Effect of brown and green seaweeds on diet digestibility, ruminal fermentation patterns and enteric methane emissions using the rumen simulation technique
- 1Teagasc Animal and Bioscience Research Department, Teagasc Grange, Meath, Ireland
- 2University of Galway, School of Natural Sciences and Ryan Institute, Galway, Ireland
- 3School of Agricultural and Food Science, University College, Dublin, Ireland
- 4Food BioSciences Department, Teagasc Food Research Centre, Dublin, Ireland
Inclusion of the red seaweed Asparagopsis taxiformis as a feed additive, has led to significant reductions in methane (CH4) production from ruminants. However, dietary supplementation with this seaweed is negatively associated with health and environmental concerns mainly due to its bromoform content, a compound with potential carcinogenic properties. Thus, there is renewed focus on ascertaining the anti-methanogenic potential of locally grown brown and green seaweeds, which typically do not contain bromoform. The objective of this study was to investigate the effects of selected brown and green seaweeds on diet digestibility, ruminal fermentation patterns, total gas (TGP) and CH4 production in vitro, using the rumen simulation technique system. In experiment 1, Pelvetia canaliculata (PEC) was examined. In experiment 2, Cystoseira tamariscifolia (CYT), Bifurcaria bifurcata (BIB), Fucus vesiculosus (FUV), Himanthalia elongata (HIM) and Ulva intestinalis (ULI) were analysed. Ascophyllum nodosum (ASC) was included in both experiments. A diet containing A. taxiformis (ASP1; ASP2) and an unsupplemented diet (CON) were included as positive and negative controls, respectively in both experiments. All seaweeds were included at a rate of 10 g/kg dry matter (DM) into a control diet of 50:50 (w:w) forage:concentrate. The seven brown and green seaweeds assessed failed to affect absolute CH4 emissions or alter fermentation patterns. In experiment 1, seaweed treatment had no effect on diet digestibility, CH4%, CH4 mmol/d or CH4 L/d (P>0.1), however ASP1 reduced CH4 mmol/g DOM by 49% (P<0.01) relative to the control. Both ASC and ASP1 tended to increase TGP (P<0.1) relative to the control. In addition to this, the inclusion of seaweed in experiment 1 reduced the production of NH3-N (P<.0001) compared to the control. In experiment 2, seaweed treatment had no effect on diet digestibility or TGP. Both ASP2 and FUV reduced CH4% (P<0.01) but only ASP2 significantly reduced CH4 mmol/d, CH4 L/d and CH4 mmol/g DOM (P<0.05). Daily mMol butyrate was reduced by ASP2 relative to the control and most other seaweeds (P<.0001). In both experiment 1 and 2, seaweed inclusion had no effect on daily total VFA, acetate or propionate production or the acetate:propionate ratio relative to the control. To conclude, including the bromoform-free brown and green seaweeds at 10g/kg DM has no negative effects on diet digestibility or fermentation patterns but also failed to reduce the production of enteric CH4 in vitro.
1. Introduction
Agriculture accounts for 37.1 and 12.4% of Irish and European greenhouse gas (GHG) emissions respectively (DECC, 2021). Methane (CH4) is a potent GHG which has a global warming potential 28 times that of CO2 and is responsible for 40-46% of global agricultural emissions (Searchinger et al., 2021). The majority of CH4 derived from agriculture is produced from ruminal fermentation during the digestion of feed in cattle and sheep (enteric CH4) predominantly, with the remainder (~10%) produced from stored manure and slurries (Epa, 2020). Therefore, in order to reduce the effects of global warming, it is necessary to reduce GHGs from all sectors, with a particular focus on ruminant production in the agricultural sector.
The European Green Deal sets out the European Union’s ambition to reach climate neutrality and to reduce GHGs by at least 55% by 2050 (compared to 1990 levels), with a more recent assessment proposing to reach these targets by 2030. The Paris Agreement, a legally binding international treaty, has also set out the objective to limit global warming to well below 2°C, and preferably to 1.5°C. As a result, there has been a recent proliferation of research on the development of strategies to mitigate methane emissions from agriculture. One of the most promising approaches to achieving these targets in the shorter terms is the development and validation of anti-methanogenic feed additives to significantly reduce enteric CH4 emissions.
The use of seaweed supplementation as an approach to reduce ruminal enteric CH4 has been on-going for over a decade in vitro with a particular emphasis on Asparagopsis taxiformis, however their assessment in animal studies has only emerged in recent years. In general, the tropical, red seaweed Asparagopsis spp., has been shown to have anti-methanogenic properties in studies conducted in vitro (Kinley et al., 2016) and with sheep (Li et al., 2016), beef (Roque et al., 2021), and dairy cattle (Roque et al., 2019). The anti-methanogenic properties of A. taxiformis and other red seaweeds is thought to be mainly due to their relatively high content of the halogenated compound, bromoform, which can inhibit the cobamide-dependent methyl transferase step of the methanogenic pathway and hence block the production of CH4 (Roque et al., 2019). However, bromoform, a known carcinogen (Vaskoska, 2021), has been associated with ongoing health and environmental concerns (Abbott et al., 2020) even when included at low doses: addition of 67g DM A. taxiformis (84.42 µg bromoform) resulted in rumenitis and residues in both urine and milk (10 and 9.1 µg bromoform, respectively) after day 1 of feeding A. taxiformis (Muizelaar et al., 2021). The health and residue issues of bromoform are stated with a point of caution, there are still very few studies published which define the long-term effects of feeding bromoform rich seaweed on animal productivity, animal health and residue deposition in milk and/or meat.
Given the aforementioned potential of red seaweeds to induce both animal and human disease, the lack of A. taxiformis in European waters and the cost of it, there is growing interest in the development of sustainably sourced, non-bromoform containing seaweed as an alternative for reducing enteric CH4 in ruminants. As a result, the application of brown and green seaweeds that are abundant on European shorelines, with promising anti-methanogenic properties are of increasing interest (Vijn et al., 2020; Abbott et al., 2020) for the successful utilisation of seaweed supplementation as a methane mitigation strategy in Ireland and in Europe. Phlorotannin (PT) containing brown seaweeds (Li et al., 2011), have shown to reduce total gas production (TGP) and hence CH4 (Belanche et al., 2016b) and increased protein metabolism (Mueller-Harvey, 2006) but are also associated with anti-nutritional effects when included at levels >5% of ruminant’s daily dry matter intake (DMI) (Abbott et al., 2020). The level of PT in brown seaweeds varies significantly both within and between species, as a consequence of biotic and abiotic factors and can range from 0.5-20% of the algal DW. Phlorotannin content of the seaweeds used in the current study vary from 0 – 321 mg/g DM (Table 2) therefore a variation in anti-methanogenic potential was expected.
Abbott et al. (2020) reported Ascophyllum nodosum, Fucus vesiculosus and Cystoseira tamariscifolia to contain 34.9, 42.3 and 815.82 mg PT/kg, respectively. Tannins are hypothesised to reduce the Tannins are hypothesised to reduce the archaeal and protozoal populations in the rumen archaeal and protozoal populations in the rumen (Piñeiro-Vázquez et al., 2015), as well as some fibre digesting, hydrogen (H2) producing cellulolytic bacteria (Fagundes et al., 2020), and therefore reduce methanogenesis. While green seaweeds contain phenolics, chlorophyll and carotenoids, which are all free radical scavengers (Tierney et al., 2010), their anti-methanogenic properties are yet to be determined.
The rumen simulation technique (RUSITEC) system designed by Czerkawski and Breckenridge (1977) has been used to assess dietary feed additives such as tannins, essential oils, plant secondary metabolites (Tedeschi et al., 2022), and seaweeds (Belanche et al., 2016a) on total gas output, CH4 production, digestibility parameters (Smith et al., 2020), and volatile fatty acid (VFA) production (Castro-Montoya et al., 2012). This system is an ideal example of both the reduction and refinement concepts for animal science experimentation, whereby feed additives can be screened for their anti-methanogenic properties prior to being brought forward to in vivo animal experiments, which can be expensive, time consuming and labour intensive.
Mccauley et al. (2020) concluded that future research on algal derived feed additives should focus on screening more species and evaluating different inclusion levels whilst subsequently ensuring that effects are sustained over longer periods of time. The authors hypothesised that quantification and stabilisation of bioactive components whether it be bromoform or PT in red and brown seaweeds is also paramount to the anti-methanogenic potential of seaweeds. Therefore, the objective of this study was to investigate the effects of selected brown and green seaweeds on diet digestibility, ruminal fermentation patterns and, total gas and CH4 production, using the RUSITEC continuous culture fermentation system.
