Effects of Revegetation on Soil Physical and Chemical Properties in Solar Photovoltaic Infrastructure
- 1Department of Earth and Environmental Science, Temple University, Philadelphia, PA, United States
- 2Department of Land, Air and Water Resources, University of California, Davis, Davis, CA, United States
- 3National Renewable Energy Laboratory, Center for Strategic Energy Analysis, Golden, CO, United States
- 4Department of Architecture and Environmental Design, Temple University, Ambler, PA, United States
Solar photovoltaic (PV) technology is being deployed at an unprecedented rate. However, utility-scale solar energy development is land intensive and its large-scale installation can have negative impacts on the environment. In particular, solar energy infrastructure can require extensive landscape modification that transforms soil ecological functions, thereby impacting hydrologic, vegetative, and carbon dynamics. However, reintroducing native vegetation to solar PV sites may be a means of restoring their soils. To this end, we investigated critical soil physical and chemical parameters at a revegetated photovoltaic array and an adjacent reference grassland in Colorado, United States. Seven years after revegetation, we found that carbon and nitrogen remained lower in the PV soil than in the reference soil and contained a greater fraction of coarse particles. We also found that the PV modules introduced heterogeneity in the soil moisture distribution, with precipitation accumulating along the lower edges of panels. The redistribution of soil moisture by panel arrays could potentially be used in concert with planting strategies to maximize plant growth or minimize soil erosion, and should be considered when evaluating the potential to co-locate vegetation with solar infrastructure.
Introduction
Solar photovoltaic (PV) technologies have been among the fastest growing energy technologies in recent years due to technological advancements and favorable government policies (U. S. Department of Energy, 2012; Breyer et al., 2017). Further, solar power has the greatest technical potential among renewable energy technologies (Jacobson, 2009; Edenhofer et al., 2011; De Marco et al., 2014; Ellabban et al., 2014) and is expected to fulfill 20–29% (32,700–133,000 GW) of global electrical demand by 2100 (Breyer et al., 2017). Although solar generation of electricity has many advantages, including low emission rates, an ability to reclaim degraded land, and an ability to improve quality of life in off-grid rural areas, deploying large-scale solar PV infrastructure can have negative impacts on ecological functions including carbon sequestration (Ravi et al., 2014; Hernandez et al., 2019). Moreover, solar PV is space-intensive, with the large-scale, non-integrated deployment of solar PV estimated to require more land area than coal, nuclear, or natural gas technologies (Fthenakis and Kim, 2009). Under an optimistic scenario for global solar energy deployment by 2050 (8500 GWp) (IRENA-International Renewable Energy Agency, 2018) and assuming utility-scale PV installations require 3 ha per MWp of capacity-weighted average direct land use (Ong et al., 2013), around 25 million hectares of land will be transformed in the next 30 years if all arrays are ground-mounted. Further, requirements for access roads, transmission lines, etc. can raise the total land area transformed by solar PV installations substantially (Ravi et al., 2014).
Conventional, utility-scale solar energy infrastructure modifies landscapes extensively through the site preparation process: native vegetation is removed, the ground surface is graded, and fill is added and compacted (Hernandez et al., 2014). Such modifications transform soil physical, chemical, and biological properties, thereby impacting moisture and nutrient dynamics, and thus soil’s ability to support vegetation and perform a host of associated ecological processes. Strategies to mitigate the cumulative impacts of solar PV installation and operation on landscape function have therefore been the focus of recent research (Turney and Fthenakis, 2011; Phillips, 2013; Hernandez et al., 2014, 2015; Armstrong et al., 2016; Walston et al., 2016). One promising strategy is to integrate other, vegetated land cover types (e.g., crop cultivation or native grassland) into PV facilities, as these may increase their ability to support ecosystem services like pollination and carbon sequestration (Dupraz et al., 2011; Macknick et al., 2013; Hernandez et al., 2014; Ravi, 2015; Hoffacker et al., 2017; Walston et al., 2018). The concept of co-locating solar PV and agriculture (i.e., “agrivoltaics”) was first suggested by Goetzberger and Zastrow (1982). Since then, a number of modeling (Dupraz et al., 2011; Ravi et al., 2014, 2016) and field studies (Marrou et al., 2013b, c; Beatty et al., 2017; Barron-Gafford et al., 2019) have investigated the technological, economic, and environmental feasibility of co-location approaches.
Assessing the impacts of PV deployment on land surface characteristics can inform guidelines for site preservation and help determine the optimum environmental and financial outcomes co-location strategies could bring about. Ultimately, such information could be used to determine if proposed co-located PV installations are likely to be successful. Prior studies of the environmental impacts of solar infrastructure have been focused on modeling runoff and monitoring microclimatic impacts (Cook, 2011; Marrou et al., 2013a; Barron-Gafford et al., 2016). For example, one review found that site preparation for PV arrays typically remove vegetation and degrade soil, resulting in significant increases in onsite runoff and soil erosion (Cook, 2011). Further, changes in structure of the terrain, vegetation cover, and albedo can raise air temperatures over PV arrays relative to surrounding natural areas (Barron-Gafford et al., 2016). However, other studies have identified effects of PV infrastructure that are likely to be favorable for co-located vegetation; these include increases in soil moisture content, biomass, and plant water use efficiency (Marrou et al., 2013a; Hassanpour Adeh et al., 2018; Barron-Gafford et al., 2019). In addition, lower PV panel temperatures have been observed in arrays with underlying agricultural crops vs. those without (attributed to greater evapotranspiration rates), highlighting the potential of co-location to increase electrical generation in arid regions (Barron-Gafford et al., 2019).
Despite the above-mentioned advances, it is unclear if reintroducing native vegetation at existing solar PV sites can successfully mitigate changes to soil hydrology and ecology. In cases where natural land is leased for solar projects, leases typically span 20–30 years; during this time soil hydrological and ecological processes may be negatively impacted or made spatially heterogeneous. In addition to diminishing the landscape’s ability to support ecosystem services during the solar facility’s lifespan, these changes may leave legacy effects that persist long after the installation is removed. Although many studies have quantified the environmental footprints of photovoltaic facilities (Fthenakis and Kim, 2010, 2011; Sherwani et al., 2010; Turney and Fthenakis, 2011; Fthenakis et al., 2012; Perez and Fthenakis, 2012; Leccisi et al., 2016), there have been few field-based investigations of the impacts of PV arrays and co-location strategies on soil properties (e.g., hydrology, geomorphology, and chemistry) (Armstrong et al., 2014; Hassanpour Adeh et al., 2018). Given this, we investigated whether revegetation of a PV facility is able to engender soil physical and chemical properties similar to those at an undisturbed reference site and, further, if the PV modules introduce spatial variation in soil properties. We did this at a PV installation that had been experimentally revegetated with native grasses. Soil properties at the PV site were compared (a) to those in an adjacent, undisturbed grassland characteristic of the region’s native land cover and (b) spanning the range of location types under and between PV modules.
