Soil solarization as a non-chemical weed control method in tree nursery production systems of the Pacific Northwest, USA
- Department of Crop and Soil Science, Oregon State University, Corvallis, OR, United States
Introduction: Herbicide application in tree nurseries is limited because of the potential for chemical injury to the large diversity of trees species grown, the lack of registered products, and increasing restrictions on herbicide use, necessitating the costly practice of hand weeding. Soil solarization can reduce the weed seedbank by trapping solar energy under clear plastic film, resulting in high soil temperatures lethal to imbibed weed seeds and seedlings. The objective of this study was to determine if soil solarization would be an effective weed management strategy in Pacific Northwest, USA, tree production systems.
Methods: Field studies were conducted at three commercial tree nurseries in Oregon and Washington over two years to test soil solarization in reducing the naturally occurring weed seedbank and the time required to hand weed fields. Further field and laboratory tests were conducted with five weed species: Poa annua, Polygonum pensylvanicum, Amaranthus retroflexus, Portulaca oleracea, and Cyperus esculentus. Weed seeds and tubers were buried in packets at 5 and 10 cm to determine their viability after 6 weeks of solarization. A laboratory study was conducted with all but C. esculentus to quantify the exposure time at 45, 50, and 55°C required for 90% death (T90).
Results: Soil solarization was particularly effective in reducing the emergence of naturally occurring weeds in the fall and winter, when weed emergence was reduced by 94-96%. Emergence was reduced 67-81% during the subsequent spring and early summer. Nine to ten months after solarization, solarized areas had a 52 – 69% reduction in hand weeding time compared to non-solarized areas. In field trials with buried seed and tuber packets, mortality differed by location and depth, with P. annua and P. pensylvanicum having the greatest percent seed mortality followed by A. retroflexus and variable results for P. oleracea and C. esculentus. In lab studies, seed mortality differed depending on species and temperature; however, at 55°C, there was a relatively rapid drop in seed viability for all species, and T90 values ranged from 1.2 to 41 h whereas at 45°C the range was 47 to > 3000 h. Similar to the field studies, P. annua and P. pensylvancium were more sensitive to heat, followed by A. retroflexus and P. oleracea.
Conclusion: Soil solarization can be an effective weed management tool in reducing the weed seedbank in Pacific Northwest tree nurseries and other fall-sown crops but may not work for certain, thermotolerant weed species such as C. esculentus.
1 Introduction
Production of tree seedlings is an integral part of the agricultural and forest products economy in the Pacific Northwest (PNW), USA (USDA, 2014). Over $1.1 billion in nursery and greenhouse products were sold in 2020 with approximately one-third of the crop value from field-grown trees (ODA, 2022). PNW nurseries also produce bareroot conifer and hardwood seedlings for reforestation; in 2022, 79 million seedlings were produced and 185,350 hectares were planted in Oregon and Washington (Pike et al., 2023). Because of the increased restrictions for use of certain soil fumigants (EPA, 2012), the limited number of herbicide options, and the potential for crop injury, tree seedling nurseries rely on hand weeding after crop establishment which is labor intensive and costly. For these reasons, there is a strong demand to find an alternative to soil fumigation.
Soil solarization is a non-chemical pre-planting practice which has been found to be comparable to other methods to manage soilborne pathogens and weeds in regions with high solar radiation (Stapleton and DeVay, 1986; Gullino et al., 2022). Soil solarization creates conditions lethal to many mesophilic weed species that grow between 20 and 45°C by heating soil under a clear film applied over the soil surface during the summer months. To ensure good contact of the film with the soil surface, soil solarization is applied in the following steps: soil is cultivated, smoothed, irrigated, and the film is laid (Elmore et al., 1997; Wilen and Elmore, 2007). The film is sealed by burying the edges to reduce heat escape. The treatment durations differ depending on local conditions, but there is general agreement that 6 weeks of solarization is effective against many pests (Stapleton et al., 2005). The Pacific Northwest was previously considered to be a marginally suitable area for soil solarization because of its short, mild summers. However, Parke (2016) found that clear plastic film with anti-condensation (AC) and infra-red retaining (IR) properties increased the maximum soil temperature achieved during soil solarization compared to the previous studies conducted in Oregon (Pinkerton et al., 2000; Peachey et al., 2001). These new types of plastics improved energy capture and heat retention and made soil solarization a more feasible practice comparable to locations, for example California, where soil solarization has been utilized previously with success (Stapleton et al., 2005).
The effect of soil solarization is greatest near the soil surface and decreases with depth (Stapleton, 2000). Because most of the viable seeds in the soil seedbank are concentrated in the top 5 cm, soil solarization works best when it is followed by planting practices with little to no disturbance of the soil (Akinola et al., 1998). Reduced survival of weed seeds in soil ultimately reduces the weed population density, thus, reducing the cost of weed control. However, the efficacy of soil solarization can vary based on the weed species and environmental conditions (Stapleton et al., 2005).
The main mode of action of soil solarization is hydrothermal killing of seeds and seedlings (Katan and DeVay, 1991; Stapleton, 2000). Annual weeds, such as Sonchus oleraceus (L.), Poa annua (L.), and Polygonum equisetiforme (S.), are more effectively controlled by soil solarization than are perennial species (Rubin and Benjamin, 1984; Peachey et al., 2001). Among the annual species, winter annuals have lower thermotolerance than summer annuals because they are better adapted to germinate at lower soil temperatures (Rubin and Benjamin, 1984; Egley, 1990; Elmore, 1991; Hoyle and McElroy, 2009). Winter annual species, compared to summer annual species, germinate in shorter day conditions, are more temperature-sensitive and require smaller temperature increases to be effectively controlled (Egley, 1990). A 1-week solarization period was enough to control susceptible winter annuals such as Poa annua, Montia perfoiata (Donn ex Willd.) Howell and Senecio vulgaris L. (Katan and DeVay, 1991), whereas summer annual species required higher solarization temperatures and/or a longer duration (Egley, 1990). Hard seeded species, regardless of the life cycle, are generally not controlled by soil solarization (Elmore, 1991).
A wide range of weed species have been reported to be susceptible to soil solarization (Cohen and Rubin, 2007), but results can be inconsistent due to the variation in environmental conditions, soil type, plastic type used, evaluation methods, weed seed source, and seed position in the soil profile (Standifer et al., 1984; Al-Hammadi, 2006). Imbibed seeds become more vulnerable to high temperatures due to increased metabolic activity (Egley, 1990). In addition, for some weed species, soil solarization can promote germination by creating higher temperatures in the soil profile (Londale, 1993) and increased concentrations (Rubin and Benjamin, 1984; Baskin and Baskin, 1998). Both maximum soil temperature and accumulated soil temperature determine the hydrothermal effect on weed seeds (Stapleton et al., 2005). Soil moisture is needed for seed imbibition but also improves the temperature conductivity so that heat reaches deeper depths within the soil profile (Maher et al., 1986).
Although hydrothermal killing is the main mode of action in solarization, there are other factors that can influence the state of seeds or soilborne pathogens such as fluctuating daily temperatures, soil moisture, nutrient composition, and microbial community shifts (DeVay and Katan, 1991; Funahashi and Parke, 2016; Funahashi and Parke, 2018; Funahashi and Parke, 2020; Funahashi et al., 2021). These changes in environmental conditions created by soil solarization can induce or release seed dormancy and affect the sensitivity of seeds depending on species. Induced seed dormancy caused by soil solarization does not reduce the seedbank. However, dormancy could still reduce the weed infestation in the following seasons by increasing crop competition, reducing control costs, and decreasing seed viability due to microbial decay or predation. Soil solarization that does not increase temperatures enough to cause seed mortality may still reduce seed vigor of sensitive species which survive the treatment (Stapleton, 1990).
When soil solarization releases seeds from dormancy, fatal germination may occur at depths greater than a seedling can emerge. In addition, seedlings are typically more sensitive to heat than seeds. Thus, if seeds germinate, seedlings could be killed before they reach the soil surface by soil temperatures created by soil solarization. Fatal germination can contribute to the long-term effect of soil solarization by diminishing the number of viable seeds in the soil profile.
The objective of the study was to determine if soil solarization would be an effective non-chemical weed management strategy in PNW commercial tree nurseries. Studies were conducted to evaluate the use of soil solarization to reduce naturally occurring weed seedbank populations, reduce the time required for hand weeding in planted tree seedling fields and to predict the response of selected weed species to soil solarization by determining thermal death curves under controlled laboratory conditions.
2 Materials and methods
2.1 Field studies
2.1.1 Site description
Field experiments were conducted at three tree seedling nursery sites in the Pacific Northwest: Clackamas Co., Oregon; Yamhill Co., Oregon; and Thurston Co., Washington during the summers of 2016 and 2017 (Table 1). Seed bed preparation, fertilization, seeding, and hand weeding were conducted by the nursery staff.
2.1.2 Plot establishment
For both naturally occurring and buried seed packets, plots were established on three raised beds with three replications of two treatments (solarized or non-solarized). The treatment duration was 6 weeks during July and August. Individual plots were 1.2 m by 30.5 m with a 4.7 m buffer between the treatments.
2.1.3 Naturally occurring weed emergence
In order to assess soil solarization efficacy on naturally occurring weed populations, non-solarized plots were sprayed with glyphosate at 0.75 kg a.e. ha -1 to control weeds that emerged during the 6-week solarization period. Three to 11 months after soil solarization and film removal, naturally-occurring weeds were identified and counted in the Clackamas and Yamhill trials before weed control measures were taken. Quadrats (50 cm x 50 cm) were placed at 1, 8, 15, and 22 m along the center of each plot to avoid edge effects and disturbed areas caused by seed packet and instrumentation removal.