2. Materials and methods
2.1. Experimental licensing
The experiment was conducted at the Teagasc Grange Animal and Bioscience Research Department, Co. Meath, Ireland (Longitude 6˚ 40' W; Latitude 53˚ 30’ N). Rumen fluid was harvested from four cannulated lactating Holstein-Friesian cows at Teagasc Moorepark Animal and Grassland Research and Innovation Centre, Co. Cork, Ireland (experiment 1) and four cannulated Aberdeen Angus × Friesian steers at Teagasc Grange Animal and Bioscience Research Department, Co. Meath (experiment 2). Animals utilised for the collection of rumen fluid and digesta were cared for in accordance with the Teagasc Animal Ethics Committee. All procedures were approved by the Health Product Regulatory Authority (AE19132/P026) and (AE19132/P113), conducted under the European Directive 2010/63/EU and S.I. No. 543 of 2012.
2.2. Apparatus and experimental design
Four RUSITEC systems (Sanshin Industrial Co. Ltd., Yokohama, Japan) were used to simulate the rumen environment, each of which incorporated eight fermentation vessels. Each vessel had an effective volume of 850 ml with the apparatus, operation and general incubation procedure as described by Czerkawski and Breckenridge (1977). Vessels were incubated in a water bath maintained at 39°C using a water heater under continuous vertical agitation. The displaced effluent and fermentation gasses from each vessel were collected in 1 L effluent bottles and 5 L reusable polyethylene gas bags, respectively. The study consisted of two separate 21-day experiments (14 days for microbial adaptation and fermentation stabilisation, and seven days for sample recording); each experimental treatment consisted of four replicates blocked by machine and randomly allocated within block to a fermentation vessel. Each individual vessel was considered an experimental unit.
2.3. Experimental diets and rumen inoculum
A number of brown and green seaweeds were supplied by the Marine Functional Foods Research Initiative (NutraMara project). The project (Grant-Aid Agreement No. MFFRI/07/01) was carried out under the Sea Change Strategy with the support of the Irish Marine Institute and the Department of Agriculture, Food and the Marine, funded under the National Development Plan 2007–2013. The seaweeds were then selected for the current study as they were representative of the seaweeds found off the Irish coastline. Authors hypothesised that Ascophyllum nodosum (ASC) would reduce CH4 production in vitro and due to its abundance on European shorelines, it was included in both experiments to produce repeatable results. Seaweed names, abbreviations, pigmentation, origin and season of harvest are presented in Table 1. In experiment 1, A. taxiformis (ASP1), ASC and Pelvetia canaliculata (PEC) were included at a rate of 10g/kg DM. In experiment 2, A. taxiformis (ASP2), ASC, C. tamariscifolia (CYT), Bifurcaria bifurcata (BIB), F. vesiculosus (FUV), Himanthalia elongata (HIM) and Ulva intestinalis (ULI) were included at a rate of 10 g/kg DM. Both ASP samples were harvested from the Azores, Portugal, at different seasons. All seaweeds were rinsed immediately post-harvest, ASP2 was stored at -20°C and freeze dried and milled immediately prior to the experiment, all other seaweeds were freeze dried and milled directly post-harvest and stored at -80°C. Donor animals were offered a 50:50 grass silage (GS) (21% DM, 12% CP) to concentrate diet (86% DM, 16% CP), on a DM basis, for three weeks prior to the start of each experiment. The in vitro experimental base diet was formulated similarly. The basal diet was weighed and added to nylon bags (ANKOM TM Technology, Macedon, NY, USA) each day, consisting of 10g of dried GS (100-µm pore size, 10 x 20 cm) and 10g of dried and milled concentrate feed plus the inclusion of the respective experimental seaweed (100-µm pore size; 5 x 10 cm). The GS used in the fermenters was dried for 48 hours at 55°C. Concentrates were dried at 55°C and ground to pass through a 1mm2 sieve size. The seaweeds utilised were freeze dried and ground using a pestle and mortar.
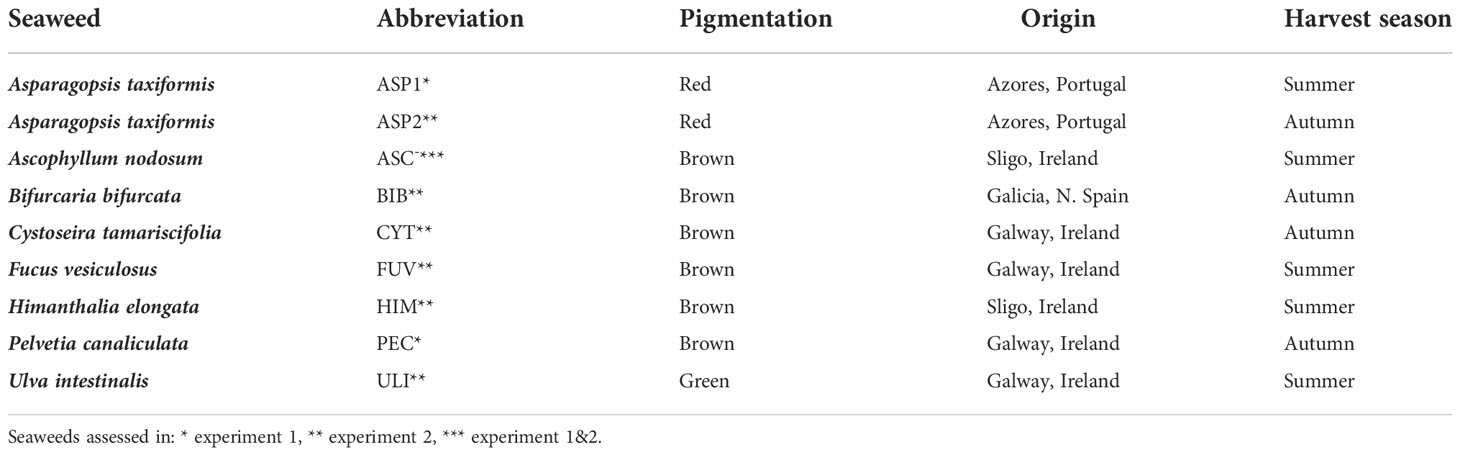
Table 1 Abbreviations, pigmentation, origin and harvest season of seaweeds assessed in experiment 1 and 2.
2.4. Experimental procedure
On day 0, rumen fluid and digesta were harvested from four rumen-cannulated animals. Rumen digesta was pooled immediately following collection and rumen fluid was filtered through a 1mm sieve and pooled. Rumen digesta was transferred to the laboratory at 39°C under anaerobic conditions. The fermentation vessels were inoculated with 450 ml rumen fluid and 350 ml anaerobically prepared artificial saliva. Three nylon bags, each containing one of the following (i) 80 g pooled digesta (fresh weight), (ii) 10 g forage DM and (iii) 10 g concentrate DM plus the respective dietary seaweed additive, were added to each vessel. Fermentation vessels were maintained in a warm water bath at 39°C (Thermal Robo TR-1 α, Axel Global), under continuous vertical agitation (6 revolutions per minute). Each vessel was continuously infused with artificial saliva (prepared daily) using a Watson-Marlow 500 series peristaltic pump (Watson-Marlow Fluid Technology Group, Cornwall, United Kingdom), at a rate of 27.5 ml/hr (dilution rate of 4.2%/h) for the duration of the incubation period.
On day one of the experiment, at 0900h (and each day thereafter), fermentation vessels were individually removed from the warm water bath, opened and infused with carbon dioxide (CO2). The pH of the fermentation fluid was recorded. Nylon bags containing digesta and the concentrate/seaweed diet were discarded. From day two, nylon bags containing forage, which had been fermented for 48 h and the concentrate/seaweed diet (fermented for 24 h) were removed. Upon removal, each bag was rinsed with 25 ml artificial saliva and the liquid fraction of the washings was returned to the vessel along with two new nylon bags, containing 10 g DM GS and 10 g DM concentrates plus seaweed treatment of interest (forage bag from previous day remained). The CO2 flow was removed, vessel sealed and returned to the 39°C water bath. Thus, three feed bags remained in each fermenter at any time. Forage bags remained in the fermentation vessels for 48 hours, concentrate/seaweed bags were fermented for 24 hours. Volume of outflow effluent, gas volume and percentage methane were recorded daily for each vessel. This procedure was repeated each day for the 14 day acclimatisation period.
2.5. Diet digestibility, rumen fermentation and methane production
On days 15-21, nutrient disappearance, total gas volume, CH4%, pH of fermentation fluid, and pH and volume of outflow fermentation effluent were measured. After removal from fermenters, the nylon bags were submerged in an ice bath followed by a 30 minute, detergent free, cold rinse, to remove loosely attached microbiota. Bags containing feed residues were dried in a 55°C forced air oven for 48 hours and hot-weighed, the dried hot weight was taken from the initial undigested weight to determine DM disappearance. Contents were then ground (1 mm sieve) for subsequent nutrient disappearance analysis. Disappearance of organic matter (OM), CP and neutral detergent fibre (NDF) was determined as the difference between the amount of the components in the substrate before incubation and the amount remaining in the residue after incubation. Fermentation gases were collected in reusable polyethylene bags, each morning total gas volume was measured via manual expulsion through the dual diaphragm DC-1A dry test gas meter (Sinagawa Corp. Tokyo, Japan) and CH4% was estimated using the Guardian NG Gas Monitor (Edinburgh Instruments Ltd, Livingston, UK). Daily calibration of the Guardian NG Gas Monitor was conducted with a 10% methane span gas.
