Characteristics and Application of Rhodopseudomonas palustris as a Microbial Cell Factory
- 1Energy-Rich Compound Production by Photosynthetic Carbon Fixation Research Center, Shandong Key Lab of Applied Mycology, Qingdao Agricultural University, Qingdao, China
- 2College of Life Sciences, Qingdao Agricultural University, Qingdao, China
- 3Haiyang Comprehensive Administrative Law Enforcement Bureau (Agriculture), Haiyang, China
- 4Qingdao Garden Forestry Technology School, Qingdao, China
Rhodopseudomonas palustris, a purple nonsulfur bacterium, is a bacterium with the properties of extraordinary metabolic versatility, carbon source diversity and metabolite diversity. Due to its biodetoxification and biodegradation properties, R. palustris has been traditionally applied in wastewater treatment and bioremediation. R. palustris is rich in various metabolites, contributing to its application in agriculture, aquaculture and livestock breeding as additives. In recent years, R. palustris has been engineered as a microbial cell factory to produce valuable chemicals, especially photofermentation of hydrogen. The outstanding property of R. palustris as a microbial cell factory is its ability to use a diversity of carbon sources. R. palustris is capable of CO2 fixation, contributing to photoautotrophic conversion of CO2 into valuable chemicals. R. palustris can assimilate short-chain organic acids and crude glycerol from industrial and agricultural wastewater. Lignocellulosic biomass hydrolysates can also be degraded by R. palustris. Utilization of these feedstocks can reduce the industry cost and is beneficial for environment. Applications of R. palustris for biopolymers and their building blocks production, and biofuels production are discussed. Afterward, some novel applications in microbial fuel cells, microbial electrosynthesis and photocatalytic synthesis are summarized. The challenges of the application of R. palustris are analyzed, and possible solutions are suggested.
1 Introduction
Rhodopseudomonas palustris belonging to purple nonsulfur bacterium (PNSB) is widely distributed in nature, mainly in anaerobic water environments with sufficient light, such as lakes, soils, swamps, and the sea (Guan et al., 2017). The PNSB constitute a group of versatile organisms in which most exhibit four modes of metabolism: photoautotrophic, photoheterotrophic, chemoheterotrophic and chemoautotrophic, switching from one mode to another depending on conditions available (Figure 1) (Larimer et al., 2003). This metabolic versatility allows R. palustris to use light, inorganic, and organic compounds as its carbon and energy sources under anaerobic or aerobic conditions (Madigan and Jung, 2009). Therefore, R. palustris is of interest for all sorts of industrial and environmental applications.
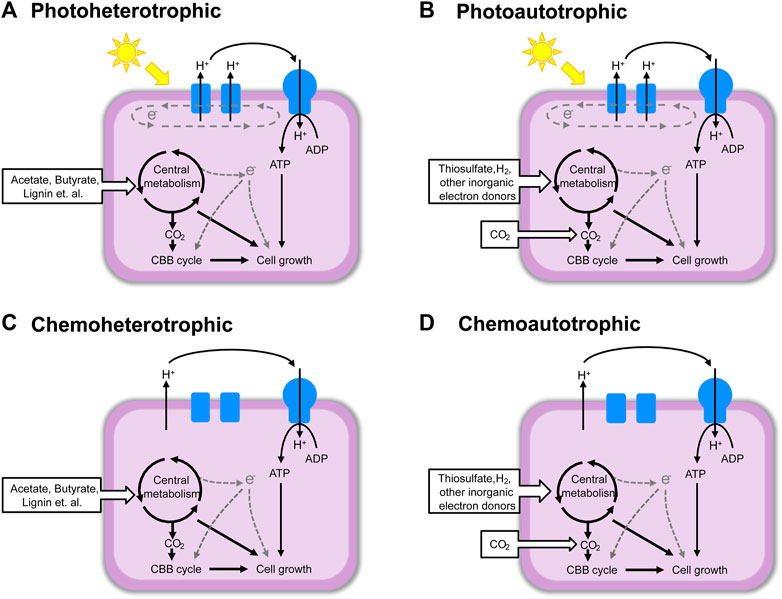
FIGURE 1. The four types of metabolism of R. palustris. (A) Photoheterotrophic; (B) Photoautotrophic; (C) Chemoheterotrophic; (D) Chemoautotrophic. This figure was modified according to the figure in reference (Larimer et al., 2003).
Traditionally, R. palustris is widely utilized in the areas of aquaculture industry and wastewater treatment. It is rich in biologically active chemicals, including proteins, vitamins, polysaccharides, pantothenic acid and folic acid, which contribute to its function as feed supplement. It can also increase the oxygen content of aquaculture water, stabilize the pH and purify the aquaculture water environment (Peirong and Wei, 2013). The addition of R. palustris to aquaculture water can improve the immunity of aquatic organisms and prevent diseases (Zhou et al., 2010). R. palustris has biodetoxification and biodegradation capacities toward components in livestock waste and industrial waste, such as lignin, nitride, chlorides and aromatic compounds (Harwood et al., 1998). Analysis of the complete genome sequence of R. palustris indicated a large inventory of genes encoding the four distinct ring cleavage pathways for degradation of the aromatic compounds, confirming its outstanding ability of biodegradation (Larimer et al., 2003).
In addition to the above applications, R. palustris has great potential to be applied in many other fields. As a microbial cell factory, R. palustris has been extensively researched for hydrogen production (McKinlay and Harwood, 2010b) via photo-fermentation or via complementation of photo-fermentation and dark-fermentation (Patel et al., 2012). Other biofuels production in R. palustris, such as methane (Fixen et al., 2016) and butanol (Doud et al., 2017), has also been reported. Production of valuable chemicals in R. palustris, such as poly-beta-hydroxybutyrate (PHB) (Wu et al., 2012), polysaccharide (Fritts et al., 2017) and isoprenoid (Xu et al., 2016), indicates the potential of R. palustris as a chassis organism for biopolymers and their building blocks production. Moreover, R. palustris can transfer electrons to solid electron acceptor such as electrode, enabling its application in microbial fuel cells (MFC) for electricity production (Lai et al., 2017). R. palustris also has the ability of electrons uptake from solid materials, leading to its application in the field of microbial electrosynthesis (MES) (Xing et al., 2008; Rengasamy et al., 2018). Similarly, R. palustris can absorb electrons from CdS nanoparticles attached to the cell surface, and a CdS-R. palustris hybrid system has been constructed for photocatalytic synthesis (Wang et al., 2019).
In this review, the advantages of R. palustris as a microbial cell factory were analyzed. Then, the applications of R. palustris in biopolymers and their building blocks production were discussed. Traditional application of R. palustris in the fields of wastewater treatment, bioremediation, agriculture, aquaculture and livestock breeding were summarized. Application in other fields, including biofuel production, microbial fuel cells, microbial electrosynthesis and photocatalytic synthesis, were also summarized. Finally, the challenges of applications of R. palustris are analyzed, and possible solutions are suggested.
2 Advantages of R. palustris as a Microbial Cell Factory
2.1 Utilization of Diverse Carbon Sources, Including CO2
R. palustris can utilize various carbon sources for growth, including small molecule organic acids, alcohols, inorganic and aromatic compounds, which are the main components in industrial waste (Table 1). R. palustris can absorb light as an energy source to produce ATP. Therefore, R. palustris has great advantages as a chassis organism in terms of carbon and energy sources.
First, R. palustris CGA009 can use inorganic matters as the carbon source, such as CO2, which is fixed by the Calvin Bassham Benson (CBB) cycle to participate in cell growth metabolism using thiosulfate as the electron donor (Huang et al., 2010). Generally, photoautotrophic cyanobacteria have received much attention in the field of photosynthesis of valuable biofuels and chemicals because they can fix CO2 through oxygenic photosynthesis with an higher efficiency than plant (Melis, 2009). PNSB, another group of photoautotrophic organisms, have received increasing attention in recent years. PNSB can capture CO2 under anaerobic conditions, a photoautotrophic mechanism very different from cyanobacteria (Grattieri, 2020). Furthermore, ribulose 1,5-bisphosphate (RuBP) carboxylase/oxygenase (RubisCO) is responsible for CO2 fixation by catalyzing the carboxylation of RuBP via the CBB cycle. Usually, the form I RubisCOs are phylogenetically divided into a green type, which is present in cyanobacteria, and a red type (Tabita, 1999; Badger and Bek, 2008; Tabita et al., 2008). Several red-type RubisCOs were demonstrated to have higher CO2/O2 specificity than green-type RubisCOs (Read and Tabita, 1994; Tabita, 1999). Most PNSB species, including R. palustris, contain the red-type form I RubisCO with higher CO2/O2 specificity (Swingley et al., 2009). Moreover, in R. palustris, a proportion of CO2 and electrons can be directly catalyzed by remodeled nitrogenases to produce methane (Fixen et al., 2016). In total, except for cyanobacteria, PNSB including R. palustris has garnered considerable attention for photosynthetic conversion of CO2 into value-added chemicals in recent years.
Second, R. palustris can degrade and utilize most short-chain organic acids such as acetate, butyrate, fumarate, succinate and lactate, which are often present in agricultural and industrial wastewater. The metabolism of acetate and butyrate assimilation is initiated by converting to acetyl-CoA, which is mainly assimilated by the glyoxylate shunt, with a few carbon flux entering into the TCA cycle and into pyruvate catalyzed by pyruvate dehydrogenase (McKinlay and Harwood, 2010a; McKinlay and Harwood, 2011). Fumarate and succinate are assimilated directly through the TCA cycle (McKinlay and Harwood, 2011). The assimilation pathway of lactate in R. palustris has not been reported; however, a gene (RPA3503) encoding D-lactate dehydrogenase and a gene (RPA1136) encoding lactate permease were predicated in the genome of R. palustris CGA009 (Larimer et al., 2003). Under different organic acid conditions, the growth of R. palustris and its productivity are different. Seven different organic acid salts were individually added into the culture medium of methane-producing R. palustris as carbon sources, and the highest yield of methane was obtained when fumarate was added (Fixen et al., 2016). R. palustris cannot readily use lactate as a sole carbon source, and coutilization with other substrates stimulated its consumption (Govindaraju et al., 2019). When different concentrations (2–10 mmol/L) of lactate were added into the culture medium, different hydrogen yields were obtained (Lazaro et al., 2017). Therefore, when R. palustris is utilized as a microbial cell factory, screen of the optimal carbon source is necessary.