Materials and Methods
Site Description
This study took place at a 1.1 MW solar PV facility in the National Renewable Energy Laboratory’s National Wind Technology Center. This facility is located on the eastern slope of the Front Range in northern Jefferson County, Colorado, USA and has a mean annual precipitation of 374 mm (Beatty et al., 2017). When the site was constructed in 2009, the topsoil was largely removed and the site was graded, leveled to < 1% slope, and compacted (Beatty et al., 2017). Surficial soils at the site are paleosols derived from unsorted alluvial/colluvial deposits; soil-forming processes have extensively oxidized native iron and leached clay minerals (Beatty et al., 2017). The area encompassing the site was historically a xeric tallgrass prairie dominated by big bluestem grass (Andropogon gerardii). Grazing and other activities have altered the region’s vegetation while leaving the paleosols and clay-enriched subsoils intact (Beatty et al., 2017). Following the construction of the solar PV array, a portion of the disturbed area was revegetated with a variety of native grasses (e.g., Bouteloua gracilis and Poa compressa) to determine, in part, if soil alteration could be mitigated (Beatty et al., 2017). Revegetation occurred in 2010, 7 years prior to the activities described below.
Study Design
A campaign to collect soil samples and take field measurements was carried out in the revegetated portion of the solar PV facility and in a nearby grassland in July 2017. This portion of the PV facility had four rows of PV modules, each 49 m in length (Supplementary Figures S1A,B). PV panels were mounted on single-axis solar-tracking systems 1 m above ground-level (Beatty et al., 2017). Soil measurements and samples were taken along three transects in the revegetated portion of the solar PV site (hereafter, solar transects/soils) and along a fourth transect in an adjacent, undisturbed grassland (hereafter, reference transect/soil) that is representative of the area’s vegetation (Supplementary Figure S1A). Solar transects ran perpendicular to the rows of PV modules, spanning four rows and the adjacent interspaces. The reference transect was 50 m east of the nearest point in the PV array, across an unpaved road (Supplementary Figure S1A). Soil measurements and sampling took place at four locations on solar transects: below the east edge of each panel (EE), beneath the center of each panel (BP), below the west edge of each panel (WE), and in the uncovered interspace adjacent to each panel (IS) (Supplementary Figure S1C). Transects thus consisted of 16 sampling locations, spaced ∼2.5 m apart, and were ∼10 m in length. Sampling/measurement locations on the reference transect were likewise spaced 2.5 m apart, with the transect extending 10 m. In total there were 48 solar PV sampling locations and 16 reference locations.
Field Measurements
Volumetric soil moisture (VSM) content and infiltration rates were measured at each sampling location. VSM was measured using a 15-cm long soil water content reflectometer (HydroSense II, Campbell Scientific, Logan, United States). In addition to the primary set of VSM measurements, a second set of measurements was made the next day, after an overnight rainfall event. This event delivered 6.6 mm of rainfall and the wind direction ranged from southwesterly to northwesterly. Precipitation and wind data were collected from a meteorological station located ∼200 m northwest of the PV array. The solar array was facing west at the end of the field campaign and during the precipitation event that evening.
Infiltration rates were measured along two of the three solar transects, as well as the reference transect, with two measurements made per sampling location. This modification to the sampling design was deemed appropriate given the spatial variability of infiltration in combination with the limited period of access to the site. A mini disk infiltrometer (METER, Pullman, United States) was used for measurements; it had an adjustable suction (1 cm was used) and a small footprint (5-cm diameter disk). The mini disk infiltrometer exerts a negative tension on the soil surface, thereby excluding macropores and only measuring flow in the soil matrix; the resulting data better represent properties of the soil itself. Infiltration data were used to calculate unsaturated hydraulic conductivity (Kunsat) for each sample following Zhang (Zhang, 1997).
Laboratory Measurements
Surficial soil samples (0–5 cm) were taken at all sampling locations along the four transects. After air-drying, soil samples were sieved to 2 mm, passed through a riffle sampler, and sub-sampled. One sub-sample per sample was used to characterize the particle size distribution (PSD) while another was used for chemical analysis. PSDs were measured with a laser diffraction particle size analyzer (LS 13-320 with a tornado dry powder module; Beckman Coulter, Brea, United States); the dynamic grain size ranged from 0.4 to 2000 μm. Total soil carbon (C) and nitrogen (N) were determined by Brookside Labs (New Bremen, United States) using the combustion method (McGeehan and Naylor, 1988; Nelson and Sommers, 1996).
Statistical Analysis
Data were analyzed using mixed- or fixed-effects linear models (Table 1). Separate models were generated for each response variable, which included VSM before and after the precipitation event, Kunsat, total carbon, total nitrogen, mean particle diameter, and the coarse particle fraction (> 2 mm). In all models, the location category (EE, BP, WE, IS, and Reference) was the only fixed explanatory variable. When likelihood ratio tests indicated that it was warranted (Table 1), random effects were included to account for the spatial clustering of samples along transects and/or beneath panels. F-tests, based on Type II sums of squares, were used to determine if response means differed across location categories. When they differed, pairwise comparisons were made using estimated marginal means with Tukey adjustments applied to P-values. All analyses were carried out in R 3.6.1 (R Core Team, Vienna, Austria) with P < 0.05 considered statistically significant.
