Laser weed seed control: challenges and opportunities
- Department of Plant and Environmental Sciences, Faculty of Science, University of Copenhagen, Taastrup, Denmark
Farmers are greatly interested in reducing weed seeds in their fields to avoid unnecessary weed infestation. Autonomous vehicles equipped with plant recognition systems and lasers can be used to control weed plants and may therefore be used to replace or supplement herbicides and mechanical weed control. However, less is known about the ability of laser to control weed seeds. In this study, seeds of weeds (Alopecurus myosuroides, Anisantha sterilis, Avena fatua, Centaurea cyanus, Silene noctiflora) and crops (wheat (Triticum aestivum), maize (Zea mays)) were exposed to increasing dosages of laser energy. The species represented dicots and monocots with different seed sizes and morphology. We used a thulium-doped 50 W fiber laser with a wavelength of 2 µm and a diameter of 2 mm developed for weed control. The seeds were exposed to laser directly on the seed surface or after been covered with soil (2.5 and 5 mm). Small doses of energy (0.4 J mm−2 and 0.8 J mm−2) affected the germination ability of small seeds (S. noctiflora and C. cyanus) when the seeds were irradiated directly on the seed surface, and they were completely burned at the high doses (8.0 J mm−2 and 15.9 J mm−2). However, there was not a clear relationship between seed size and sensitivity to laser dose. Additionally, increasing the laser dose on the seed surface resulted in increasing infection of fungi. Seeds covered with soil were not affected by the laser treatments except C. cyanus seeds. Controlling large seeds on the ground while weed seedlings are controlled with laser robots seems realistic in the future. However, dosages higher than 50 J (~15.9 J mm−2) is necessary to control large seeds.
1 Introduction
The pesticide directives in Europe provide a framework for action for promoting the adoption of low pesticide input approaches, particularly integrated pest management (IPM) (EU, 2009; Lefebvre et al., 2015). Integrated weed management (IWM) means integrating multiple weed control methods into a single weed management program.
Due to the challenges posed by conventional weed control, there are currently several research and commercial projects underway to develop autonomous vehicles equipped with lasers to control weed plants in field crops (e.g., https://carbonrobotocs.com; https://weedbot.eu; https://welaser-project.eu).
Recognition systems based on artificial intelligence have been developed to locate and identify small weed seedlings (Rakhmatulin et al., 2021), and mirrors are used to direct laser beams toward the target to harm or kill the weed plants with heat (Emmi et al., 2023). Laser weeding seems promising as a tool to replace or supplement herbicides and mechanical weed control (Coleman et al., 2021). A laser beam only affects the weed plants while conventional weed control affects almost the whole cropping area. In contrast to herbicide application, laser weeding leaves behind only the ash from the burned plants which may serve as fertilizer for the crop (Andreasen et al., 2022). If a laser beam accidentally hits non-target organisms on the plant or on the ground, like pests and beneficial insects, they will also be harmed or killed depending on the applied laser energy dose (Mullen et al., 2016; Gaetani et al., 2021; Andreasen et al., 2023). However, as only a tiny field area will be exposed to the laser beam, the probability of hitting non-target organisms is very small. In general, European stakeholders seems to have a positive attitude to autonomous laser-based weeding systems because this solution addresses the challenges posed by labor shortages and the negative environmental impact of conventional weed control (reduced biodiversity, soil disturbance and compaction, and CO2 emissions) (Tran et al., 2023).
Recently there has been increasing interest in IWM strategies to avoid unnecessary input to the soil weed seed bank to reduce weed infestation in the following growing seasons. This can be done by increasing seed predation in the field or using harvest weed seed control (HWSC). Weed seed predators have been suggested to increased weed seed mortality in agricultural ecosystems, including pre-dispersal and post-dispersal of predators as biological control agents (Navntoft et al., 2009; Baraibar et al., 2011; Sarabi, 2019). HWSC systems have been developed to collect and/or kill weed seeds at harvest, for example, by collecting chaff in carts, or using weed seed destructors (Walsh et al., 2012, 2018). There seems to be a great potential to reduce weed seed input to the soil seed bank in European fields using such methods (Bitarafan and Andreasen, 2020a, 2020b; Akhter et al., 2023).
Farmers have also addressed whether lasers could be used to kill weed seeds on the ground and in the soil. Recognition systems based on visual images, multispectral imaging, and deep learning for seed identification are currently being developed (e.g., Arefi et al., 2011; Olesen et al., 2014; Shrestha et al., 2015; Luo et al., 2023), and commercial products have been marketed to the seed industry to identify and count the number of seeds of different plant species and deviant crop seeds in purity tests in laboratories (see e.g., https://videometer.com/videometer-seedlab). Similarly, artificial intelligence can be used to develop seed recognition systems to find weed seeds on the soil surface in the field, and a laser beam can potentially harm or destroy the seeds.
We used a 50 W thulium-doped fiber laser with a wavelength of 2 µm and a diameter of 2 mm to describe the dose-response relationship between laser energy application (up to 15.9 J mm−2) and the germination ability of seeds of different weed species with different morphology and biomass. The laser was developed to control weed seedlings in fields by an autonomous vehicle (Emmi et al., 2023). The fiber laser is considered more beneficial for weed control than a CO2 laser, in which energy is primarily absorbed on the surface of the plant (Wieliczka et al., 1989). The water inside the target cells mainly absorbs the 2 µm wavelength given by the fiber laser. Therefore, a thulium-doped fiber laser with a wavelength of 2 µm has been installed in the autonomous vehicle for laser weeding developed in the EU project WeLASER (https://welaser-project.eu/). Weed plants on the cotyledons and two permanent leaf stages are usually killed when they are exposed to about 20 J mm−2 (Heisel et al., 2002; Andreasen et al., 2022). The aim was to investigate how increasing laser dose affected the germination of different common weed and crop species on the soil surface. We also studied whether the treatments could affect seeds in the uppermost soil layer.
2 Materials and methods
2.1 Laser equipment
We used a thulium-doped 50 W fiber laser with a collimated beam (Ø: 2 mm) with a wavelength of 2 µm manufactured by Futonics Laser GmbH, Katlenburg-Lindau, Germany (Figure 1A). The laser was placed within a steel box (68 cm × 68 cm × 68 cm) with a door with a metal interlock (Andreasen et al., 2023). On laser activation, the door locked automatically to avoid the risk of laser exposure.
