream.
The daily power oscillations of Flat-medium MFCs are evidenced in Figure 4, which is also highlighted in the zoomed graph of the power trends of the single MFCs during 10 days of June 2015. This graph shows for all the four MFCs a daily variation of power much more pronounced than the weekly or multi-day variation, which results synchronized with the sun hours and the variation of flow rate rather with the temperature variation. The same oscillating behavior was noticed for Flat-small MFCs (Figures 5–7). Daily power trends of Flat-small MFCs (three of six) and of both boats during a single day are shown in Figure 6 with Temperature, Q and COD concentration of the wastewater sampled at the plant inlet (20–21 June 2017). The graphs show that the MFC performance improves concurrently with daytime, the increase of the COD dissolved and the increase in water flow. A noticeable power peak for the Flat-small MFCs is, in fact, in advance of hours with respect to the water temperature variation.
It could be inferred that the cathode electrode was limiting in the case of small size MFCs, not able to sustain the anodic oxidation, especially when anodes are exposed to very low substrate levels (10–20 mg/L COD). The presence of microalgae could favor the local increase in the concentration of oxygen on the cathode resulting in higher power output (Gajda et al., 2015) when exposed to light, and higher power over the long term. Also, a contribution of photosynthetic microorganisms in producing the power could be responsible for MFCs performing well-during the hours of solar radiation. Several microorganisms as well as cyanobacteria could be involved, as reported in the literature (Rosenbaum et al., 2010; Huarachi-Olivera et al., 2018). For instance, purple non-sulfur bacteria were frequently detected in biocathodes of single chamber microbial fuel cells (Cristiani et al., 2013) during previous tests performed at laboratory level using the same wastewater from the nitrification tank of the Milano-Nosedo WWTP (Cristiani et al., 2013; Rago et al., 2017). This aspect deserves further investigation in any future analyses.
It may be finally noted that the black color of the material used for the cathode electrode could increase the temperature oscillation in it, affecting the intensity of the MFC response due to sun exposure. Nevertheless, a test performed with a thermoresistance (PT 100) placed on the electrode surface of a flat MFC exposed to the sun demonstrated that the temperature variation during insulation did not vary more than 2–3°C with respect to the temperature of the water bulk, thereby excluding the immediate effect of temperature.
MFC Output vs. Environmental Factors
Figure 6 shows the trend of the six floating cells immersed in the anoxic basin of the Carimate WWTP, with electrodes measuring 10 × 13 cm and anodes short-circuited. In this way, the different output of the single cell is due to the cathode performance and not to the (common) anode. The figure show daily oscillating power trends as already registered for the smallest flat MFCs operated at the Milano Nosedo WWTP. Therefore, the power limit, in this case as in the case of the other small flat MFCs, is underlined with the underperforming cathodes. The graph shows a strong daily variability, especially during the first months. At the end of November, the cathodes were variously covered by a layer of mud that strongly limited the exchange with the oxygen of the air as well as impeding, to some extent, the arrival of light on the cell. In that period, the power, and also the daily variation, were much more contained, although a direct relationship of power production with sun irradiation and meteorological events was still maintained. The presence of photosynthetic anaerobic bacteria producing hydrogen in such conditions could play a relevant role, enhancing the cathodic reaction in synergy with the rich anaerobic pool (Cristiani et al., 2013; Rago et al., 2015). This phenomenon deserves further investigation to be confirmed. Figure 6 shows also a zoom of the voltage trend of the six flat cells immersed in the anoxic tank of the Carimate WWTP with the solar irradiation measured with a commercial solarimeter (Futura electronics, code 8220-DVM1307). Rain precipitation, expressed as mm of water, is also reported in the graph. The rain causes the dilution of the wastewater, and consequently a reduction of the organic content. This phenomenon can be easily correlated with the power drop.
MFC Output vs. COD Concentration
The trend of COD concentration measured by colorimetric method (Hatch Lange kits and instrumentation) every hour at the inlet of the (Milano–Nosedo) WWTP during 1 day (20–21 June 2017) with power trends of the Flat-small MFCs and of both the boat MFCs immersed in the same anoxic tank is reported in the Figure 7. The graph shows a significant difference in the trend of the different MFC types.
The correlation between the increase in the signal and the period of light is evident for the Flat-small MFCs, even if, in this period, it is not the sole phenomenon that determines the intensity of the power.
