The complement system and its involvement in inhibition of Batrachochytriym dendrobatidis, a lethal fungal pathogen of amphibians
- 1Earl Wooster High School, Reno, NV, United States
- 2Davidson Academy High School, Reno, Reno, NV, United States
- 3Department of Biology, University of Nevada, Reno, Reno, NV, United States
The field of ecological immunology, or ecoimmunology, has provided valuable insights on the immune responses of diverse host organisms threatened by infectious diseases in many different environments. One infectious disease that has been particularly notable for its impacts on host populations is amphibian chytridiomycosis, which has been linked with amphibian declines around the world. Amphibian immune responses to the pathogen that causes chytridiomycosis (Batrachochytriym dendrobatidis) are not well understood but thought to involve innate immune factors, including the complement system. In this study, we tested the ability of complement proteins to inhibit B. dendrobatidis in in vitro challenge assays. We found that complement proteins from amphibian plasma that were not heat inactivated reduced the viability and growth of B. dendrobatidis. The inhibitory efficacy was similar to effects on Pseudomonas fluorescens, a bacterium that is known to be inhibited by complement protein activation. These findings suggest inhibition of B. dendrobatidis that is consistent with the involvement of the complement system. In addition, we provide methods for standardizing pathogen killing assays, and set a foundation for further investigations on the amphibian complement system and other immune responses to amphibian chytridiomycosis.
1 Introduction
The emerging field of ecoimmunology is providing a deeper understanding of how immune defenses function according to the ecology and evolutionary biology of host organisms (Martin et al., 2011; Assis et al., 2022). Amphibians have gained prominence as valuable model species in ecoimmunology for at least three important reasons. First, although many components of the amphibian immune system are highly conserved among vertebrates, the diversity of immunological responses among amphibian host species provides insights into susceptibility to disease and the generation of resistance mechanisms (Assis et al., 2022; Ruiz & Robert, 2023). Second, as ectotherms, environmental conditions (e.g., temperature, relative humidity, and rainfall) that affect amphibian physiology also mediate immunity and the manifestation of disease (Raffel et al., 2006; Rollins-Smith et al., 2011; Rollins-Smith, 2017; Le Sage et al., 2021; Rosa et al., 2022). Third, infectious diseases have been implicated in severe global declines in amphibian populations and species in recent decades (Wake and Vredenburg, 2008; Scheele et al., 2019), making the study of amphibian immunity an important focus of recent research. Overall, amphibians have shed light on host immune responses, disease dynamics, and the interactions of environmental variables and immunity for a wide variety of organisms (Assis et al., 2022). As such, a better understanding of amphibian immunity can make meaningful contributions to the field of ecoimmunology, disease ecology, and the study of infectious disease in general (Assis et al., 2022; Ruiz & Robert, 2023).
Similar to other vertebrates, amphibian immunity actively maintains a diverse set of physiological mechanisms, orchestrated by cells, tissues, and organs that collectively form the innate and adaptive branches of the immune system (Grogan et al., 2018a; Ruiz and Robert, 2023). While innate immunity is the less specific, first line of defense against harmful pathogens, adaptive immunity triggers antigen-specific responses (Parham, 2014; Murphy & Weaver et al., 2016). Within the innate immune system, key immune defense cells (e.g., macrophages, granulocytes, neutrophils etc.), are often assisted by additional, complementary components of the immune system (Parham, 2014; Murphy & Weaver et al., 2016). For example, the complement cascade (the complement system) involves specialized proteins, produced by the liver, that bolster specific immune responses following pathogen colonization (Carroll, 1998; 2004; Janeway et al., 2001). In this way, the complement system augments immune reactions, improving the efficacy of host defenses against infectious agents (Carroll, 1998; 2004; Janeway et al., 2001).
Complement proteins found in blood plasma play a role in enhancing the effectiveness of the immune system against harmful pathogens, including bacteria, viruses, fungal pathogens, and parasites (Rus et al., 2005; Carroll, 2008; Speth et al., 2008). Upon pathogen colonization, the complement system can be activated by one of three pathways: the classical, lectin, and alternative complement pathways (Carroll, 1998; 2004; Janeway et al., 2001; Parham, 2014). These pathways are triggered by detection of different pathogen-associated molecular patterns [PAMPs, e.g., carbohydrate structures (Janeway et al., 2001; Parham, 2014)] or by antigen-antibody complexes (e.g., in the case of the classical pathway; Janeway et al., 2001; Parham, 2014). Following activation, complement components undergo a series of biochemical reactions that can enhance the inflammatory response, agglutinate pathogens, attract phagocytes and other immune cells to the location of the infection, and destroy an infectious agent (Janeway et al., 2001; Parham, 2014).