Third, R. palustris can also use alcohols such as ethanol, crude glycerol and butanol as carbon sources. Crude glycerol is the main byproduct in biodiesel industry (Habe et al., 2009). Due to the rapid development of the biodiesel market, large amounts of crude glycerol are produced and treated as industrial waste (Sabourin-Provost and Hallenbeck, 2009). In R. palustris, crude glycerol can be converted to hydrogen at a conversion efficiency nearing 90% (Pott et al., 2013; Pott et al., 2014). Based on environmental and economic considerations, efficient utilization of crude glycerol in R. palustris is beneficial for its application as a microbial cell factory.
Last, R. palustris can also use multiple aromatic compounds as carbon sources. Utilization of lignocellulosic biomass hydrolysates, an abundant and renewable resource, is the research hotspot in recent years; however, the toxic aromatic compounds in the hydrolysates limit the utilization (Austin et al., 2015). R. palustris harbors a benzoate-degrading benzoyl-CoA pathway (Figure 2), through which the aromatic compounds in the hydrolysates are catabolized (Harwood and Gibson, 1988; Egland et al., 1997; Harwood et al., 1998). Conversion of lignocellulosic biomass to valuable chemicals in R. palustris is of great value in industrial application.
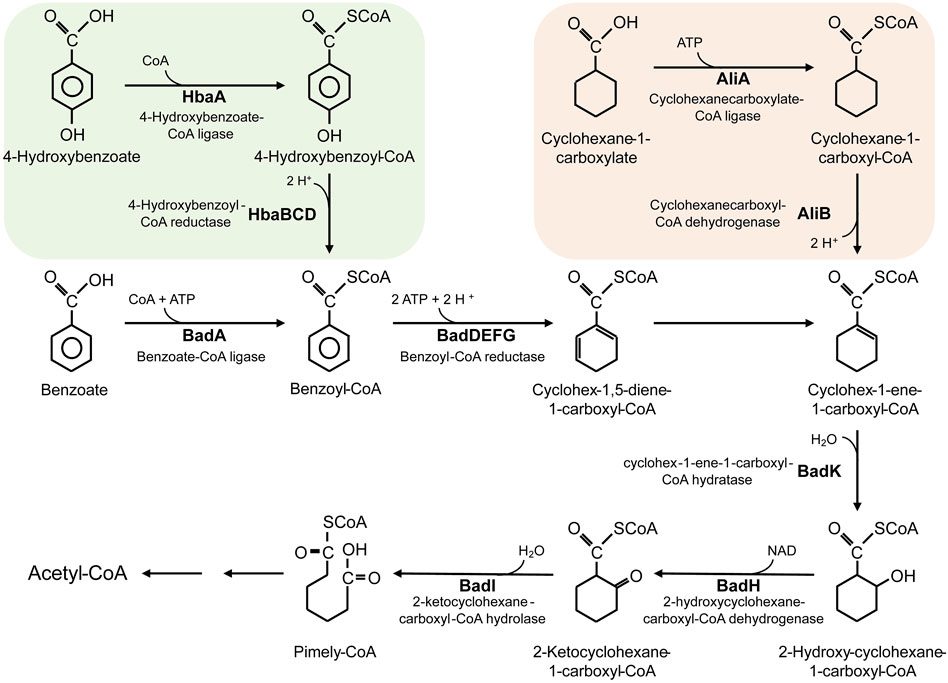
FIGURE 2. The metabolic pathway for degradation of benzoate, 4-hydroxybenzoate and cyclohexane-1-carboxylate in R. palustris.
In summary, due to the diversity of available carbon sources, R. palustris has great potential to be utilized as a microbial cell factory for valuable chemicals production, and environmental and economic factors can be considered at the same time.
2.2 Exploration of Genetic Engineering Strategies
Development of genetic engineering tools is necessary for manipulation at the genetic level, such as gene knockout and gene overexpression, which can modify the organism as desired. In R. palustris, a few gene editing tools have been reported up to date. A plasmid pMG101 (15 kb), which can replicate in PNSB, was obtained from R. palustris among 400 strains isolated from a natural environment. Then, based on pMG101, Escherichia coli-R. palustris shuttle vectors pMG103 (5.68 kb) and pMG105 (5.68 kb) were constructed, which were stably maintained in R. palustris (Inui et al., 2000). In R. palustris TIE-1, three genes crtE, hpnD and dxs were cloned into pMG103, and squalene production (15.8 mg/g DCW) was obtained (Xu et al., 2016). The pBBR1MCS series, as broad-host-range plasmids, can also be used for vector construction and gene expression in R. palustris. The adhE2 gene was cloned into the pBBR1MCS-2 plasmid, which was then transformed into R. palustris CGA009 for n-butyrate production (Doud et al., 2017). The overexpression of fix gene cluster in R. palustris was realized by cloning into the pBBR1MCS-5 plasmid (Huang et al., 2010). Other plasmids in the pBBR1MCS series with different antibiotic selectors, including pBBR1MCS-1, pBBR1MCS-3, and pBBR1MCS-4, can also be used for gene expression in R. palustris (Obranic et al., 2013). The pMG103 and pBBR1MCS harbor different origin of replication (ori) sources, and co-transformation of pMG103 and pBBR1MCS into R. palustris is promising, which is beneficial for overexpression of several genes in the same strain, broadening the application of R. palustris as a microbial cell factory.
In addition to gene overexpression, gene mutations mediated by suicide plasmid have also been reported in R. palustris. pJQ200SK is a suicide vector that is widely applied in Gram-negative bacteria, featuring the P15A ori, a gentamicin selection marker and the gene sacB from Bacillus subtilis, encoding sucrose-6-fructosyltransferase, which can catalyze the decomposition of sucrose to high-molecular fructan, which has a lethal effect on Gram-negative bacteria (Quandt and Hynes, 1993). The suicide plasmid pJQ200SK was used to insert the mutated nifA*571, nifA*574, and nifDV75AH201Q into the chromosome of R. palustris CGA009 to study the function of nitrogenase (Fixen et al., 2016).
To perform more molecular research of R. palustris for its further application and characterization, exploring more genetic editing tools is necessary and urgent. In another PNSB, Rhodobacter sphaeroides, several other vectors have also been used for gene expression aside from the pBBR1MCS series. The broad-host-range plasmids of the RK2 family, including pRK310, have been widely utilized in R. sphaeroides (Keen et al., 1988; Scott et al., 2003; Ryu et al., 2014). The strain harboring pRK310 showed a higher growth rate and higher gene expression levels than the strain harboring pBBR1MCS-2 (Scott et al., 2003). An IPTG-inducible plasmid (pIND4) was also constructed based on plasmid pMG160, and applied for gene expression (Ind et al., 2009; Qiang et al., 2019). In another PNSB, R. capsulatus, a set of novel broad-host-range vectors (pRho) with T7 promoters were constructed and further utilized for heterogeneous gene expression for sesquiterpenoids synthesis (Katzke et al., 2010; Troost et al., 2019). Application of these vectors in R. palustris is promising, satisfying the requirement of special expression mode in R. palustris.
In R. palustris, even though the tools for gene overexpression and gene deletion have been reported, the time-consuming genetic editing process limits the widespread application of R. palustris. Exploration of novel genetic editing tools is necessary. A SpCas9-sgRNA-based genomic DNA targeting system for R. sphaeroides was developed for gene knock-out, knock-in and single nucleotide substitutions, which indicates the development of a similar Cas9-based genome editing tool in R. palustris in future research (Mougiakos et al., 2019). In 2020, base editing systems for R. sphaeroides, cytosine base editors (CBEs) and adenine base editors (ABEs), all based on CRISPR/Cas9 systems, were also generated (Luo et al., 2020). Development of novel genetic modification tools in R. palustris would promote the application of R. palustris as a microbial cell factory.
3 Applications of R. palustris
3.1 Application in Biopolymers and Their Building Blocks
Biopolymers are biodegradable polymers that are synthesized from renewable resources by living organisms, which allow them to replace the fossil fuel–based polymers. R. palustris is rich in valuable compounds such as PHB, polysaccharide and isoprenoid, building blocks for biopolymers production, making it a promising microbial cell factory (Table 2).
3.1.1 Poly-Beta-Hydroxybutyrate Production
Biodegradable polymers, such as polyhydroxyalkanoates (PHAs), have been identified as potential alternatives to traditional plastics, which have been a heavy burden to the environment due to their recalcitrant nature (Kalia et al., 2021). Depending on the carbon atoms per monomer, PHAs can be classified as short-chain-length PHAs (scl-PHAs, C3–C5) and medium-chain-length PHAs (mcl-PHAs, C6–C14) (Li et al., 2016). PHAs are accumulated by many bacteria as a carbon- and energy- storage compound, and the most accumulated PHA is PHB, a polymer composed of 3-hydroxybutyrate, belonging to scl-PHAs (Lee, 1996). Although PHB is the most studied PHA in literature, the bioplastic products derived solely from it are typically brittle and stiff, which are undesirable in many applications (McAdam et al., 2020). Nonetheless, the material properties could be improved by adding 3-hydroxyvalerate units into a PHB biopolymer, obtaining copolymer poly(3-hydroxybutyrate-co-3-hydroxyvalerate), also named PHBV (Li et al., 2016). Due to their inherent biodegradability, excellent biocompatibility and non-toxicity, PHAs have been widely applied in the fields of agriculture, aquaculture, and human health sectors (Kalia et al., 2021).
PHB is produced as an energy- and carbon-storage compound by PNSB under special stress conditions (Monroy and Buitrón, 2020). Production of PHB from CO2 (carbon source) via photoautotrophy in R. palustris is considered to be sustainable and environmental-friendly. When the electron donor was a poised electrode (photoelectroautotrophy) or a ferrous iron (photoferroautotrophy), it showed the highest PHB electron yield (7.34%) and specific productivity (8.4 × 10−14 mg/cell/h) in R. palustris TIE-1 (Ranaivoarisoa et al., 2019). In the CdS-R. palustris hybrid system, the CdS nanoparticles cotaded on the surface of R. palustris formed by Cd2+ bioprecipitation through the cysteine desulfurase can absorb solar energy and release electrons, and the biomass, PHB and carotenoid production were improved by 148%, 147%, and 122%, respectively (Wang et al., 2019). PHB production in R. palustris from lignocellulosic biomass which is considered to be the most economic carbon source in the world has received much attention. R. palustris can utilize p-coumarate, the major lignin breakdown product, to produce PHB (Brown et al., 2020; Alsiyabi et al., 2021). R. palustris can produce a copolymer of PHB called PHBV, which has more ideal thermomechanical properties than PHB alone (Li et al., 2016). Phasin has been shown to control the size and number of granules in the cell, affect PHB accumulation, and promote localization of granules, and heterologous expression of phasin in R. palustris resulted in a significantly higher PHBV titer (0.7 g/L) from p-coumarate (Brown et al., 2021). PHB production from other carbon sources have also been researched, and PHB of different level are obtained with different substrates. Among the different short-chain organic acids utilized, only acetate and propionate can lead to the production of PHB in R. palustris, achieving 17.1% and 11.8% substrate conversion efficiency, respectively (Wu et al., 2012). Moreover, mixtures of acetate, propionate and butyrate as substrates had a significant effect on PHB production, 16.4 mg/g/day (Cardena et al., 2017). When acetate was used as the carbon source, under limiting ammonium concentrations, acetate was consumed through the glyoxylate pathway to produce PHB; however, under nitrogen starvation conditions, acetate was consumed through the TCA cycle and PHB production was increased by 30% (McKinlay et al., 2014). Some agroindustrial residues and energy crops were also investigated for PHB formation, and the highest PHB production, 11.53% TS, was obtained in olive pomace effluent (Corneli et al., 2016). In summary, PHB production in R. palustris from different carbon sources, like CO2, lignocellulosic biomass, short-chain organic acids, agroindustrial residues and energy crops, has been focused. However, in R. palustris, hydrogen production competes for the reducing equivalents and metabolites with PHB (Wu et al., 2012), genetic engineering to redirect more metabolic flux from hydrogen to PHB is proposed in future study.