The COD fluctuation in the denitrification tank also affected the MFC power production of each type and size. The larger boat output could be better correlated to the COD level than the others exposed to the light and also the small boat, as it was not performing well-during that period. The dynamic behavior of nutrient concentration could then be monitored via an on-line signal, and the treatment could be more efficiently controlled, and therefore cost-effective in the long term (Tanwar et al., 2008).
Energy Harvesting and Data Transmission
The simple low-energy remote system (LoRa) that was set for the MFC data transmission is described in Supplementary Information (Figures S3, S4). It was successfully tested over a distance of 500 m, between the transmitter and the receiver installed at the WTTPs. This system requires a minimum tension of 3.3 V and 0.3 mA (about 1 mW) to operate in sleeping mode and consumes about 130 mA (during the data transmission, that lasts only about 70 μs).
Based on the results achieved, it could be concluded that a single Flat-large MFC which produced continuously an average between 0.4 and 3 mW (0.2–0.6 V) connected to a DC/DC converter in a harvesting circuit, like those described in previous works (Schievano et al., 2017), is able to supply enough power for the transmitter. For a two time a day message, the additional power of 858 mW for 140 μs is needed, which is, in terms of energy (0.12 mWs), negligible if compared to the sleeping mode consume.
In the case of Big boat, the minimum requested power of 0.4 mW is not produced in continuous mode. Therefore, the number of MFCs for the future construction of the boat should be increased.
In both cases, due to the variability of the produced power in time, the connection of two or more MFC systems should be recommended to reliably power the LoRa transmitter.
Post-operation Observation of Flat MFCs
Image of immersed flat and cylindrical reactors in MFC boats during operation are reported in Figures S5, S6. Post-operation observations of flat MFCs are reported in Figure S7.
The flat MFCs were quickly covered by photosynthetic organisms (Figure S7). As time progressed, spontaneous vegetation of grass, herbs and shrubs grew on the cathode of these MFCs (top surface, exposed to air), somewhat demonstrating a wetland phenomenon, whose root system gently covered the electrode without damaging it, allowing the oxygen to reach cathode and preventing its permeation through (Figure S7). This phenomenon probably allowed Flat-large MFC to outperform other set-ups in long time. For the tubular configuration in the boats, there was no vegetation growth on the cathodes. Differently, activate sludge can excessively accumulate on the cathodes, inhibiting the power production. The tubular cathodes, i.e., internal cathodes, suffered for sludge incoming which obstructed aeration, especially the ones in the small boat as they operated for a longer period time (since 2014). This last phenomenon, in particular, resulted critical and has to be prevented for using the small size of both kinds of MFC.
Conclusions
Both the tested floating MFCs types (flat and tubular) were demonstrated to be able to provide electric power for years, although with oscillations, despite the low concentration of organic matter in the wastewater of the denitrification tanks. They clearly demonstrated the viability of the MFC system as an energy harvester in a real environment.
The dissolved COD in the wastewater as well as the meteorological conditions (sun irradiation and rainfall), mainly caused the oscillation in MFC's output. The daily temperature and water flow variations had a negligible effect.
Floating MFCs reached a power peak in about 2 weeks from the installation and do not need maintenance during the whole experimental period. Photosynthetic species (bacteria, microalgae, and plants) growing on the floating MFCs have a positive effect, both as visual impact and in avoiding mud encrustation on the cathodes. These aspects allowed a long-time operation of the MFCs. On the contrary, sludge accumulation on the cathodes of both tubular and flat MFCs resulted in a much-reduced output and most critically required prevention in future designs of MFC's as sensors and energy harvesters. A new, simple low-energy remote transmission system (LORA) successfully tested, shows great promise for a cost-effective way of data transmission that could be implemented in monitoring and controlling water quality based on floating MFCs.
A single unit of Flat-large MFCs and boats can guarantee energy enough for daily remote transmission of signals from the tested system, although not continuously.
The simplicity in setting up such a monitoring system lends itself well-toward the development of citizen science and for remote environmental control.
Data Availability Statement
All datasets generated for this study are included in the article/Supplementary Material.
Author Contributions
PC: experiment design, analyses of data, writing parts, and revising the manuscript. IG: MFC prototypes building, data acquisition and elaboration, writing part of the article, and final approval of the version to be submitted. II: design of the test, interpretation of data, revising of the manuscript, and final approval of the version to be submitted. FP: supervision of the experiment in plant, revising the manuscript, and final approval of the version to be submitted. JG: contribution on biological aspects revising of the manuscript, and final approval of the version to be submitted. PB: set-up of data acquisition and transmission device, writing a part of the manuscript, and final approval of the version to be submitted.