The complement cascade is thought to be a particularly important defense mechanism against the lethal disease known as amphibian chytridiomycosis (Rosenblum et al., 2009; Gervasi et al., 2014; Savage et al., 2016; Grogan et al., 2018a, Grogan et al., 2018b). Chytridiomycosis is caused by the fungal pathogen Batrachochytrium dendrobatidis, a pathogenic fungus in the phylum Chytridiomycota (Berger et al., 1998; Longcore et al., 1999). This disease has been identified as one of the leading causes of disease-induced amphibian declines and extinctions worldwide, causing severe declines in an estimated 500+ species (Scheele et al., 2019). B. dendrobatidis can be transmitted rapidly among individual amphibian hosts by means of free-swimming infectious cells called zoospores (Longcore et al., 1999). Subsequently, the zoospores colonize epidermal cells, causing infection, and leading to physiological deterioration and ultimately death in many different amphibian host species (Berger et al., 2005; Voyles et al., 2009; Wu et al., 2019).
Although some innate defenses of amphibian skin have been studied [e.g., skin mucous, (reviewed in Grogan et al., 2018a)], other nonspecific defenses, such as the complement system, have yet to be thoroughly investigated (Rodriguez & Voyles, 2020). The lack of investigation on the complement system is puzzling because early studies using molecular approaches suggested that an early activation of complement pathways may confer resistance to B. dendrobatidis and reduce the likelihood of disease development and mortality (Rosenblum et al., 2009; Grogan et al., 2018b). Indeed, some of these previous studies speculated that complement suppression may be a “characteristic feature” of chytridiomycosis (Rosenblum et al., 2009) and that complement activity may be a “useful predictor” of amphibian susceptibility (Savage et al., 2016). While the complement system may be a critical determinant of susceptibility or resistance to lethal chytridiomycosis, to date there is little empirical evidence that amphibian complement proteins can effectively inhibit B. dendrobatidis [as has been demonstrated for other bacterial pathogens (e.g., Pseudomonas fluorescens; Wu et al., 2022)].
In this study, we aimed to address this gap in knowledge of prospective amphibian immune factors that are important for defense against chytridiomycosis. We hypothesized that amphibian complement proteins can inhibit the growth of B. dendrobatidis. To test this hypothesis, we assessed the ability of complement proteins to limit viability of two microbes, one bacterium (P. fluorescens, which we used as a reference microorganism) and B. dendrobatidis, in in vitro challenge assays. We predicted that complement proteins would inhibit the growth of both microbes, providing preliminary evidence that this immune mechanism may be implicated in amphibian host resistance or susceptibility to chytridiomycosis.
2 Materials and methods
To collect amphibian blood plasma, we obtained Lithobates catesbeianus frogs from a commercial supplier (Carolina Biological, North Carolina, USA). This species is thought to be tolerant of B. dendrobatidis infection (Daszak et al., 2004). We euthanized ten healthy frogs using 1% of tricaine methanesulfonate (MS-222, Western Chemical, USA) neutralized to pH 7.0 with sodium bicarbonate (Wright and Whitaker, 2001). We used heparinized syringes with a 20-gauge needle for cardiocentesis, withdrawing approximately 10 ml of blood per frog. We centrifuged the blood samples at 3200 x G for 15 m to separate the red blood cells from the plasma. We withdrew the plasma and transferred it to a sterile 2 ml cryotube that we froze at -80°C until the start of our experiment.
We obtained P. fluorescens from a commercial source (Carolina Biological, North Carolina, USA). We used the B. dendrobatidis isolate called “Rio Maria” that was isolated from Panama where chytridiomycosis led to severe amphibian declines (Voyles et al., 2018). The isolate has been genotyped and it is part of the Global Panzootic Lineage (i.e., BdGPL; Voyles et al., 2018). We maintained P. fluorescens and B. dendrobatidis on plates with agar media prior to the start of our experiment. We used standard protocols to make Tryptic Soy Agar (TSA) for P. fluorescens (Stone & Johnson, 2002) and Tryptone Gelatin hydrolysate Lactose (TGhL) agar plates for B. dendrobatidis (Longcore et al., 1999). We streaked a new TSA plate with P. fluorescens and incubated at 20°C for 24 h prior to the start of our experiment. Because the growth rate of B. dendrobatidis is comparatively slower (4-7 days at 20°C to reach maximum growth; Voyles et al., 2017), we created a new TGHL plate 5 d prior to the start of our experiment and monitored for maximum growth with light microscopy (Voyles, 2011).
To prepare the experiments, we added one sterile ~1 µl loop of actively growing P. fluorescens to 25 ml of sterile Tryptic Soy Broth (TSB) in a 50 ml sterile conical tube, and vortexed the tube to homogenize the solution. We counted cells of P. fluorescens using a hemocytometer and diluted the culture solution with TSB to generate an inoculum with dilution of 1 x 107 cells ml-1. For B. dendrobatidis isolate, we filtered active culture through sterilized filter paper to remove sporangia (Voyles, 2011). We quantified the dilution of zoospores using a hemocytometer and diluted with TGhL broth to reach a final dilution of 5 x 105 zoospores ml-1.