2.1.4 Hand weeding time in solarized and non-solarized treatments
Standard weeding protocols were used at each site by nursery staff. The time required to hand weed solarized and non-solarized plots (1.2 m by 30.5 m) was recorded following emergence counts of naturally occurring weeds in the trials at the Clackamas and Yamhill locations during fall/winter and spring/summer months.
2.1.5 Seed packet preparation
Five weed species were tested: Poa annua (L.), Polygonum pensylvanicum (L.), Amaranthus retroflexus (L.), Portulaca oleracea (L.) and at one site, Cyperus esculentus (L.). Portulaca oleracea and P. pensylvanicum seeds were planted, and plants were maintained in a greenhouse located in Corvallis, OR, to produce seeds for the study. Populations of the other species were obtained through seed collection from fields in Benton or Yamhill Co, OR. Seeds were stored at room temperature (21°C) in dry, dark conditions until use. Germination of the stored seeds was > 95% for each species (data not shown). Fifty seeds of each species were sealed in a packet of water permeable nylon mesh (105-µm, 4 cm by 4 cm; Pentair Aquatic Eco-Systems, Inc., Apopka, FL). A packet of each species was placed randomly inside a larger flat bag made of window screen and sealed (26 cm by 10 cm). Nylon mesh packets and window screen bags were sealed using an impulse sealer (AIE-305; American International Electric Inc., City of Industry, CA).
Cyperus esculentus tubers were collected at the experimental site in Thurston Co, WA. Tuber size ranged between 0.8 cm to 1.5 cm. Cyperus esculentus was included only in the Thurston trials to avoid the introduction of the species to the other locations. Fifty tubers of C. esculentus were sealed in a window screen bag (12 cm by 10 cm).
2.1.6 Buried packet placement
Weed packets were buried at 5 and 10 cm depths at the center of each plot. Soil temperature was monitored at 5 cm and 15 cm with CS655 sensors attached to a CR-1000 datalogger (Campbell Scientific, Logan, UT) (Hill, 2019) and at 10 cm using iButtons (Wada, 2019) (Thermochron DS1922L, OnSolution Pty. Ltd., Castle Hill, NSW, Australia). Measurements were taken every 30 min throughout the duration of the trial. Sensors were installed at the center of each plot near where packets were buried. Total accumulated temperature hours during the 6-week studies were grouped into 5°C ranges and classified by solarized and non-solarized plots, depth, and location. An HMP60 Campbell SCI weather station was used at each location to monitor air temperature.
The plots were irrigated to field capacity at the beginning of the trials using overhead irrigation the night before the plastic application at the Yamhill and Thurston locations. At the Clackamas location, three lines of drip irrigation tape were installed on top of the beds, and plots were irrigated after the plastic was applied. Non-solarized plots were irrigated the same as the solarized treatment at each site.
Solarized plots were covered with clear plastic film ‘C790-IR-AC low tunnel’ (1.4 mil; Ginegar Plastic Products, Ltd, Santa Maria, CA). The edges of the film were held in place by covering the edge with a 30-cm wide band of soil along the raised beds. No film was applied to the non-solarized treatment.
2.1.7 Seed viability assessment
Seed packets were removed after 6 weeks and seed viability was assessed in the laboratory. Any seeds in the packets that germinated pre-removal were counted as dead (fatal germination). Intact seeds were placed in a Petri dish containing a blotter paper moistened with 10 mL deionized water. Seeds were incubated in a dark growth chamber set to 12 h alternating temperatures of 15/20°C for P. annua and 20/26°C for the other species. Germinated seeds were counted after 14 days. Seeds were considered germinated when the emerged radicals were greater than 3 mm long. Seeds that did not germinate during this period were assessed using the tetrazolium (TZ) staining method to confirm whether seeds were dormant or dead (Patil and Dadlani, 2009). Seed coats were partially removed or pierced with a fine needle and soaked in 1% triphenyl TZ chloride solution (Sigma-Aldrich, St. Louis, MO). Seeds were incubated at 30°C for 6 h in the dark for P. annua and 10 h for other species. The embryos were exposed under a dissection microscope and counted as viable when the embryo stained red and had no deformation or fungal infection. Percent total seed viability (TV) was calculated as the sum of non-dormant and dormant seed (% positive TZ test).
2.1.8 Tuber viability
Cyperus esculentus seedlings that emerged during the trial were uprooted and transplanted individually in a pot (3 × 3 × 6 cm, Growers Nursery Supply, Inc. Salem, OR) and placed in a greenhouse after the trial to determine viability. The greenhouse environment was 27/20°C day/night with 14 h of light in addition to ambient sunlight. Survival counts were taken after 2 weeks. The number of tubers recovered from the packets was recorded, and tubers were planted in a plastic tray (25 × 25 × 6 cm) filled with potting mix (Sunshine Mix 1 Potting Mix, Sun Gro Horticulture, Bellevue, WA) and grown under the same greenhouse conditions as the transplanted seedlings. Trays were watered as necessary, and sprouting was assessed after 4 wk. Tuber viability based on tuber sprouting and survival rate of transplanted seedlings was compared between non-solarized and solarized samples.
2.2 Statistical analysis
2.2.1 Field studies
The buried seed packet, weed emergence, and hand weeding time data were subjected to Welch’s t-test to compare the mean of the response variables to solarized and non-solarized treatments from the same trial year and site. The buried seed packet trial also included burial depth as a response variable. The location and timing data for weed emergence were not pooled because of sample variance (Levene’s test, P< 0.05), and differences in mean and interactions tested by ANOVA (P< 0.05). R (version 3.5.2) and the Agricolae package were utilized for each analysis.
2.2.2 Laboratory studies
The experiment was conducted in growth chambers utilizing a completely randomized design with both temperature and duration as independent variables. Seed viability was a dependent variable to the treatment. The study was repeated. Based on Levene’s test for homogeneity of variance, there were no differences in the variability of seed viability in the two trials for each species of the same treatment and duration (P > 0.05). Therefore, data from the two trials were pooled and analyzed as 6 replications. Seed mortality data were analyzed using the DRC package on R (version 3.5.2) and fitted to the 2-parameter Weibull model defined as follows:
where v is percent viability of seeds, b is the slope of the curve, the parameter d is a duration of the treatment in hours, and the parameter a is a duration of the treatment in hours at the inflection point of the viability curve. The upper limit of seed viability was fixed to 100%, and the lower limit to 0%. For each temperature treatment, parameter estimates, the time required to kill 90% of seeds tested (), and 95% confidence intervals were determined using the summary and estimated effective temperature and time for seed mortality.
2.3 Laboratory study
2.3.1 Seed preparation
Four weed species were tested: P. annua, P. pensylvanicum, A. retroflexus, and P. oleracea. The same weed seed collections were used in the laboratory study as the field study. Twenty-five seeds of each species were placed on moist blotter paper (Steel Blue Blotter; Anchor Paper Corporation, St. Paul, MN) and sealed in a capsule (Meter Group, Pullman, WA; 3.9 x 1.1 cm diameter) before being placed in germination chambers.
2.3.2 Heat treatments
Three temperature treatments, 45, 50, 55°C, were chosen from the range of reported temperatures in the top 5 cm of the soil profile during the soil solarization field trials (Table 2). The capsules enclosing the imbibed seeds were incubated at a constant temperature. The study included three replications for each treatment and species. The study was repeated. The sampling time interval varied from 0.5 h to 24 h based on the sensitivity of a species to each temperature treatment. The incubation trial was continued until a species reached 100% seed mortality for each temperature or for 336 h. Deionized water was added as necessary to maintain similar moisture levels within the capsules for incubation trials that lasted more than 7 d.
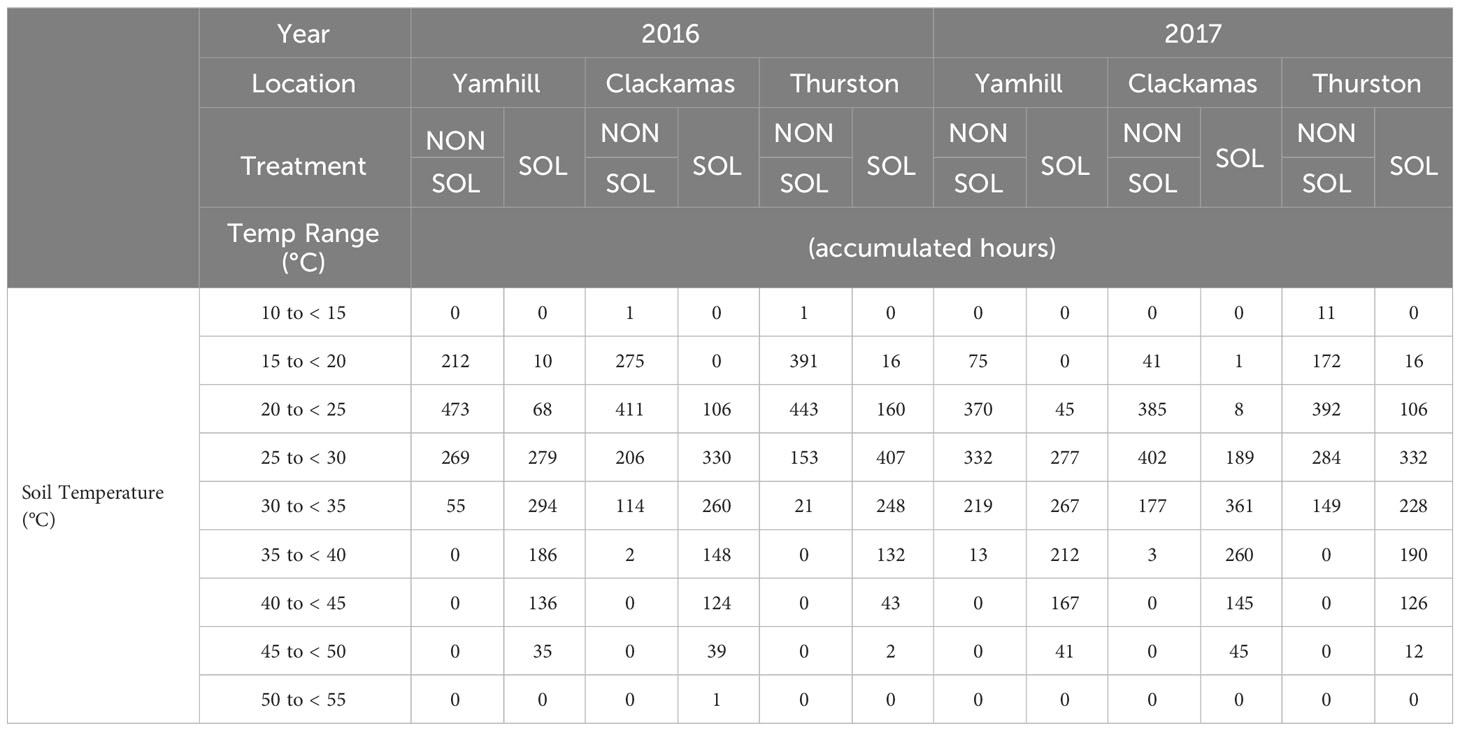
Table 2 Season-long accumulated thermal hours summary at the 5 cm depth for non-solarized (NONSOL) and solarized (SOL) treatments.