Funding
This work has been financed by the Research Fund for the Italian Electrical System in compliance with the Decree of Minister of Economic Development April 16, 2018. The work done for the boat MFC made in the Bristol BioEnergy Centre, has been funded by the Bill & Melinda Gates Foundation (Grant No. OPP1094890) and the EPSRC, UK (Grant No. EP/L002132/1).
Conflict of Interest
PC was employed by company Ricerca sul Sistema Energetico – RSE SpA. FP was employed by company MilanoDepur SpA.
The remaining authors declare that the research was conducted in the absence of any commercial or financial relationships that could be construed as a potential conflict of interest.
Supplementary Material
The Supplementary Material for this article can be found online at: https://www.frontiersin.org/articles/10.3389/fenrg.2019.00119/full#supplementary-material
References
Bourgeois, W., Burgess, J.E., and Stuetz, R. M. (2001). On-line monitoring of wastewater quality: a review. J. Chem. Technol. Biotechnol. 76, 337–348. doi: 10.1002/jctb.393
Cristiani, P., Franzetti, A., Gandolfi, I., Guerrini, E., and Bestetti, G. (2013). Bacterial DGGE fingerprints of biofilms on electrodes of membraneless microbial fuel cells. Int. Biodeterior. Biodegradation 84, 211–219. doi: 10.1016/j.ibiod.2012.05.040
Dong, H., Yu, H., and Wang, X. (2012). Catalysis kinetics and porous analysis of rolling activated carbon-PTFE air-cathode in microbial fuel cells. Env. Sci. Technol. 46, 13009–13015. doi: 10.1021/es303619a
Gajda, I., Greenman, J., Melhuish, C., and Ieropoulos, I. (2015). Simultaneous electricity generation and microbially-assisted electrosynthesis in ceramic MFCs. Bioelectrochemistry 104, 58–64. doi: 10.1016/j.bioelechem.2015.03.001
Gajda, I., Greenman, J., Melhuish, C., Santoro, C., Li, B., Cristiani, P., et al. (2014). Water formation at the cathode and sodium recovery using microbial fuel cells (MFCs). Sustain. Energy Technol. Assessments 7, 187–194. doi: 10.1016/j.seta.2014.05.001
Guerrini, E., Grattieri, M., Trasatti, S., Bestetti, M., and Cristiani, P. (2014). Performance explorations of single chamber microbial fuel cells by using various microelectrodes applied to biocathodes. Int. J. Hydrogen Energy 39, 21837–21846. doi: 10.1016/j.ijhydene.2014.06.132
Huarachi-Olivera, R., Dueñas-Gonza, A., Yapo-Pari, U., Vega, P., Romero-Ugarte, M., Tapia, J., et al. (2018). Bioelectrogenesis with microbial fuel cells (MFCs) using the microalga Chlorella vulgaris and bacterial communities. Electron. J. Biotechnol. 31, 34–43. doi: 10.1016/j.ejbt.2017.10.013
Ieropoulos, I., Greenman, J., and Melhuish, C. (2008). Microbial fuel cells based on carbon veil electrodes: stack configuration and scalability. Int. J. Energy Res. 32, 1228–1240. doi: 10.1002/er.1419
Ieropoulos, I., Greenman, J., Melhuish, C., and Horsfield, I. (2010). “EcoBot-III-A robot with guts,” in Proceedings of the Alife XII Conference (Odense), 733–740.
Ieropoulos, I., and Melhuish, C. (2005). EcoBot-II: an artificial agent with a natural metabolism. J. Adv. Robot. Syst. 2, 295–300. doi: 10.5772/5777
Kim, B. H., Chang, I. S., Gil, G. C., Park, H. S., and Kim, H. J. (2003). Novel BOD (biological oxygen demand) sensor using mediator-less microbial fuel cell. Biotechnol. Lett. 25, 541–545.
Martinucci, E., Pizza, F., Perrino, D., Colombo, A., Trasatti, S. P. M., Barnabei, L., et al. (2015). Energy balance and microbial fuel cells experimentation at wastewater treatment plant Milano-Nosedo. Int. J. Hydrogen Energy 40, 14683–14689. doi: 10.1016/j.ijhydene.2015.08.100
Philamore, H., Rossiter, J., Stinchcombe, A., and Ieropoulos, I. (2015). “Row-bot: an energetically autonomous artificial water boatman,” in 2015 IEEE/RSJ International Conference on Intelligent Robots and Systems (IROS) (IEEE), 3888–3893.