We conducted the experiments using sterile, flat-bottom 96 well plates. We added 50 µl of sterile media (TSB and TGhL for P. fluorescens and B. dendrobatidis respectively) into each well. We then added 20 µl of frog plasma to N = 5 wells and diluted the plasma before adding 10 µl of P. fluorescens or B. dendrobatids. To provide a positive control for the frog plasma, we repeated the dilution in N = 5 wells using sterile amphibian phosphate buffered saline (aPBS; Wright and Whitaker, 2001) instead of frog plasma. To provide a negative control for P. fluorescens and B. dendrobatidis growth, we subaliquoted the filtrates and heat-killed the culture by submerging the aliquot in 100°C water for 10 m (Voyles et al., 2017) and then adding heat killed cuture to N = 5 wells.
To determine if inhibition could be due to complement proteins, rather than other plasma constituents (e.g., antibodies), we sub aliquoted the frog plasma into a sterile 1.5 µl tube and incubated it in a water bath at 56°C for 30 m. This temperature treatment is a well-established treatment that deactivates complement proteins (Soltis et al., 1979), but is less concerning for altering antibody conformation or leading to antibody aggregation (Jones, 1927; Le Basle et al., 2020; Wälchli et al., 2020). As a result, we used the plasma sample with “heat inactivated” (HI) complement proteins to test for microbial inhibition. Following the temperature treatment, we added 20 µl of heat-inactivated frog plasma to N = 5 wells and diluted the plasma before adding10 µl of P. fluorescence or B. dendrobatidis.
We incubated the plates at 21°C for 12 h and 5 d respectively for each of the plates containing P. fluorescence or B. dendrobatidis. During the incubation period, we used light microscopy to inspect the wells for microbial growth (and, in the case of B. dendrobatidis, production of infectious zoospores). Following the incubation period, we used an MTT assay, which is a colorimetric test of cell metabolic activity (Lindauer et al., 2019), to quantify differences in culture viability among treatments for both P. florecences and B. dendrobatidis. To do so, we added 20 µl of MTT to each well, incubated for 2 h at 21°C, and solubilized by adding 140 µl sodium dodecyl sulfate in dimethylformamide solution (20% SDS/50% DMF w/v) and homogenized gently (Hansen et al., 1989). We collected optical density data with a microplate reader (Biotek ELx800, VT, USA) using a 570 nm wavelength filter (Lindauer et al., 2022).
For statistical analysis, we first corrected for initial culture inoculation and media color by subtracting OD values of heat-killed controls from OD values of wells containing the inoculating filtrate. We used R (Version 2023.03.0 + 386), checked for any violations of normality using QQ plots and Shapiro-Wilk’s tests and for homogeneity of variance using Levene’s Test. Because there were no violations of these assumptions, we used an ANOVA and Tukey’s HSD post hoc tests to test for differences among the treatment groups. As such, our figures represent the preliminary results from the experiments with each microbe respectively.
3 Results
We found that culture viability differed among treatment groups for both P. fluorescens (Figure 1A) and for B. dendrobatidis (Figure 1B). Specifically, compared to the positive control (i.e., with no plasma), P. fluorescens viability was reduced at the 1:10 dilution (Positive Control mean ± SD: 2.39 ± 0.22, 1:10 dilution mean ± SD: 0.39 ± 0.11) and at the 1:1000 dilution (1:1000 dilution mean ± SD: 1.06 ± 0.20; ANOVA with Tukey HSD posthoc test: F(4,4) = 17.6, P < 0.001, Figure 1A). Compared to the heat inactivated plasma, P. fluorescens viability was also significantly reduced at the 1:10 (HI 1:10 mean ± SD: 2.84 ± 0.58) and at the 1:1000 dilution (HI 1:1000 dilution mean ± SD: 0.44 ± 0.20; P < 0.001, Figure 1A).