2.3.3 Seed viability assessment
After the heat treatment, seed viability was assessed using the TZ method described for the field study (Patil and Dadlani, 2009).
3 Results
3.1 Field studies
3.1.1 Soil temperature
At the three site locations during both years, temperatures ranged from 14 to 58°C in solarized plots and 11 to 44°C in non-solarized plots at the 5 cm depth (Table 2; Supplementary Figures 1–6). At 10 cm depths, temperature ranged from 16 to 51°C in solarized plots and 13 to 36°C in non-solarized plots (Table 3; Supplementary Figures 1–6).
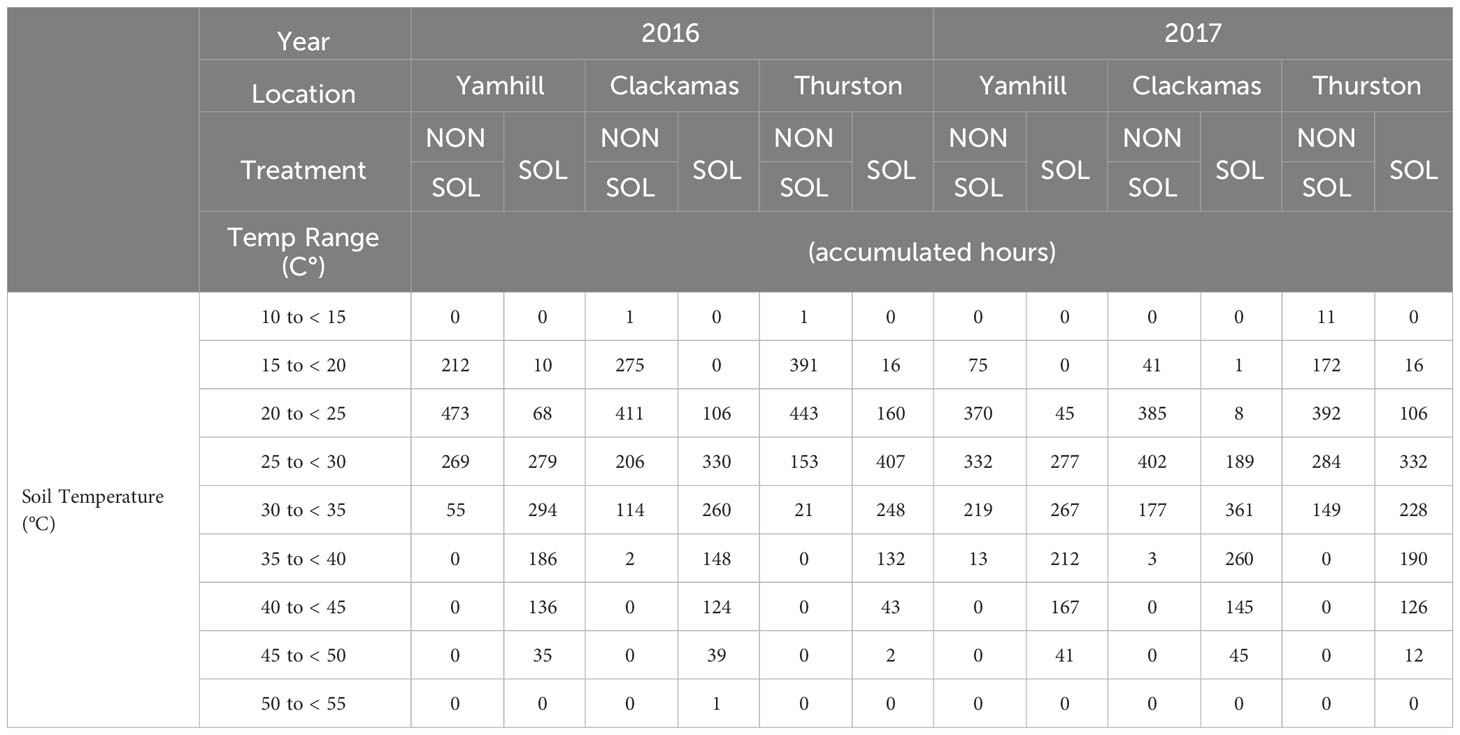
Table 3 Season-long accumulated thermal hours summary at the 10 cm depth for non-solarized (NONSOL) and solarized (SOL) treatments.
The major difference in accumulated soil temperature hours between soil solarization treatments was in the 40 to 45°C range. At this temperature range, the maxium accumulated hours in any non-solarized soil treatment was 16. There were no temperature readings above 40°C at the 10 cm depth at any site or year in non-solarzed soil plots. In contrast, accumulated hours between 40 to 45°C in solarized treatments ranged from 80 to 144 and 43 to 167 at 5 and 10 cm, respectively, over both years and across all sites. Solarized plots accumulated between 13 and 79 hr above 50°C at the 5 cm depth and only 1 hr at 10 cm.
The Washington site had fewer soil temperature accumulated hours above 40°C than the two Oregon sites in both years. The maximum soil temperatures were similar in solarized treatments during both years at the different locations; however, the maximum air temperature averaged between 0.5-3°C warmer at both Oregon locations depending on the year (Figures 1, 2). In 2017, the average maximum air temperature was ≥ 2°C warmer at each location and would account for the greater accumulated thermal hours ≥40°C than in 2016 at both the 5 and 10 cm depths (Figure 3).
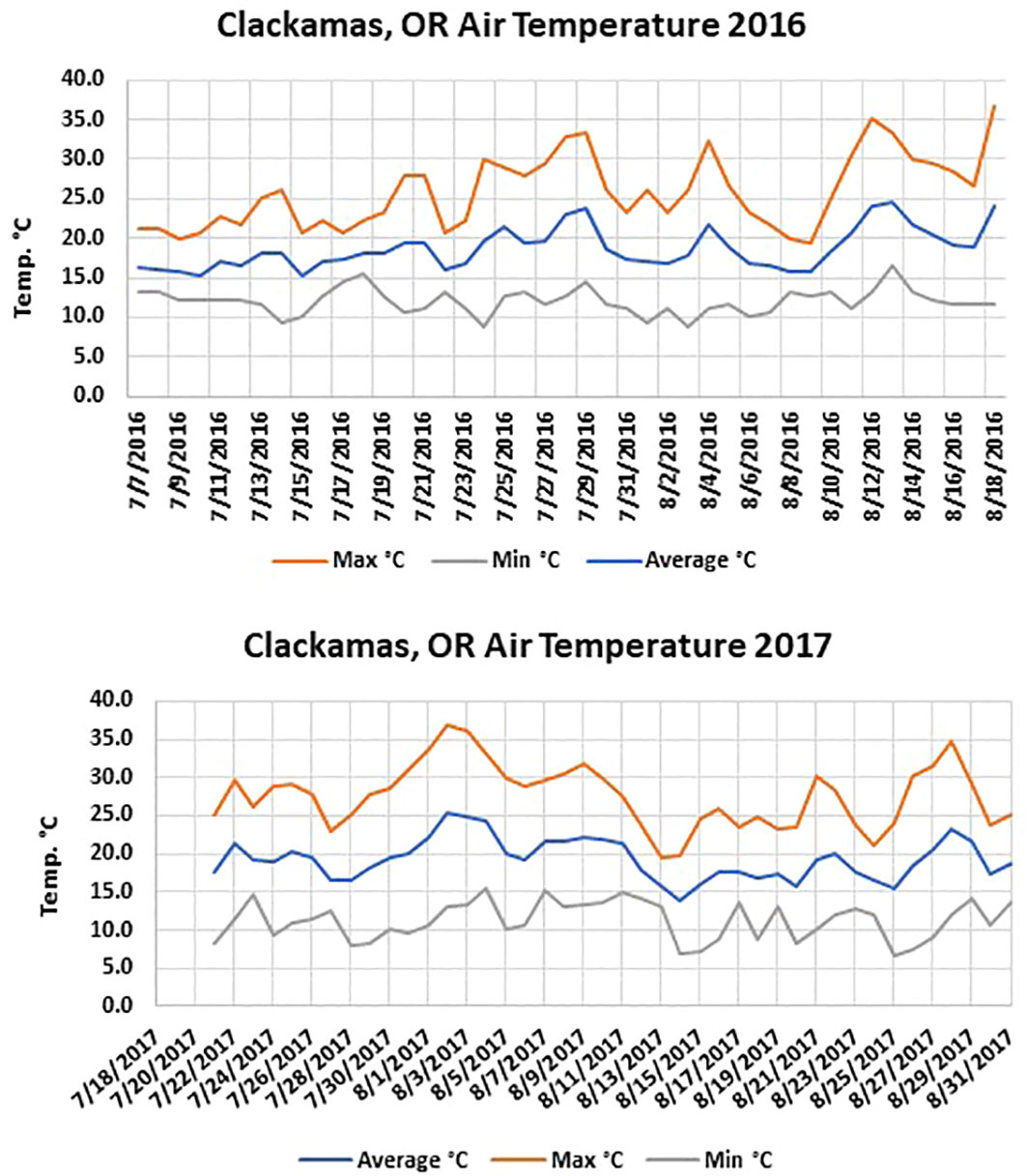
Figure 1 Air temperature at the Clackamas, OR location during the 2016 and 2017 solarization studies.