3.1.2 Polysaccharide Production
Polysaccharide biopolymers are composed of mono saccharides linked together by O-glycosidic bonds (Hangasky et al., 2019). Due to their versatile properties like thickening, crosslinking and adsorption, polysaccharide biopolymers have been widely applied in the petroleum industry (Xia et al., 2020). Moreover, based on its properties of low production cost, nontoxicity and biocompatibility, polysaccharides is considered as green biopolymer for in situ gel formulation for drug delivery (Chowhan and Giri, 2020). R. palustris was reported to produce unipolar polysaccharide for biofilm formation under diverse conditions (Fritts et al., 2017). In the genome of R. palustris CGA009, uppE (RPA2750) and uppC (RPA4833) were identified to be responsible for unipolar polysaccharide production (Fritts et al., 2017). Unipolar polysaccharide production was enhanced in response to three photoheterotrophic conditions, including nutrient limitation, less-preferred nutrients and high salinity; however, cell growth rate was affected (Fritts et al., 2017). Design of the culture conditions of R. palustris is of critical importance to balance the cell growth and unipolar polysaccharide production, which affects the overall process economics. Further genetical modification would improve polysaccharide production.
3.1.3 Production of Building Blocks Belonging to Isoprenoids
Among the chemicals belonging to isoprenoids, isoprene and squalene are building blocks for biopolymer production. R. palustris is rich in carotenoids, another kind of isoprenoids, which include lycopene, rhodopin, 3,4-didehydro-rhodopin, anhydro-rhodovibrin, rhodovibrin, hydroxy-spirilloxanthin and spirilloxanthin (Chi et al., 2015). The produced carotenoids are connected to the transmembrane proteins through a noncovalent bond, which can stabilize the transmembrane proteins and capture light for the light harvesting complex in the cell membrane (Lang and Hunter, 1994; Takaichi, 2004). The precursors for isoprenoids production, isopentenyl diphosphate (IPP) and dimethylallyl diphosphate (DMAPP), are supplied by the methylerythritol phosphate (MEP) pathway or the mevalonate (MVA) pathway (Li et al., 2019). Genes of the MEP pathway and carotenoid synthetic pathway were identified in the genome of R. palustris (Figure 3). The high carotenoids content indicates that R. palustris has great potential to be developed as a microbial cell factory for isoprenoids production.
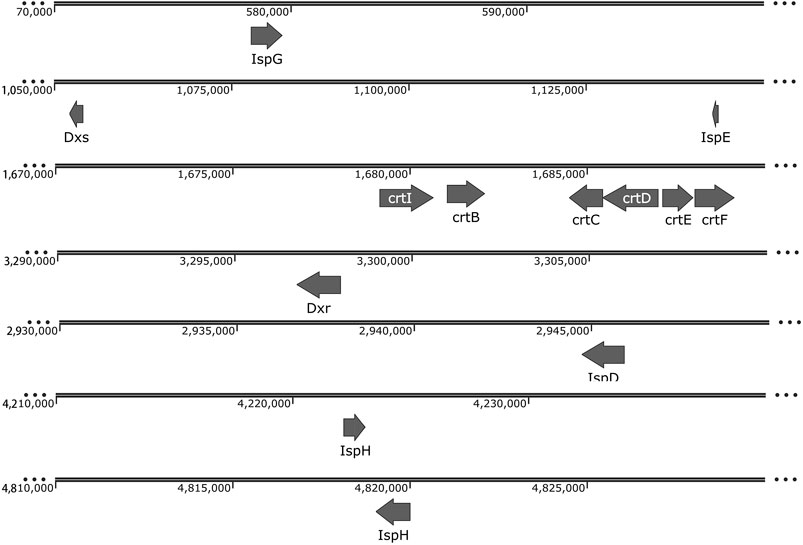
FIGURE 3. Genome distribution of genes in the carotenoid synthetic pathway in R. palustris. Abbreviations: 1-deoxy-D-xylulose-5-phosphate synthase (DXS); 1-deoxy-D-xylulose 5-phosphate reductoisomerase (DXR); 2-C-methyl-D-erythritol 4-phosphate cytidylyltransferase (IspD); 4-diphosphocytidyl-2-C-methyl-D-erythritol kinase (IspE); 4-hydroxy-3-methylbut-2-enyl diphosphate synthase (IspG); 4-hydroxy-3-methylbut-2-enyl diphosphate reductase (IspH); amine oxidase/Phytoene desaturase (crtI), squalene/phytoene synthase (crtB), hydroxyneurosporene synthase (crtC), FAD dependent oxidoreductase (crtD), polyprenyl synthetase (crtE), O-methyltransferase family 2 (crtF).
Carotenoids, such as lycopene and β-carotene, have great application value as food additives and in the pharmaceutical industry. Research about carotenoids production by R. palustris have been mainly focused on the changes in the culture conditions, such as light sources, light intensity, pH, carbon and nitrogen sources. Among the tested light sources, blue LED showed the highest growth, the highest carotenoid content (1,782 μg/g biomass) and the highest carotenoid productivity (1,800 μg/g/Watt) (Kuo et al., 2012). The light/dark cycle ratio and light intensities also influence the carotenoid yield, and light/dark cycle of 16 h/8 h and light intensity of 150 μmol-photons/m2/s were proven to be the best light parameters in R. palustris (Liu et al., 2019). Moreover, the composition of the carotenoids are different under different light illumination conditions (Muzziotti et al., 2017). Among the various carotenoids in R. palustris, the content of lycopene is the highest, which can reach 79% of the total carotenoids under certain light conditions (Muzziotti et al., 2017). Therefore, lycopene production in R. palustris is very promising. Considering the high total carotenoids production in R. palustris, further engineering at the gene level would enhance the metabolic flux to a specific carotenoid and isoprenoid.
Squalene production in R. palustris has been reported. Due to its antioxidant properties and unique structure, squalene is usually utilized extensively in the pharmaceutical, food, cosmetic and biofuel industries (Kim and Karadeniz, 2012). Squalene derivatives, such as tetramethylsqualene epoxides, botryoxanthins and braunixanthins, could be incorporated to resistant biopolymers by condensation with polyaldehydes (Okada et al., 2000). In R. palustris TIE-1, through deletion of the shc gene (encoding the squalene hopene cyclase), fusion of two consecutive enzymes (CrtE and HpnD) and overexpression of the dxs gene, squalene yield reached 15.8 mg/g (Xu et al., 2016). In another study, the yield of hopanoids, a downstream product of squalene, was more than 36.7 mg/g DCW in wild-type R. palustris TIE-1 under certain culture conditions (Welander et al., 2009), which indicates the high potential of further improvement of squalene production.
Isoprene is a platform chemical for natural rubber (cis-1,4-polyisoprene) production. Studies on the production of isoprene in R. palustris has not been reported; however, our group found that the wild type R. palustris can produce isoprene, which was usually produced in plant. Due to its high carotenoids-producing capacity, high isoprene production is promising in R. palustris. Isoprene is produced from precursor DMAPP catalyzed by isoprene synthase (Li et al., 2018). Except for the utilization of the native MEP pathway to accumulate DMAPP, heterologous expression of the MVA pathway in R. palustris would improve isoprenoid production dramatically. The plasmid harboring the enzymes of the MVA pathway in the vector pBBR1MCS-2 was transformed into another PNSB, R. sphaeroides, resulting in an 8-fold increase in amorphadiene production (Orsi et al., 2019). Transformation of the same plasmid into R. palustris is expected in future research.
In conclusion, R. palustris has great potential to produce biopolymers and their building blocks. R. palustris can accumulate biopolymers naturally, such as PHB and polysaccharide, under various conditions. Another beneficial property of R. palustris as a microbial cell factory is that industry waste, CO2 and lignocellulosic biomass can be converted to valuable biopolymers. The light-harvesting system in the cell membrane of R. palustris can capture light as an energy source, contributing to photosynthesis in R. palustris. Bioproduction of these valuable chemicals using many traditional chassis organisms, such as E. coli, Saccharomyces cerevisiae, and Corynebacterium glutamicum, has been researched in recent years, and high production has been realized. However, carbon and energy sources of these chassis organisms limit its sustainable and cost-effective application in industry. Therefore, in terms of economy of the fermentation process, R. palustris is more suitable as a microbial cell factory for valuable chemicals production. However, bioproduction in R. palustris suffers from the low growth rate, and improvement of growth rate is urgently required. Moreover, most research of R. palustris has focused on the optimization of culture conditions to improve chemicals accumulation in R. palustris; but genetic manipulation of R. palustris is seldomly executed due to its complicated genetical engineering process.