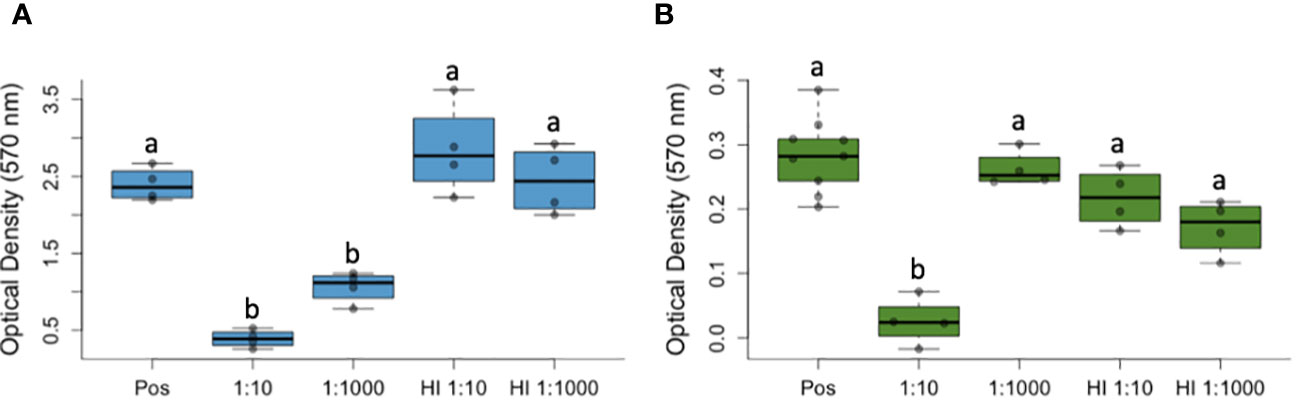
Figure 1 Complement proteins from amphibian plasma reduces the viability of Pseudomonas fluorescens (A) and Batrachochytrium dendrobatidis (B), a lethal pathogen of amphibians that causes the disease chytridiomycosis. Optical density measurements (with a 570 nm filter) suggest reduced viability of both microbes compared to a positive control (containing no plasma, Pos) and to heat treatments for the plasma that inactivated complement proteins (i.e., heat inactivated, HI). In both box-and-whisker plots, the bars represent variability outside of quartiles and letters (a, b) indicate significant differences among groups. Labels on the x-axis (1:10, 1:1000) represent the dilution of frog plasma.
For B. dendrobatidis, culture viability was significantly reduced at one dilution (1:10) compared to all other groups, including the positive control with no plasma (Positive Control mean ± SD: 0.28 ± 0.06, 1:10 Dilution mean ± SD: 0.03 ± 0.04; ANOVA with Tukey HSD posthoc test: F(4,4) = 23.3, P = 0.002, Figure 1A) and the wells containing heat-inactivated plasma. Using light microscopy, we observed signs of cytotoxicity over the course of the experiment, with no signs of agglutination (i.e., clumping that would indicate involvement of antibodies), and reduced growth (no encystment, maturation, or zoospore production).
4 Discussion
Investigators have made considerable advances in the pursuit of understanding amphibian immune responses in recent decades (Raffel et al., 2006; Rollins-Smith et al., 2011; Le Sage et al., 2021; Assis et al., 2022; 2017; Rosa et al., 2022; Ruiz and Robert, 2023). However, there is still much to learn about how amphibian defenses operate in response to infection with Batrachochytrium dendrobatidis and the development of chytridiomycosis (reviewed in Grogan et al., 2018b; Rodriguez & Voyles, 2020). In this study, we investigated inhibition of B. dendrobatidis that would be consistent with complement system activity. We found that viability of B. dendrobatidis was significantly reduced when exposed to amphibian blood plasma compared to positive control treatments containing no plasma. Furthermore, B. dendrobatidis viability was significantly reduced compared to treatment wells where the complement proteins were heat inactivated. Taken together, these results suggest that complement proteins specifically (rather than other plasma constituents) can inhibit B. dendrobatidis in in vitro assays. As such, these findings set a foundation for further investigations on the amphibian complement system, and other immune responses, to B. dendrobatidis infection and the development of chytridiomycosis.
Previous research on complement activation during B. dendrobatidis infection has used two main approaches: (1) in vitro challenge assays known as bacterial killing assays (commonly referred to as “BKAs”) or (2) molecular techniques to quantify the production of RNA transcripts (i.e., transcriptomics) using microarrays or RNAseq (reviewed in Rodriguez & Voyles, 2020). In many cases, these methods of assessing complement activation have been integrated with B. dendrobatidis infection experiments where blood or tissue samples have been collected over time post inoculation (Rosenblum et al., 2009; Gervasi et al., 2014; Savage et al., 2016; Grogan et al., 2018a, Grogan et al., 2018b), which is an ideal approach to evaluate immune responses occurring over an unknown time frame. However, collectively these studies had many important differences in their methodological approaches (e.g., using different isolates and doses of B. dendrobatidis, inoculation and sample collection methods, host species, etc.) and produced mixed results, leaving multiple questions concerning the complement system to be investigated (Rodriguez & Voyles 2020). For example, some of the previous studies used whole blood rather than blood serum or plasma and/or did not incorporate heat inactivation to distinguish between fungicidal activity of complement proteins or other blood components [e.g., lymphocytes, lysozyme, antibodies, etc. (reviewed in Rodriguez & Voyles 2020)]. As such, the existing literature provides promising information, but leaves many unknowns to unravel concerning the role of the complement system in chytridiomycosis.