2.6. Chemical analysis and ammonia and VFA production
The DM content (g/kg) of the basal and experimental diets (silage, concentrates, seaweeds) (Table 2) and feed residue samples (Tables 3, 4) were determined after drying samples at 55°C for 48 h in a forced air oven. Dried feed samples were ground in Foss™ CT 293 Cyclotec™ General Purpose Sample Mill fitted with a 1 mm screen for subsequent chemical analysis (Foss, Nils Foss Allé 1, DK-3400 Hilleroed, Denmark). Ash concentrations (g/kg DM) were determined by complete combustion in a muffle furnace (Nabertherm, GmbH, Lilienthal, Germany) at 550°C for 5 h. Concentration of CP (g/kg DM) for all samples except the seaweed was determined by obtaining the nitrogen concentration (g/kg DM) of the feed and residue samples using a LECO FP 528 instrument (Leco Instruments UK ltd., Stockport, UK) and then multiplying this figure by a nitrogen-protein conversion factor of 6.25 (Mariotti et al., 2008). For the seaweed, the same protocol was used but the nitrogen-protein conversion factor was determined for each individual seaweed as described by Biancarosa et al. (2017). Determination of NDF was conducted using the ANKOM220 Fibre Analyzer (ANKOM Technology, Macedon, NY, United States) as described by Van Soest et al. (1991). The forage and concentrate residue samples were analysed for aNDFom (NDF) using sodium sulphite and a heat stable amylase was included with the concentrate samples to help remove starch. NDF was expressed exclusive of residual ash.
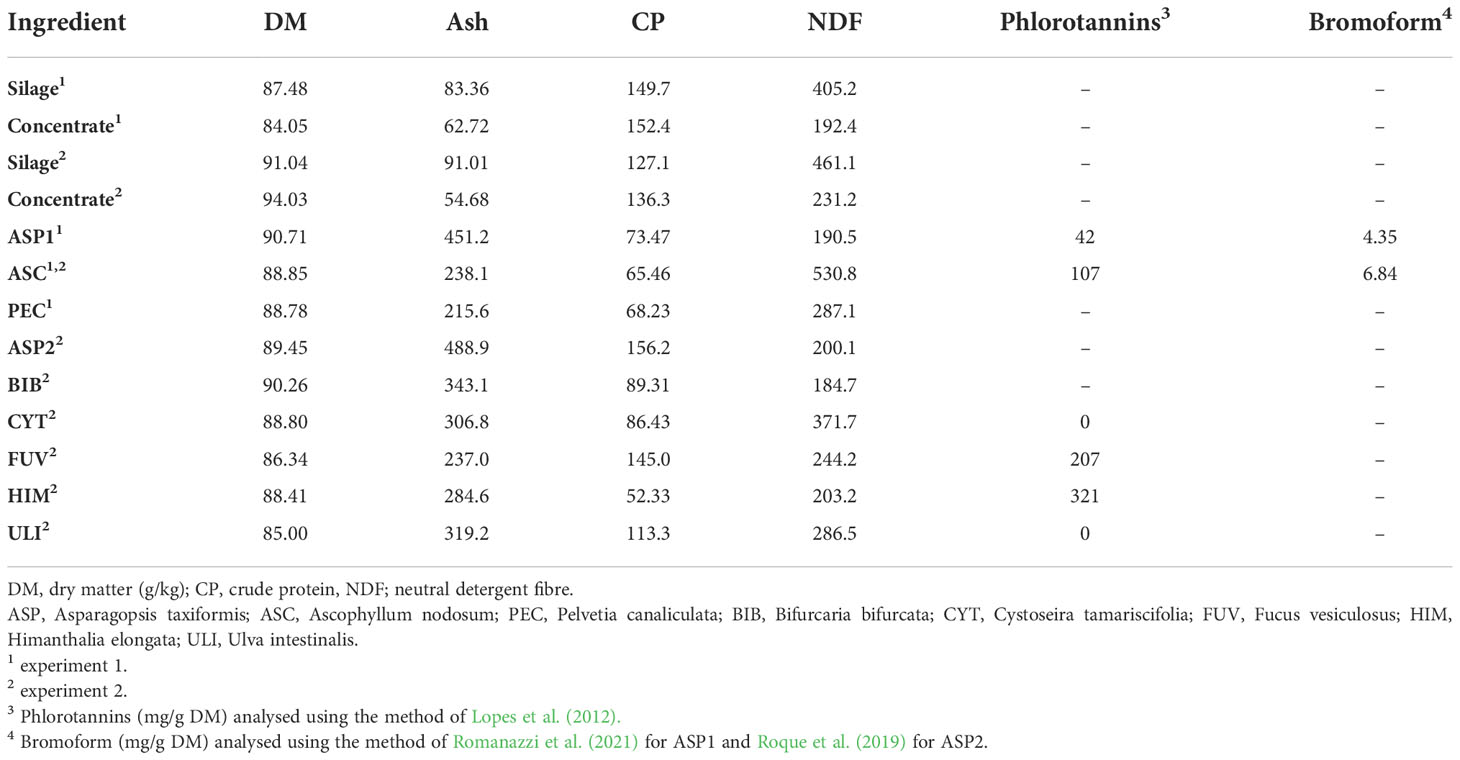
Table 2 Chemical composition of seaweed species and basal diet components used in experiment 1 and 2.
Outflow effluent was collected in individually labelled 1 L glass jars, which were submerged in a 2°C water bath to halt the fermentation process during the 24 hr collection period. During the measurement period, on days 16, 17, 18 and 19, a 4 ml sample of outflow effluent was collected using an automatic pipette and added to 1 ml 50% trichloroacetic acid (TCA). The samples were stored at -20°C for subsequent VFA and ammonia N (NH3-N) analysis. Samples were thawed for 16 h prior to extraction, and centrifuged for 10 minutes (1600g; 4°C). A 100 µL sample of supernatant was drawn off and mixed with 900 µL distilled water (dH2O) for NH3-N analysis which was analysed using the Beckman Coulter AU480 Clinical Analyser (Beckman Coulter, IN, US) and the Thermo Electron Infinity Ammonia Liquid Stable Reagent (Glenbio Ltd, Co. Antrim, UK) kinetic method as described by Owens et al. (2008). For VFA analysis, 250 µl of the supernatant, 3.75 ml dH2O and 1 ml of internal standard (0.5 g 3-metyl-n-valeric acid in one litre of 0.15 M oxalic acid) were transferred to a test tube (16x100mm). The diluted VFA sample was then centrifuged for 5 minutes (260g; 21°C) followed by filtration through a 0.45 µm filter (Cronus Syringe filter PTFE 13 mm; SMI-LabHut Ltd., Maisemore, Gloucester, UK) into three separate 4 ml GC vials (Thermo Scientific, Langerwehe, Germany), each sample was analysed in triplicate. The concentration of VFA was measured using an automated Agilent 450-GC (Agilent Technologies, Santa Clara, Canada) fitted with flame ionisation detector (Ranfft, 1973). Metabolic hydrogen [H] produced, incorporated and recovered was calculated based on VFA production, using the method of Ungerfeld et al. (2015).
2.7. Statistical analysis
Data were checked for normality and homogeneity of variance using qqplots, histograms and formal statistical tests as part of the UNIVARIATE procedure of SAS (version 9.4; SAS Institute). Data that were not normally distributed were transformed by raising the variable to the power of lambda. The appropriate lambda value was obtained by conducting a Box-Cox transformation analysis using the TRANSREG procedure of SAS. Data were analysed using a repeated measures mixed models ANOVA (PROC MIXED). Fixed effects in the model included treatment and machine. Vessel was included as a random effect, and day was included as a covariate. Differences between treatment means were determined by F-tests using Type 3 Tests sums of squares. The covariance structure with the lowest BIC was used. The PDIFF command incorporating the Tukey test was applied to evaluate pairwise comparisons between treatment means. Statistically significant means were considered when P<0.05, while a P<0.1 was considered a tendency towards statistical significance.
3. Results
3.1. Diet digestibility
The disappearance of OM, DM, CP and NDF for experiment 1 and experiment 2 are presented in Tables 3 and 4, respectively. Relative to an unsupplemented control diet, including seaweed at 1% of dietary DM had no effect (P>0.1) on nutrient disappearance (OM, DM, CP or NDF) in either Experiment 1 or 2.