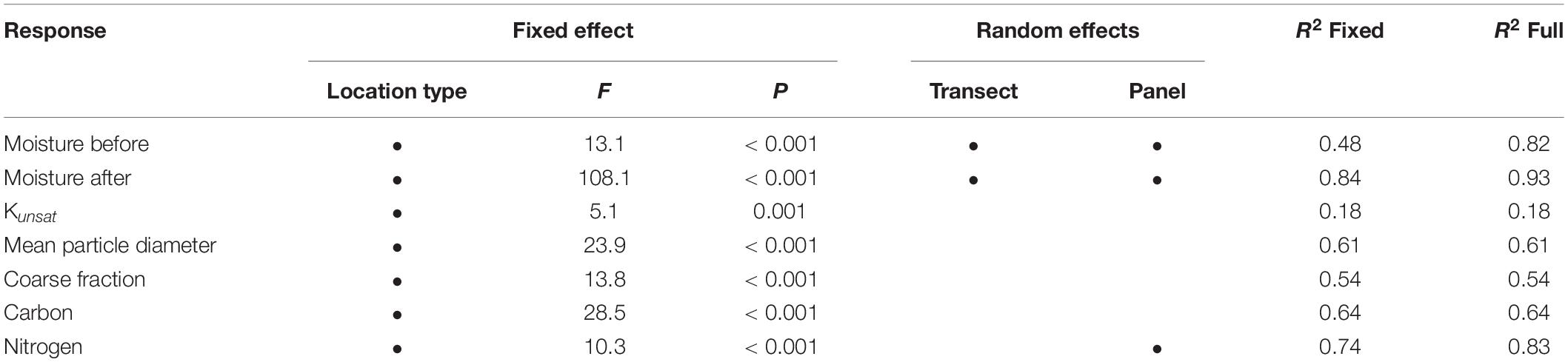
Table 1. Statistical models and results for evaluations of whether soil properties differed by location type.
Results
Soil Moisture
The reference soil had the lowest sample mean during both measurement periods, though population means were not statistically differentiable between the reference and nearly any PV location; the exception was west edges after the rain event. Soil moisture was elevated in west edge locations in PV soils, both before (Figure 1A) and after (Figure 1B) the rain event (Table 1). Moreover, mean moisture levels increased from 14.5 to 25.4% at these locations following the rain event, whereas they increased from 9.4 to 9.5%, on average, in other soils at the PV site.
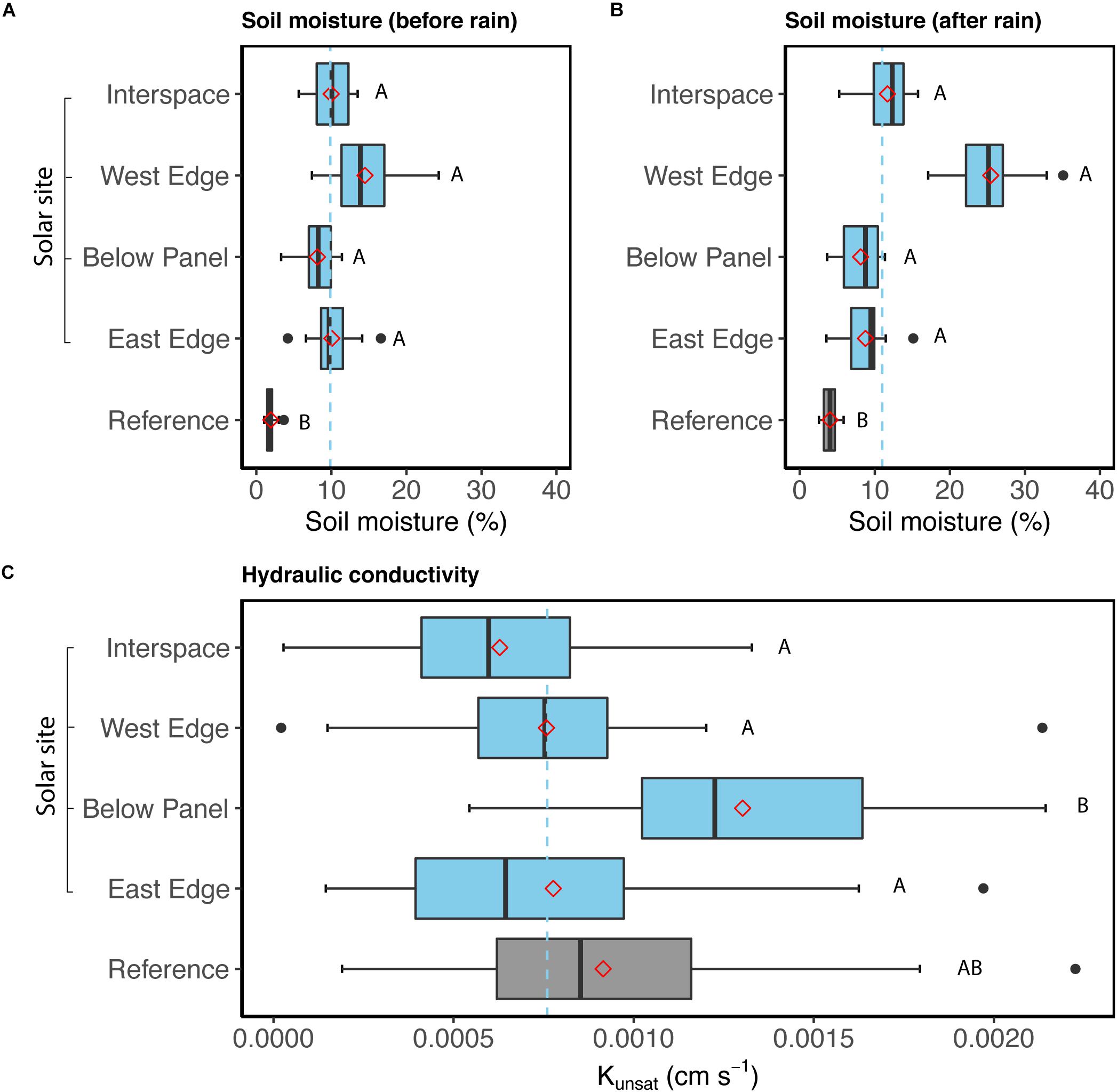
Figure 1. Soil moisture (A) before and (B) after a precipitation event in the reference grassland and in locations beneath solar modules. (C) Unsaturated hydraulic conductivity (Kunsat) in the reference soil and in locations beneath solar modules. Box plots show medians (solid lines) and interquartile ranges (box enclosures), with whiskers extending to the most extreme observation ≤ 1.5 × the interquartile range beyond the box. Also shown are means (red diamonds) and individual observations beyond whiskers (black circles). Location categories share letters if pairwise comparisons did not identify significant differences between means.
Hydraulic Conductivity
Mean Kunsat was highly variable, ranging from 0.2 × 10–4 to 21.4 × 10–4 cm s–1 in the PV array and 1.9 × 10–4–22.3 × 10–4 cm s–1 in the reference. Given the substantial overlap, Kunsat was not significantly different in reference vs. PV soils but was greater beneath panels than at edge locations or in interspaces (Figure 1C and Table 1).