Rago, L., Cristiani, P., Villa, F., Zecchin, S., Colombo, A., Cavalca, L., et al. (2017). Influences of dissolved oxygen concentration on biocathodic microbial communities in microbial fuel cells. Bioelectrochemistry 116, 39–51. doi: 10.1016/j.bioelechem.2017.04.001
Rago, L., Ruiz, Y., Baeza, J. A., Guisasola, A., and Cortés, P. (2015). Microbial community analysis in a long-term membrane-less microbial electrolysis cell with hydrogen and methane production. Bioelectrochemistry 106, 359–368. doi: 10.1016/j.bioelechem.2015.06.003
Rago, L., Zecchin, S., Marzorati, S., Goglio, A., Cavalca, L., Cristiani, P., et al. (2018). A study of microbial communities on terracotta separator and on biocathode of air breathing microbial fuel cells. Bioelectrochemistry 120, 18–26. doi: 10.1016/j.bioelechem.2017.11.005
Reimers, C. E., Tender, L. M., Fertig, S., and Wang, W. (2001). Harvesting energy from the marine sediment–water interface. Env. Sci. Technol. 35, 192–195. doi: 10.1021/es001223s
Rosenbaum, M., He, Z., and Angenent, L.T. (2010). Light energy to bioelectricity: photosynthetic microbial fuel cells. Curr. Opin. Biotechnol. 21, 259–264. doi: 10.1016/j.copbio.2010.03.010
Santoro, C., Arbizzani, C., Erable, B., and Ieropoulos, I. (2017). Microbial fuel cells: from fundamentals to applications. A review. J. Power Sources 356, 225–244. doi: 10.1016/j.jpowsour.2017.03.109
Schievano, A., Colombo, A., Grattieri, M., Trasatti, S. P., Liberale, A., Tremolada, P., et al. (2017). Floating microbial fuel cells as energy harvesters for signal transmission from natural water bodies. J. Power Sour. 340, 80–88. doi: 10.1016/j.jpowsour.2016.11.037
Tanwar, P., Nandy, T., Ukey, P., and Manekar, P. (2008). Correlating on-line monitoring parameters, pH, DO and ORP with nutrient removal in an intermittent cyclic process bioreactor system. Bioresour. Technol. 99, 7630–7635. doi: 10.1016/j.biortech.2008.02.004
Tront, J. M., Fortner, J. D., Plötze, M., Hughes, J. B., and Puzrin, A. M. (2008). Microbial fuel cell biosensor for in situ assessment of microbial activity. Biosens. Bioelectron. 24, 586–590. doi: 10.1016/j.bios.2008.06.006
Wei, B., Tokash, J. C., Chen, G., Hickner, M. A., and Logan, B. E. (2012). Development and evaluation of carbon and binder loading in low-cost activated carbon cathodes for air-cathode microbial fuel cells. RSC Adv. 2, 12751–12758. doi: 10.1039/C2RA21572A
Keywords: floating microbial fuel cells, wastewaters monitoring, low-energy remote data transmission, in-field MFC tests, anossic wastewater, long term field operation
Citation: Cristiani P, Gajda I, Greenman J, Pizza F, Bonelli P and Ieropoulos I (2019) Long Term Feasibility Study of In-field Floating Microbial Fuel Cells for Monitoring Anoxic Wastewater and Energy Harvesting. Front. Energy Res. 7:119. doi: 10.3389/fenrg.2019.00119
Received: 15 March 2019; Accepted: 11 October 2019;
Published: 01 November 2019.
Edited by:
Eileen Hao Yu, Newcastle University, United KingdomCopyright © 2019 Cristiani, Gajda, Greenman, Pizza, Bonelli and Ieropoulos. This is an open-access article distributed under the terms of the Creative Commons Attribution License (CC BY). The use, distribution or reproduction in other forums is permitted, provided the original author(s) and the copyright owner(s) are credited and that the original publication in this journal is cited, in accordance with accepted academic practice. No use, distribution or reproduction is permitted which does not comply with these terms.
*Correspondence: Pierangela Cristiani, pierangela.cristiani@rse-web.it