3.2 Traditional Application
3.2.1 Application in Wastewater Treatment and Bioremediation
R. palustris is widely applied in wastewater treatment and bioremediation as a model organism for its biodetoxification and biodegradation properties (Figure 4). Aromatic compounds in the wastewater, such as 3-chlorobenzoate (3-CBA), can be consumed by R. palustris as the sole carbon source through the innate benzoate-degrading pathway (Harwood et al., 1998). R. palustris WS17 was found to degrade 3-CBA anaerobically in the light; then, R. palustris DCP3 was found to degrade not only 3-CBA but also 2-CBA, 4-CBA and 3,5-CBA (Kamal and Wyndham, 1990; van der Woude et al., 1994; Krooneman et al., 1999). The degradation mechanism of 3-CBA in R. palustris RCB100 was deciphered, which includes three steps, reductive dechlorination of 3-CBA to 3-chlorobenzoyl coenzyme A, dehalogenation of 3-chlorobenzoyl-CoA to benzoyl-CoA, and degradation of benzoyl-CoA to acetyl-CoA (Egland et al., 2001). R. palustris can also grow on cyclohexane carboxylate (CHC), the simplest alicyclic acids, which is degraded to cyclohex-1-enecarboxyl-CoA (CHene-CoA), an intermediate of the benzoate-degrading pathway (Figure 2) (Küver et al., 1995; Hirakawa et al., 2015). Two transcription factors, BadR and BadM, were proven to regulate two operons expression related to the benzoate-degrading pathway (Hirakawa et al., 2015). Additionally, pyridine, a heterocyclic aromatic compound released into the environment as industrial waste, can be degraded by R. palustris JA1 as a sole carbon source for growth (Ramana et al., 2002). Another aromatic compound, phenol, is a toxic compound from the chemical industry and human activity, and it can be converted to 4-hydroxyphenylacetate in R. palustris PL1 (Noh et al., 2002). R. palustris CQV97 was isolated and identified as a microorganism that has the ability to degrade phenanthrene, a polycyclic aromatic compound (Zhao et al., 2011). In addition to aromatic compounds, utilization of taurine, a sulfonate, and degradation of polylactic acid (PLA), have also been reported (Novak et al., 2004; Hajighasemi et al., 2016). The biodegradable PLA is a promising candidate for the replacement of traditional petroleum-based plastics, and depolymerization and recycling utilization of PLA is essential to relieve environment pressure and save resources. The protein RPA1511 from R. palustris was identified to have the hydrolytic activity toward PLA (Hajighasemi et al., 2016).
R. palustris also has the capability of heavy metal tolerance and assimilation. Heavy metals are released in industrial processes, leading to contaminants in soils and water bodies. R. palustris is recognized as a model organism for heavy metal detoxification, especially for arsenic (As), a common soil contaminant. An As-redox transformation system exists in R. palustris that can reduce the highly toxic As5+ to As3+ and oxidize As3+ to methylated arsenic (low-toxic) (Batool et al., 2017). The As5+ resistance of R. palustris is up to 100 mM; and 62.9% of the toxic As5+ in the medium can be reduced (Batool et al., 2017). In the genome of R. palustris, three ars operons and four arsR genes related to As metabolism were identified (Zhao et al., 2015). Moreover, cobalt (Co) and nickel (Ni), serious pollutants with the development of industry, can also be assimilated by R. palustris. The Co-Ni transporter from R. palustris CGA009 was introduced into Deinococcus radiodurans R1, resulting in nearly 60% Co removal from the contaminated effluents within 90 min and was introduced into Nicotiana tabacum leading to a 5-fold Co acquisition and 2-fold Ni accumulation (Nair et al., 2012; Gogada et al., 2015). In addition, the biodetoxification of other heavy metals, including selenium (Se), uranium (U), cadmium (Cd) and zinc (Zn), have also been reported. After 9 days of cultivation, 99.9% of SeO32− was reduced to red elemental selenium in R. palustris strain N (Li et al., 2014). U can be reduced to U(IV)-phosphate or U(IV)-carboxylate compounds or accumulate in the cytoplasm and the cell wall in R. palustris (Llorens et al., 2012). Removal of Cd and Zn by 84% and 55%, respectively, were detected in R. palustris TN110 (Sakpirom et al., 2017).
In addition to heavy metals, R. palustris has high tolerance and degradation effects toward pesticides and herbicides, which are widely applied in agriculture and forestry. The residual pesticides and herbicides in the agro-ecosystem have caused significant environmental and human health concerns in recent years (Katsuda, 1999; Ray and Fry, 2006). Among them, pyrethroid pesticides have been extensively utilized for 30 years. R. palustris JSC-3b has the ability to degrade pyrethroid effectively; and the gene est3385, encoding a pyrethroid degradation ester, was identified in strain PSB-S (Zhang et al., 2014; Luo et al., 2018). Cyhalofop-butyl herbicides remaining in the soil can also cause serious environmental pollution and risk. The cyhalofop-butyl in soybean processing wastewater can be completely degraded after 5 days by R. palustris (Wu et al., 2019). Other toxic compounds detected in various environmental matrixes, such as hexabromocyclododecane and tributyl phosphate, which can cause serious human health problems, can also be degraded by R. palustris (Berne et al., 2005; Chang et al., 2020).
Food waste generated from restaurants and schools has caused many management problems in recent years. R. palustris CGA009 was reported to remove a wide variety of organic compounds, ammonia, nitrate and starch from food waste, and BOD and COD were reduced by 70% and 33%, respectively (Mekjinda and Ritchie, 2015).
An obvious characteristic of R. palustris is the possibility of simultaneous wastewater treatment and valuable chemicals bioproduction. When soybean processing wastewater was adopted as a carbon source, the toxic cyhalofop-butyl in the wastewater was degraded and the production of single cell protein, carotenoid and bacteriochlorophyll were enhanced (Wu et al., 2019). A maximum of 80 ml H2/g COD/day hydrogen was concurrently produced with efficient removal of BOD, COD, ammonia, nitrate, starch in the food waste (Mekjinda and Ritchie, 2015).
These outstanding functions make R. palustris a potential organism to be applied in wastewater treatment and bioremediation. However, due to the cell washout and unstable biodegradation, these traditional use of suspended R. palustris is inefficient. Immobilization microorganism technology, which loads bacteria into a solid carrier to enhance the abundance of bacteria in the wastewater, is attempted in recent years. The immobilized R. palustris with alginate, polyvinyl alcohol or agar beads showed 30%∼40% higher ammonia removal rate than that by free R. palustris (Zhan and Liu, 2013). R. palustris P1 was immobilized to glass pumice with a high adsorption capacity of 4.02 × 108 cells g−1, and the maximum NH4+-N and NO2−-N removal rates were 134.82 ± 0.67% and 93.68 ± 0.14% higher than those of free R. palustris P1, respectively (Xiao et al., 2019). The immobilized technology showed properties like high removal stability, easy to use in continuous reactors, high cell densities, and protecting the bacteria from predation by plankton, contributing to its highly effective aquaculture wastewater treatment.
3.2.2 Applications in Agriculture, Aquaculture and Livestock Breeding
R. palustris is traditionally utilized as a biofertilizer in agriculture, and as food additives and water purification microbes in aquaculture and livestock breeding. In agriculture, due to the detoxification and degradation properties of R. palustris mentioned above, it can be utilized to clean up the pollutants in the soil and improve the soil quality. An alternative method is to clone the specific genes from R. palustris into plants, which process endows the plants with the ability to tolerate and degrade heavy metals and pesticides in the soil. R. palustris also has the capability to promote plant growth due to its function of nitrogen fixation (Oda et al., 2005) and the production of two phytohormones, indole-3-acetic acid and ALA (Koh and Song, 2007). Plant Vigna mungo treated with R. palustris CS2 showed a 17% increase in shoot length and a 21.7% increase in root length (Batool et al., 2017). Additionally, R. palustris has the ability to induce resistance against plant disease, such as tobacco mosaic virus (TMV), one of the most destructive plant viruses. The Rhp-PSP protein with inhibitory activities against TMV in vivo and in vitro was isolated and purified from R. palustris JSC-3b (Su et al., 2015). An R. palustris GJ22 suspension was sprayed on tobacco, which induced tobacco resistance against TMV and enhanced the immune response under subsequent TMV infection (Su et al., 2017). The growth and germination of the tobacco were also promoted at the same time (Su et al., 2017). These outstanding properties, detoxification and degradation functions, plant growth promoting effects and anti-virus abilities, make R. palustris a potential candidate as a biofertilizer in agriculture.
R. palustris is also widely applied in aquaculture and livestock breeding in terms of its probiotic properties as feed additives and its wastewater treatment ability. Supplementing the drinking water of broiler chickens with R. palustris resulted in the growth promotion and the improvement of meat quality (Xu et al., 2014). The growth performance and immune response of tilapia (Oreochromis niloticus) were also improved by using R. palustris as a water additive (Zhou et al., 2010). In addition, the probiotic properties of R. palustris were further demonstrated in a model animal, rats (Fang et al., 2012). Tissue damage and bacteria translocation were not detected in rats, and no R. palustris remained in the feces of the rats 3 days after the termination of intake, indicating the biological safety of R. palustris as a probiotic bacterium (Fang et al., 2012). However, the molecular mechanism of its probiotic properties is not very clear. In 2019, an extracellular polysaccharide RPEPS-30 from R. palustris was found to enhance the host immune system and improve the growth of beneficial microbes in the gut (Zhang et al., 2019). As we mentioned above, R. palustris is suitable for wastewater treatment, which is generated from the aquaculture and livestock breeding industries. R. palustris WKU-KDNS3 was isolated from an animal waste lagoon, and its capability to eliminate skatole, a major contributor to the malodor emission from feces, was proven (Sharma et al., 2015). In another study, the isolated R. palustris from eutrophicated ponds can remove the odorous organic acids and phosphate swine wastewater (Kim et al., 2004). At present, research about the applications of R. palustris in the aquaculture and livestock industries have focused on the isolation of strains and demonstrations of their activity. Further analysis of the molecular basis of its probiotic properties is necessary, and subsequent genetic manipulation of R. palustris would improve its probiotic properties.
3.3 Application in Other Fields
3.3.1 Application in Biofuel Production
Considering the environmental pollution caused by the consumption of large amounts of nonrenewable fossil fuels, it is urgent to find alternative clean energy sources. Numerous studies have shown that R. palustris can produce biofuels, such as hydrogen, methane, ammonia and butanol (Table 3).
Ammonia is a clean fuel with features like high energy density, no carbon emission, low storage cost, difficult to explode (Bélanger-Chabot et al., 2015; Jiao and Xu, 2019). Ammonia is particularly considered as a strong option for long-haul shipping (Phillips et al., 2010; Zhao et al., 2021). Ammonia is chemically produced from hydrogen and nitrogen by the Haber-Bosch process that is conducted at a high temperature and high pressure in industry (Kandemir et al., 2013). In nature, ammonia can be produced by biological nitrogen fixation catalyzed by nitrogenase (Figure 5, Eqs 1–3), which is highly sensitive to oxygen (Oelze and Klein, 1996; Vitousek et al., 1997). In R. palustris, three different nitrogenase gene clusters, anf, vnf and nif, encoding three nitrogenase isozymes, were identified by genomic analysis, and further functional analysis demonstrated their function (Larimer et al., 2003; Oda et al., 2005). Different from algae and cyanobacteria, in which the inhibitory effect of oxygen on nitrogenase limits its wide application, R. palustris is an anoxygenic photosynthetic bacteria and the inhibitory effect of oxygen is absent (McKinlay and Harwood, 2010b). Hydrogen and methane production catalyzed by nitrogenase in R. palustris have also been reported. Actually, researchers have focused more on hydrogen and methane production than ammonia production by R. palustris.