Particle Size
Soil at the PV site had a greater coarse-particle (> 2 mm) mass fraction than did the reference soil (Figure 2A); the fraction was similar across the four PV location categories. Correspondingly, mean particle diameter was greater in soils (< 2 mm) from the PV site than those at the reference site (Figure 2B). Moreover, the reference soil had a more heterogeneous grain size distribution than did soil from the solar site, with the degree of sorting roughly proportional to the mean particle diameter [φ size-scale = -log2 (diameter in mm)] (Figure 2C). No difference in particle size characteristics were observed among locations in the PV site.
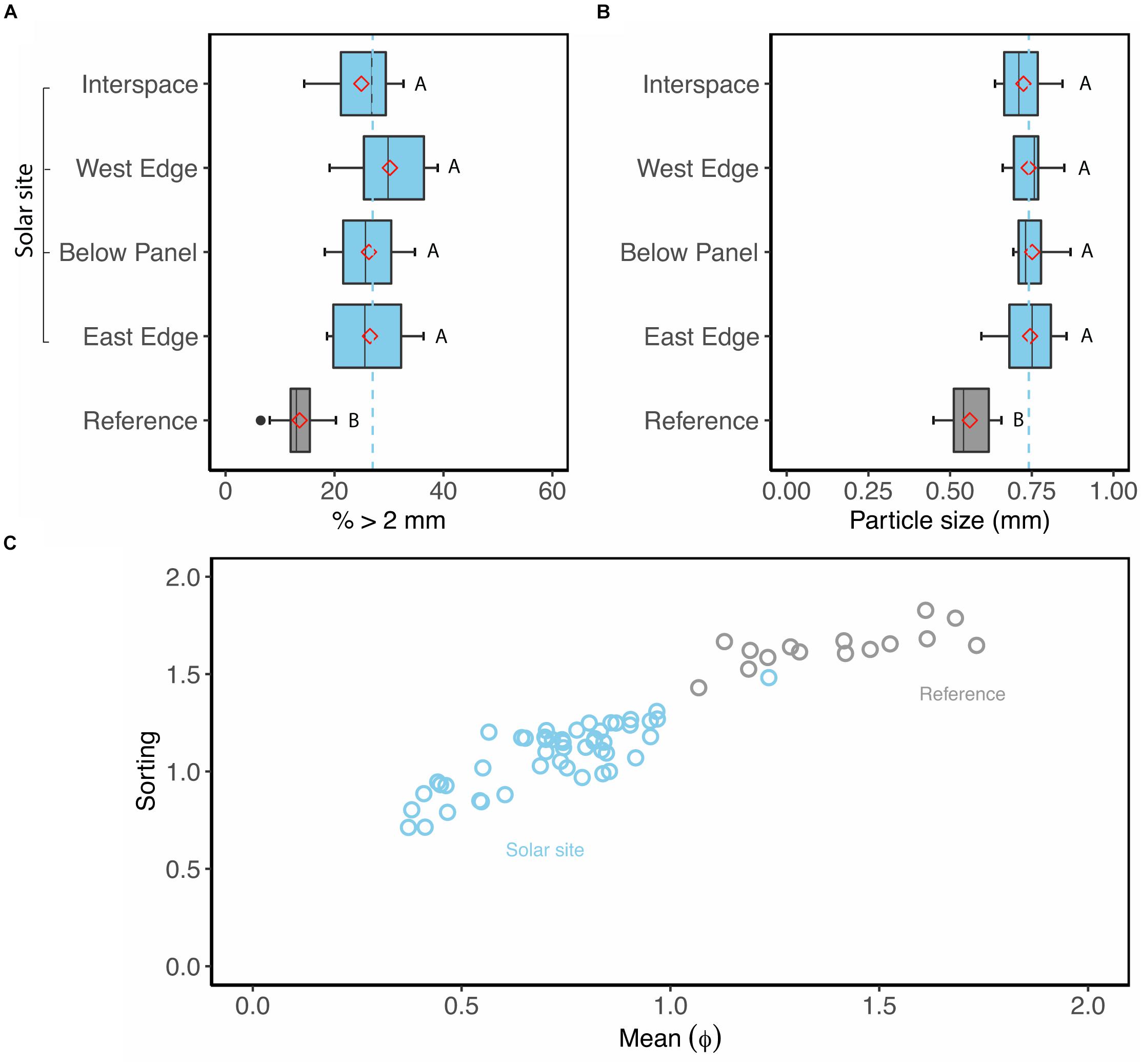
Figure 2. (A) Percentage of grain diameter larger than 2 mm and (B) the mean particle diameter (<2 mm) of the soil samples from control and solar site. (C) Sorting and mean of soil particle size (φ size scale) for control and solar site. See Figure 1 for an explanation of plot structure and abbreviations.
Carbon and Nitrogen
Soils at the solar PV site contained significantly less carbon (38%) and nitrogen (50%) than did the reference soil (Figure 3 and Table 1). However, there was no evidence of differences in mean carbon or nitrogen content among location categories at the PV site.