3.2. Fermentation variables
Fermentation variables for experiment 1 are outlined in Table 5. Ruminal pH was affected by seaweed treatment (P<0.05), whereby both ASC and PEC tended to depress pH relative to the control (P<0.1). Inclusion of seaweed reduced the production of NH3-N mg/L relative to the control, whereby all treatments (ASP1, ASC and PEC) reduced NH3-N production. Seaweed inclusion did not affect daily mmol of total VFA (TVFA), acetate or propionate production (P>0.1). Inclusion of ASP1 (P<0.1) decreased production of butyrate relative to ASC and PEC. With regard to the ratio of A:P, no seaweed supplemented in experiment 1 had an effect on the proportions of acetate and propionate produced (P>0.1).
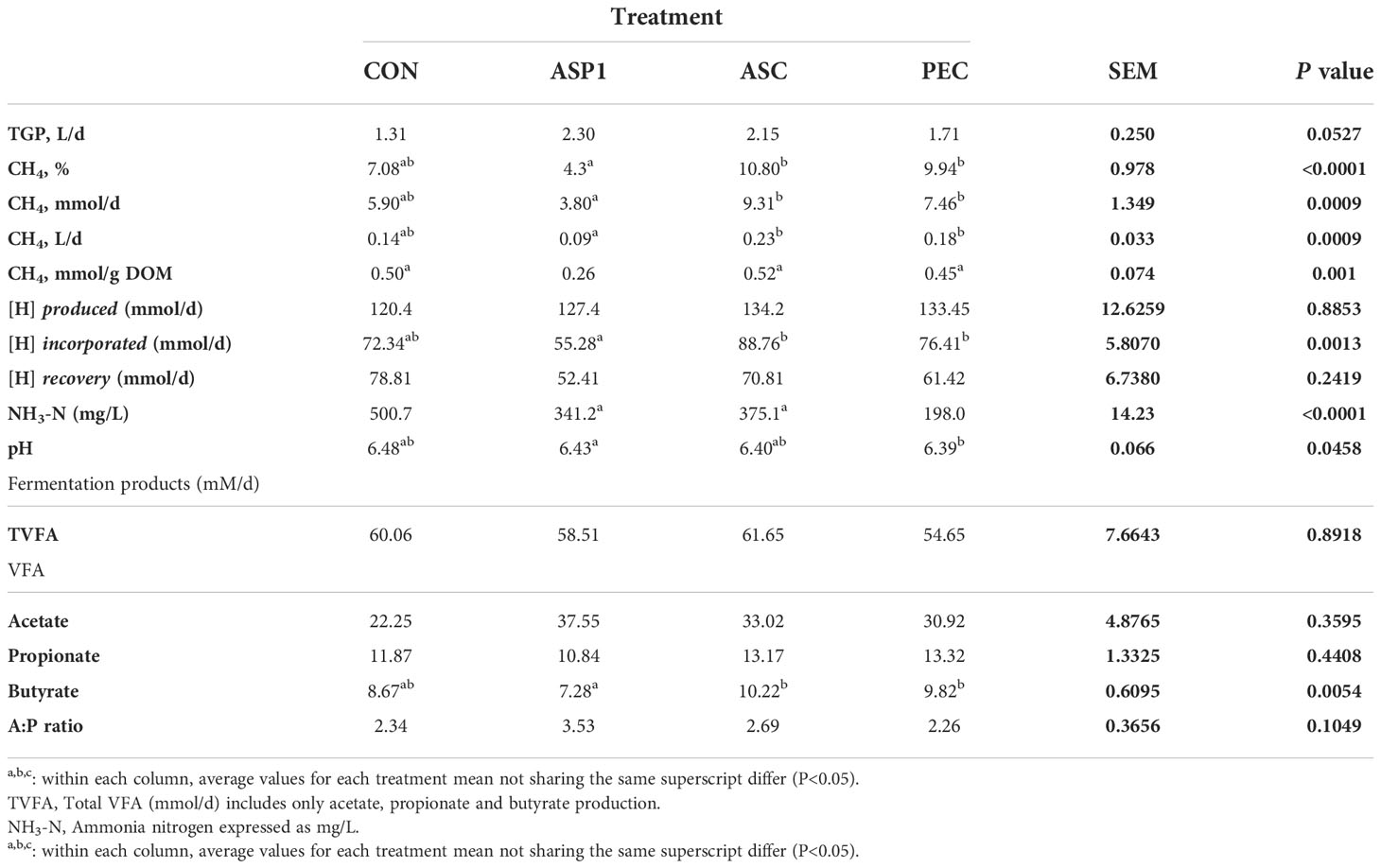
Table 5 Effects of seaweed inclusion on gas production parameters and fermentation patterns in the RUSITEC system (Experiment 1).
Mean TVFA, acetate, propionate and butyrate (mmol/d), A:P ratio, NH3-N (mg/L) and vessel pH for experiment 2 are presented in Table 6. Vessel pH significantly differed between diets (P<0.001), CYT had a lower pH than the control, as well as FUV and BIB (P<0.05). The only treatment to reduce NH3-N relative to the control was ASP2 (P<0.1), by 45%. The inclusion of ASP2 reduced daily TVFA production compared to some of the other seaweed treatments (ASC, CYT, HIM, ULI) (P<0.05), but there was no difference relative to the unsupplemented control (P>0.1). In addition to this, ASP2 reduced daily acetate production relative to all seaweeds except for FUV, and reduced the A:P ratio compared to one brown seaweed, HIM (P<0.01). There was no difference between the control and seaweed supplemented treatments for propionate production (P>0.1), however ASP2 reduced butyrate production compared to the control and all other seaweed treatments, except for FUV (P<0.05).
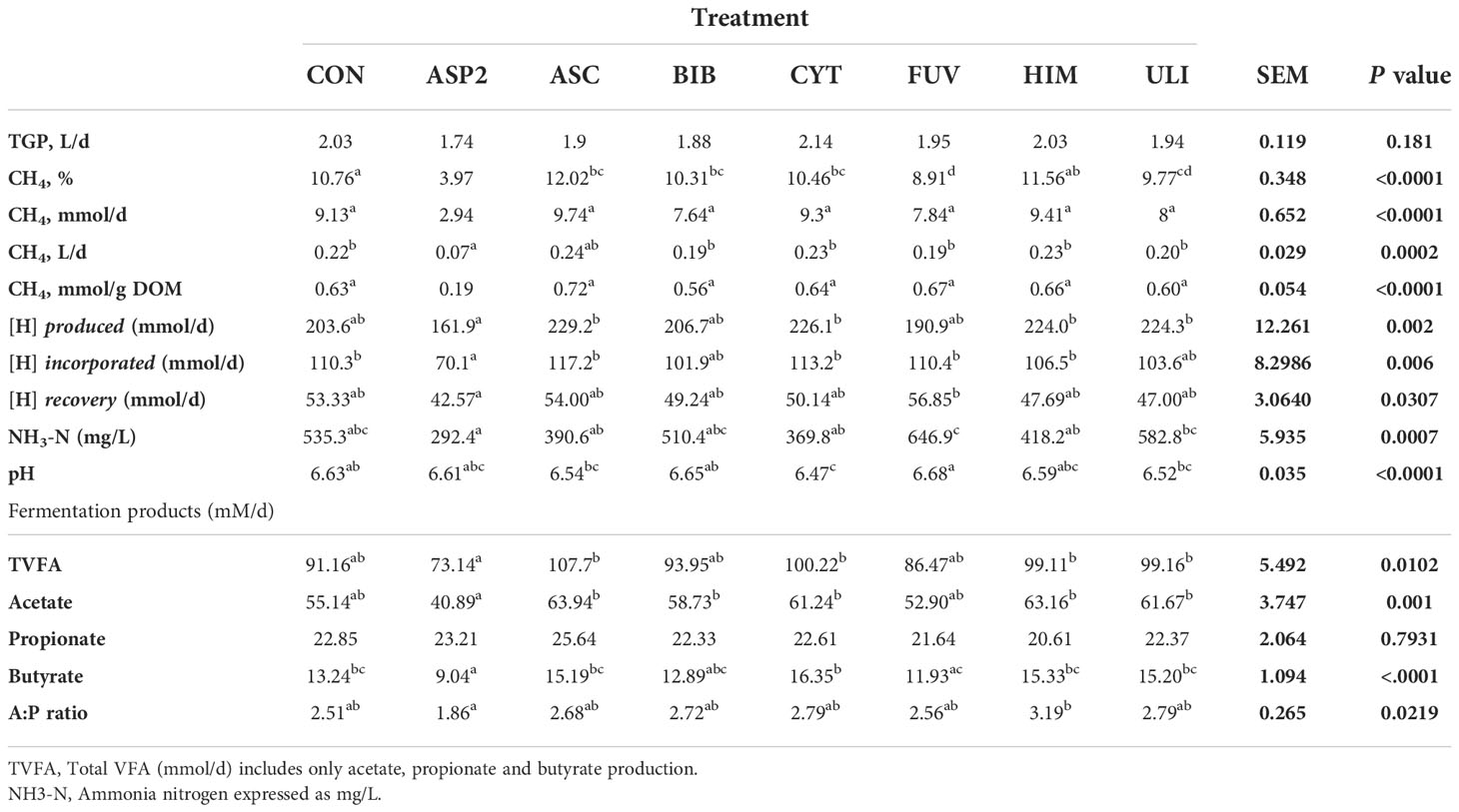
Table 6 Effects of seaweed inclusion on gas production parameters and fermentation patterns in the RUSITEC system (Experiment 2).