Our results provide a proof-of-concept verification that complement proteins can indeed inhibit B. dendrobatidis, offers methods to help standardize a B. dendrobatidis Killing Assay (or, we suggest, “BdKA”). As such, this study lays the groundwork to gain a deeper understanding of the mechanisms of pathogenesis, determinants of susceptibility, and resistance/tolerance in amphibians. Specifically, with the knowledge that complement proteins are capable of inhibiting B. dendrobatidis, we can now explore multiple questions concerning this amphibian immune defense. For example, we can use these methods to, first, determine which of the complement pathways may be used in live amphibians with in vivo experiments. B. dendrobatidis contains glycoproteins on the extracellular surface (Dillon et al., 2017), which may trigger the lectin pathway (Patin et al., 2019). Experimental work using complement depletion methods can help to resolve this question. Second, we can work to understand how environmental conditions (e.g., temperature) may alter complement (as well as other immune) responses (Maniero and Carey, 1997; Raffel et al., 2006; Moretti et al., 2019; Rodriguez & Voyles 2020). Previous studies have suggested that bactericidal efficacy in blood samples collected from leopard frogs and multiple species of toads is highly temperature dependent (Maniero and Carey, 1997; Moretti et al., 2019). Our methods can help determine how, and to what extent, temperature could be involved in complement system functioning, which may help resolve seasonal immune fluctuations that have been observed for chytridiomycosis (Le Sage et al., 2021; Rosa et al., 2022). Third, we can build on this preliminary work to understand if complement system activation underpins interspecific differences in susceptibility to chytridiomycosis (Grogan et al., 2018b) and if so, we can further refine the mechanisms of complement protein effectiveness within the epidermis. Further studies that focus on a comparison of the fungicidal ability of complement proteins from susceptible and resistant/tolerant species may provide a mechanistic explanation for why amphibian species differ in their susceptibility to chytridiomycosis (Gervasi et al., 2014; Grogan et al., 2018b). This issue is particularly important for conservation initiatives because they frequently depend on our ability to identify vulnerable species that need conservation intervention (Rodriguez & Voyles, 2020).
Previous studies have predominantly focused on the mechanisms of the complement cascade in response to bacterial or viral infections, with far less research on fungal pathogens (Kozel, 1996; Speth et al., 2008). Generally, the complement system is well known for its ability to stimulate and augment other innate and acquired immune factors (Carroll, 1998; 2004; Janeway et al., 2001). As a result, previous research has focused on unraveling the mechanisms by which complement proteins influence subsequent immunological processes, potentially protecting hosts from disease development and susceptibility to illness and death (Carroll, 1998; 2004; Janeway et al., 2001). These research foci have led to a detailed understanding of the three complement pathways, all of which involve activation of C3 (by conversion of C3 to C3b and C3a) as a central component of immune regulation (Wu et al., 2022). C3a is known to bind and have bactericidal effects for a range of bacteria, including P. fluorescens (Wu et al., 2022), yet has not been thoroughly investigated for fungi (but see Sonesson et al., 2007).
With recent emergences of multiple fungal pathogens of vertebrate hosts, several of which have proven to be highly lethal [e.g., B. dendrobatidis and B. salamandrivorans in amphibians, Geomyces destructans in bats, Aspergillus fumigatus in birds (Gargas et al., 2009; Martel et al., 2013; Arné et al., 2021)], understanding host immune responses to these fungal pathogens is increasingly important for wildlife health and conservation. Although research is progressing, we currently have a limited understanding of vertebrate immune defenses across and within diverse vertebrate taxonomic groups (Assis et al., 2022). Undertaking mechanistic research that incorporates techniques from biological subdisciplines such as microbiology and immunology will provide a greater understanding of the host defenses that confer susceptibility or resistance when threatened by infectious disease.
Data availability statement
The raw data supporting the conclusions of this article will be made available by the authors without undue reservation.
Ethics statement
The animal study was approved by University of Nevada, Reno IACUC. The study was conducted in accordance with the local legislation and institutional requirements.
Author contributions
HP: Data curation, Investigation, Project administration, Writing – original draft, Writing – review & editing. JR: Data curation, Investigation, Project administration, Writing – review & editing. MT: Data curation, Investigation, Project administration, Writing – review & editing. KR: Conceptualization, Methodology, Writing – review & editing. JV: Conceptualization, Formal analysis, Funding acquisition, Investigation, Methodology, Project administration, Resources, Supervision, Validation, Visualization, Writing – original draft, Writing – review & editing.
Funding
The author(s) declare financial support was received for the research, authorship, and/or publication of this article. This study was funded by the National Science Foundation (DEB 1846403 and 2120084 to JV), Cyanco, and I-80 Gold Corps.