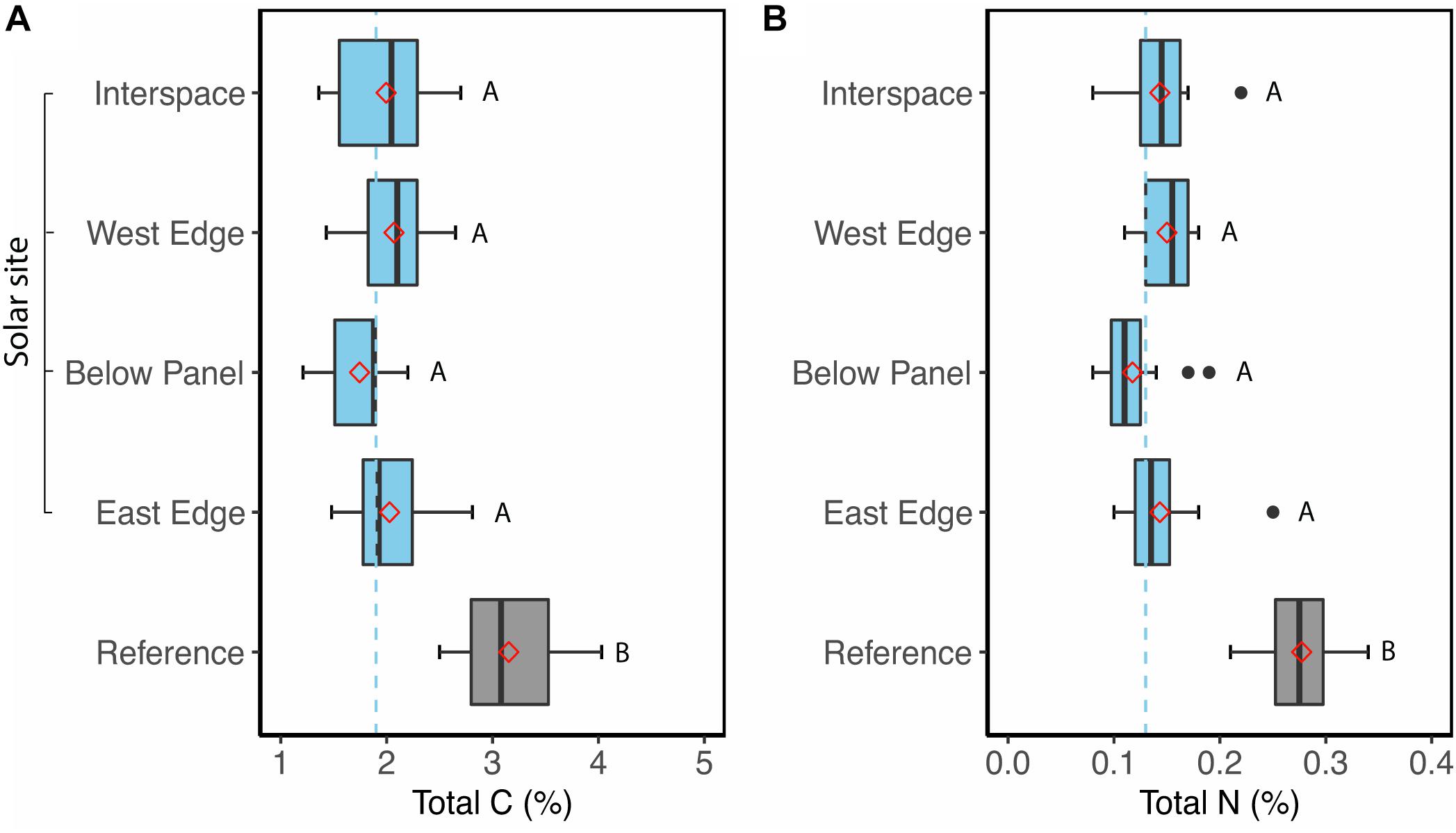
Figure 3. (A) Total carbon and (B) total nitrogen for the reference soil and in locations beneath solar modules. Also shown are means (red diamonds) and individual observations beyond whiskers (black circles). See Figure 1 for an explanation of plot structure and abbreviations.
Discussion
Soil Moisture
Although statistically equivocal, our results suggested that soil moisture may have been greater at the solar PV site compared to the reference soil. If real, this could have been due to shading and wind sheltering by the PV array, as these have been found to decrease actual evapotranspiration by 10–40% (Marrou et al., 2013a). Our results also demonstrate that PV arrays can introduce considerable heterogeneity in the spatial distribution of soil moisture. Specifically, the presence of PV modules can concentrate moisture along their low-lying edges, which were to the west in this study. While this spatial pattern was greatest following a rain event, it was also evident before the event, which was 3 days after the prior (5.6 mm). There may be important implications of soil moisture heterogeneity for plant growth in PV arrays co-located with crop or forage plants; these would be especially magnified in arid regions, where small variations in water availability could have large effects on plant growth (Noy-Meir, 1973; D’Odorico et al., 2007).
Hydraulic Conductivity and Particle Size
Even though soil grain size distribution is one of the determinants of infiltration rates (Mazaheri and Mahmoodabadi, 2012), the difference in grain size distribution did not result in measurable differences in Kunsat between the solar PV site and reference soils (Figure 1C). However, when comparing Kunsat among the location types within the solar site, Kunsat was elevated immediately below panels, even though the particle size distribution in these locations were similar to those at other locations (Arya et al., 1999). This discrepancy between expected and observed hydraulic conductivity below panels may be attributable to reduced exposure to site maintenance activities that cause compaction, as compaction decreases hydraulic conductivity (Vomocil and Flocker, 1961). Differences in compaction may likewise explain the lack of difference in Kunsat despite the difference between particle size distributions between PV and reference soils (Figures 2A,B).
It is likely that the disparity in particle size between reference and PV soils arose during the PV facility’s construction phase. As documented by other studies (Mohammad and Adam, 2010; Hernandez et al., 2014), disturbance to topsoil, the addition of fill, and the removal of native vegetation during construction can accelerate the erosion of fine particles, both by wind and water action. In the long-term absence of vegetation – a major soil erosion control – accelerated erosion results in loss of soil carbon, nutrients, and water holding capacity, leading to an overall decrease in the ability of soil to sustain vegetation (Lal, 2003; Li et al., 2008). Dust particles resulting from wind erosion can be transported long distances (Field et al., 2010; Ravi et al., 2011) or even deposited locally on solar PV panel surfaces, resulting in significant losses in solar power generation and additional operational costs for cleaning panels (Mani and Pillai, 2010; Mejia and Kleissl, 2013).
Carbon and Nitrogen
The significantly lower total carbon and nitrogen levels in the solar PV soil vs. in the reference soil (Figure 3) was likely caused by the removal of topsoil during the array’s construction. This is consistent with prior studies showing reduced soil organic matter and plant production in experiments simulating topsoil erosion by artificially removing it (Larney et al., 2000, 2016; Hölzel and Otte, 2003). Reduced C and N levels suggest that nutrient cycling had not fully reestablished 7 years after PV construction and, further, that the PV soil’s ability to sequester carbon was diminished relative to the native soil. In order to minimize the loss of these key soil functions, appropriate site preservation practices should be adopted to minimize topsoil disturbance during construction. Total N and C content for the reference site were similar to those reported by prior studies in the region (e.g., Golubiewski, 2006).