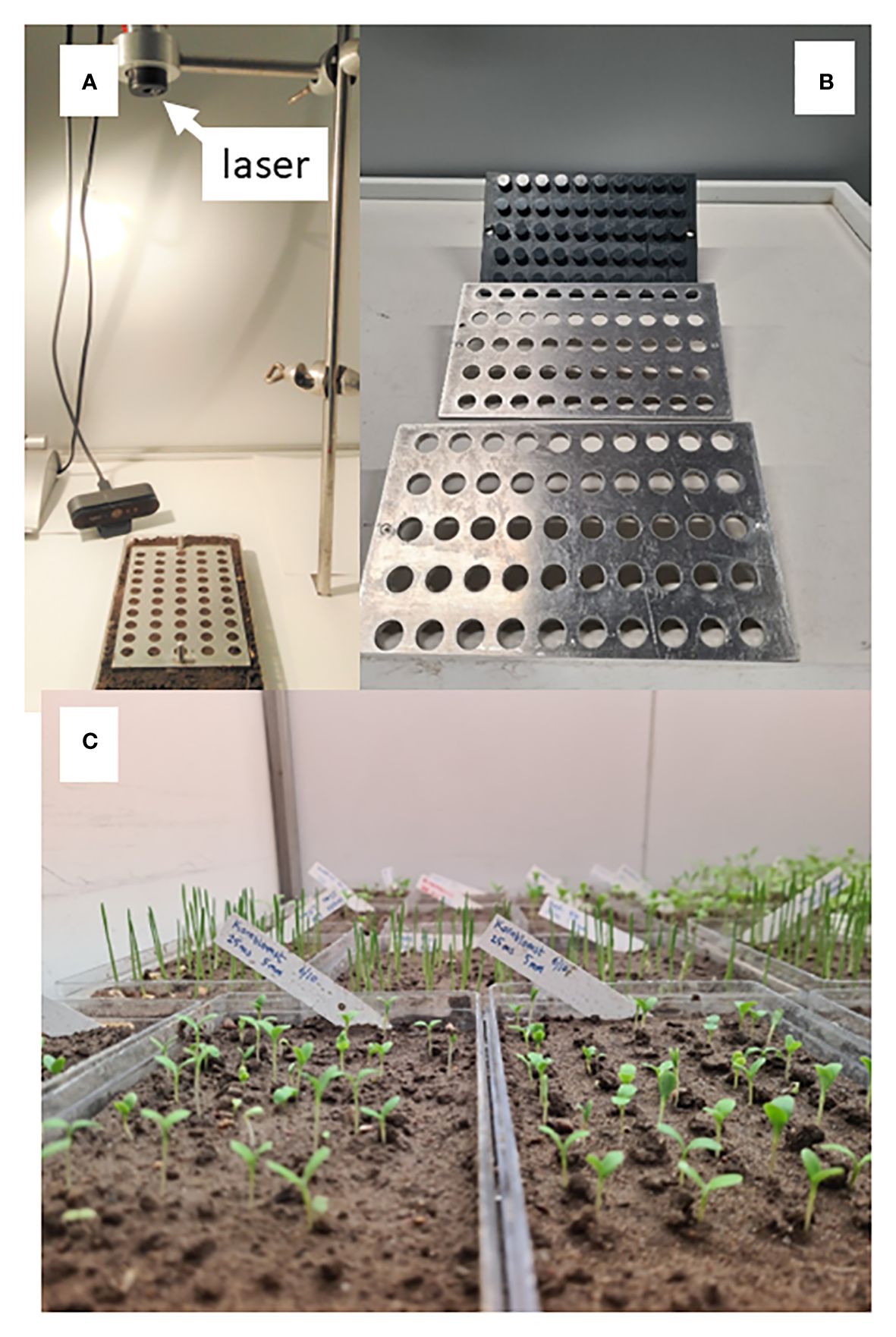
Figure 1 (A) Laser set up: Laser head pointing directly toward the seeds covered with soil in the germination box. (B) Metal frames (thickness: 2.5 and 5 mm) with holes for 50 seeds. (C) Germination boxes with emerging Centaurea cyanus seedlings.
The seeds were placed approximately 40 cm below the laser head and exposed to increasing dosages of laser energy up to a level of 15.9 J mm−2. The highest dose may be used to control seedlings of weeds in agricultural and horticultural fields.
2.2 Plant species
Two dicot and five monocot species with different weights and morphology were chosen for the experiment (Figure 2, Table 1). Alopecurus myosuroides Huds. was chosen because it is a grass weed with relatively small seeds (caryopsis). It poses increasing problems in annual Northern European winter crops like winter wheat and winter barley (Menchari et al., 2007). Herbicide-resistant biotypes are common (Heap, 2023). Anisantha sterilis (L.) Nevski was chosen because it is a grass species with long narrow seeds. It is mainly a problem in field margins and in conservation agriculture characterized by reduced mechanical soil disturbance (Clarke et al., 2000). Avena fatua L. is a noxious grass weed with large seeds (Beckie et al., 2012). In Denmark, farmers are obliged to control the weed according to the law (Anonymous, 2020). Centaurea cyanus L. is a dicot and has a special seed looking like a little brush. It occurs widely in European agricultural fields but is increasingly rare, with acetolactate synthase inhibitors-resistant biotypes only listed in Poland (Stankiewicz-Kosyl et al., 2020). Silene noctiflora L. is a dicot and was chosen because of the small seed size. The species is relatively common in summer annual crops (Andreasen and Stryhn, 2012). All weed seeds were produced from plants collected in fields belonging to the University of Copenhagen, Højbakkegaard, Taastrup (55° 38’ N, 12° 17’ E), Denmark. The two crop species, wheat (Triticum aestivum L. cv. Complet) and corn (Zea mays L.cv. Augustus KWS), were chosen because their seeds have a large biomass compared to the weed seeds. Wheat often occurs as a volunteer in the following crop (Anderson and Soper, 2002).
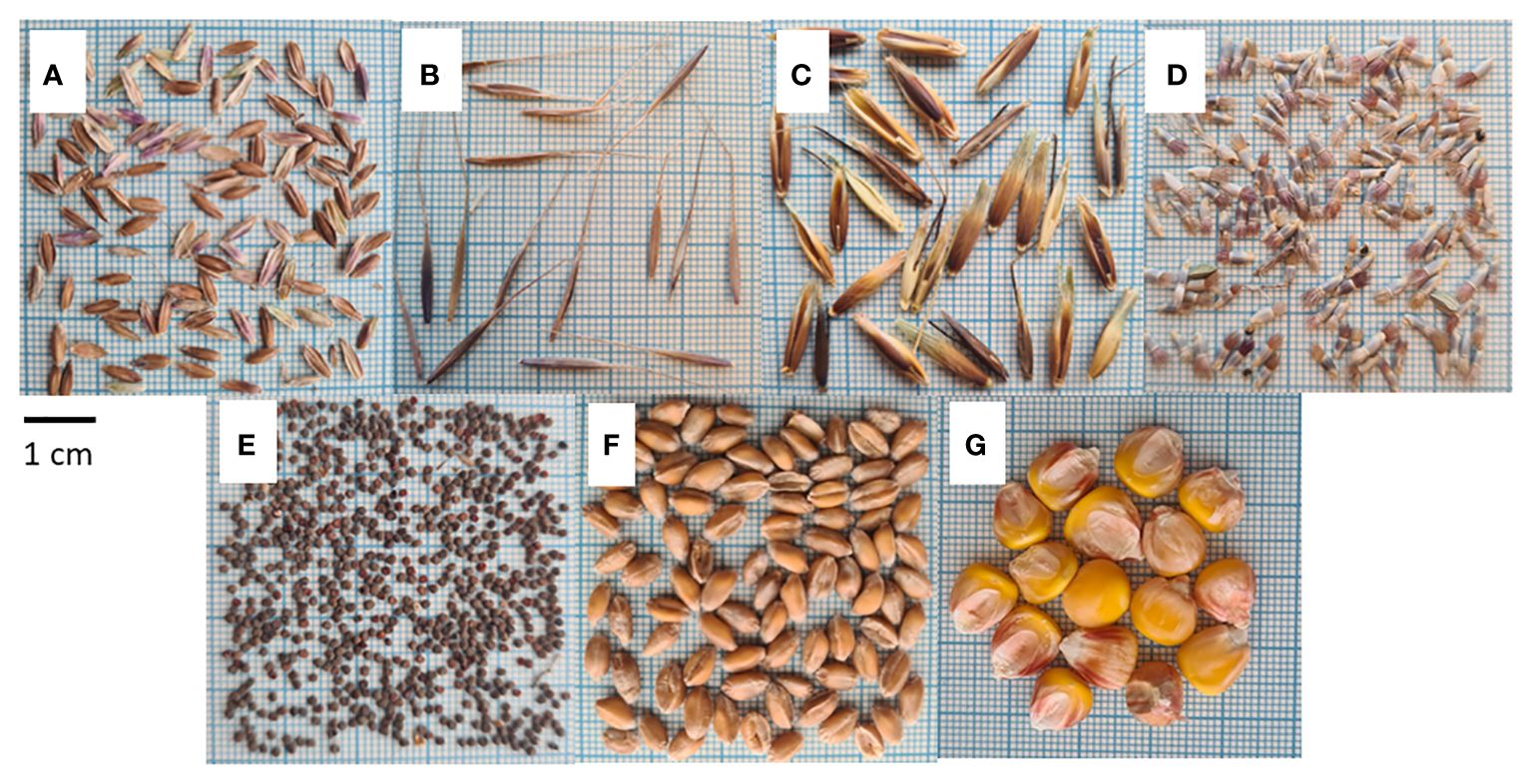
Figure 2 Seeds exposed to laser irradiation: (A) Alopecurus myosuroides (caryopsis) (B) Anisantha sterilis (caryopsis), (C) Avena fatua (caryopsis), (D) Centaurea cyanus (achene), (E) Silene noctiflora (achene), (F) wheat (Triticum aestivum) (caryopsis), (G) corn (Zea mays).