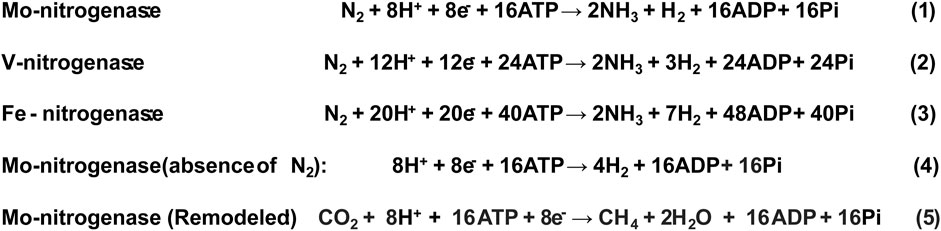
FIGURE 5. Stoichiometries of production of ammonia, hydrogen and methane through nitrogenase in R. palustris. The references were (McKinlay and Harwood, 2010b; Yang et al., 2012; Fixen et al., 2016; Harwood, 2020).
Hydrogen has been recognized as promising alternative due to properties like high conversion efficiency, renewability, clean burning nature and high mass-based energy density (Patel et al., 2012; Hu et al., 2018). Biological hydrogen production has significant potential in the hydrogen economy (Christopher and Dimitrios, 2012). Photo-fermentative hydrogen production by photosynthetic bacteria can be using energy directly from sunlight and reduced organic compounds (Hallenbeck and Ghosh, 2009). In R. palustris, hydrogen is produced as an obligate product during the nitrogen reduction process catalyzed by nitrogenase (McKinlay and Harwood, 2010b; Heiniger et al., 2012) (Figure 5, Eqs 1–3), even in the condition of nitrogen deficiency (Figure 5, Eq. 4) (McKinlay and Harwood, 2010b). In this process, electrons are obtained from organic or inorganic compounds and then transported to ferredoxin through the photosynthetic electron transport chain, which is utilized for hydrogen production via nitrogenase in combination with generated ATP (McKinlay and Harwood, 2010b). In R. palustris, the wide type nitrogenase consumed 75% reductant (ferredoxin) for ammonia production and the hydrogen production is at a low level (Rey et al., 2007). Moreover, the nitrogenase activity is inhibited by ammonia at the transcriptional and posttranslational level and is also inhibited by ADP, which competes with ATP for binding to the enzyme (Dixon and Kahn, 2004; Heiniger et al., 2012; Zheng and Harwood, 2019). Moreover, in R. palustris, PHB is synthesized as an energy- and carbon-storage compound, which competes with the metabolites and reducing equivalents for hydrogen production (Vincenzini et al., 1997). Some genetic engineering strategies were explored to improve hydrogen production efficiency, such as improvement of ammonium tolerance of the nitrogenase, elimination of PHB synthesis and disruption of the Calvin cycle. Regulatory gene nifA mutants, which lead to high nitrogenase expression even in the presence of ammonium, were screened, and up to five times hydrogen production (332 μmol/mg protein) relative to the wild type was detected (Rey et al., 2007). Disruption of the PHB synthesis gene phbC was performed in R. palustris WP3-5, and a 1.7-fold increase in hydrogen content (70%) was obtained (Yang and Lee, 2011). Hydrogen production can be improved by 4.6-fold to 145 μmol/mg protein in a ΔcbbLS ΔcbbM ΔcbbP mutant strain, in which electrons entering the Calvin cycle were blocked and redirected to hydrogen production (Zheng and Harwood, 2019). An obvious increase of hydrogen production has been obtained after genetic engineering strategies were applied.
Photo-fermentation of hydrogen suffers from the low light conversion efficiency and high energy demand by nitrogenase, leading to the low hydrogen yield. Most research on the improvement of hydrogen production in R. palustris has mainly focused on optimization of basic cultivation parameters, including the substrate, light intensity, pH, temperature and immobilization of the cells. Organic acids, such as acetate, propionate, malate and lactate, can be utilized as substrates to produce hydrogen by R. palustris WP3-5 (Wu et al., 2012). Four different carbon sources were evaluated for hydrogen production by R. palustris DSM127, and the highest hydrogen yield, 1.69, 2.38, 2.57, and 4.92 mol H2/mol substrate, were obtained using acetate (3 g/L), malate (2 g/L), lactate (2.5 g/L), and butyrate (2 g/L), respectively (Hu et al., 2018). However, when butyrate (2 g/L) was utilized as the carbon source, hydrogen production started after 400 h, longer than other carbon sources (Hu et al., 2018). Mixtures of different substrates were evaluated for the distribution of hydrogen and PHB production, and mixtures of propionate and acetate showed the highest hydrogen production, 391 ml/g/day (Cardena et al., 2017). The possibility of using various industrial and agricultural wastewater for hydrogen production has been widely investigated. Photofermentation of crude glycerol, a major side product of the biodiesel industry, to hydrogen was also studied in R. palustris, and the conversion rate was improved through adjusting the cultivation conditions (Sabourin-Provost and Hallenbeck, 2009; Ghosh et al., 2012; Pott et al., 2013). Agroindustrial residues and energy crops were also investigated for hydrogen production in R. palustris. For instance, a R. palustris CGA676 mutant cultured with wheat bran and maize effluent produced 648.6 and 320.3 ml/L hydrogen, respectively (Corneli et al., 2016). Light systems are also essential for hydrogen production. Two light systems, the fluorescent and incandescent, were investigated for cell growth and hydrogen production, and the incandescent system was proven to be more effective (Hu et al., 2018). Immobilization of R. palustris through biofilm formation and latex coatings formation can concentrate its biological reactivity and stabilize the microbes, which is beneficial for hydrogen production and light conversion efficiency (Liao et al., 2010; Tian et al., 2010). R. palustris CQK 01 was immobilized on the surface of baked glass beads to form a biofilm, and the bioreactor showed high hydrogen production, 38.9 ml/L/h and 0.2 mol/mol glucose (Tian et al., 2010). Latex coatings of R. palustris CGA009 were constructed in both dry and wet conditions, and high-level hydrogen production was detected (Gosse et al., 2012; Piskorska et al., 2013). In summary, hydrogen production by R. palustris has been widely studied, and abundant genetic engineering strategies and optimization of culture conditions have been performed.
Moreover, the intracellular redox balance can also influence hydrogen production in R. palustris. During photoheterotrophic conditions, R. palustris will produce a large amount of reducing equivalents, a part of which are consumed by nitrogenase for hydrogen production for intracellular redox balance (McKinlay and Harwood, 2011). However, the native Calvin cycle can also absorb a large section of the reducing equivalents (McKinlay and Harwood, 2010a; McKinlay and Harwood, 2011). The CbbRRS system in the Calvin cycle can respond to the redox signal and regulate the carbon flux (Romagnoli and Tabita, 2007; Laguna et al., 2010). Therefore, the Calvin cycle competes for reducing equivalents with hydrogen production. When the Calvin cycle was blocked in R. palustris, the hydrogen produced was enhanced on all substrates (McKinlay and Harwood, 2011). Hence, for hydrogen production, attention should be paid to the oxidation state of substrates and the Calvin cycle flux to avoid redox imbalance.
The efficiency of hydrogen production can be further improved via complementation of photo-fermentation and dark-fermentation. In this sequential two-stage dark- and photo-fermentation process, dark hydrogen production was conducted using acidogenic bacteria like Clostridium butyricum (Su et al., 2009a), C. pasteurianum (Chen et al., 2008), Caldicellulosiruptor saccharolyticus (Özgür et al., 2010), and E. coli (Sangani et al., 2019), and photo hydrogen production was conducted using photosynthetic bacteria, like R. palustris. A sequential dark- and photo-fermentation system including C. pasteurianum and R. palustris has been constructed for hydrogen production using sucrose as a feedstock (Chen et al., 2008). The overall hydrogen yield increased from the maximum of 3.8 mol H2/mol sucrose in dark fermentation to 10.02 mol H2/mol sucrose by sequential dark and photofermentation (Chen et al., 2008). In the sequential dark- and photo-fermentation system, photo-fermentation can utilize the resulting effluent from dark fermentation, mainly consisting of butyric and acetic acid. It is reported that the sequential dark- and photo-fermentation system could achieve a theoretically maximum hydrogen yield (Su et al., 2009b); therefore, is has been considered as an efficient system to increase hydrogen production yield.
Methane is a commonly used energy source, and nearly half of hydrogen production in industry is from methane. A purified Mo-dependent nitrogenase from Azotobacter vinelandii with two mutations, V70A and H195Q, in NifD near the FeMo cofactor, was proven to be capable of reducing carbon dioxide to methane in vitro (Yang et al., 2012). Then, homologous mutations, V75A and H201Q, in NifD from R. palustris, were produced and methane production from carbon dioxide was also detected in vivo (Figure 5, Eq. 5) (Fixen et al., 2016; Zheng and Harwood, 2019). Further optimization indicated that utilization of nongrowing cells which are not carrying out the competing biosynthesis can enhance the yield of methane by 9-fold to 900 nmol/mg total protein using fumarate as the substrate (Zheng and Harwood, 2019). The analogous mutation of the V-dependent nitrogenase (VnfDV57AH180Q) can also catalyze the synthesis of methane in R. palustris (Zheng et al., 2018). The wild type of another Fe-only nitrogenase in R. palustris also has the ability to synthesize methane, ammonia and hydrogen simultaneously, but the proportion of methane is relatively low (Zheng et al., 2018). With in-depth study of the mechanisms of nitrogenase, improving the ability of nitrogenase to synthesize hydrogen and methane through genetic engineering and synthetic biology methods are the direction of further research.
In addition, the production of butanol by R. palustris has also been reported. Compared to ethanol, butanol is an attractive renewable biofuel with a higher energy content, lower volatility, higher viscosity and higher intersolubility with traditional fuels (Jin et al., 2011). The adhE2 gene from Clostridium acetobutylicum ATCC 824 encodes alcohol-aldehyde dehydrogenase, which catalyzes the synthesis of n-butanol from n-butyrate, was expressed in R. palustris (Doud et al., 2017). A selectivity (butanol production from butyrate) up to 40%, close to the theoretical maximum selectivity 45%, was achieved in the engineered R. palustris (Doud et al., 2017). However, a very low n-butanol production rate, 0.03 g L−1 d−1, was obtained as a result of the slow growth of R. palustris, and the redox flux is speculated to be the limiting factor (Doud et al., 2017). Further engineering to improve its growth rate and metabolic rate is proposed to improve butanol and other biofuels production.