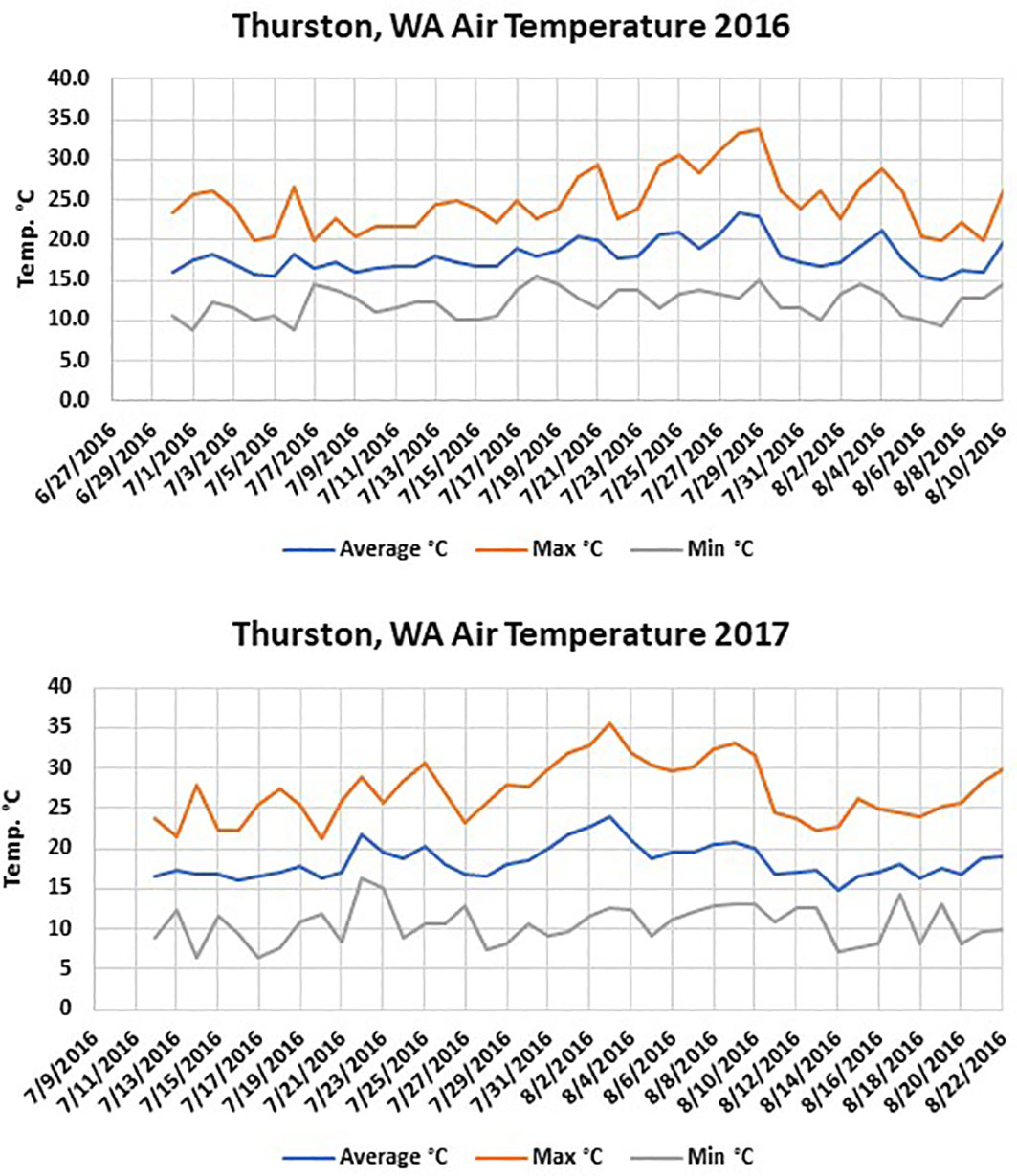
Figure 3 Air temperature at the Thurston, WA location during the 2016 and 2017 solarization studies.
3.2 Emergence of naturally occurring weed species
Naturally occurring weed species varied by location and date of emergence. Each weed species used in the burial packets, other than P. pensylvanicum, was also found at one of the sites. The other primary weeds accounting for >50% of emergence were Cardamine oligosperma (L.), Cerastium vulgatum (L.), Draba verna (L.), Capsella bursa-pastoris (L.), and Lamium amplexiaule (L.) (Supplementary Table 1). At each location and date, weed seedling emergence was significantly reduced in the solarized treatment compared to the non-solarized treatment. At the Clackamas site in 2016, fall weed emergence counts were 11 and 273 weeds m¯² in solarized and non-solarized plots, respectively (Figure 4). Spring weed emergence counts were 8 and 40 weeds m¯² in solarized and non-solarized plots, respectively. The winter weed emergence counts in 2017 at the Clackamas site were 7 and 115 weeds m¯² in solarized and non-solarized plots, respectively. Spring weed emergence counts from the 2017 plots were 7 and 37 weeds m¯² in solarized and non-solarized plots, respectively.
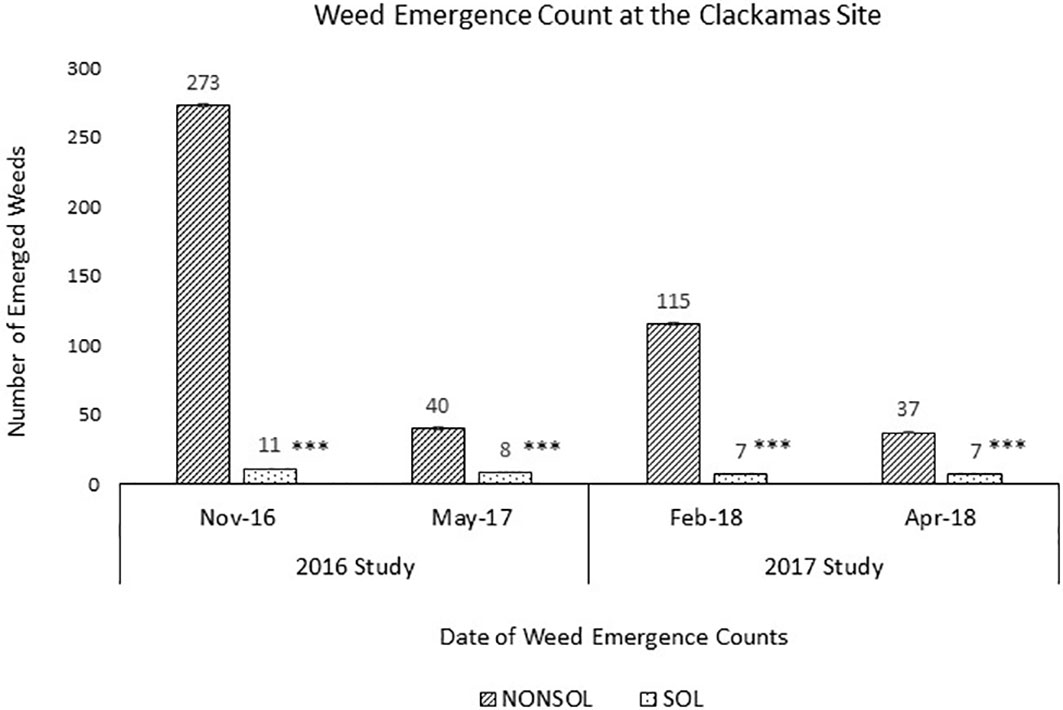
Figure 4 Weed emergence counts at the Clackamas site after solarization in 2016 and 2017 studies. Four m2 counts were taken per individual plot across three replications and averaged for non-solarized (NONSOL) and solarized (SOL) treatments. Welch's two sample t-test were carried out to compare the emergence counts between non-solarized and solarized treatments. Asterisks indicate the statistical significance with the p-value <0.05(*), 0.01(**) or 0.001(***). Values are mean of seedling count per 1m2 area.
Spring weed emergence counts on the 2016 plots at the Yamhill site were 8 and 24 weeds m¯² in solarized and non-solarized plots, respectively (Figure 5). Spring weed emergence counts in 2017 plots were 4 and 21 weeds m¯² in solarized and non-solarized plots, respectively.