3.3. Total gas and methane parameters
Mean coefficients for the effect of seaweed treatment on total gas (L/d), CH4% of total gas, CH4 mmol/day, CH4 volume (L/d), and CH4 mmol/g digestible organic matter (DOM), and metabolic hydrogen produced, incorporated and recovered during the fermentation process for experiment 1 are presented in Table 5. The inclusion of seaweed treatment tended to affect daily gas production (P=0.05) and significantly affected all CH4 parameters (P<0.001) although no differences were observed between seaweed treatment and the unsupplemented control. Both ASP1 and ASC increased TGP relative to control (P<0.01) but had no effect on CH4%, CH4 volume or CH4 mmol/d. With regard to CH4 mmol g/DOM, ASP1 resulted in a reduction of 49% (P<0.01) relative to the control. Inclusion of 1% seaweed relative to an unsupplemented control had no effect on metabolic hydrogen produced or recovered, however there was less metabolic hydrogen incorporated in the vessels containing ASP1 compared to those containing ASC and PEC (P<0.05).
Mean figures for total gas (L/d) and methane parameters (%, L/d, mmol/d, mmol/g DOM) for experiment 2 are presented in Table 6. Inclusion of seaweed had a statistically significant effect on all methane parameters (P<0.001) whilst having no effect on TGP (P>0.1). ASP2 was the only seaweed treatment to reduce all methane parameters (CH4%, P<0.001; CH4 mmol/d, P<0.001; CH4 L/d, P<0.05; CH4 mmol/g DOM, P<0.01) relative to the control and all other dietary treatments. ASC increased CH4% relative to the control and all seaweed treatments (P<0.01), except for HIM. Other than ASP2, the only other seaweed to reduce CH4% when compared to the unsupplemented control was FUV (P<0.01). No other seaweeds reduced methane emissions relative to the control to a level of statistical significance (P<0.05). The amount of metabolic hydrogen produced, incorporated and recovered (presented in Table 6) was unaffected by experimental diets when compared to the unsupplemented control, however ASP2 produced, incorporated and recovered less hydrogen than some of the other brown and green seaweeds supplemented at 1% (P<0.05).
4. Discussion
4.1. Total gas and methane parameters
The 7 indigenous brown and green seaweeds assessed in the present study; A. nodosum, B. bifurcata, C. tamariscifolia, F. vesiculosus, H. elongata, P. canaliculata and U. intestinalis, failed to reduce absolute methane emissions (mmol CH4/day, CH4 L/day) or positively alter fermentation patterns in vitro. Contrary to expectations, inclusion of ASP1 also failed to reduce CH4%, CH4 mmol/d or CH4 L/d relative to the control although numerical reductions of 41, 36 and 36% were observed, respectively. Consistent with the literature (Kinley et al., 2016; Machado et al., 2016), including ASP2 at a rate of 10 g/kg DM significantly reduced CH4 percentage by 63%, and both CH4 mmol/d and CH4 L/d by 68%. The bromoform content of ASP1 and ASP2 was 4.35 and 6.84 mg/g DM, respectively. Therefore the authors are confident that the differences in anti-methanogenic potential between the two samples was due to the differences in bromoform concentration potentially due to harvesting season and/or method of processing. Both A. taxiformis samples were harvested from The Azores, Portugal, however ASP1 was harvested in summer and ASP2 in autumn. Mata et al. (2012) observed an increase in bromoform content in A. taxiformis biomass cultivated in an environment with increased CO2. Concentrations of CO2 are said to be elevated in winter in the North Atlantic Ocean and surrounding areas (Takahashi et al., 1993), therefore this may explain the higher bromoform level in the autumn harvested sample, ASP2. The other potential differentiating factor between both ASP samples was the method of processing, ASP1 was rinsed then freeze dried post-harvest and stored at -80°C, ASP2 was rinsed, stored at -20°C and freeze dried directly prior to incubation in the RUSITEC. Although storage of the freeze-dried sample at -80°C should preserve the bromoform content, there is more research required into the processing and storage methods of seaweeds to best preserve their bioactive content. The conventional method of processing seaweed is freeze drying, which is said to be effective at retaining the bioactive compounds in seaweeds although for what period of time is unknown (Magnusson et al., 2020). Investigating the most effective method of stabilising bromoform content in A. taxiformis, Magnusson et al. (2020) concluded that the homogenisation and immersion of the fresh seaweed in vegetable oil allowed for the stabilisation of high concentrations of bromoform (20.6 mg/g DW). Furthermore, storage in oil for 12 weeks at 4°C further increased bromoform concentration of the oil (26.1 mg/g DW), however storage at 25°C did not further affect bromoform concentration. Immersion in water followed by storage for 12 weeks resulted in bromoform losses of 40 and 47% respectively compared to the oil mixture. The freeze dried seaweed had an initial bromoform concentration of 16.7 mg/g DW, followed by losses of 37.8, 5.0 and 1.3% post storage for 12 weeks at 25°C, 4°C and -20°C, respectively. Differences in bromoform, and other bioactives (i.e. PT) concentrations can also be due to the harvest season or geographical origin of the sample and highlights the environmental sensitivity of the bioactive components of seaweeds (Makkar et al., 2016; De La Moneda et al., 2019). Some of the brown and green seaweeds assessed in the present study have shown reductions in CH4 at inclusion rates >1% (>10g/kg DM) in vitro; A. nodosum (Wang et al., 2008), P. canaliculata (Molina-Alcaide et al., 2017) although reported results are largely inconsistent, especially with lowering inclusion levels. Out of 13 marine derived seaweeds, Dubois et al. (2013) reported that Cystoseira trinodis yielded the most promising reductions in gas and CH4. The aforementioned species are brown seaweeds, whose PT concentrations can also vary between 0.5-20% of algal DW (Abbott et al., 2020), additionally PT has been quantified to be 34.9 for A. nodosum and 516.24, 815.82 and 288.3 mg/g DM for 3 different Cytoseira spp. (Pavia and Toth, 2000; Lopes et al., 2012) explaining the inconsistent anti-methanogenic capabilities between similar species included at differing rates. Increasing the inclusion rate of the algal species will inevitably increase the concentration of the bioactive component, justifying the greater CH4 reductions observed with elevated seaweed inclusion. Previous published reports have indicated a methane suppressing role for Cystoseira spp. when added to ruminant diets (Dubois et al., 2013; Machado et al., 2014), therefore CYT was selected for this study with the expectation to reduce CH4, the lack of reduction in CH4 production highlights the within species variation of bioactive compounds. The majority of in vitro studies assessing the anti-methanogenic potential of A. taxiformis have proven to be effective at 2% inclusion (Kinley et al., 2016; Machado et al., 2016), conversely brown and green seaweeds to-date have been evaluated in batch culture experiments at inclusion levels >1% (Maia et al., 2016; Belanche et al., 2016b; Molina-Alcaide et al., 2017) therefore the inclusion of seaweeds at 1% in a long term (21 d) RUSITEC experiment has yielded novel results; including the brown and green seaweeds harvested at the location and season specified in Table 1, at 10 g/kg DM had no effect on methane and fermentation parameters whilst A. taxiformis significantly reduced CH4. It is important to mention that the same seaweed species harvested in differing conditions (i.e. time of year, geographical location) could potentially yield different results due to changes in the bioactive concentrations.
The aim of this study was to identify native seaweeds with strong anti-methanogenic characteristics that could potentially be brought forward to animal studies at realistic commercially viable farm-level inclusion rates. Limiting product availability, potential anti-nutritional effects, palatability concerns as well as a lack of an anti-methanogenic effect in previous and the present study highlight the necessity for the identification of minimum effective inclusion rates of brown and green seaweed species. Indeed, A. taxiformis has proven to be effective at inclusion levels even lower than 1% but the tropical nature, harmful bioactive content and cost of the commodity makes it unsuitable for this purpose.
4.2. Diet digestibility
Ensuring that anti-methanogenic dietary feed additives do not have negative effects on substrate digestibility or rumen function is pivotal to the development and validation of the mitigation strategy prior to being assessed in vivo. Total gas production was not significantly affected by any of the seaweed treatments except for experiment one, where ASP1 tended to reduce and ASC tended to increase TGP. High TGP is indicative of proficient ruminal fermentation to support the growth of ruminal microbes (Gemeda and Hassen, 2015) therefore, a significant reduction in TGP could be indicative of a drop in digestion, hence fermentation due to the reduced activity of cellulolytic bacteria from PT, which are present in seaweed.