Acknowledgments
We thank J. Green, UNR Upward Bound, and the STEM Sisters Program.
Conflict of interest
The authors declare that the research was conducted in the absence of any commercial or financial relationships that could be construed as a potential conflict of interest.
Publisher’s note
All claims expressed in this article are solely those of the authors and do not necessarily represent those of their affiliated organizations, or those of the publisher, the editors and the reviewers. Any product that may be evaluated in this article, or claim that may be made by its manufacturer, is not guaranteed or endorsed by the publisher.
References
Arné P., Risco-Castillo V., Jouvion G., Le Barzic C., Guillot J. (2021). Aspergillosis in wild birds. J. Fungi 7, 241. doi: 10.3390/jof7030241
Assis V. R., Titon S. C., Voyles J. (2022). Ecoimmunology: what unconventional organisms tell us after two decades. Integr. Comp. Biol. 62, 1528–1535. doi: 10.1093/icb/icac148
Berger L., Speare R., Daszak P., Green D. E., Cunningham A. A., Goggin C. L., et al. (1998). Chytridiomycosis causes amphibian mortality associated with population declines in the rain forests of Australia and Central America. Proc. Natl. Acad. Sci. 95, 9031–9036. doi: 10.1073/pnas.95.15.9031
Berger L., Speare R., Skerratt L. F. (2005). Distribution of Batrachochytrium dendrobatidis and pathology in the skin of green tree frogs Litoria caerulea with severe chytridiomycosis. Dis. Aquat. organisms 68, 65–70. doi: 10.3354/dao068065
Carroll M. C. (1998). The role of complement and complement receptors in induction and regulation of immunity. Annu. Rev. Immunol. 16, 545–568. doi: 10.1146/annurev.immunol.16.1.545
Carroll M. C. (2004). The complement system in regulation of adaptive immunity. Nat. Immunol. 5, 981–986. doi: 10.1038/ni1113
Carroll M. C. (2008). Complement and humoral immunity. Vaccine 26, I28–I33. doi: 10.1016/j.vaccine.2008.11.022
Daszak P., Strieby A., Cunningham A. A., Longcore J. E., Brown C. C., Porter D. (2004). Experimental evidence that the bullfrog (Rana catesbeiana) is a potential carrier of chytridiomycosis, an emerging fungal disease of amphibians. Herpetological J. 14, 201–208.
Dillon M. J., Bowkett A. E., Bungard M. J., Beckman K. M., O'Brien M. F., Bates K., et al. (2017). Tracking the amphibian pathogens Batrachochytrium dendrobatidis and Batrachochytrium salamandrivorans using a highly specific monoclonal antibody and lateral-flow technology. Microbial Biotechnol. 10, 381–394. doi: 10.1111/1751-7915.12464
Gargas A., Trest M. T., Christensen M., Volk T. J., Blehert D. S. (2009). Geomyces destructans sp. nov. associated with bat white-nose syndrome. Mycotaxon 108, 47–154. doi: 10.5248/108.147
Gervasi S. S., Hunt E. G., Lowry M., Blaustein A. R. (2014). Temporal patterns in immunity, infection load and disease susceptibility: understanding the drivers of host responses in the amphibian-chytrid fungus system. Funct. Ecol. 28, 569–578. doi: 10.1111/1365-2435.12194
Grogan L. F., Cashins S. D., Skerratt L. F., Berger L., McFadden M. S., Harlow P., et al. (2018b). Evolution of resistance to chytridiomycosis is associated with a robust early immune response. Mol. Ecol. 27, 919–934. doi: 10.1111/mec.14493
Grogan L. F., Robert J., Berger L., Skerratt L. F., Scheele B. C., Castley J. G., et al. (2018a). Review of the amphibian immune response to chytridiomycosis, and future directions. Front. Immunol. 9. doi: 10.3389/fimmu.2018.02536
Hansen M. B., Nielsen S. E., Berg K. (1989). Re-examination and further development of a precise and rapid dye method for measuring cell growth/cell kill. J. Immunol. Methods 119, 203–210. doi: 10.1016/0022-1759(89)90397-9
Janeway C. A. Jr., Travers P., Walport M., Shlomchik M. J. (2001). “The complement system and innate immunity,” in Immunobiology: the immune system in health and disease, 5th edition (New York City, USA: Garland Science).