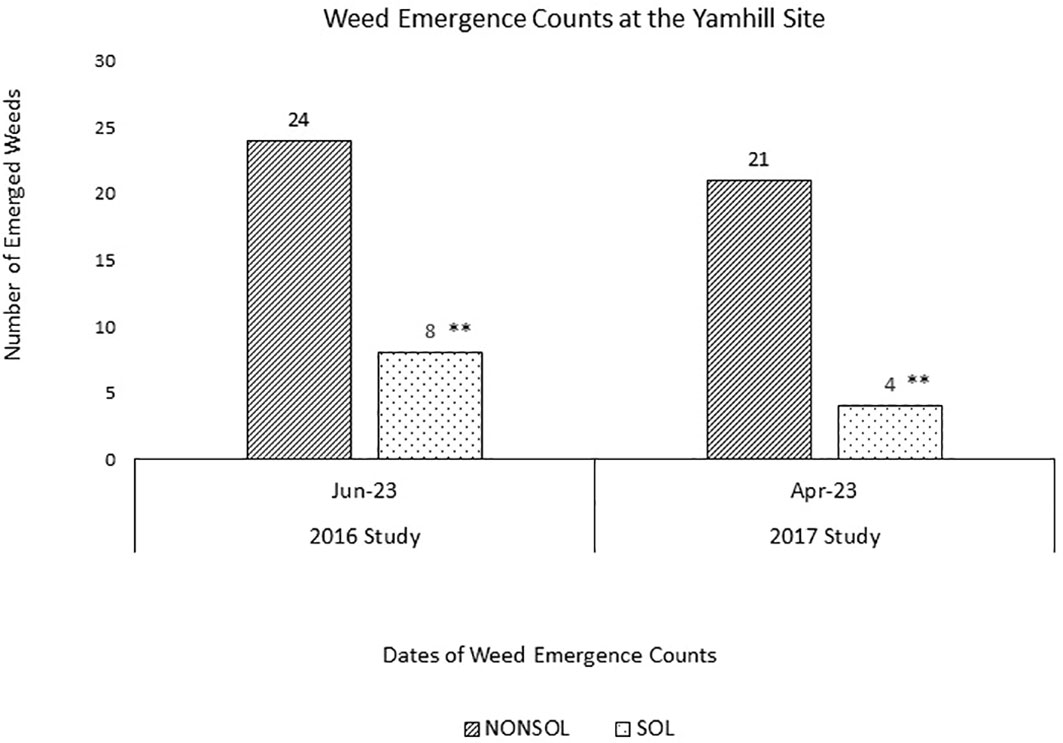
Figure 5 Weed emergence counts at the Yamhill site after solarization in 2016 and 2017 studies. Four m2 counts were taken per individual plot across three replications and averaged for non-solarized (NONSOL) and solarized (SOL) treatments. Welch's two sample t-test were carried out to compare the emergence counts between non-solarized and solarized treatments. Asterisks indicate the statistical significance with the p-value <0.05(*), 0.01(**) or 0.001(***). Values are mean of seedling count per 1m2 area.
3.3 Time required to hand weed
Soil solarization reduced weed emergence after the nursery tree seeds were planted in the fall and the effect of solarization persisted to the following early summer when hand weeding was conducted. The decrease in weed emergence resulted in a reduction in hand weeding time, translating to savings in labor costs. In 2016 and 2017, the hand weeding time was reduced in solarized beds by 69 and 63% at Clackamas, and by 56 and 52% at Yamhill, respectively (Table 4).
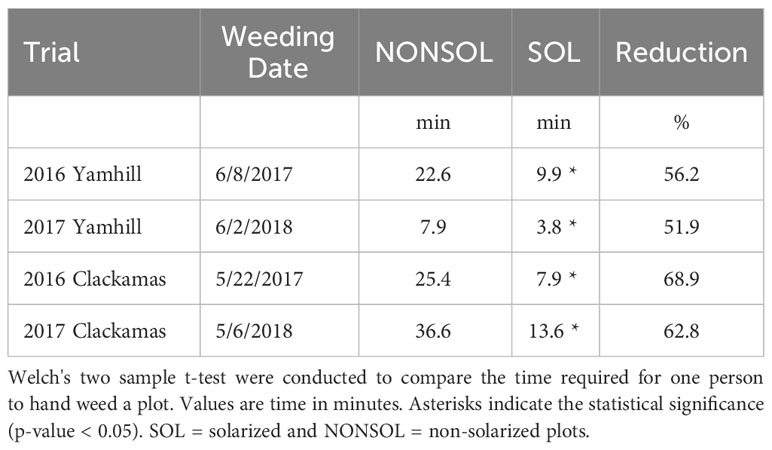
Table 4 Time (minutes) required for one person to hand weed a non-solarized (NONSOL) verses a solarized (SOL) 1.2 m x 30.5 m plot.
3.4 Fate of weed seed in buried packets
3.4.1 Fate of Poa annua weed seed in buried packets
In 2016, at the 5 cm depth, 97, 83, and 93% of P. annua seed were dead after the 6-week study in solarized plots compared to 20, 5, and 3% in non-solarized plots at the Yamhill, Clackamas, and Thurston study sites, respectively (Table 5). In solarized plots at 10 cm, 73, 64, and 57% of P. annua seed were dead compared to 7, 6, and 3% dead seed in non-solarized plots at the Yamhill, Clackamas and Thurston sites, respectively.
In 2017, at the 5 cm depth, there were 0 viable seed in solarized plots at both locations, as compared to 100% or 80% viable seed in non-solarized plots at Clackamas and Yamhill, respectively. Poa annua viability at the 10 cm depth was more variable in 2017, with 69, 90, and 95% dead seed in solarized plots and 5, 94, and 37% viable seed in non-solarized plots and at the Yamhill, Clackamas, and Thurston sites, respectively.
3.4.2 Fate of Polygonum pensylvanicum weed seed
Solarization killed P. pensylvanicum primarily by fatal germination. In the solarized plots, fatal germination ranged from 89 to 100% at the 5 cm depths in 2016 (Table 6). In 2017, solarization killed 87% of P. pensylvanicum by fatal germination at the Yamhill site and 100% of seeds were dead at the Clackamas and Yamhill sites at the 5 cm depth. In solarized plots at the 10 cm depth, seed mortality was greater than 90% at Yamhill and Thurston during both years and 91 and 65% in Clackamas in 2016 and 2017, respectively. In 2016, the non-solarized plot seed viability was 62 to 78% in the Thurston trial at 5 and 10 cm, respectively. Seed viability was more than 90% at both depths in non-solarized plots at the Yamhill and Clackamas sites.
3.4.3 Fate of Amaranthus retroflexus weed seed
Seed mortality of A. retroflexus was 2 to 84% at the 5 cm depth in solarized plots for both years and locations (Table 7). Seed mortality in solarized plots at the 10 cm depth ranged between 0 to 40% depending on the site and year. Seed mortality in non-solarized plots ranged between 0 to 1% at both 5 and 10 cm depths depending on the location and year.
In most cases the percentage of dormant seeds in solarized plots was greater than in non-solarized plots. This was true for both depths but especially at the 10 cm depth (Supplementary Table 5). In 2016, A. retroflexus seed at the 10 cm depth in solarized plots was 52, 62, and 30% dormant and seed in non-solarized plots was 16, 0, and 6% dormant at Yamhill, Clackamas, and Thurston sites, respectively. In 2017, seed at the 10 cm depth in solarized plots was 0, 92, and 50% dormant and seed in non-solarized plots was 0, 28, and 32% dormant at Yamhill, Clackamas, and Thurston sites, respectively. These results suggest A. retroflexus may be sensitive to other changes caused by the solarization, such as an imbalance of gaseous compounds (Horowitz et al., 1983), rather than the hydrothermal process alone. As a summer annual, A. retroflexus, is less sensitive to greater heat variation. Soil solarization did reduce viable seed in some years and even with increasing the proportion of dormant seed, minimal disturbance during nursery planting can reduce the number of emerged weeds.
3.4.4 Fate of Portulaca oleracea weed seed
Portulaca oleracea had the most heat-tolerant seed. In 2016, at both depths and all sites, viable seed was 63 to 85% in solarized plots and 80 to 91% viable seed in non-solarized plots (Table 8). In 2017, viable seed was 89 to 100% in solarized plots and 98 to 100% in non-solarized. The greatest seed mortality in solarized plots was 37% at 10 cm in Thurston in 2016.
3.4.5 Fate of Cyperus esculentus tubers
Solarization suppressed the sprouting of tubers during the treatment period, however, it did not reduce the frequency of sprouting of recovered tubers in comparison to the non-solarized treatment (Table 9). Chase et al., 1999, found similar results where oscillating temperatures of 45 and 26°C (day and night, respectively) slowed sprouting of C. esculentus but did not cause mortality. In a controlled study, 100% mortality of C. esculentus was achieved after 16, 8, and 2 h for 50, 55, and 60°C constant temperatures (Webster, 2003). However, a 6-week soil solarization field study was conducted where temperatures were >60°C for 49% of the study and Cyperus spp. tubers were still viable (Chase, 1999). Variation in nutsedge mortality likely reflects its different response to oscillating vs. constant high temperature (Miles et al., 1996). As this northernmost location was the only field site where C. esculentus was studied, it is not known if soil solarization at other, more southerly locations in the PNW would be effective.
4 Results – controlled study
The thermal death curve of each species is presented in Figure 6. The parameter estimates are summarized in Table 10.
