Duplex droplet digital PCR detection of Streptococcus uberis and Streptococcus dysgalactiae, major etiological agents of bovine mastitis
- 1Laboratorio de Microbiología, Departamento de Patobiología, Facultad de Veterinaria, Universidad de la República, Montevideo, Uruguay
- 2Laboratorio Biología Computacional, Departamento de Desarrollo Biotecnológico, Instituto de Higiene, Facultad de Medicina, Universidad de la República, Montevideo, Uruguay
- 3Department of San Jose, Laboratorio de la Rural de San José, San José, Uruguay
- 4Facultad de Ciencias Veterinarias, Universidad Nacional del Litoral, Esperanza, Santa Fe, Argentina
- 5Department of Animal and Dairy Sciences, University of Wisconsin-Madison, Madison, WI, United States
Bovine mastitis is one of the most important diseases affecting dairy cattle worldwide, resulting in significant economic losses due to high costs mainly associated with decreased production, antimicrobial treatment, and early culling of animals. The genus Streptococcus is among the primary bacterial pathogens causing bovine mastitis worldwide. The correct and timely diagnosis of mastitis is critical for the dairy industry, not only from the point of view of milk hygiene but also for economic, public health, and animal welfare reasons. Herein, we developed a diagnostic test of bovine intramammary infection employing a duplex droplet digital PCR (dddPCR) to detect and quantify Streptococcus uberis and Streptococcus dysgalactiae in milk, which outperforms the gold standard culture-based technique and the endpoint PCR. Indeed the detection limit for cultures and mock samples for dddPCR was a hundred times lower than the endpoint PCR. Additionally, the CFU/mL estimated based on the number of copies/uL obtained through dddPCR exhibited a strong correlation with the observed CFU/mL from the culture (r^2 > 0.99, p-value < 0.001), indicating that dddPCR provides a dependable estimate of this parameter. Moreover, the sensitivity of endpoint PCR, determined from artificial samples, was 40% for S. uberis and 55.4% for S. dysgalactiae meanwhile, the sensitivity of dddPCR was 80% and 100% for S. uberis and S. dysgalactiae, respectively, while the specificity was 100% for both techniques and pathogens. In conclusion, we propose a robust and reliable technique standardized for detecting and quantifying two of the most important bacteria that cause bovine mastitis. This dddPCR method may be particularly suitable to detect pathogens in milk samples with low bacterial loads or intermittently shedding and should be further tested with a larger sample size in future research.
Introduction
Bovine mastitis is one of the most important diseases affecting dairy cattle worldwide, resulting in significant economic losses due to the high costs associated with milk production losses, antibiotic treatment, and early culling of animals (Ruegg, 2017). Mastitis can be classified in clinical or subclinical. The clinical form is diagnosed based on visual inflammation of the affected quarter and clots or flakes in the milk. On the other hand, the mammary gland and milk of subclinical cows are not visibly affected. Still, there is an increased number of polymorphonuclear leukocytes, which is reflected in greater Somatic Cell Count (SCC) in the milk (Koskinen et al., 2010). Mastitis is predominantly infectious, being bacteria the principal etiological agents. The agents that produce intramammary infections (IMI) are classically classified based on epidemiological studies in contagious and environmental (Garcia, 2004). Pathogens that are exclusively contagious, such as Staphylococcus aureus, Streptococcus agalactiae, and Mycoplasma spp, can be transmitted from an infected to a healthy animal during milking through hands, towels, or the milking machine, which act as fomites. In contrast, pathogens such as Streptococcus dysgalactiae (S. dysgalactiae) and Streptococcus uberis (S. uberis) come from the animal’s environment, such as bedding material, soil, feces, and standing water or from an infected animal, categorizing them as environmental or contagious pathogens (Garcia, 2004). The genus Streptococcus is among the primary bacterial pathogens causing bovine mastitis worldwide, including Uruguay (Kaczorek et al., 2017; Gianneechini et al., 2014; Gianneechini et al., 2002). In particular, the species S. dysgalactiae and S. uberis are the most prevalent, leading to significant losses in dairy production in Uruguay (Gianneechini et al., 2014). Mastitis caused by S. uberis is generally of environmental origin; however, cases of infection between animals have been observed (Garcia, 2004). This bacterium is primarily alpha-hemolytic, but some non-hemolytic strains have also been demonstrated (Kabelitz et al., 2021). Biochemical identification by culture is performed by determining the CAMP phenotype (Christine-Atkinson-Munch-Peterson test) and the degradation of aesculin, sodium hippurate, and inulin, which are variables among strains (Kromker et al., 2014; Kabelitz et al., 2021). The Lancefield classification of S. uberis is challenging, as some strains have been positive for several groups (E, G, P, or U) (Kabelitz et al., 2021).
On the other hand, the classification of S. dysgalactiae as environmental or contagious is not as precise, as it can survive within the host and the environment, so it is described as an intermediate pathogen (Wente & Krömker, 2020). Most strains of S. dysgalactiae are nonhemolytic, although there are alpha-hemolytic exceptions. Phenotypically, it is CAMP negative, does not degrade aesculin, belongs to Lancefield’s group C and is mainly associated with bovine infections, but it can also infect other ruminants such as goats and sheep (Kabelitz et al., 2021).
Mastitis diagnosis Should be early, rapid, and accurate in order to implement effective prophylactic or therapeutic treatments or management strategies to prevent the spread of the disease in the dairy herd (Sharun et al., 2021). Approximately 30% of mastitis cases cannot be diagnosed by culture since only viable pathogens that grow in conventional culture media can be detected; hence, there is a high rate of false negative results (Król et al., 2009; Ashraf and Imran, 2018). In addition, the variability in the shedding rate of elimination of different pathogens from the cow’s mammary gland may result from of individual differences in the inflammatory response. Moreover, in animals experimentally challenged, it has been demonstrated that the total bacterial count in milk, measured as CFU/mL, increases rapidly at the beginning of the disease but falls to a lower level after that. Therefore classical culture techniques may not be useful for the final diagnosis (Carroll et al., 1964; Sears et al., 1990; Britten, 2012), and the development of new diagnostic tools are needed.
Current molecular techniques such as polymerase chain reaction (PCR), mass spectrometry (MALDI-TOF), or next-generation sequencing (NGS) are used for rapid and specific identification of mastitis-producing pathogens down to the species and subspecies level and have allowed considerable progress in the diagnosis of this disease (Gillespie & Oliver, 2005; Zadoks & Watts, 2009; Duarte et al., 2015; Chakraborty et al., 2019). Several studies have combined conventional microbiology with multiplex PCR to identify bacteria associated with IMI in cattle with promising results (Raemy et al., 2013; Carvalho-Castro et al., 2017). A molecular technique that has emerged in the last decade is the third generation of PCR, droplet digital polymerase chain reaction (droplet digital PCR/ddPCR). This technique allows the absolute measurement of nucleic acids by partitioning PCR reactions into hundreds of millions of partitions (i.e., droplets). The fluorescence intensity of each droplet is measured using a Poisson algorithm; each droplet will be classified as positive or negative (McDermott et al., 2013; Powell and Babady, 2018). In this way, ddPCR allows quantification of amplicons and the number of pathogens without a calibration curve (Ramírez et al., 2018). The ddPCR technique has been used to detect viruses, bacteria, and parasites of medical and veterinary importance from various biological samples (Zheng et al., 2021; De Brun et al., 2022; Wu et al., 2022). It has also been implemented to detect foodborne pathogens (Cremonesi et al., 2016; Powell & Babady, 2018; Du et al., 2022).
Herein, we developed an advanced diagnostic test for bovine IMI using duplex droplet digital PCR (dddPCR) in. ilk to detect and quantify two of the four most prevalent primary pathogens that cause mastitis in dairy cows: Streptococcus uberis and Streptococcus dysgalactiae.
Materials and methods
Bacterial strains and preparation of mock samples of mastitic milk
Two ATCC strains were used for analysis: Streptococcus uberis ATCC 9927 and Streptococcus dysgalactiae ATCC 12394. Both strains were grown on Trypticase Soy Agar (TSA) medium enriched with 4% sheep blood and incubated in aerobiosis at 37˚C ± 2˚C for 48 hr. The isolated colonies were subsequently used for DNA extraction and inoculation of sterile milk. These strains were used to contaminate 10% whole milk sterilized at 111°C for 30 min to evaluate the performance of dddPCR and conventional PCR. For this purpose, pure culture inocula, equivalent to 0.5 of the McFarland scale (1.5x108 CFU/mL) were used. The CFU/mL of each inoculum was confirmed by plate count on Blood Agar (BS) using the 6x6 drop plate method (Chen et al., 2003). Finally, 10 mL of sterile whole milk was contaminated with 1 mL of each prepared bacterial inoculum. Three mock samples of mastitic milk were generated, one for each pathogen (Sube Milk and Sdys Milk), and a third sample was prepared using both inoculums and making a pool (Sube Milk + Sdys Milk), then the CFU/mL was calculated based on Chen et al. (2003). Serial dilutions in base ten were made from dilution -1 to dilution -7 using milk as the diluent and subsequently used for DNA extraction.