Biofuel production, mostly hydrogen, in R. palustris has been widely researched in the fields of diverse substrates and genetical modification to improve the metabolic flux. However, the low production yield still limits its application in industry. Combination of biofuel production with industrial and agricultural wastewater treatment or biodegradation might be a promising strategy to reduce cost.
3.3.2 Application in Microbial Fuel Cells, Microbial Electrosynthesis and Photocatalytic Synthesis
In recent years, some novel application fields were reported for R. palustris, utilizing its electron exchange ability with solid-phase materials, such as MCF, MES and photocatalytic synthesis (Figure 6). Construction of MFC has attracted much attention in recent years due to its electricity generation ability. In the MFC system, microbes can oxidize organic substrates in the anode and release electrons and protons, which are transferred to the cathode and react with oxygen to generate water, while generating electricity at the same time (Figure 6A) (Slate et al., 2019). Photo-microbial fuel cells (PMFC), which can convert light energy to electricity due to the photosynthetic activity of the photosynthetic microorganisms, are also being developed. R. palustris has been utilized as a model organism in PMFC. The power density of R. palustris DX-1 achieved 2,720 mW/m2 under light with acetate as the electron donor (Xing et al., 2008). Carbon sources from wastewater and contaminated sites can also be utilized as electron donors in the PMFC system. R. palustris RP2 can produce a current (power density 720 μW/m2) using petroleum hydrocarbons from oil contaminated sites as the energy source (Venkidusamy and Megharaj, 2016). In another study, R. palustris G11 could accumulate polyphosphate and PHB when a sufficient carbon source was provided, and the stored polyphosphate and PHB were further used for electricity production in a carbon source-insufficient condition (Lai et al., 2017). Power production under carbon source-insufficient conditions indicates the simultaneous application of PMFC for energy production and wastewater treatment, in which the carbon source is not enriched. Recently, a novel hybrid hydrogen-photosynthetic microbial fuel cell was constructed, which can produce hydrogen and a maximum power density of 2.39 mW/m2 in R. palustris ATCC 17007 at the same time (Pankan et al., 2020). The performance of PMFC may be affected by the electrode material, reactor, membrane, substrate and operating conditions (Aghababaie et al., 2015). Application of PMFC is mostly limited by its low power generation and expensive electrode materials, and research should be focused on improving the rate of electron transfer to/from the electrode and the exploration of advanced materials as the electrode.
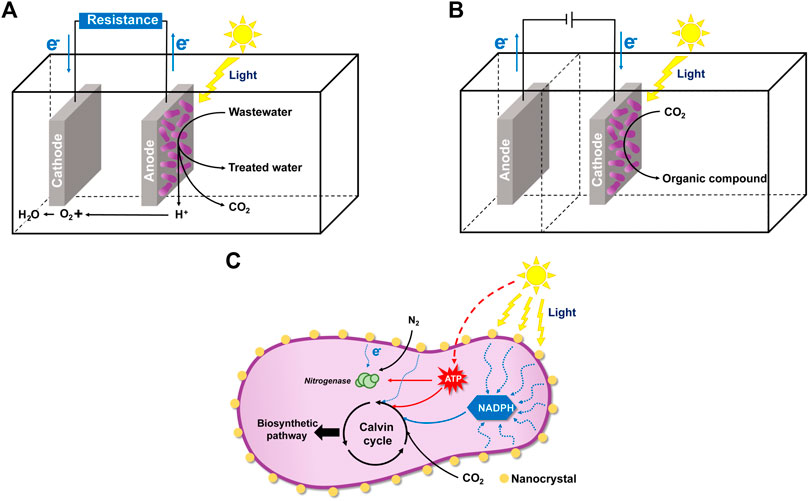
FIGURE 6. Illustration of the microbial fuel cell (MFC) and microbial electrosynthesis (MES) of R. palustris and CdS-R. palustris hybrid system. (A) In the MFC system, R. palustris in the anode can oxidize organic substrates in the wastewater and release electrons and protons. Electricity current is generated, and the protons are transferred to the cathode and react with oxygen to generate water. (B) In the MES system, the electrons from the cathode is absorbed by R. palustris for organic chemicals production from inorganic carbon dioxide and light energy. (C) In the CdS-R. palustris hybrid system, R. palustris can absorb electrons from the CdS nanoparticles (NPs) coated on its cell surface.
Microorganisms applied in MCF can release electrons to the solid-phase material and generate electricity. However, microorganisms can also absorb electrons from the cathode for MES, a bioelectrochemical process (Rabaey et al., 2011). In an MES system, desired chemicals are produced from an inorganic substrate and the absorbed electrons at the cathode-microbe interface (Figure 6B) (Karthikeyan et al., 2019). Photoautotrophic iron-oxidizing R. palustris TIE-1 can accept electrons from a solid-phase electrode, with carbon dioxide as the sole carbon source and electron acceptor (Bose et al., 2014). In R. palustris TIE-1, the pio operon is responsible for electron uptake from the electrode, and electron uptake stimulates ruBisCo form I expression, indicating the enhancement of carbon fixation during MES (Jiao and Newman, 2007; Bose et al., 2014). The major obstacle for using R. palustris TIE-1 for MES is the low electron uptake efficiency from cathode, which is reflected by the low maximum current density values (Ranaivoarisoa et al., 2019). To further enhance the electron uptake ability by R. palustris TIE-1, several iron-based redox mediators coated cathodes were tested and the immobilized Prussian blue (PB)-coated cathode was proven to be the best (Rengasamy et al., 2018). In another study, to enhance the electron uptake rates of R. palustris TIE-1, a photobioelectrochemical system with two uncoupled reactors, an electrochemical system and a photobioreactor, was developed, which had an electron uptake rate 56-fold higher than the single system (Doud and Angenent, 2014). In summary, the application of R. palustris in MES has been reported in recent years, but electron transfer efficiency is still the key factor limiting its efficiency. Actually, even after a decade of development, using MES for chemical synthesis cannot compete with the traditional fossil-fuel-derived production (Prevoteau et al., 2020). However, MES is still promising for chemical production from carbon dioxide, and the performance of MES can be improved.
R. palustris can also absorb electrons from the nanoparticles (NPs) coated on its cell surface. An inorganic-biological hybrid system, a CdS-R. palustris hybrid system, was constructed, in which CdS NPs coated on the cell surface can absorb solar energy, release electrons, then participate in various biosynthetic pathways (Figure 6C) (Wang et al., 2019). In this semiartificial photosynthetic platform, solar energy is directly utilized for the chemical production. CdS NPs on the surface of R. palustris are formed by Cd2+ bioprecipitation through the cysteine desulfurase (Bai et al., 2009). In the CdS-R. palustris hybrid system, carbon dioxide reduction was enhanced and the production of solid biomass, carotenoids and PHB were increased to 148%, 122%, and 147%, respectively (Wang et al., 2019). The synthesized CdS NPs can also locate to the cytoplasm, absorb solar energy, and enhance the nitrogen fixation catalyzed by nitrogenase (Sakpirom et al., 2019). Research about the nanoparticles-photosynthetic hybrid system is still in the initial stage. Further research on different nanomaterials and the genetic modification of R. palustris are expected to improve its catalytic efficiency.
4 Challenges and Possible Solutions
R. palustris has great potential for application in many fields: valuable chemicals production as a chassis organism; wastewater treatment and bioremediation for its biodetoxification and biodegradation properties; agriculture, aquaculture and livestock breeding as food additives; MCF, MES and photocatalytic synthesis for its electron exchange ability with solid materials. However, some properties of R. palustris limit its wide application. First, even though the versatile R. palustris can use a variety of carbon sources, its metabolic efficiency and growth rate are very low. Random mutation and screening are expected to improve its metabolic efficiency. Second, to our knowledge, except for a few gene expression vectors (pMG103 and pBBR1MCS series) and a gene knockout vector (pJQ200SK), no other genetic manipulation tools available in R. palustris have been reported. Exploring more genetic tools for R. palustris is necessary. Genetic manipulation tools, such as pRK404 and pIND4, which are available in other PNSBs, may be suitable for R. palustris. In addition, the implementation of some novel gene manipulation technologies, such as CRISPR-Cas and antisense RNA, in R. palustris is promising. Third, most of the research about R. palustris is at the physiological and biochemical levels, and genetic manipulation to enhance its efficiency is rarely reported. For example, for hydrogen production, most of the research has focused on the optimization of culture conditions to improve its production, and only limited research about the genetic manipulation of R. palustris has been reported. However, genetic manipulation could improve the properties of R. palustris significantly. Development of genetic engineering tools would facilitate genetic manipulation of R. palustris to improve its performance.
Author Contributions
ML and PN searched for the literatures and prepared the manuscript. YS helped to polish the manuscript. JL and JY put forward the concept of the manuscript and revised the manuscript. All authors have read and agreed to the published version of the manuscript.
Funding
This work was supported by grants from the “First class grassland science discipline” program in Shandong Province, the National Natural Science Foundation of China (31860011), Key Laboratory of Biobased Materials, Qingdao Institute of Bioenergy and Bioprocess Technology, Chinese Academy of Sciences (BMF-2020-01), the Talents of High Level Scientific Research Foundation (grants 6651120032, 6651117005, and 6651119011) of Qingdao Agricultural University, Natural Science Foundation of Shandong Province (Grant No. ZR2020QC069), and Key Laboratory of Biofuels, Qingdao Institute of Bioenergy and Bioprocess Technology, Chinese Academy of Sciences (CASKLB201805).
Conflict of Interest
The authors declare that the research was conducted in the absence of any commercial or financial relationships that could be construed as a potential conflict of interest.
Publisher’s Note
All claims expressed in this article are solely those of the authors and do not necessarily represent those of their affiliated organizations, or those of the publisher, the editors and the reviewers. Any product that may be evaluated in this article, or claim that may be made by its manufacturer, is not guaranteed or endorsed by the publisher.