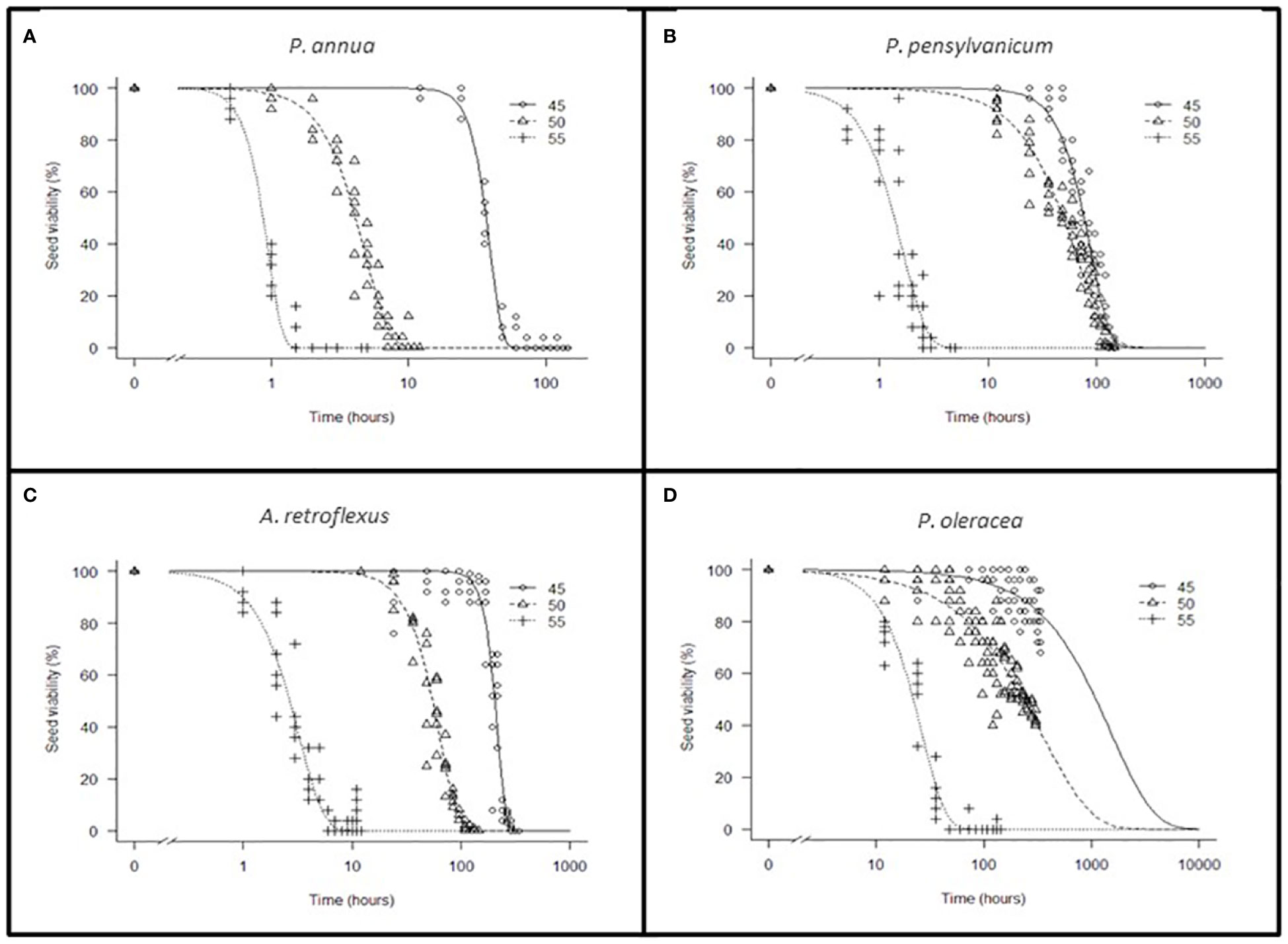
Figure 6 Thermal dose-response curve of Poa annua (A), Polygonum pensylvanicum (B), Amaranthus retroflexus (C), Portulaca oleracea (D) percent viability vs. time at constant temperature treatments. Seed viability at each sampling time at 45 (ο), 50 (Δ), and 55(+) °C. The x-axis of P. oleracea (D) is a factor of 10 greater than other axes.
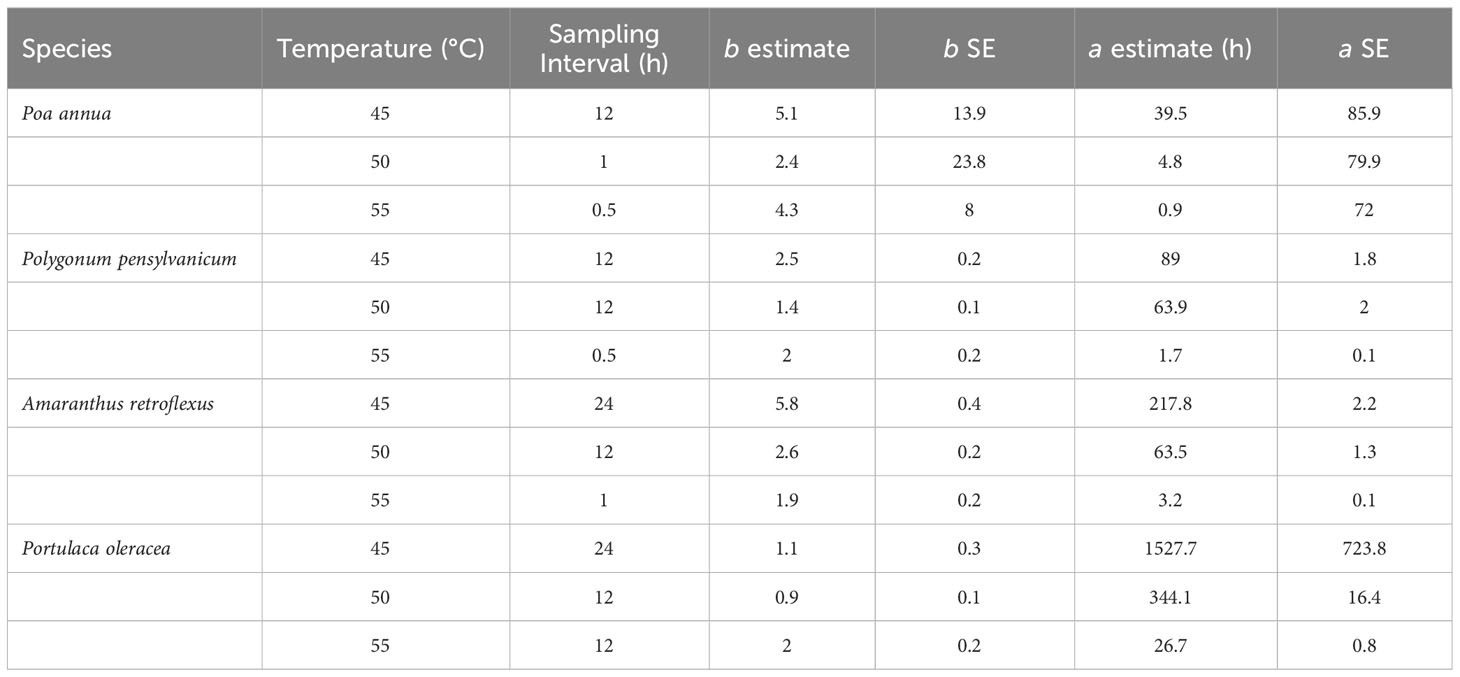
Table 10 The sampling intervals and parameter estimates for each species and temperature in the controlled study.
The T90 differed among species for each temperature treatment. For all species, 95% confidence intervals were smaller at higher temperature treatments. Among the four species, P. annua was most sensitive with a under 10 h at 50°C. Polygonum pensylvanicum and A. retroflexus required 10 h at 55°C for a similar response. Portulaca oleracea was the only species that did not reach 100% mortality in the 45 and 50°C treatments.
The susceptibility of the different weed species varied depending on accumulated thermal hours; however, the rate of mortality for all species increased rapidly at 55°C. The lower the temperature and the greater the thermotolerance of a species, the more variable the effects on seed viability, resulting in wide confidence intervals and large standard errors for the parameter estimates. The thermal dose-response curves described in this study are a simple and conservative form of predicting the susceptibility of weed seeds, which can be applied as fundamental information to develop models to predict effectiveness of soil solarization.
5 Discussion
Solarized soils accumulated 140 hours of temperatures >45°C at the 5 cm depth across all locations and both years. Based on laboratory results, only P. annua and P. pensylvanicum had estimated alpha values below 140 hours for 90% mortality at 45°C. Amaranthus retroflexus and P. oleracea had an estimated accumulation of 218 and >3000 hours at 45°C for 90% mortality, respectively. The effect of soil solarization on weed seeds was species dependent and the field studies were consistent with the laboratory studies with P. annua and P. pensylvanicum having the greatest percent seed mortality followed by A. retroflexus and variable results for P. oleracea and C. esculentus.
Naturally occurring populations of P. annua emergence were reduced by 97 – 100% and buried packets had >90% seed mortality after soil solarization. Similar results were achieved with P. annua in other soil solarization studies (Chase et al., 1999; Peachey et al., 2001; Benlioğlu et al., 2005).
Polygonum pensylvanicum was not present in naturally occurring populations at the different locations, however Polygonum persicaria (L.) was present and soil solarization reduced natural population emergence by 97%. Similar results were achieved in buried packets of P. pensylvanicum where 89 – 100% seed mortality occurred from soil solarization. Polygonum pensylvanicum and P. persicaria are summer annuals; however, both species were sensitive to soil solarization.
Naturally occurring A. retroflexus was present at one location with 100% reduction in weed emergence in solarized soil compared to non-solarized. Soil solarization decreased A. retroflexus seed viability in both years and depths in buried seed packets; however, results were inconsistent ranging from 0 to 84% seed mortality. In buried seed packets, there was an increase in percent dormant seed of A. retroflexus compared with the non-solarized treatment. Dormancy increased at both the 5 cm and 10 cm depths with ≥50% of the seeds dormant after solarization at each location. Most viable seeds in the soil seedbank are concentrated in the top 5 cm (Akinola et al., 1998), and for many species, emergence is reduced significantly as the depth increases. The ability to emerge from deeper depths is related to the size of the seeds (Benvenuti et al., 2001; Grundy et al., 2003). Benvenuti et al. (2001) reported 50% of A. retroflexus germinated at 5.4 cm and no seeds germinated deeper than 8 cm. Our results demonstrate a potential long-term soil seed bank survival mechanism for A. retroflexus, even under the higher temperatures that soil solarization produces, and which killed other weed species. Therefore, this study demonstrated that seed viability must be tested with a TZ test to confirm seed mortality.