DNA extraction from pure cultures and mock samples of mastitic milk
DNA was extracted from 1 mL of pure cultures and 1 mL of the mock samples previously prepared (Sube Milk, Sdys Milk, and Sube Milk + Sdys Milk), including dilutions from -1 to -7. GenElute™ Bacterial Genomic DNA kit (SIGMA-Aldrich) added with lysozyme (SIGMA-Aldrich) at a concentration of 9 mg/sample was used following the manufacturer’s instructions. For mock samples, three consecutive centrifugations were performed at 13,300 rpm at 4˚C for 5 minutes before extraction, and the pellet obtained was washed with sterile saline. The quantification of the DNA collected was determined by Qubit 4 fluorimeter using the 1X dsDNA HS (high sensitivity) assay kit (Thermo Fisher Scientific). Extracted DNA was stored at -20°C until subsequent use in PCR analysis.
Primers and probes design
A comparative genomics approach was applied to identify candidate molecular markers for S. dysgalactiae and S. uberis (Supplementary Figure 1). Briefly, all available genome sequences of S. dysgalactiae and S. uberis were downloaded from the NCBI genome database (Supplementary Table 1). Genome annotation was carried out by Prokka (Seemann, 2014). The set of conserved genes, i.e., the core-genome, was determined for each species using Roary software (Page et al., 2015). In order to identify species-specific genes, the core genome of both species was compared by tBLASTx (Altschul et al., 1990) using a minimum amino acid identity value of 30% and minimum coverage of 70%. Putative unique genes identified in both species were blasted against other Streptococcus genomes at the NCBI genome database using BLASTp with default parameters (Altschul et al., 1990). A gene was considered species-specific if the gene was not present in any other species of Streptococcus genus, with a minimum amino acid identity of 90%. Primers and probes design was performed using Primer3 software (Untergasser et al., 2012) with the following parameters: i) amplification product length < 180 bp; ii) G or C preference for starting nucleotide; iii) primers length between 18 and 24 bp; iv) probe length between 30 and 38 bp; v) GC content ≥ 50%, vi) similar melting temperature between both primers and the probe, and vii) NCBI blast hits only with target species.
Endpoint PCR amplification
To validate the designed primers, an endpoint PCR was done using the DNA extracted from pure cultures of both Streptococcus strains. PCR reactions were performed using NZYTaq II 2× Green Master Mix from NZYtech. The mixture for PCR reactions was performed in a final volume of 25 μL: 12.5 μL of master mix, 1 μL of each primer at a concentration of 10 μM, 9.5 μL of DNAse- and RNAse-free water, and 4 ng of DNA. The PCR runs were performed on a C1000 TouchTM thermal cycler (Bio-Rad). The conditions were as follows: initial denaturation at 95°C for 4 minutes followed by 30 cycles of 1 minute at 94°C, 1 min at 60°C, and 30 seconds at 72°C, ending with a final extension of 5 minutes at 72°C. To confirm the presence of the amplified fragment, the PCR mixture was subjected to 2% agarose gel electrophoresis in TAE buffer using 5 μL of Thermo scientific GeneRuler 100bp molecular weight marker. The run was performed at 100V for 45 minutes, and amplicons were visualized by staining with SYBER® Safe DNA gel stain from Invitrogen and exposure to UV light. PCR products were purified and sequenced using forward and reverse primers by Macrogen Inc, Seoul, South Korea. The chromatogram received from the sequencing service was edited using the Bioedit program (Hall, 1999), and typical Sanger sequencer errors were eliminated by visual inspection. The final consensus sequence for each pure culture was obtained using Bioedit.
Duplex droplet digital polymerase chain reaction assay
The ddPCR reactions were performed using the QX200TM Droplet Digital PCR System (Bio-Rad Laboratories, Hercules, CA, USA). Previously designed probes and primers were used for each pathogen (Table 1). The ddPCR reaction for the duplex (dddPCR) was performed in a final volume of 20 µL containing 10µL of ddPCR™ Supermix for Probes (Bio-Rad), 900nM of each primer, 450 nM of each probe, and 1µL of DNA. The total reaction volume was reached with DNA-RNAase-free water. For partitioning the reaction and obtaining droplets, 20 µL of reaction and 70 µL of Droplet Generation oil for Probes (Bio-Rad) were placed in an eight-well cartridge that was subsequently placed in the QX200 droplet generator (Bio-Rad). The droplets obtained were subsequently transferred to ddPCR™ 96-Well Plates (Bio-Rad) and subjected to amplification cycles in a C1000 TouchTM thermal cycler (Bio-Rad). Amplification conditions were as follows: 10 minutes at 95°C for DNA polymerase activation, followed by 40 cycles of 30 seconds at 94°C for denaturation, and 1 minute at 60°C for hybridization and extension. Finally, enzyme deactivation and droplet stabilization were performed for 10 minutes at 98°C with subsequent cooling to 4°C. Using the QX200 droplet reader (Bio-Rad Laboratories), the number of resulting positive and negative events were determined by fluorescence detection and the data were analyzed in the QuantaSoft v.1.7.4 software (Bio-Rad). Concentration was expressed in copies/µL from positive events using the Poisson distribution for analysis. All samples were analyzed in triplicate. The CFU/mL was calculated from the copies/µL obtained in the ddPCR and taking into account the volume of sample from which the DNA was extracted, the volume of DNA elution in the extraction, and that the amplified gene is only once in the genome (1 copy=1 CFU). The CFU/mL was determined from the DNA copies obtained for each pathogen.
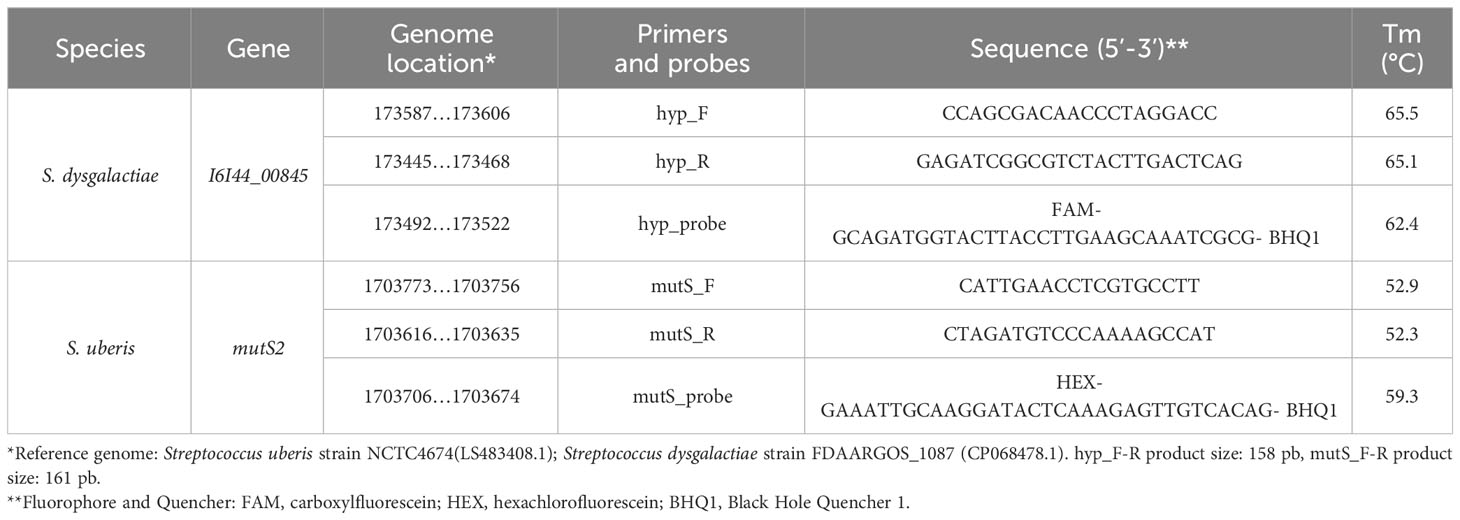
Table 1 Primers and probes designed for endpoint PCR and duplex ddPCR techniques for the detection of S. uberis and S. dysgalactiae.
Specificity and detection limit of primers and probes
The specificity of each primer pair was evaluated by endpoint PCR and dddPCR from purified genomic DNA of the previously mentioned ATCC reference strains. Reference strains of the genera Staphylococcus and Streptococcus were used as negative controls since they correspond to the other most prevalent bacterial genera that produces mastitis and may also be present in some field samples. The minimum detection limit (LoD) for endpoint PCR and dddPCR was established using pure cultures, starting from an initial concentration of 4 ng/µL (4000 pg/µL) of DNA template and subsequently diluting at a ratio of 1:10 until reaching a concentration of 0.04 pg/µL. Additionally, the LoD for both techniques was evaluated using artificially contaminated milk samples. This was achieved by diluting the contaminated milk samples at a 1:10 ratio, ranging from 3.5 x 107 CFU/mL to 35 CFU/mL.