References
Adessi, A., Concato, M., Sanchini, A., Rossi, F., and De Philippis, R. (2016). Hydrogen Production under Salt Stress Conditions by a Freshwater Rhodopseudomonas palustris Strain. Appl. Microbiol. Biotechnol. 100 (6), 2917–2926. doi:10.1007/s00253-016-7291-4
Aghababaie, M., Farhadian, M., Jeihanipour, A., and Biria, D. (2015). Effective Factors on the Performance of Microbial Fuel Cells in Wastewater Treatment - a Review. Environ. Technol. Rev. 4 (1), 71–89. doi:10.1080/09593330.2015.1077896
Alsiyabi, A., Brown, B., Immethun, C., Long, D., Wilkins, M., and Saha, R. (2021). Synergistic Experimental and Computational Approach Identifies Novel Strategies for Polyhydroxybutyrate Overproduction. Metab. Eng. 68, 1–13. doi:10.1016/j.ymben.2021.08.008
Austin, S., Kontur, W. S., Ulbrich, A., Oshlag, J. Z., Zhang, W., Higbee, A., et al. (2015). Metabolism of Multiple Aromatic Compounds in Corn Stover Hydrolysate by Rhodopseudomonas palustris. Environ. Sci. Technol. 49 (14), 8914–8922. doi:10.1021/acs.est.5b02062
Badger, M. R., and Bek, E. J. (2008). Multiple Rubisco Forms in Proteobacteria: Their Functional Significance in Relation to CO2 Acquisition by the CBB Cycle. J. Exp. Bot. 59 (7), 1525–1541. doi:10.1093/jxb/erm297
Bai, H. J., Zhang, Z. M., Guo, Y., and Yang, G. E. (2009). Biosynthesis of Cadmium Sulfide Nanoparticles by Photosynthetic Bacteria Rhodopseudomonas palustris. Colloids Surfaces B Biointerf. 70 (1), 142–146. doi:10.1016/j.colsurfb.2008.12.025
Batool, K., Tuz Zahra, F., and Rehman, Y. (2017). Arsenic-Redox Transformation and Plant Growth Promotion by Purple Nonsulfur Bacteria Rhodopseudomonas palustris CS2 and Rhodopseudomonas faecalis SS5. BioMed Res. Int. 2017, 1–8. doi:10.1155/2017/6250327
Bélanger‐Chabot, G., Rahm, M., Haiges, R., and Christe, K. O. (2015). Ammonia-(Dinitramido)boranes: High‐Energy‐Density Materials. Angew. Chem. Int. Ed. 54 (40), 11730–11734. doi:10.1002/anie.201505684
Berne, C., Allainmat, B., and Garcia, D. (2005). Tributyl Phosphate Degradation by Rhodopseudomonas palustris and Other Photosynthetic Bacteria. Biotechnol. Lett. 27 (8), 561–566. doi:10.1007/s10529-005-2882-7
Bose, A., Gardel, E. J., Vidoudez, C., Parra, E. A., and Girguis, P. R. (2014). Electron Uptake by Iron-Oxidizing Phototrophic Bacteria. Nat. Commun. 5, 3391. doi:10.1038/ncomms4391
Brown, B., Immethun, C., Alsiyabi, A., Long, D., Wilkins, M., and Saha, R. (2022). Heterologous Phasin Expression in Rhodopseudomonas palustris CGA009 for Bioplastic Production from Lignocellulosic Biomass. Metab. Eng. Commun. 14, e00191. doi:10.1016/j.mec.2021.e00191
Brown, B., Immethun, C., Wilkins, M., and Saha, R. (2020). Rhodopseudomonas palustris CGA009 Polyhydroxybutyrate Production from a Lignin Aromatic and Quantification via Flow Cytometry. Bioresour. Technol. Rep. 11, 100474. doi:10.1016/j.biteb.2020.100474
Cardeña, R., Valdez-Vazquez, I., and Buitrón, G. (2017). Effect of Volatile Fatty Acids Mixtures on the Simultaneous Photofermentative Production of Hydrogen and Polyhydroxybutyrate. Bioprocess Biosyst. Eng. 40 (2), 231–239. doi:10.1007/s00449-016-1691-9
Chang, T.-H., Wang, R., Peng, Y.-H., Chou, T.-H., Li, Y.-J., and Shih, Y.-h. (2020). Biodegradation of Hexabromocyclododecane by Rhodopseudomonas palustris YSC3 Strain: A Free-Living Nitrogen-Fixing Bacterium Isolated in Taiwan. Chemosphere 246, 125621. doi:10.1016/j.chemosphere.2019.125621
Chen, C.-Y., Yang, M.-H., Yeh, K.-L., Liu, C.-H., and Chang, J.-S. (2008). Biohydrogen Production Using Sequential Two-Stage Dark and Photo Fermentation Processes. Int. J. Hydrogen Energy 33 (18), 4755–4762. doi:10.1016/j.ijhydene.2008.06.055
Chi, S. C., Mothersole, D. J., Dilbeck, P., Niedzwiedzki, D. M., Zhang, H., Qian, P., et al. (2015). Assembly of Functional Photosystem Complexes in Rhodobacter sphaeroides Incorporating Carotenoids from the Spirilloxanthin Pathway. Biochim. Biophys. Acta Bioenerg. 1847 (2), 189–201. doi:10.1016/j.bbabio.2014.10.004
Chowhan, A., and Giri, T. K. (2020). Polysaccharide as Renewable Responsive Biopolymer for In Situ Gel in the Delivery of Drug through Ocular Route. Int. J. Biol. Macromol. 150, 559–572. doi:10.1016/j.ijbiomac.2020.02.097
Christopher, K., and Dimitrios, R. (2012). A Review on Exergy Comparison of Hydrogen Production Methods from Renewable Energy Sources. Energy Environ. Sci. 5 (5), 6640–6651. doi:10.1039/C2EE01098D
Corneli, E., Adessi, A., Dragoni, F., Ragaglini, G., Bonari, E., and De Philippis, R. (2016). Agroindustrial Residues and Energy Crops for the Production of Hydrogen and Poly-β-Hydroxybutyrate via Photofermentation. Bioresour. Technol. 216, 941–947. doi:10.1016/j.biortech.2016.06.046
Dixon, R., and Kahn, D. (2004). Genetic Regulation of Biological Nitrogen Fixation. Nat. Rev. Microbiol. 2 (8), 621–631. doi:10.1038/nrmicro954
Doud, D. F. R., and Angenent, L. T. (2014). Toward Electrosynthesis with Uncoupled Extracellular Electron Uptake and Metabolic Growth: Enhancing Current Uptake with Rhodopseudomonas palustris. Environ. Sci. Technol. Lett. 1 (9), 351–355. doi:10.1021/ez500244n
Doud, D. F. R., Holmes, E. C., Richter, H., Molitor, B., Jander, G., and Angenent, L. T. (2017). Metabolic Engineering of Rhodopseudomonas palustris for the Obligate Reduction of N-Butyrate to N-Butanol. Biotechnol. Biofuels 10 (1), 178. doi:10.1186/s13068-017-0864-3
Dutton, P. L., and Evans, W. C. (1969). The Metabolism of Aromatic Compounds by Rhodopseudomonas palustris. A New, Reductive, Method of Aromatic Ring Metabolism. Biochem. J. 113, 525–536. doi:10.1042/bj1130525
Egland, P. G., Gibson, J., and Harwood, C. S. (2001). Reductive, Coenzyme A-Mediated Pathway for 3-Chlorobenzoate Degradation in the Phototrophic Bacterium Rhodopseudomonas palustris. Appl. Environ. Microbiol. 67 (3), 1396–1399. doi:10.1128/aem.67.3.1396-1399.2001
Egland, P. G., Pelletier, D. A., Dispensa, M., Gibson, J., and Harwood, C. S. (1997). A Cluster of Bacterial Genes for Anaerobic Benzene Ring Biodegradation. Proc. Natl. Acad. Sci. U.S.A. 94, 6484–6489. doi:10.1073/pnas.94.12.6484
Fang, L. C., Li, Y., Cheng, P., Deng, J., Jiang, L. L., Huang, H., et al. (2012). Characterization of Rhodopseudomonas palustris Strain 2C as a Potential Probiotic. Apmis 120 (9), 743–749. doi:10.1111/j.1600-0463.2012.02902.x
Fixen, K. R., Zheng, Y., Harris, D. F., Shaw, S., Yang, Z.-Y., Dean, D. R., et al. (2016). Light-driven Carbon Dioxide Reduction to Methane by Nitrogenase in a Photosynthetic Bacterium. Proc. Natl. Acad. Sci. U.S.A. 113 (36), 10163–10167. doi:10.1073/pnas.1611043113
Fritts, R. K., LaSarre, B., Stoner, A. M., Posto, A. L., and McKinlay, J. B. (2017). A Rhizobiales-Specific Unipolar Polysaccharide Adhesin Contributes to Rhodopseudomonas palustris Biofilm Formation across Diverse Photoheterotrophic Conditions. Appl. Environ. Microbiol. 83 (4), e03035–03016. doi:10.1128/AEM.03035-16
Ghosh, D., Sobro, I. F., and Hallenbeck, P. C. (2012). Stoichiometric Conversion of Biodiesel Derived Crude Glycerol to Hydrogen: Response Surface Methodology Study of the Effects of Light Intensity and Crude Glycerol and Glutamate Concentration. Bioresour. Technol. 106, 154–160. doi:10.1016/j.biortech.2011.12.021
Gogada, R., Singh, S. S., Lunavat, S. K., Pamarthi, M. M., Rodrigue, A., Vadivelu, B., et al. (2015). Engineered Deinococcus Radiodurans R1 with NiCoT Genes for Bioremoval of Trace Cobalt from Spent Decontamination Solutions of Nuclear Power Reactors. Appl. Microbiol. Biotechnol. 99 (21), 9203–9213. doi:10.1007/s00253-015-6761-4
Gosse, J. L., Chinn, M. S., Grunden, A. M., Bernal, O. I., Jenkins, J. S., Yeager, C., et al. (2012). A Versatile Method for Preparation of Hydrated Microbial-Latex Biocatalytic Coatings for Gas Absorption and Gas Evolution. J. Ind. Microbiol. Biotechnol. 39 (9), 1269–1278. doi:10.1007/s10295-012-1135-8
Govindaraju, A., McKinlay, J. B., and LaSarre, B. (2019). Phototrophic Lactate Utilization by Rhodopseudomonas palustris is Stimulated by Coutilization with Additional Substrates. Appl. Environ. Microbiol. 85 (11), e00048–00019. doi:10.1128/AEM.00048-19
Grattieri, M. (2020). Purple Bacteria Photo-Bioelectrochemistry: Enthralling Challenges and Opportunities. Photochem. Photobiol. Sci. 19 (4), 424–435. doi:10.1039/c9pp00470j