Implications
The revegetation of our study site was intended, in part, to render the soil at a solar PV facility similar to those of the region’s grasslands. A number of the investigated soil characteristics differed between reference and PV array, indicating that this was not fully achieved in 7 years. Further, the presence of photovoltaic arrays induced spatial heterogeneity in soil moisture and Kunsat, specifically coupling soil moisture to the position and orientation of the PV modules. Despite these persistent changes to soil properties, other studies indicate that co-location can restore certain ecological functions. For example, a previous study at our site indicated that the revegetation was sufficient to suppress erosion and provide wildlife habitat (Beatty et al., 2017).
The environmental heterogeneity introduced by PV modules may have some advantages, even if soil properties differ from those of native grasslands. For instance, it may be possible for co-located PV panels to be strategically oriented in a manner that would direct intercepted rain to or away from the adjacent soil, depending on the irrigation requirements of the plants. This control over rainfall distribution could benefit vegetation types with specific watering requirements, like many agricultural crops. Further, the rapidly-draining soils beneath PV modules could be suitable for cultivating drought-resistant crops (Ravi et al., 2016). Co-locating vegetation would also suppress dust emissions, reducing rates of dust deposition on panels (Ravi et al., 2016; Elamri et al., 2018a, b). Conversely, crops that require protection from excessive rainfall at the seedling stage could be placed under the PV panels during the seedling stage and replanted on the side of the lower edge of the PV array in their adult stage to receive more water (Choi, 2019). Thus, understanding the timing and direction of rainfall is crucial to placing and orienting PV panels in order to bring about a controlled and favorable soil moisture distribution.
The effects of panel orientation on precipitation redistribution by solar panels indicate that solar arrays could be used to manipulate soil moisture distribution, an important factor when evaluating the potential to co-locate crops with solar infrastructure. Further, the environmental consequences of PV installation like those identified here should be quantified and modeled to inform site preservation, to evaluate long-term impacts of onsite management strategies, and to optimize soil moisture, nutrient, and sunlight availability when co-locating natural vegetation or crops with photovoltaic arrays.
Data Availability Statement
The datasets generated for this study are available on request to the corresponding author.
Author Contributions
CC, AC, JM, and SR conceived and planned the study. CC, AC, and JM carried out the field data collection. CC, SR, JC, and DB conducted the laboratory and data analyses. CC, SR, JC, and JM interpreted the results. CC, SR, and JC wrote the manuscript. All authors provided critical feedback and helped shape the research, analysis, and manuscript.
Funding
This study was funded by the Meeting SunShot Cost and Deployment targets through Innovative Site Preparation and Impact Reductions on the Environment (INSPIRE) program of the US Department of Energy.
Conflict of Interest
The authors declare that the research was conducted in the absence of any commercial or financial relationships that could be construed as a potential conflict of interest.
Acknowledgments
Special thanks to Robert Bickhart for assistance in the field. Publication of this article was funded in part by the Temple University Libraries Open Access Publishing Fund.
Supplementary Material
The Supplementary Material for this article can be found online at: https://www.frontiersin.org/articles/10.3389/fenvs.2020.00140/full#supplementary-material
References
Armstrong, A., Ostle, N. J., and Whitaker, J. (2016). Solar park microclimate and vegetation management effects on grassland carbon cycling. Environ. Res. Lett. 11:074016. doi: 10.1088/1748-9326/11/7/074016
Armstrong, A., Waldron, S., Whitaker, J., and Ostle, N. J. (2014). Wind farm and solar park effects on plant-soil carbon cycling: uncertain impacts of changes in ground-level microclimate. Glob. Chang. Biol. 20, 1699–1706. doi: 10.1111/gcb.12437
Arya, L. M., Leij, F. J., Shouse, P. J., and van Genuchten, M. T. (1999). Relationship between the hydraulic conductivity function and the particle-size distribution. Soil Sci. Soc. Am. J. 63, 1063–1070. doi: 10.2136/sssaj1999.6351063x
Barron-Gafford, G. A., Minor, R. L., Allen, N. A., Cronin, A. D., Brooks, A. E., and Pavao-Zuckerman, M. A. (2016). The photovoltaic heat island effect: larger solar power plants increase local temperatures. Sci. Rep. 6:35070. doi: 10.1038/srep35070
Barron-Gafford, G. A., Pavao-Zuckerman, M. A., Minor, R. L., Sutter, L. F., Barnett-Moreno, I., Blackett, D. T., et al. (2019). Agrivoltaics provide mutual benefits across the food–energy–water nexus in drylands. Nat. Sustain. 2, 848–855. doi: 10.1038/s41893-019-0364-5
Beatty, B., Macknick, J., Mccall, J., Braus, G., and Buckner, D. (2017). Native Vegetation Performance Under a Solar PV Array at the National Wind Technology Center. Washington, DC: U.S. Department of Energy, doi: 10.2172/1357887
Breyer, C., Bogdanov, D., Gulagi, A., Aghahosseini, A., Barbosa, L. S. N. S., Koskinen, O., et al. (2017). On the role of solar photovoltaics in global energy transition scenarios. Prog. Photovoltaics Res. Appl. 25, 727–745. doi: 10.1002/pip.2885
Choi, C. S. (2019). Combined Land use of Solar Infrastructure and Agriculture for Socioeconomic and Environmental Co-Benefits. Maters Thesis, Temple University, Philadelphia, PA.