Sensitivity and specificity of end-time PCR and ddPCR
To determine the sensitivity and specificity of end-time PCR and dddPCR, 10 mL of milk were inoculated with 1x108 CFU/mL of field strains of S. uberis (10 strains), S. dysgalactiae (9 strains) and S. aureus (10 stains) and diluted to dilution -5 since this was the last dilution at which we had growth in the culture. PCR previously identified all these field strains in our laboratory. The sensitivity and specificity were determined (Martin, 1984) and the concordance index (kappa index) of both techniques with the classical culture diagnostic technique was also determined.
Field sample analysis of dairy milk
Milk samples were sent by veterinarians or dairy farmers from March to July 2021 from farms located in San José – Uruguay (34°20′20″S 56°42′37″O). For milk samples SCC assessment the reference system for SCC assessment (i.e., automated electronic cell counters based on flow cytometric methods) was implemented. Sixty-seven quarter milk samples with high SCC (>200.000 cel/mL) were cultured following the protocol recommended by the National Mastitis Council (Firdaus, 2017).
Results
Primer and probe design
The design of specific primers and probes for the determination of S. dysgalactiae and S. uberis was done based on comparative genome approaches. The pangenome analyses of 120 and 68 genomes of S. dysgalactiae and S. uberis, respectively, were done using Roary software (Page et al., 2015). The assembly accession number of each genome used in the present study is available in Supplementary Table 1. The core genome of S. dysgalactiae and S. uberis comprised 806 and 101 genes, respectively. The core genes were compared by tBLASTx software resulting in 716 and 22 putative unique genes found in S. dysgalactiae and S. uberis, respectively and 68 shared genes were found among the two species (Supplementary Table 1). To avoid the amplification of other Streptococcus species by the designed primers, the unique genes were compared to the Streptococcus genomes available at the GenBank database using the web protein BLAST tool (blast.ncbi.nlm.nih.gov). Genes that only match with S. dysgalactiae or S. uberis were considered species-specific and used for further analyses. A total of 166 species-specific genes were found in S. dysgalactiae and two in S. uberis (Supplementary Table 1). One chromosomal gene from each specie was chosen at random. Endonuclease mismatch repair protein MutS and putative class A beta-lactamase-related serine hydrolase protein (named I6I44_00845 gene) were selected as molecular markers of S. uberis and S. dysgalactiae, respectively. In the reference genome of S. dysgalactiae, mutS gene is located in a conserved region in the main chromosome, next to TrxA_2 (thioredoxin) and NCTC4674_01802 gene (membrane protein). The I6I44_00845 gene is located in a conserved region in the main chromosome of S. uberis, flanked by tilS (tRNA lysidine(34) synthetase) and I6I44_00850 (a septum formation initiator family protein-coding gene). The primer annealing region was selected according to the conservation sequence (no more than 3 nucleotides differences (Table 1). The amplified sequences in S. uberis and S. dysgalactiae have an expected length of 158 bp and 161 bp, respectively. Both probes have a length of 32 bp.
Analysis of primer specificity
Endpoint PCR and dddPCR assays correctly identified both pathogens from the culture. The banding patterns obtained in the agarose gel electrophoresis showed that the designed primers do not generate nonspecific amplifications at the established temperature. Both observed PCR product bands are within the expected length for S. uberis and S. dysgalactiae. Neither amplification was obtained for any of the non-Streptococcus species analyzed, nor in the negative control (Supplementary Figure 2). The Sanger sequence of the amplified PCR product confirmed for both species that the target sequence is mutS and I6I44_00845 (Supplementary Figure 3). The quantification of bacterial load at -3 dilution from mixed and separated DNA isolation from culture samples of S. uberis and S. dysgalactiae showed similar results (Supplementary Figure 4). The dddPCR results support primer specificity and no inhibition between both target Streptococcus species. The similarity search by BLASTn in the Genbank database shows that both sequences are fully conserved within the species, with 100% identity at the nucleotide level. Taken together, these results show that the designed primers are effectively species-specific.
Standardization of quantitative dddPCR
First, the LoD in pure cultures was established. Conventional PCR and duplex ddPCR were performed in triplicate, and the DNA extracts of both pathogens were mixed and diluted from -1 to -5. In the case of endpoint PCR, the amplification was observed up to a dilution -3 (4pg/µL), while the duplex ddPCR was able to quantify up to a dilution of -5 (0.04 pg/µl) for both pathogens (Table 2). To determine the detection capability of both PCR techniques from milk samples three mock samples of mastitic milk were analyzed: Sube Milk, Sdys Milk, and Sube + Sdys Milk. The product of endpoint PCRs showed a band of the expected size up to dilution -4 (1x103 CFU/mL) (Figures 1A, C). The duplex ddPCR was able to determine the copies/µl of S. uberis and S. dysgalactiae in all mock samples and replicates up to a dilution of -6 (10 CFU/mL) (Table 3; Figures 1B, D, E). The determination of CFU/mL in the mock Sube + Sdys Milk sample from the copies/µl obtained by duplex ddPCR for each pathogen is highly correlated with those calculated by plate count (r2 > 0.99, p-value < 0.001; Figure 2).
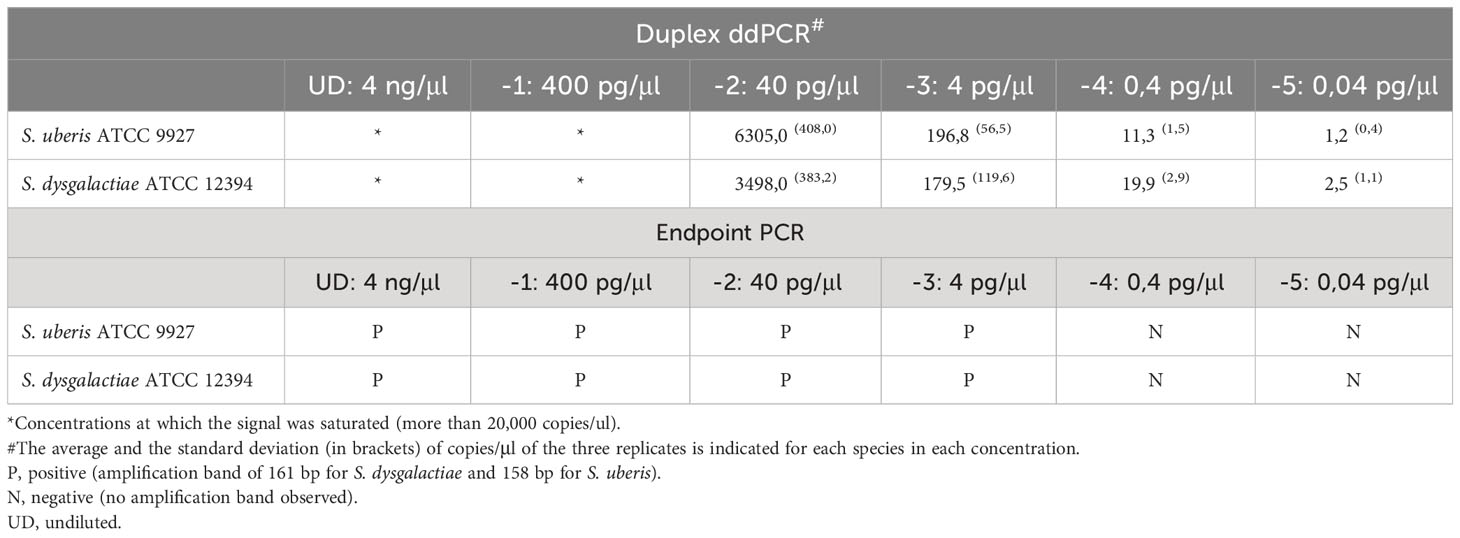
Table 2 Limit of detection (LoD) of endpoint PCR and duplex ddPCR for S. uberis and S. dysgalactiae from genomic DNA of pure cultures.