2.3 Irradiation of seeds
Three experiments were conducted with dry seeds. In experiment 1, single seeds were placed below the laser head on a piece of paper and irradiated directly on the seed surface in the middle of the seed. Seeds were irradiated on the dorsal, ventral, or in any other random natural position. Four replicates of fifty seeds of each plant species were irradiated with one of six laser dosages (0, 1.25, 2.5, 5, 25, and 50 J, corresponding to 0, 0.4, 0.8, 1.6, 8.0, and 15.9 J mm−2). Afterwards, the seed was moved to a germination plate with filter paper placed in a transparent germination box (11. 5 cm × 7.5 cm × 5 cm) with a plastic lid. The filter paper was kept continuously moist by a wick, extending down into an underlying water bath. Seeds were spaced uniformly and adequately apart on the filter paper. This setup ensured appropriate oxygen and water availability during the experiment (ISTA, 2011). The experiment was done twice (50 seeds × 4 replicates × 6 doses × 6 species × 2 experiments). The general recommendations concerning germination tests were followed (ISTA, 2011). After the treatment, the boxes were placed in a germination chamber at 20°C ± 2°C with 12 hours of light and 12 hours of darkness. The germination was recorded after ten days. The seeds were considered germinated when a sprout of a minimum 2 mm was observed from the seed.
In experiments 2 and 3, 50 seeds were covered with soil before irradiation. Four transparent boxes (21 cm × 11 cm × 3.5 cm) with 50 seeds were used for each of the same laser doses as in experiment 1. Each seed was placed in the middle of a hole in a metal frame with 50 holes and with a thickness of 2.5 or 5 mm (Figure 1B). The frame was placed on the surface of moist sandy soil containing 9% clay, 10% silt, 32% fine sand, 47% coarse sand, and 2% organic matter. Like in experiment 1, the seeds were placed randomly in the middle of the hole, so the upper surface of the seed was on the same level as the soil surface. The seeds laid on the dorsal, ventral, or in any other random natural position (Figure 2). Hereafter, the seeds were covered with 2.5 mm (Exp. 2) or 5 mm (Exp. 3) unsterilized soil before irradiation, corresponding to the thickness of the frame (Figure 1B). We aimed to hit the soil with the laser beam in the middle of the holes. The soil was exposed to the laser treatment immediately after the seed was covered with soil leaving very few minutes for the seeds to take up water from the soil before the treatment. For each laser dose, there were four replicates in each experiment. The experiments were repeated (50 seeds × 4 replicates × 6 doses × 2 seeding depth × 2 experiments). The doses that were used were the same as in experiment 1. After the treatment, the frame was removed, and the boxes were placed under the same conditions as in Experiment 1 (Figure 1C). The germination percentage was estimated after 10 days. Emerged plants were identified and recorded. Natural incidence of germinating weed seeds of other plant species in the soil were removed daily. All experiments were kept moist during the experimental period to ensure good germination conditions.
2.4 Statistical analyses
All data sets, defined by repetition and sowing depth, were individually analyzed using a dose-response model assuming a binomial distribution of the germination data. Different dose-response models were used according to best fit as judged by AIC (Akaikes Information Criteria). Overview of the used models is shown in Table 2. Estimated parameter values for all repetitions were combined in a meta-analytic linear mixed model (Jensen et al., 2020) with parameter as fixed effect and experiment as random effect and an unstructured variance-covariance matrix. The effective doses, ED10, ED50, and ED90, resulting in a 10, 50, or 90 percentage reduction in the germination percentage, respectively, was estimated from the combined model.
To estimate the mean germination percentage if no dose-response trend was present, data were analyzed using a logistic regression model with intercept only.
The germination percentage from the highest laser doses were estimated using a logistic regression model and germination percentages were compared between depths within species by post hoc pairwise comparisons. All analyses were done with the statistical software R version 4.2.0 (R Core Team, 2022), and specifically the extension package drc (Ritz et al., 2019) for dose-response modeling, metafor for meta-analytic models (Viechtbauer, 2010), lme4 for logistic mixed models (Bates et al., 2015), and multcomp (Hothorn et al., 2008) for post hoc pairwise comparisons.
3 Results
The effect of the increasing laser energy doses on the germination of the plant species are shown in Figures 3–9. Estimated parameters are shown in Supplementary Table 1 in the Supplementary Material. When dry A. myosuroides seeds were irradiated on the soil surface with increasing energy doses, the germination ability of the seed population decreased. The highest dose (15.9 J mm−2) reduced the germination percentage to about 50% (Figure 3). Less seeds germinated within the 10 days in the control groups when the seed samples were covered with soil. When A. myosuroides seeds were covered with 2.5 or 5 mm soil, there was no effect of the irradiation.
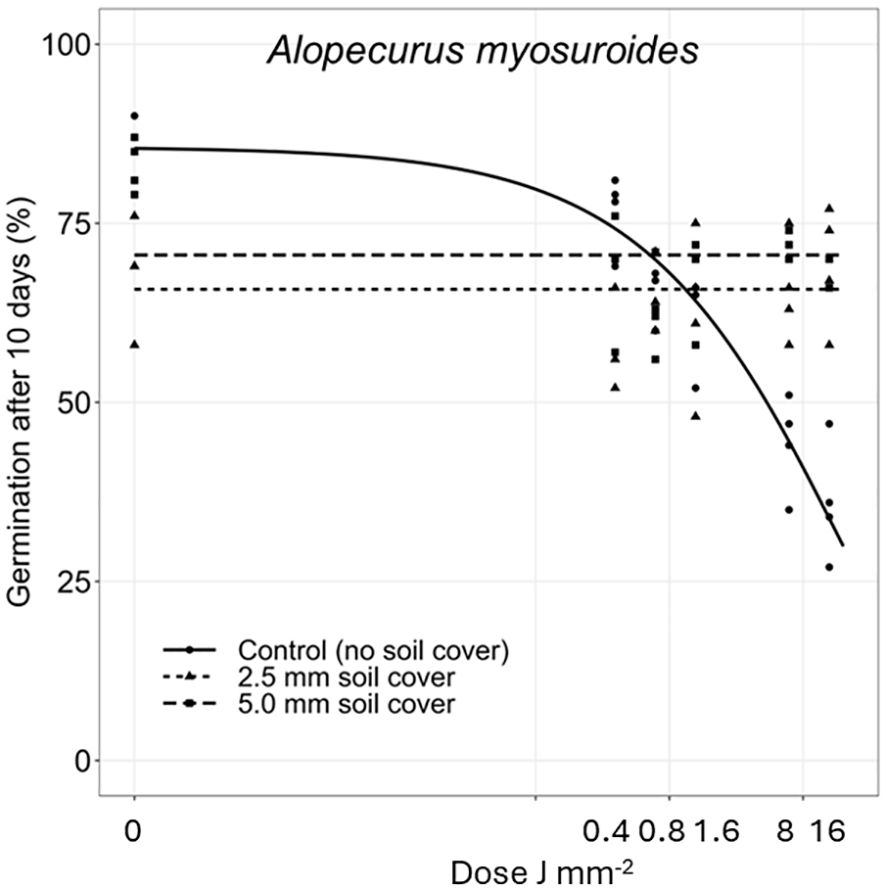
Figure 3 Dose-response experiments with Alopecurus myosuroides. Seeds were treated directly on the seed surface, and thereafter covered with 2.5 or 5 mm of soil.