Cook, P. (2011). Infrastructure, rural electrification and development. Energy Sustain. Dev. 15, 304–313. doi: 10.1016/j.esd.2011.07.008
De Marco, A., Petrosillo, I., Semeraro, T., Pasimeni, M. R., Aretano, R., and Zurlini, G. (2014). The contribution of Utility-scale solar energy to the global climate regulation and its effects on local ecosystem services. Glob. Ecol. Conserv. 2, 324–337. doi: 10.1016/j.gecco.2014.10.010
Dupraz, C., Marrou, H., Talbot, G., Dufour, L., Nogier, A., and Ferard, Y. (2011). Combining solar photovoltaic panels and food crops for optimising land use: towards new agrivoltaic schemes. Renew. Energy 36, 2725–2732. doi: 10.1016/j.renene.2011.03.005
D’Odorico, P., Caylor, K., Okin, G. S., and Scanlon, T. M. (2007). On soil moisture-vegetation feedbacks and their possible effects on the dynamics of dryland ecosystems. J. Geophys. Res. Biogeosci. 112:G4. doi: 10.1029/2006JG000379
Edenhofer, O., Pichs-Madruga, R., Sokona, Y., Seyboth, K., Eickemeier, P., Matschoss, P., et al. (2011). “IPCC, 2011: summary for policymakers,” in IPCC Special Report on Renewable Energy Sources and Climate Change Mitigation, (Cambridge: Cambridge University Press), doi: 10.5860/CHOICE.49-6309
Elamri, Y., Cheviron, B., Lopez, J. M., Dejean, C., and Belaud, G. (2018a). Water budget and crop modelling for agrivoltaic systems: application to irrigated lettuces. Agric. Water Manag. 208, 440–453. doi: 10.1016/j.agwat.2018.07.001
Elamri, Y., Cheviron, B., Mange, A., Dejean, C., Liron, F., and Belaud, G. (2018b). Rain concentration and sheltering effect of solar panels on cultivated plots. Hydrol. Earth Syst. Sci. 22, 1285–1298. doi: 10.5194/hess-22-1285-2018
Ellabban, O., Abu-Rub, H., and Blaabjerg, F. (2014). Renewable energy resources: current status, future prospects and their enabling technology. Renew. Sustain. Energy Rev. 39, 748–764. doi: 10.1016/j.rser.2014.07.113
Field, J. P., Belnap, J., Breshears, D. D., Neff, J. C., Okin, G. S., Whicker, J. J., et al. (2010). The ecology of dust. Front. Ecol. Environ. 8:423–430. doi: 10.1890/090050
Fthenakis, V., and Kim, H. C. (2009). Land use and electricity generation: a life-cycle analysis. Renew. Sustain. Energy Rev. 13, 1465–1474. doi: 10.1016/j.rser.2008.09.017
Fthenakis, V., and Kim, H. C. (2010). Life-cycle uses of water in U.S. electricity generation. Renew. Sustain. Energy Rev. 14, 2039–2048. doi: 10.1016/j.rser.2010.03.008
Fthenakis, V. M., Betita, R., Shields, M., Vinje, R., Blunden, J., Shields, M., et al. (2012). “Life cycle analysis of high-performance monocrystalline silicon photovoltaic systems: energy payback times and net energy production value,” in 27th European Photovoltaic Solar Energy Conference, Frankfurt, 4667–4672.
Fthenakis, V. M., and Kim, H. C. (2011). Photovoltaics: life-cycle analyses. Sol. Energy 85, 1609–1628. doi: 10.1016/j.solener.2009.10.002
Goetzberger, A., and Zastrow, A. (1982). On the coexistence of solar-energy conversion and plant cultivation. Int. J. Sol. Energy 1, 55–69. doi: 10.1080/01425918208909875
Golubiewski, N. E. (2006). Urbanization increases grassland carbon pools: effects of landscaping in Colorado’s front range. Ecol. Appl. 16, 555–571. doi: 10.1890/1051-0761
Hassanpour Adeh, E., Selker, J. S., and Higgins, C. W. (2018). Remarkable agrivoltaic influence on soil moisture, micrometeorology and water-use efficiency. PLoS One 13:e0203256. doi: 10.1371/journal.pone.0203256
Hernandez, R. R., Armstrong, A., Burney, J., Ryan, G., Moore-O’Leary, K., Diédhiou, I., et al. (2019). Techno–ecological synergies of solar energy for global sustainability. Nat. Sustain. 2, 560–568. doi: 10.1038/s41893-019-0309-z
Hernandez, R. R., Easter, S. B., Murphy-Mariscal, M. L., Maestre, F. T., Tavassoli, M., Allen, E. B., et al. (2014). Environmental impacts of utility-scale solar energy. Renew. Sustain. Energy Rev. 29, 766–779. doi: 10.1016/j.rser.2013.08.041
Hernandez, R. R., Hoffacker, M. K., Murphy-Mariscal, M. L., Wu, G. C., and Allen, M. F. (2015). Solar energy development impacts on land cover change and protected areas. Proc. Natl. Acad. Sci. U.S.A. 112, 13579–13584. doi: 10.1073/pnas.1517656112
Hoffacker, M. K., Allen, M. F., and Hernandez, R. R. (2017). Land-sparing opportunities for solar energy development in agricultural landscapes: a case study of the great central valley. CA, United States. Environ. Sci. Technol. 51, 14472–14482. doi: 10.1021/acs.est.7b05110
Hölzel, N., and Otte, A. (2003). Restoration of a species-rich flood meadow by topsoil removal and diaspore transfer with plant material. Appl. Veg. Sci. 6, 131–140. doi: 10.1111/j.1654-109X.2003.tb00573.x
IRENA-International Renewable Energy Agency (2018). Global Energy Transformation: A Roadmap to 2050. Abu Dhabi: IRENA.
Jacobson, M. Z. (2009). Review of solutions to global warming, air pollution, and energy security. Energy Environ. Sci. 2, 148–173. doi: 10.1039/B809990C
Lal, R. (2003). Soil erosion and the global carbon budget. Environ. Int. 29, 437–450. doi: 10.1016/S0160-4120(02)00192-7
Larney, F. J., Li, L., Janzen, H. H., Angers, D. A., and Olson, B. M. (2016). Soil quality attributes, soil resilience, and legacy effects following topsoil removal and one-time amendments. Can. J. Soil Sci. 96, 177–190. doi: 10.1139/cjss-2015-0089
Larney, F. J., Olson, B. M., Janzen, H. H., and Lindwall, C. W. (2000). Early impact of topsoil removal and soil amendments on crop productivity. Agron. J. 92, 948–956. doi: 10.2134/agronj2000.925948x
Leccisi, E., Raugei, M., and Fthenakis, V. (2016). The energy and environmental performance of ground-mounted photovoltaic systems - A timely update. Energies 9:622. doi: 10.3390/en9080622
Li, J., Okin, G. S., Alvarez, L., and Epstein, H. (2008). Effects of wind erosion on the spatial heterogeneity of soil nutrients in two desert grassland communities. Biogeochemistry 88, 73–88. doi: 10.1007/s10533-008-9195-6
Macknick, J., Beatty, B., and Hill, G. (2013). Overview of Opportunities for Co-Location of Solar Energy Technologies and Vegetation. Golden, CO: National Renewable Energy Laboratory.