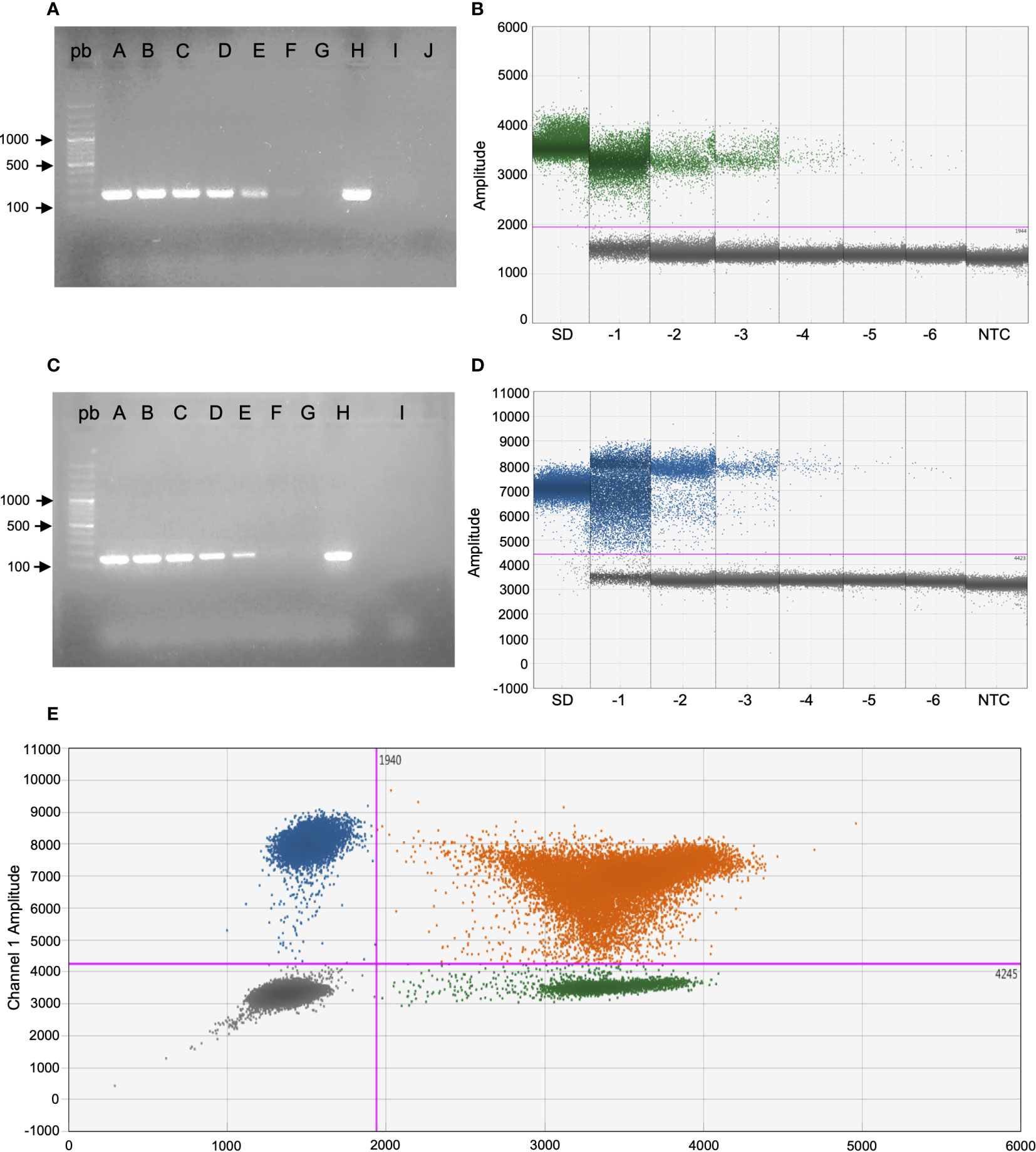
Figure 1 Sensitivity and specificity of end-time PCR and duplex ddPCR of S. dysgalactiae (A, B) and S. uberis (C, D) from the mock sample with both reference strains. (A, B) From lane A to G and SD to -6: dilutions of the mock sample from undiluted to -6 (3.5 x107 CFU/mL - 3.5 x101 CFU/mL), lane H: S. dysgalactiae DNA from culture, lane I: S. uberis DNA from culture, lane J: reaction control with milk sterile and bp: 100bp molecular weight marker, NTC: no template control. (C, D) From lane A to G and SD to -6: dilutions of the mock sample from undiluted to -6 (3.5 x107 CFU/mL - 3.5 x101 CFU/mL), lane H: S. uberis DNA from culture, lane I: S. dysgalactiae DNA from culture, lane bp: 100bp molecular weight marker, NTC: no template control. (E) 2D plot of ddPCR duplex: droplets positive only for S. uberis (blue), droplets positive only for S. dysgalactiae (green), and double positive droplets (orange).
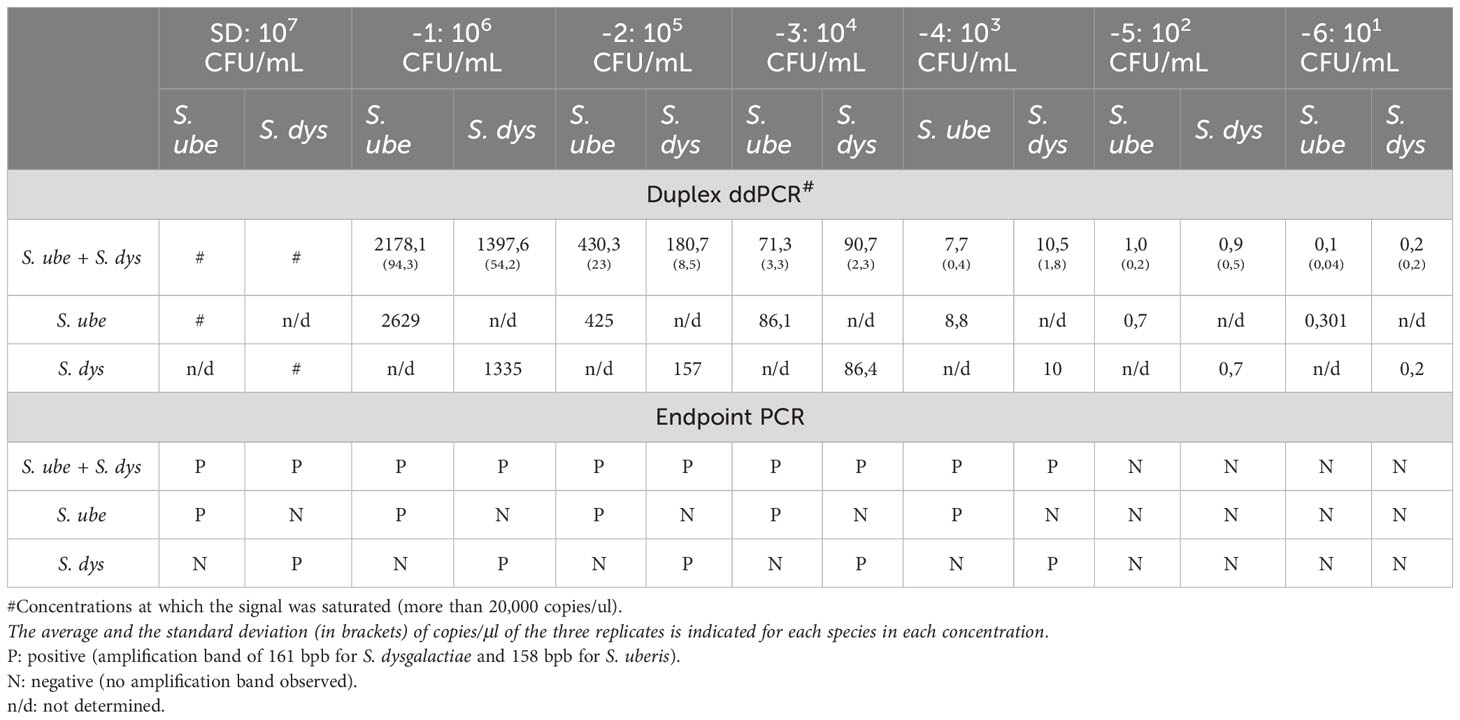
Table 3 Limit of detection (LoD) of endpoint PCR and dddPCR for S. uberis and S. dysgalactiae from milk DNA artificially inoculated with both pathogens (mock sample).