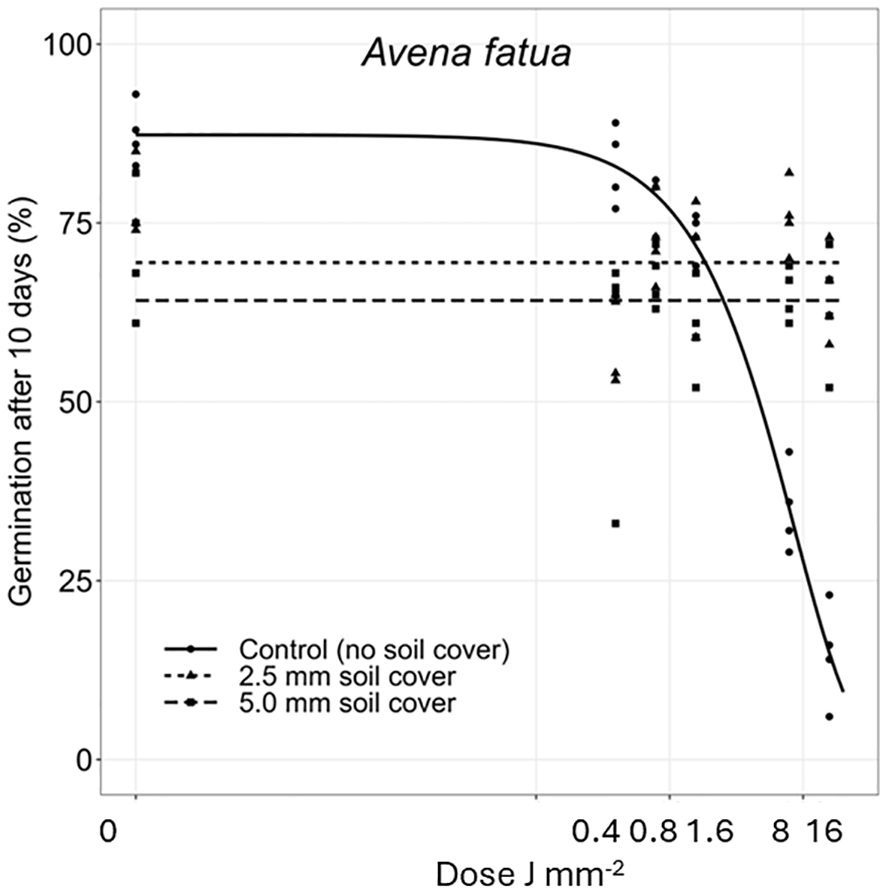
Figure 4 Dose-response experiments with Avena fatua. Seeds were treated directly on the seed surface, and thereafter covered with 2.5 or 5 mm of soil.
The deeper the seeds were buried the longer time it took to penetrate the soil layer and emerge. Therefore, the germination percentages did not reach the same level within the 10 days as for the uncovered seeds. Avena fatua and S. noctiflora seeds showed the same tendency as A. myosuroides (Figures 4, 5). Soil covered Centaurea cyanus seeds were slightly affected by the largest laser dosages (Figure 6). Silene noctiflora and C. cyanus having the smallest seeds, were completely burned at the highest doses (8.0 and 15.9 J mm−2) when they were uncovered. Even at a low dose (0.40 mm J−2), the germination ability of S. noctiflora was decreased. Generally, the larger the seed biomass, the less affected was the germination percentage of the treatment. The germination percentage of A. sterilis seeds covered with soil was not affected by the laser dosages (Figure 7). The same was the case for wheat and maize seeds although there seemed to be a weak decrease in the germination percentage at the highest dose (15.9 J mm−2) (Figures 8, 9).
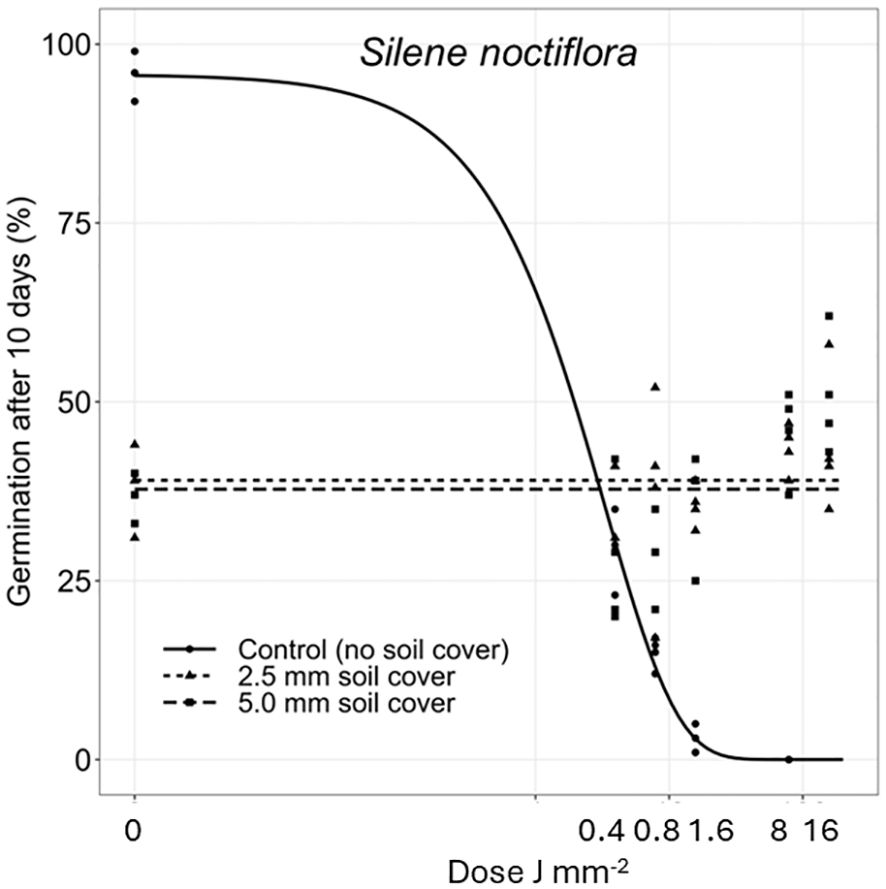
Figure 5 Dose-response experiments with Silene noctiflora. Seeds were treated directly on the seed surface, and thereafter covered with 2.5 or 5 mm of soil.
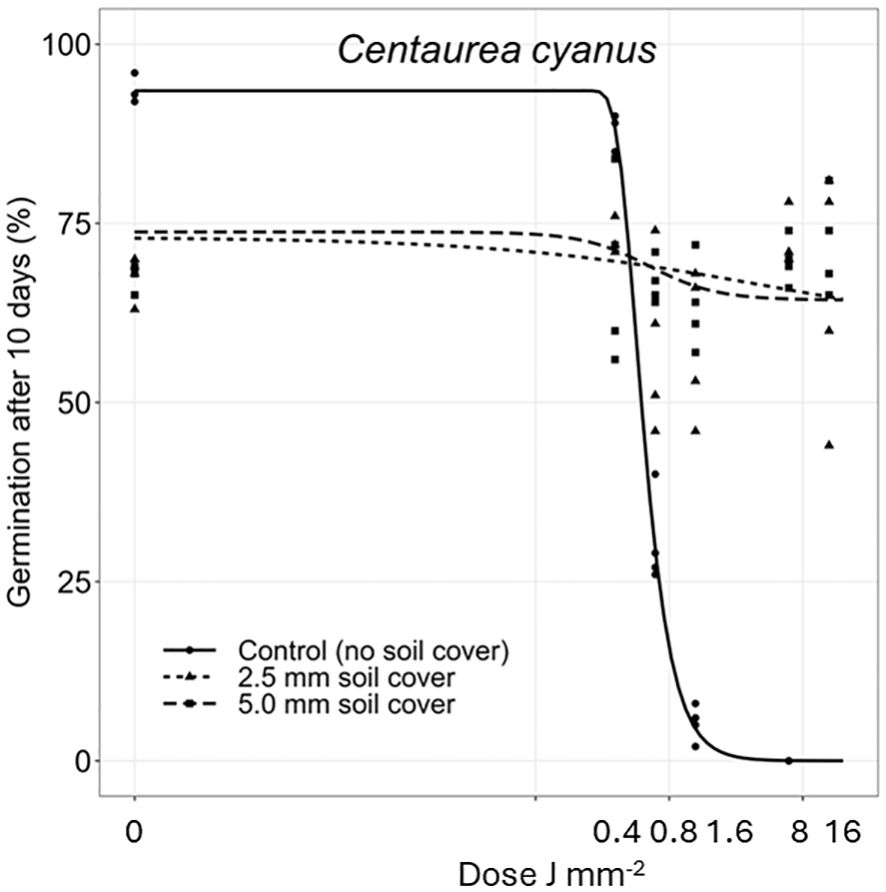
Figure 6 Dose-response experiments with Centaurea cyanus. Seeds were treated directly on the seed surface, and thereafter covered with 2.5 or 5 mm of soil.