Jones F. S. (1927). The effect of heat on antibodies. J. Exp. Med. 46, 291–301. doi: 10.1084/jem.46.2.291
Kozel T. R. (1996). Activation of the complement system by pathogenic fungi. Clin. Microbiol. Rev. 9 (1), 34–46. doi: 10.1128/cmr.9.1.34
Le Basle Y., Chennell P., Tokhadze N., Astier A., Sautou V. (2020). Physicochemical stability of monoclonal antibodies: a review. J. Pharm. Sci. 109, 169–190. doi: 10.1016/j.xphs.2019.08.009
Le Sage E. H., LaBumbard B. C., Reinert L. K., Miller B. T., Richards-Zawacki C. L., Woodhams D. C., et al. (2021). Preparatory immunity: Seasonality of mucosal skin defences and Batrachochytrium infections in Southern leopard frogs. J. Anim. Ecol. 90, 542–554. doi: 10.1111/1365-2656.13386
Lindauer A., May T., Rios-Sotelo G., Sheets C., Voyles J. (2019). Quantifying Batrachochytrium dendrobatidis and Batrachochytrium salamandrivorans viability. EcoHealth 16, 346–350. doi: 10.1007/s10393-019-01414-6
Longcore J. E., Pessier A. P., Nichols D. K. (1999). Batrachochytrium dendrobatidis gen. et sp. nov., a chytrid pathogenic to amphibians. Mycologia 91, 219–227. doi: 10.1080/00275514.1999.12061011
Maniero G. D., Carey C. (1997). Changes in selected aspects of immune function in the leopard frog, Rana pipiens, associated with exposure to cold. J. Comp. Physiol. B 167, 256–263. doi: 10.1007/s003600050072
Martel A., Spitzen-van der Sluijs A., Blooi M., Bert W., Ducatelle R., Fisher M. C., et al. (2013). Batrachochytrium salamandrivorans sp. nov. causes lethal chytridiomycosis in amphibians. Proc. Natl. Acad. Sci. 110, 15325–15329. doi: 10.1073/pnas.1307356110
Martin L. B., Hawley D. M., Ardia D. R. (2011). An introduction to ecological immunology. Funct. Ecol. 25, 1–4. doi: 10.1111/j.1365-2435.2010.01820.x
Moretti E. H., Titon S. C. M., Junior B. T., Marques F. S., Gomes F. R. (2019). Thermal sensitivity of innate immune response in three species of Rhinella toads. Comp. Biochem. Physiol. Part A: Mol. Integr. Physiol. 237, 110542. doi: 10.1016/j.cbpa.2019.110542
Murphy K., Weaver C. (2016). Janeway's immunobiology (New York City, USA: Garland science). doi: 10.1201/9781315533247
Parham P. (2014). The immune system (New York City, USA: Garland Science). doi: 10.1201/9781317511571
Patin E. C., Thompson A., Orr S. J. (2019). “Pattern recognition receptors in fungal immunity,” in Seminars in cell & developmental biology (Academic Press), 24–33. doi: 10.1016/j.semcdb.2018.03.003
Raffel T. R., Rohr J. R., Kiesecker J. M., Hudson P. J. (2006). Negative effects of changing temperature on amphibian immunity under field conditions. Funct. Ecol., 819–828. doi: 10.1111/j.1365-2435.2006.01159.x
Rodriguez K. M., Voyles J. (2020). The amphibian complement system and chytridiomycosis. J Exp. Zool. A Ecol. Integr. Physiol. 333 (10), 706–719. doi: 10.1002/jez.2419
Rollins-Smith L. A. (2017). Amphibian immunity–stress, disease, and climate change. Dev. Comp. Immunol. 66, 111–119. doi: 10.1016/j.dci.2016.07.002
Rollins-Smith L. A., Ramsey J. P., Pask J. D., Reinert L. K., Woodhams D. C. (2011). Amphibian immune defenses against chytridiomycosis: impacts of changing environments. Integr. Comp. Biol. 51, 552–562. doi: 10.1093/icb/icr095
Rosa G. M., Perez R., Richards L. A., Richards-Zawacki C. L., Smilanich A. M., Reinert L. K., et al. (2022). Seasonality of host immunity in a tropical disease system. Ecosphere 13, e4158. doi: 10.1002/ecs2.4158
Rosenblum E. B., Poorten T. J., Settles M., Murdoch G. K., Robert J., Maddox N., et al. (2009). Genome-wide transcriptional response of Silurana (Xenopus) tropicalis to infection with the deadly chytrid fungus. PloS One 4, e6494. doi: 10.1371/journal.pone.0006494
Ruiz V. L., Robert J. (2023). The amphibian immune system. Philos. Trans. R. Soc. B 378, 20220123. doi: 10.1098/rstb.2022.0123
Rus H., Cudrici C., Niculescu F. (2005). The role of the complement system in innate immunity. Immunologic Res. 33, 103–112. doi: 10.1385/IR:33:2:103
Savage A. E., Terrell K. A., Gratwicke B., Mattheus N. M., Augustine L., Fleischer R. C. (2016). Reduced immune function predicts disease susceptibility in frogs infected with a deadly fungal pathogen. Conserv. Physiol. 4, 11. doi: 10.1093/conphys/cow011
Scheele B. C., Pasmans F., Skerratt L. F., Berger L., Martel A. N., Beukema W., et al. (2019). Amphibian fungal panzootic causes catastrophic and ongoing loss of biodiversity. Science 363, 1459–1463. doi: 10.1126/science.aav0379
Soltis R. D., Hasz D., Morris M. J., Wilson I. D. (1979). The effect of heat inactivation of serum on aggregation of immunoglobulins. Immunology 36, 37.