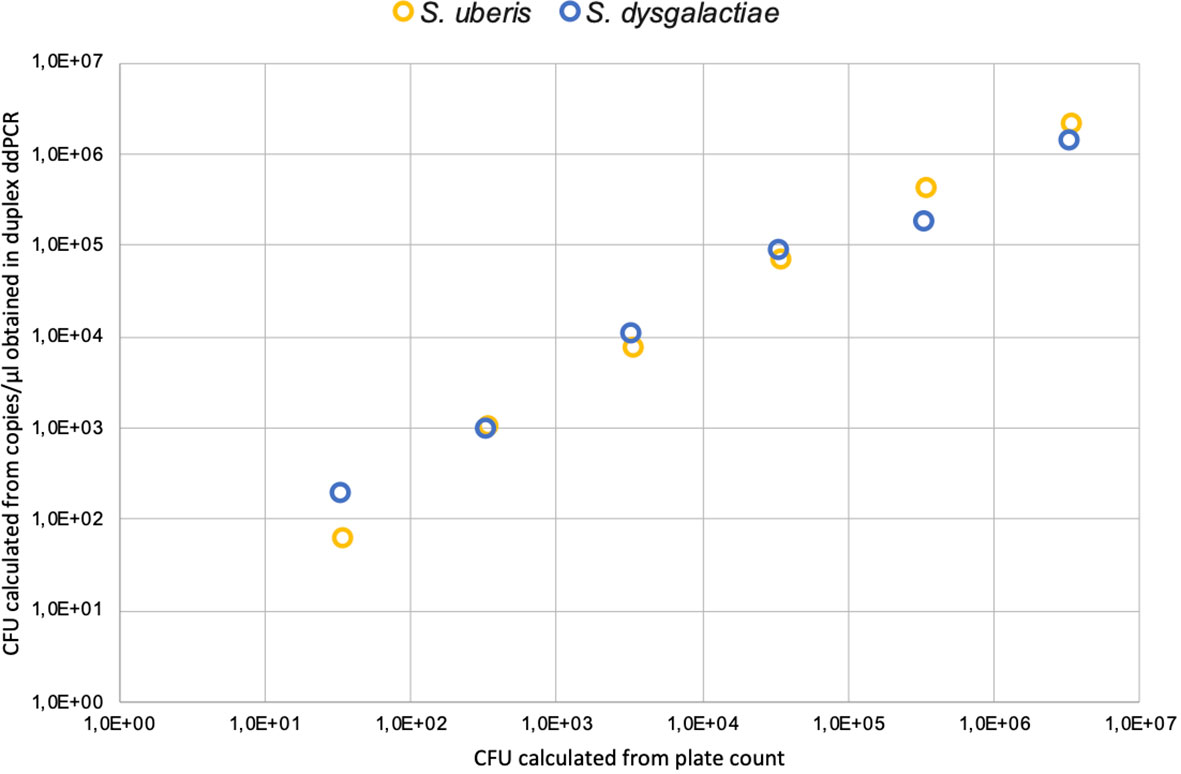
Figure 2 Correlation of CFU calculated from plate count and copies/μl obtained in duplex ddPCR for each pathogen.
Analysis of mock samples and dairy milk samples
The sensitivity (Se) and specificity (Sp) of dddPCR and endpoint PCR for the detection of each of the pathogens could be determined by analyzing milk samples inoculated with field strains.
The Se of the end-time PCR for detecting S. uberis was 40%, while the Se for detecting S. dysgalactiae was slightly higher (55.4%). On the other hand, the Se for the dddPCR technique was 100% and 80% for S. dysgalactiae and S. uberis, respectively. The Sp was 100% for both techniques for both pathogens.
The Kappa indices obtained determined a moderate concordance (kappa = 0.47) for culture and end-time PCR in the diagnosis of S. uberis, while for the diagnosis of S. dysgalactiae it was a good concordance (kappa = 0.63).
However, the concordance indices for dddPCR with culture were 1 and 0.84, determining a very good concordance for both pathogens.
Sixty-seven high SCC samples were analyzed using the two PCR techniques developed. Five culture samples were positive for S. uberis, twelve for S. dysgalactiae, and sixteen for S. aureus or coagulase-negative staphylococci (SCNS). Twenty-eight samples that did not present bacterial growth (negative culture) and six with isolation of Streptococcus sp. (species not determined) were also analyzed. All (12/12) field milk samples with positive culture for S. dysgalactiae were negative for this pathogen by endpoint PCR, and the copies/µl obtained by dddPCR allowed us to determine that they had less than 340 CFU/mL (Table 4). Moreover, 87.5% (11/12) of those samples were positive by endpoint PCR for S. uberis, and from the copies/µl obtained by ddPCR, it was possible to determine that they had a high number of S. uberis (between 4.17x103 and 2.6x106 CFU/mL). One sample (SJ6344) was negative by endpoint PCR for S. uberis but showed a low S. uberis load (887 CFU/ml calculated from dddPCR results). Three samples with positive cultures for S. uberis (SJ2011, SJ1992, and SJ8296AD) were also positive for this pathogen in the endpoint PCR. It is important to note that SJ1992 showed a relatively low amplification rate in the endpoint PCR (weak band in agarose gel electrophoresis). The quantification of S. uberis from dddPCR data for samples SJ1992 and SJ2011 was 8.87 copies/µl, corresponding to 8.87 x103 CFU/ml and 32.6 copies/µl corresponding to 3.26 x104 CFU/ml, respectively. Endpoint PCR and dddPCR showed that sample SJ2011 was also positive for S. dysgalactiae. Moreover, quantification by dddPCR suggested that the abundance of S. dysgalactiae in the sample was many times higher than that of S. uberis (Table 4).
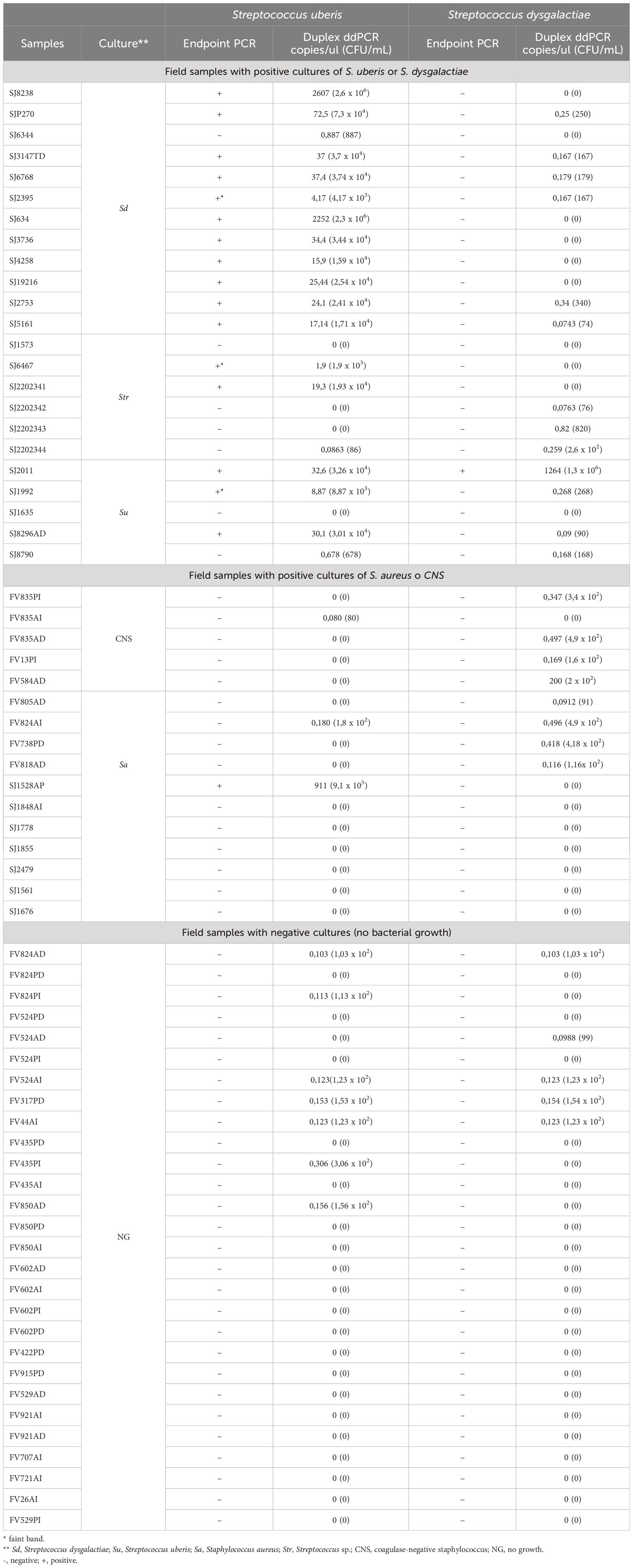
Table 4 Results obtained from the analysis of dairy milk samples by endpoint PCR and dddPCR for both pathogens.
93,75% (15/16) of the samples that presented positive culture for the Staphylococcus genus were negative for the two Streptococcus species in the endpoint PCR. In agreement, the load of S. uberis and S. dysgalactiae calculated from the dddPCR results for all these samples was low (< 496 CFU/mL). Only the sample SJ1528AP, yielded a quantification of 911 copies/µl of S. uberis, corresponding to 9,1 x105 CFU/ml. Finally, 28 samples that resulted in no growth when cultured (negative culture) showed no amplification in the endpoint PCR of either pathogen and low quantification from duplex ddPCR (< 306 CFU/mL, Table 4).
Discussion
A rapid and accurate tool for diagnosing pathogens that cause mastitis is fundamental for effectively treating and controlling this infectious disease in dairy herd (Mahmmod, 2015). The gold standard technique for the diagnosis of mastitis is the microbiological culture; nonetheless, this methodology has some disadvantages. The number of bacteria released in the milk at different stages of an IMI is highly variable among cows (Dohoo and Meek, 1982; Sears et al., 1990; Britten, 2012), causing culture-based diagnoses to be inaccurate and non-robust. The detection threshold of routine culture-based diagnosis is limited to a minimum bacterial load of 100 CFU/ml in milk (Britten, 2012). Thus, in cases where no growth is obtained in culture, increasing the seeding volume from 10 µl to 100 µl, it is recommended to decrease the risk of false negatives from 40% to 25% (Britten, 2012). Consequently, single sampling is not adequate for diagnosis. Indeed, it is recommended that a second sample is taken and the culture is repeated pre-incubating the milk sample at 37°C for 4 hrs before seeding or re-seeding the same sample at 24 hrs (Erskine and Eberhart, 1988). The proposed optimization procedures for diagnosis by culture-based methods lead to an increased diagnostic time, delaying on-farm decision-making.