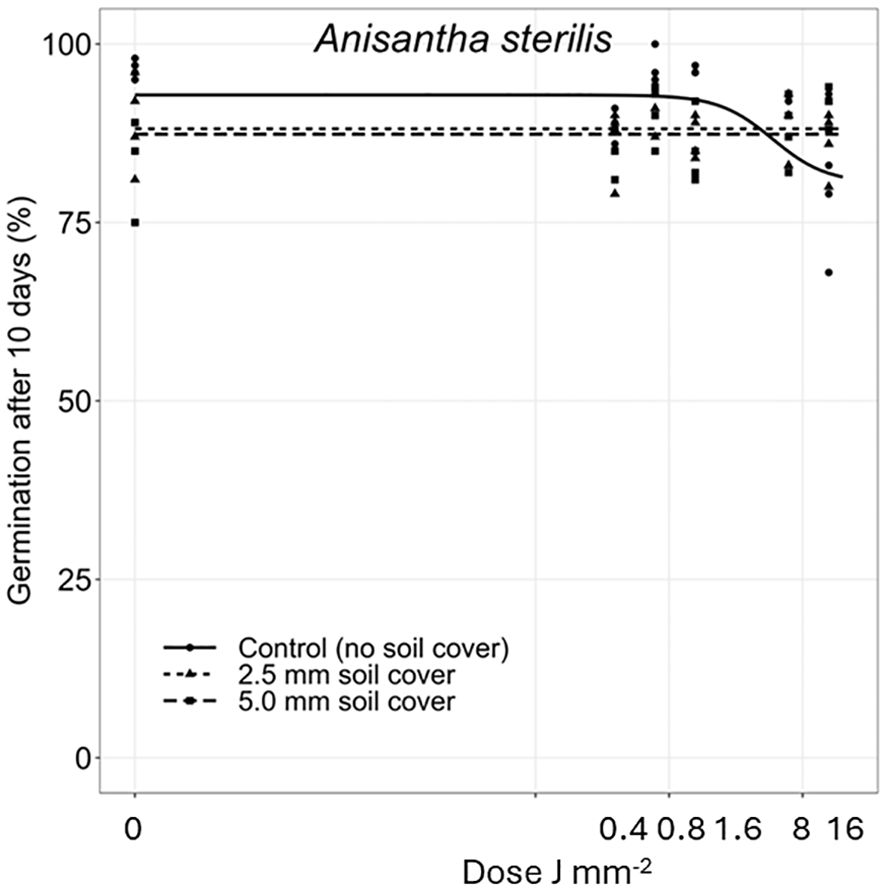
Figure 7 Dose-response experiments with Anisantha sterilis. Seeds were treated directly on the seed surface, and after covered with 2.5 or 5 mm of soil.
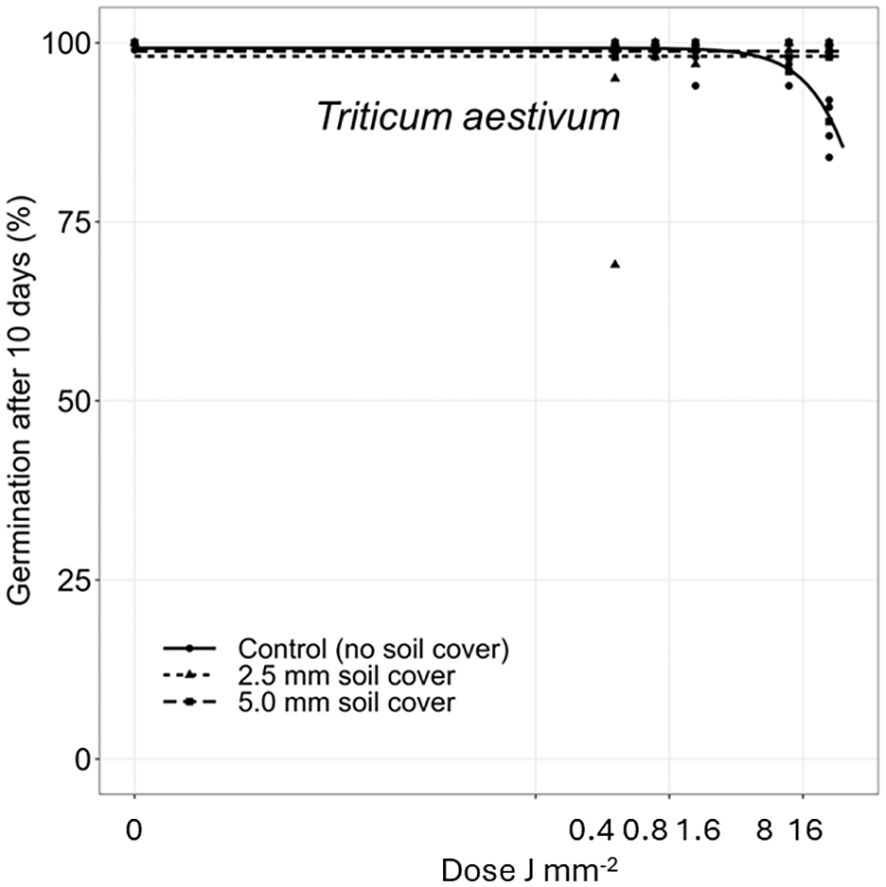
Figure 8 Dose-response experiments with wheat (Triticum aestivum). Seeds were treated directly on the seed surface, and after covered with 2.5 or 5 mm of soil.