No effects on disappearances of DM and its chemical constituents were observed in this study, which is a promising finding most likely due to the low inclusion levels of the seaweed treatments, this finding is also consistent with the lack of effect on fermentation parameters. Other studies observed reductions in OM digestibility at inclusion levels >10% (Kinley et al., 2016; Ramin et al., 2019). Brown seaweeds contain varying levels of PTs which can result in enzyme inhibition, particularly α-glucosidase and α-amylase which play a significant role in the digestion of complex carbohydrates (Li et al., 2011) and hence fibre digestion. This would explain the anti-nutritional effect observed when seaweed is included at levels >5% (Abbott et al., 2020). In addition to the aforementioned anti-nutritional effects, these feeding levels are not practical at farm level, due to issues with palatability leading to reduced DMI. No anti-nutritional effects were expected in the present study due to the low inclusion rate of the seaweed treatments, however subsequent studies with higher inclusion rates are warranted to determine whether these species have any potential as CH4 suppressants, nonetheless this should be cautioned on the basis of possible palatability and digestive issues.
4.3. Fermentation parameters
A reduction in ruminal pH is associated with the inhibition of methanogens and hence a reduction in CH4 (Beauchemin et al., 2008), Ellis et al. (2008) stated that at pH<6.5 methanogenesis is reduced and it is almost halted at pH <6.0. In contradiction to this, the only seaweed treatments to reduce ruminal pH below 6.5 in experiment 2 was CYT and there was no observed reducing effect on CH4 production. A simultaneous negative effect on digestibility would indicate that the reduction in pH did in fact hamper the effects of fibre digesting bacteria, which was also not the case. Including A. nodosum at levels up to 5% had no effect on feed disappearance in the RUSITEC (Belanche et al., 2016a) and actually linearly improved digestibility of nutrients in finishing buffaloes (Chaji et al., 2020). Contrastingly, Wang et al. (2008) observed a significant decline in NDF disappearance with increasing dosage rates of PT from the brown seaweed A. nodosum, emphasising the necessity to identify a seaweed species that is effective in sparing quantities. Inclusion of seaweeds ASP1, ASC and PEC reduced the production of NH3-N in the outflow effluent relative to the control. Autumn harvested, brown seaweed, PEC was the most effective at reducing NH3-N. The authors hypothesise that the reduction in NH3-N is due to differences in overall CP content, the CP content of PEC in the current study was 68.23 g/kg DM (second lowest of all seaweeds assessed), in line with this, Molina-Alcaide et al. (2017) reported the CP content of autumn harvested P. canaliculata to be 75 g/kg DM. In addition to this, out of a range of brown, green and red seaweeds harvested in spring and autumn, Molina-Alcaide et al. (2017) reported autumn harvested P. canaliculata to have the lowest concentrations of NH3-N after 24 h in vitro incubation. Reductions in enteric CH4 have been linked with a significant shift in the A:P ratio (Li et al., 2016; Machado et al., 2016), however the shift in A:P was not significant in the present study potentially due to the insignificant reduction in CH4. Regarding fermentation parameters of the brown seaweeds in the experiment, ASC inclusion failed to have an effect on VFA production, similar findings have been previously reported (Belanche et al., 2016b). The inclusion of ASP1 and ASP2 had no significant reduction on TVFA production compared to the unsupplemented control, which is consistent with findings from Kinley et al. (2016), but contrary to that of Machado et al. (2016) who observed a significant reduction in TVFA when including A. taxiformis at a rate as low as 0.5% up to 16.7%, the authors hypothesise that the conflicting TVFA results at similar A. taxiformis inclusion rates are due to differences in the bromoform content, however the bromoform contents are not quantified in the aforementioned studies to confirm this. Inclusion of A. taxiformis did not affect propionate production, relative to other treatments, highlighting the importance of quantifying the bioactive content of each seaweed sample prior to assessing its efficacy. Increasing concentration of propionate is strongly associated with decreasing CH4 production. Decreasing A:P ratios have been observed in vitro and in vivo using anti-methanogenic additives including seaweeds, which may be indicative of the excess H2 being sequestered for propionate production (Roque et al., 2021).
4.4. Potential of seaweed as an anti-methanogenic technology
There are over 600 seaweed species native to Ireland, ~ 45% of which have brown and green pigmentation (Guiry, 1996). Seaweed is a very low DM (10-20%) commodity, hence bulky to transport which stresses the importance of identifying indigenous seaweeds with anti-methanogenic properties, which can in-turn be locally farmed and thus reduce the carbon footprint and indirect emissions of transporting it across the globe, generating a more sustainable product. Furthermore, Vijn et al. (2020) calculated that supplementing the 93 million cattle in the USA with 1% seaweed inclusion, would require 3-3.4 million tonnes of dried seaweed. To-date, only Asparagopsis spp. has been proven at this low inclusion rate, hence the successful adoption of seaweed supplementation at farm-level would require a vast surge in global seaweed production. The effects of further intensifying seaweed production have not been fully described yet. Benefits of mass seaweed production could include carbon sequestration, removal of excess nutrients in eutrophied waters and the provision of a rich habitat for marine animals. Simultaneously, the artificial growing environment could cause marine mammal entanglement and escaped lines after storm damage (Vijn et al., 2020).
There is still a dearth of information on the environmental and seasonal variation of seaweed composition. It is known that the bioactive compound bromoform is affected by processing technique and storage (Magnusson et al., 2020). Phlorotannin concentration in brown seaweeds is also affected by processing and storage; non-washed freeze dried seaweed yield highest PT concentrations. The stabilisation of PT is necessary, storing dried PT containing seaweed at low temperatures (4°C), out of direct sunlight, in air-tight containers will preserve the PT content. Storage time also depletes the PT concentration, with research into encapsulating the active ingredient to extend its shelf-life (Cassani et al., 2020).
The utility of seaweed as a ruminant feedstuff is impacted by the composition of the biomass which is affected by a multitude of inherent (species, growth rate, habitat) and external (temperature, light, nutrient availability) factors (Roque et al., 2019). The variation in CH4 mitigation between both A. taxiformis samples is likely due to the amount of bromoform that has escaped from the biomass during storage prior to the experiment. The chemical composition of seaweeds is also affected by geographical location and environment. Harvest locations of the seaweeds utilised in this experiment are described in Table 1, most of which came from Irish shore lines, except for ASP1 and ASP2 (Azores, Portugal) and BIB (Spain). As previously mentioned, the bioactives bromoform and PT are the ingredients that hamper the methanogenesis pathway, but the concentration of these actives fluctuates within seaweed types and species. Kinley et al. (2020) and Roque et al. (2019) found the bromoform content of A. taxiformis and Asparagopsis armata to be 6.55 and 1.32 mg/g, respectively. In a batch culture experiment, De La Moneda et al. (2019) harvested eight Norwegian seaweeds both in spring and in autumn and assessed their seasonal variation. This study found harvesting season to be significant, as seaweed harvested in spring had greater ash, nitrogen and total extractable polyphenol content as well as a reduction in gas production parameters in comparison to autumn harvested seaweed but had no effect on VFA production in vitro. This seasonal variation is backed up by Mata et al. (2012), who observed an increase in nutrient content in water towards spring, which increased production of seaweed biomass. Furthermore, environmental interactions from integrated aquaculture leading to increasing concentrations of dissolved inorganic carbon and total NH3-N in the water significantly increase the bromoform content of A. taxiformis.
As previously mentioned, bromoform and other halogenated compounds are the active anti-methanogenic component of many red seaweed species. Offering A. taxiformis containing a predetermined amount (1.26 mg/kg DM) of bromoform to lactating Holstein dairy cows at three inclusion rates (67, 133 and 333 g/day), Muizelaar et al. (2021) found that bromoform caused inflammation to the rumen wall and residues were excreted in urine and milk. Conversely, other researchers (Roque et al., 2019; Stefenoni et al., 2021) have observed no elevation of bromoform in meat, milk, fat or faeces from lactating dairy cows fed A. taxiformis. In addition to this, it has been said that bromoform causes renal and hepatotoxicity in rodents, however the quantities fed to rodents are 183-2890 times higher than that fed to ruminants for CH4 mitigation (Glasson et al., 2022). A point of caution with regards to brown seaweeds is their trace mineral content, particularly their elevated iodine (I) (Abbott et al., 2020). Brown seaweeds will potentially need to be supplemented at higher levels than red seaweeds to achieve a reduction in CH4 which could lead to elevated I levels in the animal and animal products (Antaya et al., 2019) and therefore quantification of I is important when evaluating seaweeds in future studies. In the event of the adoption of seaweed as a strategy to reduce enteric CH4, residue analysis of meat, milk, urine and faeces with reliable detection methods will be required prior to implementation at farm level.