Over the past decade, several molecular techniques have been developed to diagnose bovine IMI, either from culture isolates or mastitic milk samples. These techniques include endpoint PCR, qPCR, and MALDI-TOF, among others (Nonnemann et al., 2019). Compared with bacterial culture, these techniques are faster, easier to automate, and more sensitive; however, PCR techniques are typically designed for genomic DNA identification rather than the detection of viable cells, and additional evaluation is required to determine the clinical significance of their outcome (Koskinen et al., 2010).
To our knowledge, droplet digital PCR, the novel and latest PCR technique has not yet been utilized for IMI diagnosis, despite being state-of-the art. As a first step in developing the proposed methodology, we demonstrated that the designed primers and probes were specific for S. uberis and S. dysgalactiae. First, by in-silico analyses of sequences deposited at GenBank, we determined that the selected marker genes were species-specific. The endpoint PCR from pure cultures, including S. uberis, S. dysgalactiae, S. agalactiae, and Staphylococcus species frequently associated with mastitis, also supports the primers are specific and that there is no cross-amplification with other species. When analyzing the duplex ddPCR quantification of each pathogen at -3 dilution (1x 104 CFU/mL) of the mixed or non-mixed pathogen’s DNA, we observed that the estimated copies for both pathogens were similar. It is important to note that the DNA from both pathogens was mixed in equal quantities, and the total amount of DNA in these reactions was the same. Therefore, the number of copies/µl when DNA pathogens were analyzed separately was expected to be twice as high as when mixed. Since the analysis of mixed DNA was just barely lower than expected, we could not completely rule out some minimal inhibition in the reaction, which could decrease the number of expected copies obtained when the DNA of both pathogens was mixed. Taken together, the results of the ddPCR from pure cultures support the specificity of primers and the probes designed. This is relevant for its proper use as a diagnostic tool since the probes increase the specificity of this technique concerning endpoint PCR (Powell and Babady, 2018).
Phuektes et al. (2001) developed a multiplex PCR to detect S. aureus, S. uberis, S. dysgalactiae, and S. agalactiae by endpoint PCR determining LoD of 5 pg/uL of DNA from pure cultures of each strain. Our results are in alignment with those reported, having determined an endpoint PCR LoD of 4 pg/ul of DNA from pure cultures of S. uberis and S. dysgalactiae. In addition, the LoD of ddPCR was 0.04 pg/µl of DNA from pure cultures for both pathogens, indicating a higher sensitivity of ddPCR compared to endpoint PCR. On the other hand, the same authors determined that the LoD of the endpoint PCR for mock mastitic milk samples was 103 CFU/mL for both pathogens, consistent with those obtained in our study (103 CFU/mL).
Gillespie and Oliver (2005), determined the minimum level of detection by qPCR of S. uberis from inoculated UHT milk samples with pre-enrichment overnight at 37˚C ± 2˚C with trypticase soy broth (TSB) being 10 CFU/mL, while without enrichment, the limit of detection was 102 CFU/mL. For these authors, the pre-enrichment of milk would reduce the number of inhibitors present in the milk, allowing the detection of pathogens even if they are in small quantities. The dddPCR developed here demonstrated a minimum detection level of 10 CFU/mL for both pathogens without pre-enrichment. These results indicate that dddPCR is more sensitive than qPCR, with a sensitivity a hundred times higher than endpoint PCR for both pathogens. The higher sensitivity of dddPCR may be explained by the higher tolerance of this technique to different inhibitors usually present in milk samples (e.g., Ca2+, fats, proteins). This tolerance has been previously described and attributed to the partitioning of the reaction performed in this technique, which may reduce the interference of inhibitors (Huggett et al., 2013; Rački et al., 2014; Yang et al., 2014). It should be noted that in the mock samples, for all the dilutions and both pathogens, we determined the CFU/ml from the copies/µl obtained by dddPCR. This result agrees with the CFU/mL obtained by plate count-based techniques. Thus, we conclude that the bacterial load derived from dddPCR results is comparable to that of conventional plate count (r2 > 0.99, p-value < 0.001).
A recent study compared qPCR with conventional bacterial culture in field samples (Koskinen et al., 2010). These authors used 780 animals and showed that 89% of the samples were positive for some pathogen by qPCR, while only 77% were detected by culture. To test the newly developed methodology, we analyzed 67 mastitic milk samples with high SCC using dddPCR. Although we expected these samples to be more heterogeneous than the analyzed mock samples in terms of the microorganisms present, the amount of DNA from somatic cells, and the variable concentration of protein and fat, all the Streptococcus culture-positive samples were positive for this pathogen by dddPCR. Previously, Koskinen et al. (2010) identified 73 samples with S. dysgalactiae and 81 samples with S. uberis by bacterial culture, and most of these samples were also identified by qPCR. A small fraction of these samples, 10,9% (8/73) and 12,3% (10/81), could not be assigned to either species, respectively. This suggests that the dddPCR method developed was more effective in detecting positive samples than the qPCR-based method described by Koskinen et al. (2010).
Surprisingly, all samples that were culture positive for S. dysgalactiae were not positive for this pathogen by endpoint PCR, while ddPCR quantified it below 250 CFU/ml. This result for endpoint PCR can be explained first by the low load of this pathogen in these samples, being below the detection limit of the technique, which, as stated above, was 1.000 CFU/mL. Another reason for positive culture results and negative PCR outcomes is that the PCR might produce “false negatives” when species are misidentified during the culture process. Laboratories performing routine mastitis diagnostics experienced a misidentification rate of approximately 9% to 37% for bacteria (Pitkälä et al., 2005). Finally, but no less importantly, it has been reported that 12% of S. uberis strains isolated from clinical and subclinical mastitis samples exhibit significant phenotypic variability, making their identification challenging (Pitkälä et al., 2005). Moreover, more than 80% of these samples were positive by endpoint PCR for S. uberis and the CFU/mL determined from the copies/µl obtained from dddPCR quantification was between 103 and 106 CFU/mL.
These results highlight the importance of choosing the appropriate diagnostic techniques to detect mastitis pathogens. Our results showed that the diagnosis based on culture was inaccurate in the phenotypic identification of Streptococcus at the species level. This issue has already been reported and may be related to the fact that different strains of the same species are variable in the expression of widely used phenotypic traits used to determine species (Jiang et al., 1996; Riffon et al., 2001; Raemy et al., 2013; Kabelitz et al., 2021). A plausible explanation for our results is that the pathogen isolated in these samples was S. uberis, as demonstrated by molecular techniques, and that it was misidentified as S. dysgalactiae by the culture-based identification test. Reaching an incorrect diagnosis leads to inappropriate management decisions in the herd (Sharun et al., 2021). This is especially true for mastitis caused by S. uberis, as the existence of strains within this species exhibiting both environmental and contagious behavior has been described through proteomic and genomic approaches. (Davies et al., 2016; Esener et al., 2018; Sharun et al., 2021).
Second, it is possible that S. dysgalactiae was also present in these samples but with a load that was difficult to detect by culture or endpoint PCR, but successfully detected by dddPCR. This is particularly important if considering the variable Shedding of pathogens in milk in different animals that has previously been reported (Britten, 2012). Thus, we cannot rule out the possibility that S. dysgalactiae also contributed to mastitis but in a stage of lower release. To discard this possibility, it is necessary to study the animal’s immunological status and to carry out studies over time. For sample SJ2011, in which both pathogens were identified by endpoint PCR and dddPCR. This could indicate a rare case of co-infection, where S. dysgalactiae is the most abundant pathogen according to dddPCR and is probably generating the disease. Another possibility is that S. uberis has an environmental origin, while S. dysgalactiae is the etiological agent of mastitis. In a study published by Steele et al. (2017), it was determined that the PCR technique is not indicated for diagnosing S. uberis in early lactation stages since the sensitivity decrease from 98% to 77% (Steele et al., 2017). Samples in which S. uberis was isolated by culture but were not detected by PCR could belong to animals in an early lactation stage; however, since the information on days on lactation of the cows from which the submitted samples were taken was not available, it is not possible to draw conclusions in this regard.