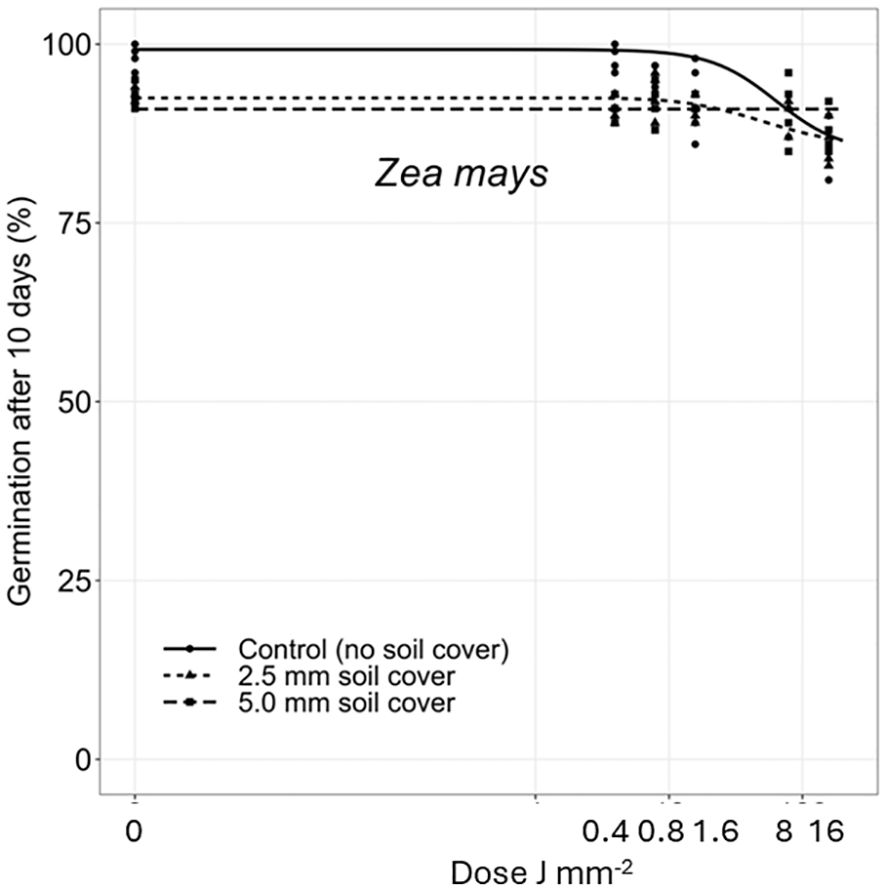
Figure 9 Dose-response experiments with maize (Zea mays). Seeds were treated directly on the seed surface, and after covered with 2.5 or 5 mm of soil.
Fungal infection was observed on seeds treated with laser on the seed surface. The infection increased with increasing laser dose (Figure 10) and over time. A slight infection was seen in wheat and maize germination boxes on Day 5 at a dose of 15.9 J mm−2. On Day 8, most of the maize and wheat seeds (>90%) were germinated in all boxes except for the highest dose (15.9 J mm−2), and fungal infection was developed in all boxes with laser treated seeds. On Day 10, all boxes were moldy apart from the controls where no fungal infection was observed.
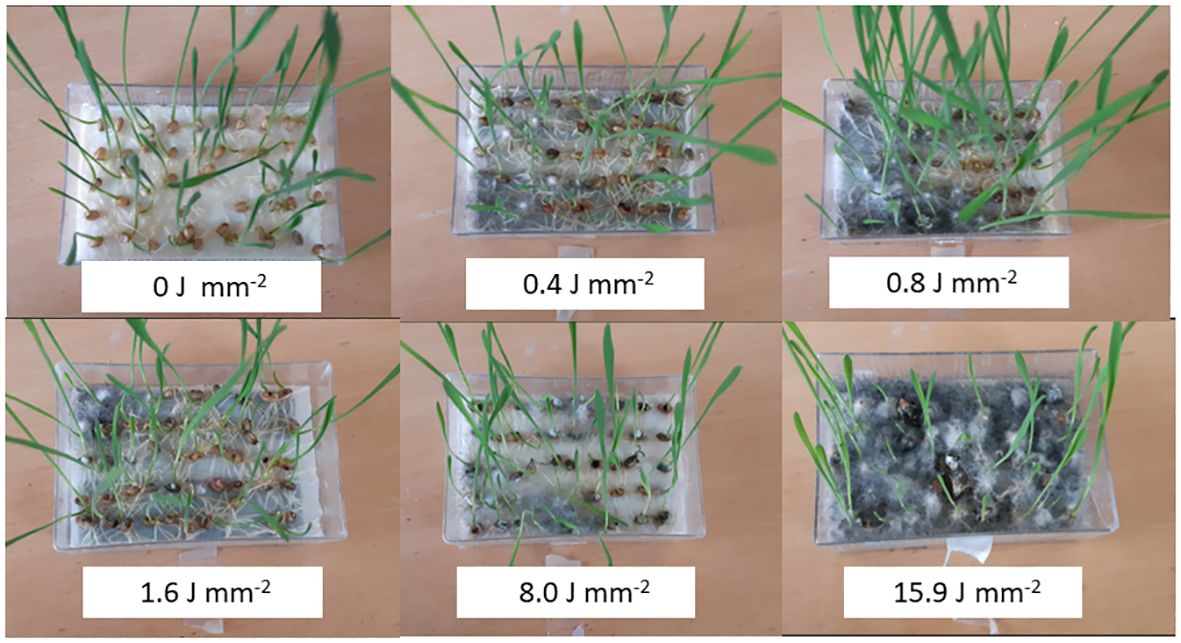
Figure 10 Dose-response experiments with wheat (Triticum aestivum). Increasing energy exposure to the seed surfaces resulting in increasing infection with fungi. At the highest dose (15.9 J mm−2) all seeds became black after 10 days, but the germination percentage remained high.
4 Discussion
The effect of the laser dosages on the germination percentage of the plant species deviated between the species when the seed surfaces were exposed directly to the laser beam. All species were affected by the highest dosages. There was no unambiguous relation between seed size and susceptibility to laser probably because the chosen plant species had different seed morphologies. The grass seeds were protected by glumes and lemmas, and the thickness of the seed coat, the biomass, and the position of the seed germ and endosperm varied greatly between the species (see Figure 2, Table 1). However, it was clear that the smallest seeds were the most susceptible.
The laser did not affect the germination percentage of seeds covered with 2.5 mm or 5 mm soil except for C. cyanus seeds, which were, however, only slightly affected. Generally, seeds placed in 2.5−5 mm depth seemed well-protected from laser dosages up to 15.9 J mm−2. The soil was only exposed for one second for the laser beam at the highest dose, which we assume was not enough to transfer the heat 2.5 mm down in the moist soil but may have caused soil water evaporation. The heat spread in the soil from the laser beam depends on the water content, soil structure and composition. It cannot be excluded that other soil conditions, like a lower or higher water content or different soil textures would result in other germination responses. Andreasen et al. (2023) studied the effect of laser on different soil worm species (Enchytraeus albidus and E. crypticus) living in tubes with 10 g of different soil textures with a water content of 50% of the soil water capacity. Except in one experiment, the mortality of the worms after two weeks was unaffected by the laser energy, corresponding to 23.9 J mm−2 on the surface, indicating that a short pulse of energy did not have a significant effect on the mortality of the organisms.
Dry seeds were used in these experiments. The effect on wet or imbibed seeds may be different (Zhu et al., 2002). Fungal infection was clearly caused by the laser treatment because no control boxes became infected. The laser treatment wounded or penetrated the seed coat making access for fungi infection. The infection may have caused the minor decrease in the germination ability of maize and wheat at the highest laser dose (15.9 J mm−2) (Figure 10).
The 2 mm circular laser beam hit approximately in the middle of the seeds in all experiments. Hence, the laser energy only covered a part of the surface of the large seeds such as A. sterilis, A. fatua, wheat, and maize seeds. In contrast, the whole seed surface of S. noctiflora was exposed to irradiation. Therefore, tiny seeds are more exposed and significantly more affected than large seeds, even at small dosages. Many common weed species have considerably smaller seeds than S. noctiflora (e.g., Arenaria serpyllifolia L., Papaver rhoeas L., Stellaria media (L.) Vill.) (Holm-Nielsen, 1998), and therefore, might be even more sensitive to low laser dosages.
The diameter of the laser beam was 2 mm covering an area of (π × r2) = (22/7) × 12 mm2 = 3.14 mm2. Using a larger beam diameter (e.g., 4 mm) would cover a larger area of the seeds (12.57 mm2) and increase the probability of hitting a seed on the ground. However, distributing the energy on a larger area would be less effective or require more energy to obtain the same effect per area. The 2 mm beam diameter was chosen because it has been suggested for weed control (see https://welaser-project.eu/).
Some seeds of corn and wheat got a laser hole in the endosperm region of the seed, but the seeds germinated anyway. The higher the dose, the more fungal infection was observed, which may influence seed vigor and seedling performance. The impact on the large seeds would probably have been more pronounced if the laser had targeted the embryo of the seed. Using artificial intelligence to recognize the embryo region on larger seeds may be possible in the future (e.g., on cereals and weed seeds like Anisantha sterilis and Avena fatua seeds).