5. Conclusions
The seven indigenous brown and green seaweeds assessed in the present study had no effect on nutrient disappearance, failed to reduce absolute methane emissions or alter fermentation patterns in the artificial rumen. Only one of the two A. taxiformis samples (ASP2) significantly reduced all CH4 parameters, supporting the link between CH4 production and differing levels of bromoform. Therefore, in order to develop seaweed supplementation as a strategy to combat enteric CH4 emissions in agriculture, the anti-methanogenic bioactive in seaweeds need to be characterised and quantified which will give a clear indication of the product’s potential to reduce methane. In addition to this, more indigenous marine derived seaweed need to be screened to determine their concentration of anti-methanogenic biological compounds. Finally, the anti-methanogenic properties of these seaweeds at marginally higher inclusion rates should be assessed, followed by subsequent in vivo research to confirm their efficacy.
Data availability statement
The raw data supporting the conclusions of this article will be made available by the authors, without undue reservation.
Author contributions
ER, SW, SK and DK designed the study. MH provided the seaweed treatments for the experiments. ER, SK and CD conducted the experiments and collected all the data. ER and CD prepared the samples for VFA and NH3-N analysis, which were analysed in the lab of VF at NUIG and at Teagasc Grange. ER collabrated with DK on the statistical analysis of the data set. Results were interpreted by DK, ER, SW and SK. ER drafted the manuscript. SW, DK, MH, SK and VF revised the document. All authors approved the manuscript and agreed for accountability of the work therein. All authors contributed to the article and approved the submitted version.
Funding
This work is part of the METH-ABATE project which is financially supported by the Department of Agriculture, Food and the Marine (DAFM) (RSF 2019R479) and it is also part of the SEASOLUTIONS project, funded through the ERA-NET cofund under Horizon 2020 (696231). ER is funded through the Walsh Scholarship Programme (WS 2019229).
Conflict of interest
The authors declare that the research was conducted in the absence of any commercial or financial relationships that could be construed as a potential conflict of interest.
Publisher’s note
All claims expressed in this article are solely those of the authors and do not necessarily represent those of their affiliated organizations, or those of the publisher, the editors and the reviewers. Any product that may be evaluated in this article, or claim that may be made by its manufacturer, is not guaranteed or endorsed by the publisher.
References
Abbott D. W., Aasen I. M., Beauchemin K. A., Grondahl F., Gruninger R., Hayes M., et al. (2020). Seaweed and seaweed bioactives for mitigation of enteric methane: Challenges and opportunities. Animals 10, 2432. doi: 10.3390/ani10122432
Antaya N. T., Ghelichkhan M., Pereira A. B.D., Soder K. J., Brito A. F. (2019). Production, milk iodine, and nutrient utilization in Jersey cows supplemented with the brown seaweed Ascophyllum nodosum (kelp meal) during the grazing season. Journal of Dairy Science 102(9), 8040–8058.
Beauchemin K., Kreuzer M., O’mara F., Mcallister T. (2008). Nutritional management for enteric methane abatement: A review. Aust. J. Exp. Agric. 48, 21–27. doi: 10.1071/EA07199
Belanche A., Jones E., Parveen I., Newbold C. J. (2016a). A metagenomics approach to evaluate the impact of dietary supplementation with ascophyllum nodosum or laminaria digitata on rumen function in rusitec fermenters. Front. Microbiol. 7, 299. doi: 10.3389/fmicb.2016.00299
Belanche A., Ramos-Morales E., Newbold C. J. (2016b). In vitro screening of natural feed additives from crustaceans, diatoms, seaweeds and plant extracts to manipulate rumen fermentation. J. Sci. Food Agric. 96, 3069–3078. doi: 10.1002/jsfa.7481
Biancarosa I., Espe M., Bruckner C., Heesch S., Liland N., Waagbø R., et al. (2017). Amino acid composition, protein content, and nitrogen-to-protein conversion factors of 21 seaweed species from Norwegian waters. J. Appl. Phycology 29, 1001–1009. doi: 10.1007/s10811-016-0984-3
Cassani L., Gomez-Zavaglia A., Jimenez-Lopez C., Lourenço-Lopes C., Prieto M. A., Simal-Gandara J. (2020). Seaweed-based natural ingredients: Stability of phlorotannins during extraction, storage, passage through the gastrointestinal tract and potential incorporation into functional foods. Food Res. Int. 137, 109676. doi: 10.1016/j.foodres.2020.109676
Castro-Montoya J., De Campeneere S., Van Ranst G., Fievez V. (2012). Interactions between methane mitigation additives and basal substrates on in vitro methane and VFA production. Anim. Feed Sci. Technol. 176, 47–60. doi: 10.1016/j.anifeedsci.2012.07.007
Chaji M., Eslami M., Kordnejad E. (2020). Influence of ascophyllum nodosum algae extract on finishing growth performance and nutrient digestibility of buffalo calves in warm climates. Trop. Anim. Health Production 52, 1335–1343. doi: 10.1007/s11250-019-02138-z
Czerkawski J., Breckenridge G. (1977). Design and development of a long-term rumen simulation technique (Rusitec). Br. J. Nutr. 38, 371–384. doi: 10.1079/BJN19770102
DECC (2021). CLIMATE ACTION PLAN 2021 securing our future 17-19, 154–161. Department of environment, climate and communications. Available at: https://www.gov.ie/en/publication/6223e-climate-action-plan-2021/
De La Moneda A., Carro M. D., Weisbjerg M. R., Roleda M. Y., Lind V., Novoa-Garrido M., et al. (2019). Variability and potential of seaweeds as ingredients of ruminant diets: An in vitro study. Animals 9, 851. doi: 10.3390/ani9100851
Dubois B., Tomkins N. W., Kinley R. D., Bai M., Seymour S., Paul N. A., et al. (2013). Effect of tropical algae as additives on rumen in vitro gas production and fermentation characteristics. Am. J. Plant Sci. 4, 34–43. doi: 10.4236/ajps.2013.412A2005
Ellis J., Dijkstra J., Kebreab E., Bannink A., Odongo N., Mcbride B., et al. (2008). Aspects of rumen microbiology central to mechanistic modelling of methane production in cattle. J. Agric. Sci. 146, 213–233. doi: 10.1017/S0021859608007752
Fagundes G. M., Benetel G., Carriero M. M., Sousa R. L., Muir J. P., Macedo R. O., et al. (2020). Tannin-rich forage as a methane mitigation strategy for cattle and the implications for rumen microbiota. Anim. Production Sci. 61, 26–37. doi: 10.1071/AN19448
Gemeda B. S., Hassen A. (2015). Effect of tannin and species variation on in vitro digestibility, gas, and methane production of tropical browse plants. Asian-Australasian J. Anim. Sci. 28, 188. doi: 10.5713/ajas.14.0325
Glasson C. R., Kinley R. D., De Nys R., King N., Adams S. L., Packer M. A., et al. (2022). Benefits and risks of including the bromoform containing seaweed asparagopsis in feed for the reduction of methane production from ruminants. Algal Res. 64, 102673. doi: 10.1016/j.algal.2022.102673
Guiry M. (1996). AlgaeBase is a global algal database of taxonomic, nomenclatural and distributional information. https://www.algaebase.org/
Kinley R. D., De Nys R., Vucko M. J., Machado L., Tomkins N. W. (2016). The red macroalgae asparagopsis taxiformis is a potent natural antimethanogenic that reduces methane production during in vitro fermentation with rumen fluid. Anim. Production Sci. 56, 282–289. doi: 10.1071/AN15576
Kinley R. D., Martinez-Fernandez G., Matthews M. K., De Nys R., Magnusson M., Tomkins N. W. (2020). Mitigating the carbon footprint and improving productivity of ruminant livestock agriculture using a red seaweed. J. Cleaner Production 259, 120836. doi: 10.1016/j.jclepro.2020.120836
Li X., Norman H. C., Kinley R. D., Laurence M., Wilmot M., Bender H., et al. (2016). Asparagopsis taxiformis decreases enteric methane production from sheep. Anim. Production Sci. 58, 681–688. doi: 10.1071/AN15883
Li Y.-X., Wijesekara I., Li Y., Kim S.-K. (2011). Phlorotannins as bioactive agents from brown algae. Process Biochem. 46, 2219–2224. doi: 10.1016/j.procbio.2011.09.015
Lopes G., Sousa C., Silva L. R., Pinto E., Andrade P. B., Bernardo J., et al. (2012). Can phlorotannins purified extracts constitute a novel pharmacological alternative for microbial infections with associated inflammatory conditions? PloS One 7, e31145. doi: 10.1371/journal.pone.0031145