We identified samples with positive cultures for Staphylococcus aureus that were negative for both Streptococcus in the end point PCR. In these samples, loads of less than 4,7 x 102 CFU/mL were quantified by dddPCR. These results could indicate the presence of both pathogens in these animals but with a very low Streptococcus load relative to Staphylococcus aureus, making the culture-based detection of Streptococcus difficult. The same occurred with the culture negative samples for Streptococcus, in which the dddPCR quantified loads of both Streptococcus species lower than 1,5 x 102 CFU/mL. This load is well below the detection limit of end point PCR and culture. The high sensitivity of dddPCR may result in low quantification but positive results in animals that could be free of infection. This is one drawback of the dddPCR technique that could be tackled by establishing a threshold of quantification to consider an infected animal. In this regard, it is necessary to continue working by analyzing larger sample sizes along with additional animal – related information such as clinical signs, cow’s history of mastitis cases, somatic cell count, as well as herd information.
Conclusion
We developed a specific and sensitive technique for detecting and quantifying two of the most important bacteria that cause bovine mastitis, Streptococcus dysgalactiae and Streptococcus uberis. The direct comparison of dddPCR with other commonly used techniques for bacterial detection (i.e., endpoint PCR and bacteriological culture) allowed us to determine the detection limit of all the techniques analyzed. This work represents the first step towards consolidating the application of dddPCR to identify the etiological agents causing mastitis. The dddPCR is especially suitable for milk samples with low bacterial load or intermittently release. The proposed method enables quick control measures, such as segregating infected animals if they show clinical signs or high SCC.
Data availability statement
The original contributions presented in the study are included in the article/Supplementary Material. Further inquiries can be directed to the corresponding author.
Ethics statement
Ethical approval was not required for the study involving animals in accordance with the local legislation and institutional requirements because Veterinarians or dairy farmers sent milk samples from March to July 2021 from farms located in San José – Uruguay (34°20′20″S 56°42′37″O).
Author contributions
LD: Conceptualization, Data curation, Formal analysis, Funding acquisition, Investigation, Methodology, Project administration, Resources, Software, Validation, Writing – original draft, Writing – review & editing. GT: Data curation, Formal analysis, Investigation, Methodology, Software, Writing – review & editing. VD: Investigation, Methodology, Resources, Writing – review & editing. LC: Conceptualization, Writing – review & editing. JL: Conceptualization, Writing – review & editing. AI: Conceptualization, Data curation, Investigation, Methodology, Software, Writing – review & editing. RP: Conceptualization, Data curation, Investigation, Methodology, Supervision, Writing – review & editing.
Funding
The author(s) declare financial support was received for the research, authorship, and/or publication of this article. This work was supported by Agencia Nacional de Investigación e Innovación (ANII), Uruguay FMV_3_2020_1_162241 and Programa de Posgrado de la Facultad de Veterinaria, UdelaR, Uruguay.
Acknowledgments
GT, AI, and RP are members of the Sistema Nacional de Investigadores, Uruguay. LD is a scholarship recipient from Agencia Nacional de Investigación e Innovación (ANII), Uruguay.
Conflict of interest
The authors declare that the research was conducted in the absence of any commercial or financial relationships that could be construed as a potential conflict of interest.
The author(s) declared that they were an editorial board member of Frontiers, at the time of submission. This had no impact on the peer review process and the final decision.
Publisher’s note
All claims expressed in this article are solely those of the authors and do not necessarily represent those of their affiliated organizations, or those of the publisher, the editors and the reviewers. Any product that may be evaluated in this article, or claim that may be made by its manufacturer, is not guaranteed or endorsed by the publisher.
Supplementary material
The Supplementary Material for this article can be found online at: https://www.frontiersin.org/articles/10.3389/fanim.2023.1336816/full#supplementary-material
References
Altschul S. F., Gish W., Miller W., Myers E. W., Lipman D. J. (1990). Basic local alignment search tool. J. Mol. Biol. 215, 403–410. doi: 10.1016/S0022-2836(05)80360-2
Ashraf A., Imran M. (2018). Diagnosis of bovine mastitis: from laboratory to farm. Trop. Anim. Health Prod. 50, 1193–1202. doi: 10.1007/S11250-018-1629-0
Britten A. M. (2012). The role of diagnostic microbiology in mastitis control programs. Vet. Clin. North Am. - Food Anim. Pract. 28, 187–202. doi: 10.1016/j.cvfa.2012.03.006
Carroll E. J., Schalm O. W., LASMANIS J. (1964). Experimental coliform (aerobacter aerogenes) mastitis: characteristics. Am. J. Vet. Res. 25, 720–726.
Carvalho-Castro G. A., Silva J. R., Paiva L. V., Custódio D. A. C., Moreira R. O., Mian G. F., et al. (2017). Molecular epidemiology of Streptococcus agalactiae isolated from mastitis in Brazilian dairy herds. Braz. J. Microbiol. 48, 551–559. doi: 10.1016/J.BJM.2017.02.004
Chakraborty S., Dhama K., Tiwari R., Iqbal Yatoo M., Khurana S. K., Khandia R., et al. (2019). Technological interventions and advances in the diagnosis of intramammary infections in animals with emphasis on bovine population—a review. Vet. Q. 39, 76. doi: 10.1080/01652176.2019.1642546
Chen C. Y., Nace G. W., Irwin P. L. (2003). A 6x6 drop plate method for simultaneous colony counting and MPN enumeration of Campylobacter jejuni, Listeria monocytogenes, and Escherichia coli. J. Microbiol. Methods 55, 475–479. doi: 10.1016/S0167-7012(03)00194-5
Cremonesi P., Cortimiglia C., Picozzi C., Minozzi G., Malvisi M., Luini M., et al. (2016). Development of a droplet digital polymerase chain reaction for rapid and simultaneous identification of common foodborne pathogens in soft cheese. Front. Microbiol. 7. doi: 10.3389/FMICB.2016.01725
Davies P. L., Leigh J. A., Bradley A. J., Archer S. C., Emes R. D., Green M. J. (2016). Molecular epidemiology of streptococcus uberis clinical mastitis in dairy herds: Strain heterogeneity and transmission. J. Clin. Microbiol. 54, 68–74. doi: 10.1128/JCM.01583-15
De Brun M. L., Cosme B., Petersen M., Alvarez I., Folgueras-Flatschart A., Flatschart R., et al. (2022). Development of a droplet digital PCR assay for quantification of the proviral load of bovine leukemia virus. J. Vet. Diagn. Invest. 34, 439–447. doi: 10.1177/10406387221085581
Dohoo I. R., Meek A. H. (1982). Somatic cell counts in bovine milk. Can. Vet. J. = La Rev. Vet. Can. 23, 119–125.
Du Y., Yan Z., Song K., Jin J., Xiao L., Sun Z., et al. (2022). Development and evaluation of a multiplex droplet digital polymerase chain reaction method for simultaneous detection of five biothreat pathogens. Front. Microbiol. 13. doi: 10.3389/fmicb.2022.970973
Duarte C. M., Freitas P. P., Bexiga R. (2015). Technological advances in bovine mastitis diagnosis: an overview. J. Vet. Diagn. Invest. 27 (6), 665-672. doi: 10.1177/1040638715603087
Erskine R. J., Eberhart R. J. (1988). Comparison of duplicate and single quarter milk samples for the identification of intramammary infections. J. Dairy Sci. 71, 854–856. doi: 10.3168/JDS.S0022-0302(88)79627-7
Esener N., Green M. J., Emes R. D., Jowett B., Davies P. L., Bradley A. J., et al. (2018). Discrimination of contagious and environmental strains of Streptococcus uberis in dairy herds by means of mass spectrometry and machine-learning. Sci. Rep. 8, 1–12. doi: 10.1038/s41598-018-35867-6
Firdaus I. (2017). Laboratory handbook on bovine mastitis (Natl. Mastit. Counc). Available at: https://www.academia.edu/50651208/Laboratory_Handbook_on_Bovine_Mastitis (Accessed September 21, 2022).
Garcia A. (2004). Contagious vs. Environmental Mastitis. Available at: http://openprairie.sdstate.edu/extension_extra/126 (Accessed September 15, 2022).
Gianneechini R., Concha C., Delucci I., Gil J., Salvarrey L., Rivero R. (2014). Mastitis bovina, reconocimiento de los patógenos y su resistencia antimicrobiana en la Cuenca Lechera del Sur de Uruguay. Veterinaria 50, 111–132.