Mani, M., and Pillai, R. (2010). Impact of dust on solar photovoltaic (PV) performance: research status, challenges and recommendations. Renew. Sustain. Energy Rev. 14, 3124–3131. doi: 10.1016/j.rser.2010.07.065
Marrou, H., Dufour, L., and Wery, J. (2013a). How does a shelter of solar panels influence water flows in a soil-crop system? Eur. J. Agron. 50, 38–51. doi: 10.1016/j.eja.2013.05.004
Marrou, H., Guilioni, L., Dufour, L., Dupraz, C., and Wery, J. (2013b). Microclimate under agrivoltaic systems: is crop growth rate affected in the partial shade of solar panels? Agric. For. Meteorol. 177, 117–132. doi: 10.1016/j.agrformet.2013.04.012
Marrou, H., Wery, J., Dufour, L., and Dupraz, C. (2013c). Productivity and radiation use efficiency of lettuces grown in the partial shade of photovoltaic panels. Eur. J. Agron. 44, 54–66. doi: 10.1016/j.eja.2012.08.003
Mazaheri, M. R., and Mahmoodabadi, M. (2012). Study on infiltration rate based on primary particle size distribution data in arid and semiarid region soils. Arab. J. Geosci. 5, 1039–1046. doi: 10.1007/s12517-011-0497-y
McGeehan, S. L., and Naylor, D. V. (1988). Automated instrumental analysis of carbon and nitrogen in plant and soil samples. Commun. Soil Sci. Plant Anal. 19, 493–505. doi: 10.1080/00103628809367953
Mejia, F. A., and Kleissl, J. (2013). Soiling losses for solar photovoltaic systems in California. Sol. Energy 95, 357–363. doi: 10.1016/j.solener.2013.06.028
Mohammad, A. G., and Adam, M. A. (2010). The impact of vegetative cover type on runoff and soil erosion under different land uses. Catena 81, 97–103. doi: 10.1016/j.catena.2010.01.008
Nelson, D. W., and Sommers, L. E. (1996). “Total carbon, organic carbon, and organic matter,” in Methods of soil Analysis: Part 3 Chemical Methods, eds M. E. S. D. L. Sparks, J. M. Bartels, D. L. Sparks, A. L. Page, P. A. Helmke, R. H. Loeppert, et al. (Madison, WI: Soils Science Society of America, Inc. and American Society of Agronomy, Inc), 961–1010.
Noy-Meir, I. (1973). Desert ecosystems: environment and producers. Annu. Rev. Ecol. Syst. 4, 25–51. doi: 10.1146/annurev.es.04.110173.000325
Ong, S., Campbell, C., Denholm, P., Margolis, R., and Heath, G. (2013). Land-use Requirements for Solar Power Plants in the United States. Golden, CO: National Renewable Energy Laboratory.
Perez, M. J. R., and Fthenakis, V. (2012). Façade-integrated photovoltaics?: a life cycle and performance assessment case study. Prog. Photovolt. Res. Appl. 20, 975–990. doi: 10.1002/pip.1167
Phillips, J. (2013). Determining the sustainability of large-scale photovoltaic solar power plants. Renew. Sustain. Energy Rev. 27, 435–444. doi: 10.1016/j.rser.2013.07.003
Ravi, S. (2015). Resources: partner crop plants with solar facilities. Nature 524:161. doi: 10.1038/524161a
Ravi, S., D’Odorico, P., Breshears, D. D., Field, J. P., Goudie, A. S., Huxman, T. E., et al. (2011). Aeolian processes and the biosphere. Rev. Geophys. 49:RG3001. doi: 10.1029/2010RG000328
Ravi, S., Lobell, D. B., and Field, C. B. (2014). Tradeoffs and synergies between biofuel production and large solar infrastructure in deserts. Environ. Sci. Technol. 48, 3021–3030. doi: 10.1021/es404950n
Ravi, S., Macknick, J., Lobell, D., Field, C., Ganesan, K., Jain, R., et al. (2016). Colocation opportunities for large solar infrastructures and agriculture in drylands. Appl. Energy 165, 383–392. doi: 10.1016/j.apenergy.2015.12.078
Sherwani, A. F., Usmani, J. A., and Varun (2010). Life cycle assessment of solar PV based electricity generation systems: a review. Renew. Sustain. Energy Rev. 14, 540–544. doi: 10.1016/j.rser.2009.08.003
Turney, D., and Fthenakis, V. (2011). Environmental impacts from the installation and operation of large-scale solar power plants. Renew. Sustain. Energy Rev. 15, 3261–3270. doi: 10.1016/j.rser.2011.04.023
U. S. Department of Energy (2012). SunShot Vision Study. doi:SunShot, Energy Efficiency and Renewable Energy. NREL Report No. BK5200-47927. Washington, DC: U.S. Department of Energy.
Vomocil, J. A., and Flocker, W. J. (1961). Effect of soil compaction on storage and movement of soil air and water. Trans. ASAE 4, 0242–0246. doi: 10.13031/2013.41066
Walston, L. J., Mishra, S. K., Hartmann, H. M., Hlohowskyj, I., McCall, J., and Macknick, J. (2018). Examining the potential for agricultural benefits from pollinator habitat at solar facilities in the United States. Environ. Sci. Technol. 52, 7566–7576. doi: 10.1021/acs.est.8b00020
Walston, L. J., Rollins, K. E., LaGory, K. E., Smith, K. P., and Meyers, S. A. (2016). A preliminary assessment of avian mortality at utility-scale solar energy facilities in the United States. Renew. Energy 92, 405–414. doi: 10.1016/j.renene.2016.02.041
Keywords: land use change, infiltration, soil moisture, renewable energy, agrivoltaics, co-location
Citation: Choi CS, Cagle AE, Macknick J, Bloom DE, Caplan JS and Ravi S (2020) Effects of Revegetation on Soil Physical and Chemical Properties in Solar Photovoltaic Infrastructure. Front. Environ. Sci. 8:140. doi: 10.3389/fenvs.2020.00140
Received: 28 February 2020; Accepted: 23 July 2020;
Published: 11 August 2020.
Edited by:
Sonja Knapp, Helmholtz Centre for Environmental Research (UFZ), GermanyReviewed by:
Bracha Schindler, University of Haifa, IsraelPerrine Hamel, Nanyang Technological University, Singapore
Copyright © 2020 Choi, Cagle, Macknick, Bloom, Caplan and Ravi. This is an open-access article distributed under the terms of the Creative Commons Attribution License (CC BY). The use, distribution or reproduction in other forums is permitted, provided the original author(s) and the copyright owner(s) are credited and that the original publication in this journal is cited, in accordance with accepted academic practice. No use, distribution or reproduction is permitted which does not comply with these terms.
*Correspondence: Chong Seok Choi, chong.seok.choi@temple.edu