Although we made a great effort to place the seeds in the middle of the holes in the metal frames to ensure targeting the seeds after they were covered with soil, we cannot exclude that all seeds were just below the laser-exposed spot. Some of the smallest seeds may have been placed on the border or beside the exposed soil area, which could have affected the results.
In general, tiny weed seeds can easily be destroyed with relatively low laser energy, but they are challenging to recognize. They easily roll down between soil particles and disappear. They are difficult to hit with the laser under field conditions when an autonomous vehicle with laser equipment moves with a certain speed (e.g., 2−6 km hour−1). Large seeds like Avena fatua and volunteers (e.g., wheat and barley) are easier to identify and target but require a larger dose to harm or kill.
We do not consider using autonomous vehicles only to control weed seeds on the ground economically feasible. However, targeting larger seeds with a laser while weed seedlings are controlled with lasers may be an option in the future. Still, it requires developing seed recognition tools based on artificial intelligence (Zhao et al., 2022). Many common weed species continuously shatter their seeds during the growing season (Burton et al., 2016; Bitarafan and Andreasen, 2020b). Laser-based weed control will often require several treatments, for example, in row crops (Andreasen et al., 2022), and potential weed seeds could be detected on the ground and killed simultaneously. Our result shows that it is necessary to use higher laser energy dosages to harm large seeds than to control small weed seedlings.
The WeLASER project (https://welaser-project.eu/) focused on controlling weed seedlings in sugar beet and maize fields where row distances often are between 50−75 cm. Such crops are weak competitors to weeds early in the season because the crops cover the ground late, and 3−4 passes are often necessary to control weeds, which continuously germinate and establish if sufficient light reaches the ground. In such crops, it makes sense to control seeds on the soil surface while the laser robot controls the weed seedlings.
In conservation tillage systems, the laser may be a valuable tool to control seeds on the soil surface. However, no-till cropping systems are often characterized by a high content of organic matter on the soil surface, which may cover shattered weed seeds or makes them difficult to find. Under dry conditions, special precautions must be taken to avoid laser weeding igniting dry organic material and starting a fire.
5 Conclusion
The germination ability of the plant species was differently affected by the laser treatments. Small seeds (S. noctiflora and C. cyanus) were significantly affected by low laser energy doses on the seed surface and completely burned at high dosages (8.0 and 15.9 J mm−2). Anisantha sterilis seeds were only affected by the high laser doses. There was not a clear relation between seed size and the effect of the lasering. Increasing laser dose resulted in increasing fungal infection of seeds irradiated directly on the seed surface. Although the seed germination of maize and wheat was almost unaffected by the lasering, seeds receiving the largest dosages became more infected than seeds exposed to small doses, which may influence the vigor. Seeds covered with a soil layer (2.5 or 5 mm) were not affected by the laser treatments except C. cyanus. Controlling large weed seeds and seeds from volunteers on the ground while weed seedlings are controlled with lasers seems realistic in the future but energy doses larger than 15.9 J mm−2 would be necessary to kill large seeds like cereals. Still, it requires the development of seed recognition tools based on artificial intelligence and field tests, for example, with the WeLASER robot, to assess its suitability for weed seed control. Laser weed seed control might be more appropriate on ploughed fields in row crops like sugar beets and maize than in no-till cropping systems due to the risk of igniting dry organic material on the soil surface and starting a fire.
Data availability statement
The raw data supporting the conclusions of this article will be made available by the authors, without undue reservation.
Author contributions
CA: Conceptualization, Data curation, Formal analysis, Funding acquisition, Investigation, Methodology, Project administration, Resources, Supervision, Validation, Visualization, Writing – original draft, Writing – review & editing. EV: Data curation, Formal analysis, Methodology, Validation, Writing – review & editing, Investigation. NS: Data curation, Investigation, Validation, Writing – review & editing. KJ: Data curation, Investigation, Validation, Writing – review & editing. SJ: Data curation, Formal analysis, Methodology, Validation, Visualization, Writing – review & editing.
Funding
The author(s) declare financial support was received for the research, authorship, and/or publication of this article. This work is funded by the EU–project WeLASER “Sustainable Weed Management in Agriculture with Laser-Based Autonomous Tools,” Grant agreement ID: 101000256, funded under H2020-EU.
Acknowledgments
Thanks to Technician Mathias Mons Mørch Hansen for technical support to the experiments.
Conflict of interest
The authors declare that the research was conducted in the absence of any commercial or financial relationships that could be construed as a potential conflict of interest.
Publisher’s note
All claims expressed in this article are solely those of the authors and do not necessarily represent those of their affiliated organizations, or those of the publisher, the editors and the reviewers. Any product that may be evaluated in this article, or claim that may be made by its manufacturer, is not guaranteed or endorsed by the publisher.
Supplementary material
The Supplementary Material for this article can be found online at: https://www.frontiersin.org/articles/10.3389/fagro.2024.1342372/full#supplementary-material
References
Akhter M. J., Sønderskov M., Loddo D., Ulber L., Hull R., Kudsk P. (2023). Opportunities and challenges for harvest weed seed control in European cropping systems. Eur. J. Agron. 142, 126639. doi: 10.1016/j.eja.2022.126639
Anderson R. L., Soper G. (2002). Review of volunteer wheat (Triticum aestivum): Seedlings emergence and seed longevity in soil. Weed Tech. 17, 620–626. doi: 10.1614/0890-037X(2003)017[0620:ROVWTA]2.0.CO;2
Andreasen C., Scholle. K., Saberi M. (2022). Laser weeding with small autonomous vehicles: friends or foes? Front. Agron. 4. doi: 10.3389/fagro.2022.841086
Andreasen C., Stryhn H. (2012). Increasing frequency of weed species in Danish beet, pea and winter barley fields. Crop Prot. 36, 11–17. doi: 10.1016/j.cropro.2012.01.012
Andreasen C., Vlassi E., Johannsen K. S., Jensen S. M. (2023). Side-effects of laser weeding: quantifying off-target risks to earthworms (Enchytraeids) and insects (Tenebrio molitor and Adalia bipunctata). Front. Agron. 5. doi: 10.3389/fagro.2023.1198840
Anonymous (2020). Bekendtgørelse om flyvehavre (Copenhagen, Denmark: BEK nr 730 af 29/05/2020, Ministeriet for Fødevarer, Landbrug og Fiskeri). Available at: https://www.retsinformation.dk/eli/lta/2020/730.
Arefi A., Motlagh A. M., Khoshroo A. (2011). Recognition of weed seed species by image processing. J. Food Agricul. Environ. 9, 379–383. doi: 10.1234/4.2011.1972
Baraibar B., Carrión E., Recasens J., Westerman P. R. (2011). Unravelling the process of weed seed predation: developing options for better weed control. Biol. Control 56, 85–90. doi: 10.1016/j.biocontrol.2010.09.010
Bates D., Maechler M., Bolker B., Walker S. (2015). Fitting linear mixed-effects models using lme4. J. Stat. Software 67, 1–48. doi: 10.18637/jss.v067.i01
Beckie H. J., Francis A., Hall L. M. (2012). The biology of canadian weeds. 27. Avena fatua L. (Updated). Can. J. Plant Sci. 92, 1329–1357. doi: 10.4141/cjps2012-005
Bitarafan Z., Andreasen C. (2020a). Harvest Weed Seed Control: seed production and retention of Fallopia convolvulus, Sinapis arvensis, Spergula arvensis and Stellaria media at spring oat maturity. Agron. 10, 1–10. doi: 10.3390/agronomy10010046
Bitarafan Z., Andreasen C. (2020b). Seed retention of ten common weed species at oat harvest reveals the potential for harvest weed seed control. Weed Res. 60, 343–352. doi: 10.1111/wre.12438
Burton N. R., Beckie H. J., Willenborg C. J., Shirtliffe S. J., Schoenau J. J., Johnson E. N. (2016). Evaluating seed shatter of economically important weed species. Weed Sci. 64, 673–682. doi: 10.1614/WS-D-16-00081.1
Clarke J., Moss S., Orson J. (2000). The future for grass weed management in the UK. Pesticide outlook. Pesticide Outlook. 11, 5963. doi: 10.1039/b006322n
Coleman G., Betters C., Squires C., Leon-Saval S., Walsh M. (2021). Low energy laser treatments control annual ryegrass (Lolium rigidum). Front. Agron. 2. doi: 10.3389/fagro.2020.601542
Emmi L., Fernández R., Gonzalez-de-Santos P., Francia M., Golfarelli M., Vitali G., et al. (2023). Exploiting the internet resources for autonomous robots in agriculture. Agricult. 13, 1005. doi: 10.3390/agriculture13051005
EU (2009) Directive 2009/128/EC of the European Parliament and of the Council of 21 October 2009 establishing a framework for community action to achieve the sustainable use of pesticides. Available online at: http://eur-lex.europa.eu/LexUriServ/LexUriServ.do?uri=OJ:L:2009:309:0071:0086:en:PDF (Accessed July 17, 2023).