Machado L., Magnusson M., Paul N. A., De Nys R., Tomkins N. (2014). Effects of marine and freshwater macroalgae on in vitro total gas and methane production. PloS One 9, e85289. doi: 10.1371/journal.pone.0085289
Machado L., Magnusson M., Paul N. A., Kinley R., De Nys R., Tomkins N. (2016). Dose-response effects of asparagopsis taxiformis and oedogonium sp. on in vitro fermentation and methane production. J. Appl. Phycology 28, 1443–1452. doi: 10.1007/s10811-015-0639-9
Magnusson M., Vucko M. J., Neoh T. L., De Nys R. (2020). Using oil immersion to deliver a naturally-derived, stable bromoform product from the red seaweed asparagopsis taxiformis. Algal Res. 51, 102065. doi: 10.1016/j.algal.2020.102065
Maia M. R., Fonseca A. J., Oliveira H. M., Mendonça C., Cabrita A. R. (2016). The potential role of seaweeds in the natural manipulation of rumen fermentation and methane production. Sci. Rep. 6, 1–10. doi: 10.1038/srep32321
Makkar H. P., Tran G., Heuzé V., Giger-Reverdin S., Lessire M., Lebas F., et al. (2016). Seaweeds for livestock diets: A review. Anim. Feed Sci. Technol. 212, 1–17. doi: 10.1016/j.anifeedsci.2015.09.018
Mariotti F., Tomé D., Mirand P. P. (2008). Converting nitrogen into protein–beyond 6.25 and jones' factors. Crit. Rev. Food Sci. Nutr. 48, 177–184. doi: 10.1080/10408390701279749
Mata L., Gaspar H., Santos R. (2012). Carbon/nutrient balance in relation to biomass production and halogenated compound content in the red alga asparagopsis taxiformis (bonnemaisoniaceae) 1. J. phycology 48, 248–253. doi: 10.1111/j.1529-8817.2011.01083.x
Mccauley J. I., Labeeuw L., Jaramillo-Madrid A. C., Nguyen L. N., Nghiem L. D., Chaves A. V., et al. (2020). Management of enteric methanogenesis in ruminants by algal-derived feed additives. Curr. pollut. Rep. 6(3), 1–18. doi: 10.1007/s40726-020-00151-7
Molina-Alcaide E., Carro M. D., Roleda M. Y., Weisbjerg M. R., Lind V., Novoa-Garrido M. (2017). In vitro ruminal fermentation and methane production of different seaweed species. Anim. Feed Sci. Technol. 228, 1–12. doi: 10.1016/j.anifeedsci.2017.03.012
Mueller-Harvey I. (2006). Unravelling the conundrum of tannins in animal nutrition and health. J. Sci. Food Agric. 86, 2010–2037. doi: 10.1002/jsfa.2577
Muizelaar W., Groot M., Van Duinkerken G., Peters R., Dijkstra J. (2021). Safety and transfer study: Transfer of bromoform present in asparagopsis taxiformis to milk and urine of lactating dairy cows. Foods 10, 584. doi: 10.3390/foods10030584
Owens D., Mcgee M., Boland T. (2008). Effect of grass regrowth interval on intake, rumen digestion and nutrient flow to the omasum in beef cattle. Anim. Feed Sci. Technol. 146, 21–41. doi: 10.1016/j.anifeedsci.2007.11.012
Pavia H., Toth G. B. (2000). Inducible chemical resistance to herbivory in the brown seaweed ascophyllum nodosum. Ecology 81, 3212–3225. doi: 10.1890/0012-9658(2000)081[3212:ICRTHI]2.0.CO;2
Piñeiro-Vázquez A., Canul-Solís J., Alayón-Gamboa J., Chay-Canul A., Ayala-Burgos A., Aguilar-Pérez C., et al. (2015). Potential of condensed tannins for the reduction of emissions of enteric methane and their effect on ruminant productivity. Archivos Medicina Veterinaria 47, 263–272. doi: 10.4067/S0301-732X2015000300002
Ramin M., Franco M., Roleda M. Y., Aasen I. M., Hetta M., Steinshamn H. (2019). In vitro evaluation of utilisable crude protein and methane production for a diet in which grass silage was replaced by different levels and fractions of extracted seaweed proteins. Anim. Feed Sci. Technol. 255, 114225. doi: 10.1016/j.anifeedsci.2019.114225
Ranfft K. (1973). Determination by gas chromatography of short chain fatty acids in ruminal fluids. Arch. Tierernahrung 23, 343–352. doi: 10.1080/17450397309420984
Romanazzi D., Sanchez-Garcia C., Svenson J., Mata L., Pes K., Hayman C. M., et al. (2021). Rapid analytical method for the quantification of bromoform in the red seaweeds Asparagopsis armata and Asparagopsis taxiformis using gas chromatography–mass spectrometry. ACS Agricultural Sci. & Technol. 1(5), 436–442.
Roque B. M., Salwen J. K., Kinley R., Kebreab E. (2019). Inclusion of asparagopsis armata in lactating dairy cows’ diet reduces enteric methane emission by over 50 percent. J. Cleaner Production 234, 132–138. doi: 10.1016/j.jclepro.2019.06.193
Roque B. M., Venegas M., Kinley R. D., De Nys R., Duarte T. L., Yang X., et al. (2021). Red seaweed (Asparagopsis taxiformis) supplementation reduces enteric methane by over 80 percent in beef steers. PloS One 16, e0247820. doi: 10.1371/journal.pone.0247820
Searchinger T., Herrero M., Yan X., Wang J., Beauchemin K., Kebreab E. (2021). Opportunities to reduce methane emissions from global agriculture. Available at: https://scholar.princeton.edu/sites/default/files/methane_discussion_paper_nov_2021.pdf
Smith P. E., Waters S. M., Kenny D. A., Boland T. M., Heffernan J., Kelly A. K. (2020). Replacing barley and soybean meal with by-products, in a pasture based diet, alters daily methane output and the rumen microbial community in vitro using the rumen simulation technique (RUSITEC). Front. Microbiol. 11, 1614. doi: 10.3389/fmicb.2020.01614
Stefenoni H., Räisänen S., Cueva S., Wasson D. E., Lage C., Melgar A., et al. (2021). Effects of the macroalga asparagopsis taxiformis and oregano leaves on methane emission, rumen fermentation, and lactational performance of dairy cows. J. dairy Sci. 104, 4157–4173. doi: 10.3168/jds.2020-19686
Takahashi T., Olafsson J., Goddard J. G., Chipman D. W., Sutherland S. (1993). Seasonal variation of CO2 and nutrients in the high-latitude surface oceans: A comparative study. Global Biogeochemical Cycles 7, 843–878. doi: 10.1029/93GB02263
Tedeschi L. O., Abdalla A. L., Álvarez C., Anuga S. W., Arango J., Beauchemin K. A., et al. (2022). Quantification of methane emitted by ruminants: a review of methods. J. Anim. Sci. 100, skac197. doi: 10.1093/jas/skac197
Tierney M. S., Croft A. K., Hayes M. (2010). A review of antihypertensive and antioxidant activities in macroalgae. Botanica Marina. 53, 387–408. doi: 10.1515/bot.2010.044
Ungerfeld E. M. (2015). Shifts in metabolic hydrogen sinks in the methanogenesis-inhibited ruminal fermentation: a meta-analysis. Frontiers in Microbiology 6, 37.
Van Soest P. V., Robertson J., Lewis B. (1991). Methods for dietary fiber, neutral detergent fiber, and nonstarch polysaccharides in relation to animal nutrition. J. dairy Sci. 74, 3583–3597. doi: 10.3168/jds.S0022-0302(91)78551-2
Vaskoska R. S. (2021). “Raising a need for a risk assessment of bromoform transferred from feed to food,” in Food legal bulletin (Lawmedia Pty Ltd). Available at https://www.foodlegal.com.au/inhouse/document/2440?print=true
Vijn S., Compart D. P., Dutta N., Foukis A., Hess M., Hristov A. N., et al. (2020). Key considerations for the use of seaweed to reduce enteric methane emissions from cattle. Front. Veterinary Sci. 7, 1135. doi: 10.3389/fvets.2020.597430
Keywords: methane, RUSITEC, rumen, phlorotannins, bromoform
Citation: Roskam E, Kirwan SF, Kenny DA, O’Donnell C, O’Flaherty V, Hayes M and Waters SM (2022) Effect of brown and green seaweeds on diet digestibility, ruminal fermentation patterns and enteric methane emissions using the rumen simulation technique. Front. Anim. Sci. 3:1021631. doi: 10.3389/fanim.2022.1021631
Received: 17 August 2022; Accepted: 29 November 2022;
Published: 13 December 2022.
Edited by:
Eleni Tsiplakou, Agricultural University of Athens, GreeceReviewed by:
Valiollah Palangi, Atatürk University, TurkeyAlexandros Mavrommatis, Agricultural University of Athens, Greece
Copyright © 2022 Roskam, Kirwan, Kenny, O’Donnell, O’Flaherty, Hayes and Waters. This is an open-access article distributed under the terms of the Creative Commons Attribution License (CC BY). The use, distribution or reproduction in other forums is permitted, provided the original author(s) and the copyright owner(s) are credited and that the original publication in this journal is cited, in accordance with accepted academic practice. No use, distribution or reproduction is permitted which does not comply with these terms.
*Correspondence: Sinéad M. Waters, Sinead.waters@teagasc.ie