Gianneechini R., Concha C., Rivero R., Delucci I., Moreno López J. (2002). Occurrence of clinical and sub-clinical mastitis in dairy herds in the west littoral region in Uruguay. Acta Vet. Scand. 43, 221–230. doi: 10.1186/1751-0147-43-221
Gillespie B. E., Oliver S. P. (2005). Simultaneous detection of mastitis pathogens, Staphylococcus aureus, Streptococcus uberis, and Streptococcus agalactiae by multiplex real-time polymerase chain reaction. J. Dairy Sci. 88, 3510–3518. doi: 10.3168/jds.S0022-0302(05)73036-8
Huggett J. F., Foy C. A., Benes V., Emslie K., Garson J. A., Haynes R., et al. (2013). The digital MIQE guidelines: Minimum Information for Publication of Quantitative Digital PCR Experiments. Clin. Chem. 59, 892–902. doi: 10.1373/CLINCHEM.2013.206375
Jiang M., Babiuk L. A., Potter A. A. (1996). Cloning, sequencing and expression of the CAMP factor gene of Streptococcus uberis. Microb. Pathog. 20, 297–307. doi: 10.1006/MPAT.1996.0028
Kabelitz T., Aubry E., Van Vorst K., Amon T., Fulde M. (2021). microorganisms the role of streptococcus spp. in bovine mastitis. Microorganisms 9 (7), 1497. doi: 10.3390/microorganisms9071497
Kaczorek E., Małaczewska J., Wójcik R., Rękawek W., Siwicki A. (2017). Phenotypic and genotypic antimicrobial susceptibility pattern of Streptococcus spp. isolated from cases of clinical mastitis in dairy cattle in Poland. J. Dairy Sci. 100 (8), 6442–6453. doi: 10.3168/jds.2017-12660
Koskinen M. T., Wellenberg G. J., Sampimon O. C., Holopainen J., Rothkamp A., Salmikivi L., et al. (2010). Field comparison of real-time polymerase chain reaction and bacterial culture for identification of bovine mastitis bacteria. J. Dairy Sci. 93 (12), 5707–5715. doi: 10.3168/jds.2010-3167
Król J., Twardoń J., Mrowiec J., Podkowik M., Dejneka G., Debski B., et al. (2009). Short communication: Streptococcus canis is able to establish a persistent udder infection in a dairy herd. J. Dairy Sci. 98, 7090–7096. doi: 10.3168/JDS.2015-9454
Kromker V., Reinecke F., Paduch J.-H., Grabowski N. (2014). Bovine streptococcus uberis intramammary infections and mastitis. Clin. Microbiol. 3. doi: 10.4172/2327-5073.1000157
Mahmmod Y. (2015). The future of PCR technologies in diagnosis of bovine mastitis pathogens. Adv. Dairy Res. 02. doi: 10.4172/2329-888X.1000E106
Martin S. W. (1984). Estimating disease prevalence and the interpretation of screening. Prev. Vet. Med. 2, 463-472. doi: 10.1016/0167-5877(84)90091-6
McDermott G. P., Do D., Litterst C. M., Maar D., Hindson C. M., Steenblock E. R., et al. (2013). Multiplexed target detection using DNA-binding dye chemistry in droplet digital PCR. Anal. Chem. 85, 11619–11627. doi: 10.1021/AC403061N
Nonnemann B., Lyhs U., Svennesen L., Kristensen K. A., Klaas I. C., Pedersen K. (2019). Bovine mastitis bacteria resolved by MALDI-TOF mass spectrometry. J. Dairy Sci. 102, 2515–2524. doi: 10.3168/JDS.2018-15424
Page A. J., Cummins C. A., Hunt M., Wong V. K., Reuter S., Holden M. T. G., et al. (2015). Roary: rapid large-scale prokaryote pan genome analysis. Bioinformatics 31, 3691–3693. doi: 10.1093/BIOINFORMATICS/BTV421
Phuektes P., Mansell P. D., Browning G. F. (2001). Multiplex polymerase chain reaction assay for simultaneous detection of Staphylococcus aureus and streptococcal causes of bovine mastitis. J. Dairy Sci. 84, 1140–1148. doi: 10.3168/JDS.S0022-0302(01)74574-2
Pitkälä A., Gindonis V., Wallin H., Honkanen-Buzalski T. (2005). Interlaboratory proficiency testing as a tool for improving performance in laboratories diagnosing bovine mastitis. J. Dairy Sci. 88, 553–559. doi: 10.3168/jds.S0022-0302(05)72717-X
Powell E. A., Babady N. E. (2018). Digital PCR in the clinical microbiology laboratory: another tool on the molecular horizon. Clin. Microbiol. Newsl. 40, 27–32. doi: 10.1016/j.clinmicnews.2018.01.005
Rački N., Dreo T., Gutierrez-Aguirre I., Blejec A., Ravnikar M. (2014). Reverse transcriptase droplet digital PCR shows high resilience to PCR inhibitors from plant, soil and water samples. Plant Methods 10. doi: 10.1186/S13007-014-0042-6
Raemy A., Meylan M., Casati S., Gaia V., Berchtold B., Boss R., et al. (2013). Phenotypic and genotypic identification of streptococci and related bacteria isolated from bovine intramammary infections. Acta Vet. Scand. 55, 53. doi: 10.1186/1751-0147-55-53/FIGURES/2
Ramírez J. D., Herrera G., Hernández C., Cruz-Saavedra L., Muñoz M., Flórez C., et al. (2018). Evaluation of the analytical and diagnostic performance of a digital droplet polymerase chain reaction (ddPCR) assay to detect Trypanosoma cruzi DNA in blood samples. PloS Negl. Trop. Dis. 12, e0007063. doi: 10.1371/JOURNAL.PNTD.0007063
Riffon R., Sayasith K., Khalil H., Dubreuil P., Drolet M., Lagacé J. (2001). Development of a rapid and sensitive test for identification of major pathogens in bovine mastitis by PCR. J. Clin. Microbiol. 39, 2584–2589. doi: 10.1128/JCM.39.7.2584-2589.2001
Ruegg P. L. (2017). A 100-Year Review: Mastitis detection, management, and prevention. J. Dairy Sci. 100 (12), 10381–10397. doi: 10.3168/jds.2017-13023
Sears P. M., Smith B. S., English P. B., Herer P. S., Gonzalez R. N. (1990). Shedding pattern of staphylococcus aureus from bovine intramammary infections. J. Dairy Sci. 73, 2785–2789. doi: 10.3168/jds.S0022-0302(90)78964-3
Seemann T. (2014). Prokka: rapid prokaryotic genome annotation. Bioinformatics 30, 2068–2069. doi: 10.1093/BIOINFORMATICS/BTU153
Sharun K., Dhama K., Tiwari R., Bashir Gugjoo M., Iqbal Yatoo M., Kumar Patel S., et al. (2021). Advances in therapeutic and managemental approaches of bovine mastitis: a comprehensive review. Vet. Q. 41 (1), 107–136. doi: 10.1080/01652176.2021.1882713
Steele N. M., Williamson J. H., Thresher R., Laven R. A., Hillerton J. E. (2017). Evaluating a commercial PCR assay against bacterial culture for diagnosing Streptococcus uberis and Staphylococcus aureus throughout lactation. J. Dairy Sci. 100, 3816–3824. doi: 10.3168/jds.2016-11752
Untergasser A., Cutcutache I., Koressaar T., Ye J., Faircloth B. C., Remm M., et al. (2012). Primer3—new capabilities and interfaces. Nucleic Acids Res. 40, e115. doi: 10.1093/NAR/GKS596
Wente N., Krömker V. (2020). Streptococcus dysgalactiae-contagious or environmental? Animals 10 (11), 2185. doi: 10.3390/ani10112185
Wu J., Tang B., Qiu Y., Tan R., Liu J., Xia J., et al. (2022). Clinical validation of a multiplex droplet digital PCR for diagnosing suspected bloodstream infections in ICU practice: a promising diagnostic tool. Crit. Care 26. doi: 10.1186/s13054-022-04116-8
Yang R., Paparini A., Monis P., Ryan U. (2014). Comparison of next-generation droplet digital PCR (ddPCR) with quantitative PCR (qPCR) for enumeration of Cryptosporidium oocysts in faecal samples. Int. J. Parasitol. 44, 1105–1113. doi: 10.1016/J.IJPARA.2014.08.004
Zadoks R. N., Watts J. L. (2009). Species identification of coagulase-negative staphylococci: genotyping is superior to phenotyping. Vet. Microbiol. 134, 20–28. doi: 10.1016/J.VETMIC.2008.09.012
Keywords: ddPCR, molecular diagnosis, dairy cattle, bacterial pathogen, gram-positive
Citation: Diana L, Traglia G, Diana V, Calvinho L, Laporta J, Iriarte A and Puentes R (2024) Duplex droplet digital PCR detection of Streptococcus uberis and Streptococcus dysgalactiae, major etiological agents of bovine mastitis. Front. Anim. Sci. 4:1336816. doi: 10.3389/fanim.2023.1336816
Received: 11 November 2023; Accepted: 27 December 2023;
Published: 16 January 2024.
Edited by:
Pasquale De Palo, University of Bari Aldo Moro, ItalyReviewed by:
Marialaura Corrente, University of Bari Aldo Moro, ItalyGrazia Greco, University of Bari Aldo Moro, Italy
Copyright © 2024 Diana, Traglia, Diana, Calvinho, Laporta, Iriarte and Puentes. This is an open-access article distributed under the terms of the Creative Commons Attribution License (CC BY). The use, distribution or reproduction in other forums is permitted, provided the original author(s) and the copyright owner(s) are credited and that the original publication in this journal is cited, in accordance with accepted academic practice. No use, distribution or reproduction is permitted which does not comply with these terms.
*Correspondence: Leticia Diana, leticia.diana@fvet.edu.uy