Gaetani R., Lacotte V., Dufour V., Clavel A., Duport G., Gaget K., et al. (2021). Sustainable laser-based technology for insect pest control. Sci. Rep. 11, 11068. doi: 10.1038/s41598-021-90782-7
Heap I. (2023) The international survey of herbicide resistant Weeds. Available online at: www.weedscience.org.
Heisel T. J., Andreasen C., Christensen S. (2002). Using laser to measure stem thickness and cut weed stems. Weed Res. 42 (3), 242–248. doi: 10.1046/j.1365-3180.2002.00282.x
Holm-Nielsen C. (1998). Frø i det dyrkede land (Danmarks Jordbrugsforskning., Flakkebjerg: Ministeriet for Fødevarer, Landbrug og Fiskeri), ISBN: ISBN:87-984996-0-2.
Hothorn T., Bretz F., Westfall P. (2008). Simultaneous inference in general parametric models. Biometrical J. 50, 346–363. doi: 10.1002/bimj.200810425
ISTA (2011). International rules for seed testing, germination test (Basserdorf, Switzerland: International Seed Testing Association).
Jensen S. M., Wolkis D., Keshtkar E., Streibig J. C., Ritz C. (2020). Improved two-step analysis of germination data from complex experimental designs. Seed Sci. Res. 30, 194–198. doi: 10.1017/S0960258520000331
Lefebvre M., Langrell S. R. H., Gomez-y-Paloma S. (2015). Incentives and policies for integrated pest management in Europe: a review. Agron. Sustain. Dev. 35, 27–45. doi: 10.1007/s13593-014-0237-2
Luo T., Zhao J., Gu Y., Zhang S., Qiao X., Tian W., et al. (2023). Classification of weed seeds based on visual images and deep learning. Inform. Process. Agricult. 10, 40–51. doi: 10.1016/j.inpa.2021.10.002
Menchari Y., Délye C., Le Corre V. (2007). Genetic variation and population structure in black-grass (Alopecurus myosuroides Huds.), a successful, herbicide-resistant, annual grass weed of winter cereal fields. Mol. Ecol. 16, 3161–3172. doi: 10.1111/j.1365-294X.2007.03390.x
Mullen E. R., Rutschman P., Pegram N., Patt J. M., Adamczyk J. J. Jr., Johanson E. (2016). Laser system for identification, tracking, and control of flying insects. Optics Express 24, 11828–11838. doi: 10.1364/OE.24.011828
Navntoft S., Wratten S. D., Kristensen K., Esbjerg P. (2009). Weed seed predation in organic and conventional fields. Biol. Control 49, 11–16. doi: 10.1016/j.biocontrol.2008.12.003
Olesen M. H., Duijn B., Boelt B. (2014). Introduction of new methods: Spectral imaging. Seed Testing Int. 147, 10–13. doi: 10.5555/20143186149
Rakhmatulin I., Kamilaris A., Andreasen C. (2021). Deep neural networks to detect weeds from crops in agricultural environments in real-time: A review. Remote Sens. 13, 4486. doi: 10.3390/rs13214486
R Core Team (2022). R: a language and environment for statistical computing (Vienna, Austria: R Foundation for Statistical Computing). Available at: https://www.R-project.org/.
Ritz C., Jensen S. M., Gerhard D., Streibig J. C. (2019). Dose-response analysis using R. 1st ed (Boca Raton: Chapman and Hall/CRC). doi: 10.1201/b21966
Sarabi V. (2019). Factors that influence the level of weed seed predation: a review. Weed Biol. Manage. 19, 61–74. doi: 10.1111/wbm.12186
Shrestha S., Deleuran L. C., Olesen M. H., Gislum R. (2015). Use of multispectral imaging in varietal identification of tomato. Sensors 15, 4496–4512. doi: 10.3390/s150204496
Stankiewicz-Kosyl M., Synowiec A., Haliniarz M., Wenda-Piesik A., Domaradzki K., Parylak D., et al. (2020). Herbicide resistance and management options of Papaver rhoeas L. and Centaurea cyanus L. @ in Europe: a review. Agron. 10, 874. doi: 10.3390/agronomy1006087
Tran D., Schouteten J. J., Degieter M., Krupanek J., Jarosz W., Areta A., et al. (2023). European stakeholders' perspectives on implementation potential of precision weed control: the case of autonomous vehicles with laser treatment. Precis. Agric. 12, 1–23. doi: 10.1007/s11119-023-10037-5
Viechtbauer W. (2010). Conducting meta-analyses in R with the metafor package. J. Stat. Software 36, 1–48. doi: 10.18637/jss.v036.i03
Walsh M. J., Broster J. C., Schwartz-Lazaro L. M., Norsworthy J. K., Davis A. S., Tidemann B. D., et al. (2018). Opportunities and challenges for harvest weed seed control in global cropping systems. Pest Manage. Sci. 74, 2235–2245. doi: 10.1002/ps.4802
Walsh M. J., Raymond B., Harrington R. B., Powles S. B. (2012). Harrington seed destructor: a new nonchemical weed control tool for global grain crops. Crop Sci. 52, 1343–1347. doi: 10.2135/cropsci2011.11.0608
Wieliczka D. M., Weng S., Querry M. R. (1989). Wedge shaped cell for highly absorbent liquids. Infrared optical constants of water. Appl. Optic. 28, 1714–1719. doi: 10.1364/AO.28001714
Zhao L., Haque S. M. R., Wang R. (2022). Automated seed identification with computer vision: challenges and opportunities. Seed Sci. Technol. 50, 75–102. doi: 10.15258/sst.2022.50.1.s.05
Keywords: integrated weed management, laser weeding, non-chemical weed control, site-specific weed management, seed shattering, thermal weed control
Citation: Andreasen C, Vlassi E, Salehan N, Johannsen KS and Jensen SM (2024) Laser weed seed control: challenges and opportunities. Front. Agron. 6:1342372. doi: 10.3389/fagro.2024.1342372
Received: 21 November 2023; Accepted: 11 March 2024;
Published: 27 March 2024.
Edited by:
Baruch Rubin, Hebrew University of Jerusalem, IsraelReviewed by:
Ioannis Roussis, Agricultural University of Athens, GreeceSimerjeet Kaur, Punjab Agricultural University, India
Copyright © 2024 Andreasen, Vlassi, Salehan, Johannsen and Jensen. This is an open-access article distributed under the terms of the Creative Commons Attribution License (CC BY). The use, distribution or reproduction in other forums is permitted, provided the original author(s) and the copyright owner(s) are credited and that the original publication in this journal is cited, in accordance with accepted academic practice. No use, distribution or reproduction is permitted which does not comply with these terms.
*Correspondence: Christian Andreasen, can@plen.ku.dk