Portulaca oleracea seed remained viable at higher temperatures compared to the other species in the seed packets. There was a percent reduction in viable seeds in 2016 in solarized compared to non-solarized populations; however, accumulated thermal units were lower in 2016 compared to 2017. Benlioğlu et al. (2005) saw a >95% reduction of P. oleracea after 49 days of soil solarization in Turkey, which also has a Mediterranean climate. However, the average maximum temperature obtained in the study was 47°C, well above the average maximum temperature of 27°C at the three locations in the PNW. Portulaca oleracea weed seed have been recorded to survive soil temperatures greater than 60°C depending on the amount of moisture present and the length of exposure (Egley, 1990).
The thermal dose-response curve estimated the for P. oleracea to be > 3000 h at 45°C, 828 h at 50°C, and 40 h at 55°C. In a similar study, Dahlquist et al. (2007) using a non-linear regression model based on seed germination reported the as 19 h at 50°C and 1 h at 60°C. However, they did not test viability of non-germinated seed. The differences in results suggest the previously reported mortality may include dormant seeds and P. oleracea may be more tolerant to constant temperature treatments than previously reported. High temperatures are one of the factors which can induce secondary dormancy in summer annual seeds (Forcella et al., 2000).
The most compelling evidence that soil solarization can be an effective weed management method in PNW tree nurseries comes from field studies on naturally occurring weed species carried out in two sites over two years. Solarization reduced naturally occurring weed emergence for several months after the solarization film was removed compared with non-solarized plots. There was a 94-96% decrease in weed emergence in solarized plots compared to non-solarized plots during the fall and winter counts at Clackamas in 2016 and 2017. The large effect on fall and winter weeds may reflect a greater number of winter annual weed species present compared to summer annuals and the lower thermotolerance of winter annuals previously discussed. While counts of naturally occurring weeds were overall lower in spring and early summer, weed emergence in solarized plots was still 67-81% lower than non-solarized plots even after 7-9 months. Even during the summer following solarization, solarized plots required less time to hand weed. Hand weeding is one of the major costs in nursery production, and the current shortage of nursery workers can further exacerbate timely weed management. Hand weeding time in these studies was reduced by 50 to 69% in solarized plots. The reduction in hand weeding enables nurseries to save labor costs and/or redirect workers to tasks that do not require as much physical labor (personal communication).
In addition, with the reduced need for hand weeding there is less soil disturbance that results in fewer viable weed seed being brought closer to the soil surface and subsequently reduces to weed emergence.
6 Conclusion
These studies demonstrate that soil solarization can be an effective nonchemical weed management technique for PNW tree nurseries, even though it is not lethal to all weed species. A reduction in naturally occurring weed emergence of > 94% rivals the effectiveness of chemical herbicides (Chauhan and Abugho, 2012) without the potential confounding result of herbicide damage, the development of resistance and chemical drift (Case et al., 2005). We observed more consistent results across years in the Oregon sites than the more northerly Washington site. Solarization appears to work best on weed species intolerant of high temperatures and on seeds incapable of emerging from deeper soil depths that are not sufficiently heated by solarization. Solarization was not effective in preventing emergence of C. esculentus, a species that emerges from deeply buried, hardy tubers. Although this species was found only at the Washington site, the presence of this pernicious weed in forest nurseries previously justified the use of methyl bromide fumigation to prevent its dispersal. Solarization is not reliable enough to manage this species, but our studies show that solarization is effective against many of the weed species encountered in commercial PNW nurseries, expanding the geographic zones previously considered suitable for soil solarization (Katan and Gamliel, 2009).
Soil solarization is not without limitations. It requires the use of plastic, which is costly and represents a potential disposal hazard. In Oregon, the solarization film has been picked up and recycled for manufacturing agricultural plastics, but the market for recycled plastic is unpredictable. Nurseries must also invest in specialized equipment for laying the plastic. The Clackamas nursery included in these studies continues to solarize their seedling fields both as a cost- and labor-saving strategy, but also to reduce their weed seedbank for the long term (grower personal communication).
Fall sown crops enable solarization to occur during warmer summer months for greater accumulated thermal hours and greater weed seed mortality. This timing restricts the use of soil solarization for many cropping systems; however, there are potential opportunities to utilize solarization to manage weeds in a fallow system or a transition from conventional to organic production with limited weed control options (Mallory-Smith et al, 2019; Parke et al., 2018). In addition to weed management, solarization has been shown to kill plant pathogens (Funahashi and Parke, 2016; Funahashi and Parke, 2018). Solarization could be used as part of a management program where soil fumigation is not feasible in managing both weeds and plant pathogens due to pesticide application buffer restrictions.
Soil solarization can be a viable option for managing weeds in PNW tree nurseries. A preliminary online soil solarization program (Online Soil Solarization Program, 2019) has been established to help PNW growers determine the feasibility of achieving soil temperatures sufficient to kill certain soilborne pests. Growers can select their target pathogen or weed species, nearest weather station, prior year to use for forecasting weather, soil solarization start and end date, and lower boundary soil temperature (temperature at 50 cm depth), then run the model to determine the soil depth at which a lethal temperature will be achieved, and how long it will take. Application of the predictive model will reveal new opportunities for solarization in horticultural and forest nurseries in the PNW but also point out limitations in applying solarization for managing certain heat-resistant weed species, especially at northerly locations.
Data availability statement
The original contributions presented in the study are included in the article/Supplementary Material. The full data set for soil temperature is available at ScholarsArchive@OSU (https://ir.library.oregonstate.edu/?locale=en). Further inquiries can be directed to the corresponding author.
Author contributions
NW: Data curation, Formal analysis, Investigation, Methodology, Validation, Writing – original draft. PB: Data curation, Writing – review & editing. BH: Data curation, Investigation, Methodology, Writing – review & editing. CM-S: Conceptualization, Funding acquisition, Formal analysis, Investigation, Methodology, Project administration, Resources, Supervision, Validation, Visualization, Writing – review & editing. JP: Conceptualization, Data curation, Formal analysis, Funding acquisition, Investigation, Methodology, Project administration, Resources, Supervision, Validation, Visualization, Writing – review & editing.
Funding
The author(s) declare financial support was received for the research, authorship, and/or publication of this article. The publication was supported by funding from the Sustainable Agriculture Research and Education Project SW16-070 and the Western Integrated Pest Management Center.
Acknowledgments
We thank the three commercial nurseries for their help and cooperation in carrying out the field experiments. In particular, we wish to acknowledge Sam Doane of J. Frank Schmidt & Son Co. nursery for his professional expertise and insights that contributed so greatly to the design and quality of the field studies.
Conflict of interest
The authors declare that the research was conducted in the absence of any commercial or financial relationships that could be construed as a potential conflict of interest.
Publisher’s note
All claims expressed in this article are solely those of the authors and do not necessarily represent those of their affiliated organizations, or those of the publisher, the editors and the reviewers. Any product that may be evaluated in this article, or claim that may be made by its manufacturer, is not guaranteed or endorsed by the publisher.
Supplementary material
The Supplementary Material for this article can be found online at: https://www.frontiersin.org/articles/10.3389/fagro.2023.1321372/full#supplementary-material
References
Al-Hammadi F. (2006). Effects of soil solarizaiton on seed viability of serious weeds of crops in the UAE. [master’s thesis]. [United Arab Emirates University]: UAE.
Akinola M., Thompson K., Buckland S. (1998). Soil seed bank of an upland calcareous grassland after 6 years of climate and management manipulations. J. App. Ecol. 35, 544–552. doi: 10.1046/j.1365-2664.1998.3540544.x
Baskin C., Baskin J. (1998). Seeds, Ecology, Biogeography, and Evolution of Dormancy and Germination (San Diego: Academic Press).
Benlioğlu S., Boz Ö., Yildiz A., Kaşkavalci G., Benlioğlu K. (2005). Alternative soil solarization treatments for the control of soil-borne diseases and weeds of strawberry in the Western Anatolia of Turkey. J. Phyto 153, 423–430.
Benvenuti S., MacChia M., Miele S. (2001). Quantitative analysis of emergence of seedlings from buried weed seeds with increasing soil depth. Weed Sci. 49, 528–535. doi: 10.1614/0043-1745(2001)049[0528:QAOEOS]2.0.CO;2
Case L., Mathers H., Senesac A. (2005). review of weed control practices in container nurseries. Hortic. Tech. 15, 535–545. doi: 10.21273/HORTTECH.15.3.0535
Chase C. (1999). Effects of temperature and tuber depth on Cyperus spp. control. Weed Sci. 47, 467–535. doi: 10.1017/S0043174500092092
Chase C., Sinclair T., Chellemi D., Olson S., Gilreath J., Locascio S. (1999). Heat-retentive films for increasing soil temperatures during solarization in a humid, cloudy environment. Hort Sci. 34, 1085–1089. doi: 10.21273/HORTSCI.34.6.1085
Chauhan B., Abugho S. (2012). Effect of growth state on the efficacy of postemergence herbicides on four weed species of direct-seeded rice. Scien. Wor. Jour. 2012, 123071.
Cohen O., Rubin B. (2007). “Soil Solarization and Weed Management,” in Non-chemical Weed Management: Principles, Concepts, and Technology. Eds. Upadhyaya M. K., Blackshaw R. E. (Cambridge, MA: CAB International), 177–200.