Sonesson A., Ringstad L., Nordahl E. A., Malmsten M., Mörgelin M., Schmidtchen A. (2007). Antifungal activity of C3a and C3a-derived peptides against Candida. Biochim. Biophys. Acta (BBA)-Biomembranes 1768, 346–353. doi: 10.1016/j.bbamem.2006.10.017
Speth C., Rambach G., Würzner R., Lass-Flörl C. (2008). Complement and fungal pathogens: an update. Mycoses 51, 477–496. doi: 10.1111/j.1439-0507.2008.01597.x
Stone R. C., Johnson D. L. (2002). A note on the effect of nebulization time and pressure on the culturability of Bacillus subtilis and Pseudomonas fluorescens. Aerosol Sci. Technol. 36, 536–539. doi: 10.1080/02786820252883775
Voyles J. (2011). Phenotypic profiling of Batrachochytrium dendrobatidis, a lethal fungal pathogen of amphibians. Fungal Ecol. 4, 196–200. doi: 10.1016/j.funeco.2010.12.003
Voyles J., Johnson L. R., Rohr J., Kelly R., Barron C., Miller D., et al. (2017). Diversity in growth patterns among strains of the lethal fungal pathogen Batrachochytrium dendrobatidis across extended thermal optima. Oecologia 184, 363–373. doi: 10.1007/s00442-017-3866-8
Voyles J., Woodhams D. C., Saenz V., Byrne A. Q., Perez R., Rios-Sotelo G., et al. (2018). Shifts in disease dynamics in a tropical amphibian assemblage are not due to pathogen attenuation. Science 359, 1517–1519. doi: 10.1126/science.aao4806
Voyles J., Young S., Berger L., Campbell C., Voyles W. F., Dinudom A., et al. (2009). Pathogenesis of chytridiomycosis, a cause of catastrophic amphibian declines. Science 326, 582–585. doi: 10.1126/science.1176765
Wake D. B., Vredenburg V. T. (2008). Are we in the midst of the sixth mass extinction? A view from the world of amphibians. Proc. Natl. Acad. Sci. 105, 11466–11473. doi: 10.1073/pnas.0801921105
Wälchli R., Vermeire P. J., Massant J., Arosio P. (2020). Accelerated aggregation studies of monoclonal antibodies: considerations for storage stability. J. Pharm. Sci. 109, 595–602. doi: 10.1016/j.xphs.2019.10.048
Wright K. M., Whitaker B. R. (2001). “Pharmacotherapeutics,” in Amphibian medicine and captive husbandry (Krieger Publishing, Malabar, FL), 318–319.
Wu N. C., Cramp R. L., Ohmer M. E., Franklin C. E. (2019). Epidermal epidemic: unravelling the pathogenesis of chytridiomycosis. J. Exp. Biol. 222, jeb191817. doi: 10.1242/jeb.191817
Keywords: amphibians, Batrachochytrium dendrobatidis, chytridiomycosis, complement system, disease ecology, ecoimmunology, infectious disease
Citation: Pacheco HR, Reynoso JR, Tenneti MM, Rodriguez KM and Voyles J (2024) The complement system and its involvement in inhibition of Batrachochytriym dendrobatidis, a lethal fungal pathogen of amphibians. Front. Amphib. Reptile Sci. 2:1294491. doi: 10.3389/famrs.2024.1294491
Received: 14 September 2023; Accepted: 08 March 2024;
Published: 05 April 2024.
Edited by:
Jacob Kerby, University of South Dakota, United StatesReviewed by:
Yan-Fu Qu, Nanjing Normal University, ChinaJános Ujszegi, Hungarian Academy of Sciences, Hungary
Copyright © 2024 Pacheco, Reynoso, Tenneti, Rodriguez and Voyles. This is an open-access article distributed under the terms of the Creative Commons Attribution License (CC BY). The use, distribution or reproduction in other forums is permitted, provided the original author(s) and the copyright owner(s) are credited and that the original publication in this journal is cited, in accordance with accepted academic practice. No use, distribution or reproduction is permitted which does not comply with these terms.
*Correspondence: Jamie Voyles, jvoyles@unr.edu
†ORCID: Jamie Voyles, orcid.org/0000-0002-0073-5790