Dahlquist R., Prather T., Stapleton J. (2007). Time and temperature requirements for weed seed thermal death. Weed Sci. 55, 619–625. doi: 10.1614/WS-04-178.1
Egley G. (1990). High-temperature effects on germination and survival of weed seeds in soil. Weed Sci. 38, 429–435. doi: 10.1017/S0043174500056794
Elmore C. (1991). “Use of Solarization for Weed Control,” in Soil Solarization, Plant Production and Protection Paper. Ed. Elmore C. (Rome, Italy: FAO Corporate Document Repository), 129–138.
Elmore C., Stapleton J., Bell C., DeVay J. (1997). Soil solarization: a non-pesticidal method for controlling, diseases, nematodes, and weeds (Oakland: University of California Agriculture Natural Resource Publication), 21377.
EPA (2012) Soil Fumigation Regulations for 2012. Available at: https://www.oregon.gov/ODA/shared/Documents/Publications/PesticidesPARC/SoilFumigationRegs.pdf (Accessed 7/25/2023).
Forcella F., Benech A., Sanchez R., Ghersa C. (2000). Modeling seedling emergence. Field Crops Res. 67, 123–139. doi: 10.1016/S0378-4290(00)00088-5
Funahashi F., Myrold D., Parke J. (2021). The effects of soil solarization and application of a Trichoderma biocontrol agent on soil fungal and prokaryotic communities. Soil Sci. Soc Am. J. 86, 369–383. doi: 10.1002/saj2.20361
Funahashi F., Parke J. (2016). Effects of soil solarization and Trichoderma asperellum on soilborne inoculum of Phytophthora ramorum and Phytophthora pini in container nurseries. Plant Dis. 100, 438–443. doi: 10.1094/PDIS-04-15-0453-RE
Funahashi F., Parke J. (2018). Thermal inactivation of inoculum of two Phytophthora species by intermittent vs. constant heat. Phytopath. 108, 829–836. doi: 10.1094/PHYTO-06-17-0205-R
Funahashi F., Parke J. (2020). Soil solarization to eradicate soilborne Phytophthora spp. in container nurseries with surface gravel. J. Environ. Hortic. 38, 158–167. doi: 10.24266/0738-2898-38.4.158
Grundy C., Mead A., Burston S. (2003). Modelling the emergence response of weed seeds to burial depth: interactions with seed density, weight and shape. J. App. Ecol. 40, 757–770. doi: 10.1046/j.1365-2664.2003.00836.x
Gullino M., Garibaldi A., Gamliel A., Katan J. (2022). Soil disinfestation: from soil treatment to soil and plant health. Plant Dis. 106, 1541–1554. doi: 10.1094/PDIS-09-21-2023-FE
Hill B. C. (2019). Solarization in the Pacific Northwest: a mathematical field model for accumulation of linearized mortality from variable intermittent heat. [Master's thesis] Oregon State University.
Horowitz M., Regev Y., Herzlinger G. (1983). Solarization for weed control. Weed Sci. 31, 170–179. doi: 10.1017/S0043174500068788
Hoyle A., Mcelroy S. (2009). Relationship between temperature and heat duration on large crabgrass (Digitaria sanguinalis), Virginia buttonweed (Diodia virginiana), and cock’s-comb kyllinga (Kyllinga squamulata) seed mortality. Weed Tech. 26, 800–806. doi: 10.1614/WT-D-11-00160.1
Katan J., DeVay J. (1991). “Soil Solarization: Historical Perspectives, Principles and Uses,” in Soil Solarization. Eds. Katan J., DeVay J. E. (Boca Raton, FL: CRC Press), 23–37.
Katan J., Gamliel A. (2009). “Soil Solarization – “30 Years On: What Lessons Have Been Learned?,” in Recent Developments in Management of Plant Diseases. Plant Pathology in the 21st Century, vol. 1 . Eds. Gisi U., Chet I., Gullino M. (Dordrecht: Springer). doi: 10.1007/978-1-4020-8804-9_19
Londale W. M. (1993). Losses from the seedbank Mimosa pigra: soil microoganisms vs. temperature fluctuations. J. Appl. Ecol. 59, 84–89.
Maher B., Oldfield F., Thopmson R. (1986). Characterization of soils by mineral magnetic measurements. Phys. Earth planet. interiors. 42, 76–92. doi: 10.1016/S0031-9201(86)80010-3
Mallory-Smith C., Wada N., Parke J. (2019). Here comes the sun: soil solarization for weed management. In: Digger Magazine. Available at: http://www.diggermagazine.com/here-comes-the-sun/ (Accessed Oct. 11, 2023).
Miles J., Nishimoto R., Kawabata O. (1996). Diurnally alternating temperatures stimulate sprouting of purple nutsedge (Cyperus rotundus) tubers. Weed Sci. 44, 122–125. doi: 10.1017/S0043174500093644
ODA (2022) Oregon agriculture facts and figures. Available at: https://www.oregon.gov/oda/shared/documents/publications/administration/agstatsdirectory.pdf (Accessed 7/27/2023).
Online Soil Solarization Program (2019). Available at: https://uspest.org/soil/solarizeV2beta1 (Accessed Nov. 27, 2023).
Parke J. (2016) Soil solarization in container nurseries and field production. Available at: https://diggermagazine.com/soil-solarization-in-container-nurseries-and-field-production/ (Accessed 7/28/2020).
Parke J., Mallory-Smith C., Dragila M., Hill B., Wada N., Weidman C., et al. (2018). Soil solarization – a potential tool for organic growers to manage weeds and improve soil health. Org. Farmer 1, 12–18.
Patil N., Dadlani M. (2009). “Tetrazolium Test for Seed Viability and Vigor,” in Flowers Seeds: Biology and Technology Handbook, vol. 33. Eds. McDonald M. B., Kwon F. Y. (Oxfordshire, UK: CABI Pub).
Peachey E., Pinkerton J., Ivors K., Miller M., Moore L. (2001). Effect of soil solarization, cover crops, and metham on field emergence and survival of buried Annual bluegrass (Poa annua) seeds. Weed Tech. 15, 81–88. doi: 10.1614/0890-037X(2001)015[0081:EOSSCC]2.0.CO;2
Pike C., Haase D., Enebak S., Abrahams A., Bowersock E., Mackey L., et al. (2023). Forest nursery seedling production in the United States - fiscal Year 2022. Tree Planters’ Notes 66, 73–80.
Pinkerton J., Ivors K., Miller M., Moore L. (2000). Effect of soil solarization and cover crops on populations of selected soilborne plant pathogens in western Oregon. Plant Dis 84 (9), 952–960. doi: 10.1094/PDIS.2000.84.9.952
Rubin B., Benjamin A. (1984). Solar heating of the soil: effect on weed control and on soil-incorporated herbicides. Weed Sci. 31, 819–825. doi: 10.1017/S0043174500070806
Standifer L., Wilson P., Porche-Sorbet R. (1984). Effects of solarization of soil weed seed populations. Weed Sci. 28, 457. doi: 10.1017/S0043174500059580
Stapleton J. (1990). “Thermal Inactivation of Crop Pests and Pathogens and Other Soil Changes Caused by Solarization,” in Soil Solarization, Plant Production and Protection Paper. Ed. Elmore C. (Rome, Italy: FAO Corporate Document Repository), 109.
Stapleton J. (2000). Soil solarization in various agricultural production systems. Crop Pro. 19, 837. doi: 10.1016/S0261-2194(00)00111-3
Stapleton J., DeVay J. (1986). Soil solarization: a non-chemical approach for management of plant pathogens and pests. Crop Prot. 5, 190–198. doi: 10.1016/0261-2194(86)90101-8
Stapleton J., Molinar R., Lynn-Paterson K., McFeeters S., Shrestha A. (2005). Soil solarization provides weed control for limited-resource and organic growers in warmer climates. Cal. Ag. 59, 84–89. doi: 10.3733/ca.v059n02p84
USDA (2014) 2014 Census of horticultural specialties – Oregon. Available at: https://www.nass.usda.gov/Statistics_by_State/Oregon/Publications/Horticulture/OR_census_hort.pdf (Accessed February 21, 2018).
Wada N. (2019). Soil solarization for weed management in tree seedling nurseries in the pacific northwest. [Master's thesis] Oregon State University.
Webster T. (2003). High temperatures and durations of exposure reduce nutsedge (Cyperus spp.) tuber viability. Weed Sci. 51, 1010. doi: 10.1614/WS-03-018R
Keywords: seed viability, weed seedbank, heat unit accumulation, hand weeding reduction, integrated weed management
Citation: Wada N, Berry PA, Hill B, Mallory-Smith C and Parke JL (2024) Soil solarization as a non-chemical weed control method in tree nursery production systems of the Pacific Northwest, USA. Front. Agron. 5:1321372. doi: 10.3389/fagro.2023.1321372
Received: 14 October 2023; Accepted: 19 December 2023;
Published: 25 January 2024.
Edited by:
Euro Pannacci, University of Perugia, ItalyReviewed by:
Isabel Calha, National Institute for Agricultural and Veterinary Research (INIAV), PortugalPatricia Andrea Monquero, Federal University of São Carlos, Brazil
Copyright © 2024 Wada, Berry, Hill, Mallory-Smith and Parke. This is an open-access article distributed under the terms of the Creative Commons Attribution License (CC BY). The use, distribution or reproduction in other forums is permitted, provided the original author(s) and the copyright owner(s) are credited and that the original publication in this journal is cited, in accordance with accepted academic practice. No use, distribution or reproduction is permitted which does not comply with these terms.
*Correspondence: Pete A. Berry, Pete.berry@oregonstate.edu