Guan, C.-j., Ji, Y.-j., Hu, J.-l., Hu, C.-n., Yang, F., and Yang, G.-e. (2017). Biotransformation of Rutin Using Crude Enzyme from Rhodopseudomonas palustris. Curr. Microbiol. 74 (4), 431–436. doi:10.1007/s00284-017-1204-3
Habe, H., Fukuoka, T., Kitamoto, D., and Sakaki, K. (2009). Biotechnological Production of D-Glyceric Acid and its Application. Appl. Microbiol. Biotechnol. 84 (3), 445–452. doi:10.1007/s00253-009-2124-3
Hajighasemi, M., Nocek, B. P., Tchigvintsev, A., Brown, G., Flick, R., Xu, X., et al. (2016). Biochemical and Structural Insights into Enzymatic Depolymerization of Polylactic Acid and Other Polyesters by Microbial Carboxylesterases. Biomacromolecules 17 (6), 2027–2039. doi:10.1021/acs.biomac.6b00223
Hallenbeck, P. C., and Ghosh, D. (2009). Advances in Fermentative Biohydrogen Production: The Way Forward? Trends Biotechnol. 27 (5), 287–297. doi:10.1016/j.tibtech.2009.02.004
Hangasky, J. A., Detomasi, T. C., and Marletta, M. A. (2019). Glycosidic Bond Hydroxylation by Polysaccharide Monooxygenases. Trends Chem. 1 (2), 198–209. doi:10.1016/j.trechm.2019.01.007
Harwood, C. S., Burchhardt, G., Herrmann, H., and Fuchs, G. (1998). Anaerobic Metabolism of Aromatic Compounds via the Benzoyl-CoA Pathway. FEMS Microbiol. Rev. 22 (5), 439–458. doi:10.1111/j.1574-6976.1998.tb00380.x
Harwood, C. S., and Gibson, J. (1988). Anaerobic and Aerobic Metabolism of Diverse Aromatic Compounds by the Photosynthetic Bacterium Rhodopseudomonas palustris. Appl. Environ. Microbiol. 54, 712–717. doi:10.1128/aem.54.3.712-717.1988
Harwood, C. S. (2020). Iron-Only and Vanadium Nitrogenases: Fail-Safe Enzymes or Something More? Annu. Rev. Microbiol. 74 (1), 247–266. doi:10.1146/annurev-micro-022620-014338
Heiniger, E. K., Oda, Y., Samanta, S. K., and Harwood, C. S. (2012). How Posttranslational Modification of Nitrogenase is Circumvented in Rhodopseudomonas palustris Strains that Produce Hydrogen Gas Constitutively. Appl. Environ. Microbiol. 78 (4), 1023–1032. doi:10.1128/aem.07254-11
Hirakawa, H., Hirakawa, Y., Greenberg, E. P., and Harwood, C. S. (2015). BadR and BadM Proteins Transcriptionally Regulate Two Operons Needed for Anaerobic Benzoate Degradation by Rhodopseudomonas palustris. Appl. Environ. Microbiol. 81 (13), 4253–4262. doi:10.1128/aem.00377-15
Hu, C., Choy, S.-Y., and Giannis, A. (2018). Evaluation of Lighting Systems, Carbon Sources, and Bacteria Cultures on Photofermentative Hydrogen Production. Appl. Biochem. Biotechnol. 185 (1), 257–269. doi:10.1007/s12010-017-2655-5
Huang, J. J., Heiniger, E. K., McKinlay, J. B., and Harwood, C. S. (2010). Production of Hydrogen Gas from Light and the Inorganic Electron Donor Thiosulfate by Rhodopseudomonas palustris. Appl. Environ. Microbiol. 76 (23), 7717–7722. doi:10.1128/aem.01143-10
Ind, A. C., Porter, S. L., Brown, M. T., Byles, E. D., de Beyer, J. A., Godfrey, S. A., et al. (2009). Inducible-Expression Plasmid for Rhodobacter sphaeroides and Paracoccus denitrificans. Appl. Environ. Microbiol. 75 (20), 6613–6615. doi:10.1128/AEM.01587-09
Inui, M., Roh, J. H., Zahn, K., and Yukawa, H. (2000). Sequence Analysis of the Cryptic Plasmid pMG101 from Rhodopseudomonas palustris and Construction of Stable Cloning Vectors. Appl. Environ. Microbiol. 66 (1), 54–63. doi:10.1128/aem.66.1.54-63.2000
Jiao, F., and Xu, B. (2019). Electrochemical Ammonia Synthesis and Ammonia Fuel Cells. Adv. Mat. 31, 1805173. doi:10.1002/adma.201805173
Jiao, Y., and Newman, D. K. (2007). The Pio Operon is Essential for Phototrophic Fe(II) Oxidation in Rhodopseudomonas palustris TIE-1. J. Bacteriol. 189 (5), 1765–1773. doi:10.1128/JB.00776-06
Jin, C., Yao, M., Liu, H., Lee, C.-f. F., and Ji, J. (2011). Progress in the Production and Application of N-Butanol as a Biofuel. Renew. Sustain. Energy Rev. 15 (8), 4080–4106. doi:10.1016/j.rser.2011.06.001
Kalia, V. C., Singh Patel, S. K., Shanmugam, R., and Lee, J.-K. (2021). Polyhydroxyalkanoates: Trends and Advances Toward Biotechnological Applications. Bioresour. Technol. 326, 124737. doi:10.1016/j.biortech.2021.124737
Kamal, V. S., and Wyndham, R. C. (1990). Anaerobic Phototrophic Metabolism of 3-Chlorobenzoate by Rhodopseudomonas palustris WS17. Appl. Environ. Microbiol. 56 (12), 3871–3873. doi:10.1128/aem.56.12.3871-3873.1990
Kandemir, T., Schuster, M. E., Senyshyn, A., Behrens, M., and Schlögl, R. (2013). The Haber-Bosch Process Revisited: On the Real Structure and Stability of “Ammonia Iron” Under Working Conditions. Angew. Chem. Int. Ed. 52 (48), 12723–12726. doi:10.1002/anie.201305812
Karthikeyan, R., Singh, R., and Bose, A. (2019). Microbial Electron Uptake in Microbial Electrosynthesis: A Mini-Review. J. Ind. Microbiol. Biotechnol. 46 (9–10), 1419–1426. doi:10.1007/s10295-019-02166-6
Katsuda, Y. (1999). Development of and Future Prospects for Pyrethroid Chemistry. Pestic. Sci. 55 (8), 775–782. doi:10.1002/(sici)1096-9063(199908)55:8<775:aid-ps27>3.0.co;2-n
Katzke, N., Arvani, S., Bergmann, R., Circolone, F., Markert, A., Svensson, V., et al. (2010). A Novel T7 RNA Polymerase Dependent Expression System for High-Level Protein Production in the Phototrophic Bacterium Rhodobacter capsulatus. Protein Expr. Purif. 69 (2), 137–146. doi:10.1016/j.pep.2009.08.008
Keen, N. T., Tamaki, S., Kobayashi, D., and Trollinger, D. (1988). Improved Broad-Host-Range Plasmids for DNA Cloning in Gram-Negative Bacteria. Gene 70 (1), 191–197. doi:10.1016/0378-1119(88)90117-5
Kim, M. K., Choi, K.-M., Yin, C.-R., Lee, K.-Y., Im, W.-T., Lim, J. H., et al. (2004). Odorous Swine Wastewater Treatment by Purple Non-sulfur Bacteria, Rhodopseudomonas palustris, Isolated from Eutrophicated Ponds. Biotechnol. Lett. 26 (10), 819–822. doi:10.1023/b:bile.0000025884.50198.67
Kim, S.-K., and Karadeniz, F. (2012). “Biological Importance and Applications of Squalene and Squalane,” in Advances in Food and Nutrition Research. Editor S.-K. Kim (Waltham, MA: Academic Press), 223–233. doi:10.1016/b978-0-12-416003-3.00014-7
Koh, R. H., and Song, H. G. (2007). Effects of Application of Rhodopseudomonas spp. On Seed Germination and Growth of Tomato under Axenic Conditions. J. Microbiol. Biotechnol. 17 (11), 1805–1810.
Krooneman, J., van den Akker, S., Pedro Gomes, T. M., Forney, L. J., and Gottschal, J. C. (1999). Degradation of 3-Chlorobenzoate under Low-Oxygen Conditions in Pure and Mixed Cultures of the Anoxygenic Photoheterotroph Rhodopseudomonas palustris DCP3 and an Aerobic Alcaligenes Species. Appl. Environ. Microbiol. 65 (1), 131–137. doi:10.1128/AEM.65.1.131-137.1999
Kuo, F.-S., Chien, Y.-H., and Chen, C.-J. (2012). Effects of Light Sources on Growth and Carotenoid Content of Photosynthetic Bacteria Rhodopseudomonas palustris. Bioresour. Technol. 113, 315–318. doi:10.1016/j.biortech.2012.01.087
Küver, J., Xu, Y., and Gibson, J. (1995). Metabolism of Cyclohexane Carboxylic Acid by the Photosynthetic bacteriumRhodopseudomonas Palustris. Arch. Microbiol. 164 (5), 337–345. doi:10.1007/BF02529980
Laguna, R., Joshi, G. S., Dangel, A. W., Luther, A. K., and Tabita, F. R. (2010). Integrative Control of Carbon, Nitrogen, Hydrogen, and Sulfur Metabolism: the Central Role of the Calvin-Benson-Bassham Cycle. Adv. Exp. Med. Biol. 675, 265–271. doi:10.1007/978-1-4419-1528-3_15
Lai, Y.-C., Liang, C.-M., Hsu, S.-C., Hsieh, P.-H., and Hung, C.-H. (2017). Polyphosphate Metabolism by Purple Non-sulfur Bacteria and its Possible Application on Photo-Microbial Fuel Cell. J. Biosci. Bioeng. 123 (6), 722–730. doi:10.1016/j.jbiosc.2017.01.012
Lang, H. P., and Hunter, C. N. (1994). The Relationship Between Carotenoid Biosynthesis and the Assembly of the Light-Harvesting LH2 Complex in Rhodobacter sphaeroides. Biochem. J. 298, 197–205. doi:10.1042/bj2980197
Larimer, F. W., Chain, P., Hauser, L., Lamerdin, J., Malfatti, S., Do, L., et al. (2003). Complete Genome Sequence of the Metabolically Versatile Photosynthetic Bacterium Rhodopseudomonas palustris. Nat. Biotechnol. 22 (1), 55–61. doi:10.1038/nbt923
Lazaro, C. Z., Hitit, Z. Y., and Hallenbeck, P. C. (2017). Optimization of the Yield of Dark Microaerobic Production of Hydrogen from Lactate by Rhodopseudomonas palustris. Bioresour. Technol. 245 (Pt A), 123–131. doi:10.1016/j.biortech.2017.08.207
Lee, S. Y. (1996). Bacterial Polyhydroxyalkanoates. Biotechnol. Bioeng. 49 (1), 1–14. doi:10.1002/(SICI)1097-0290(19960105)49:1<1::AID-BIT1>3.0.CO;2-P
Li, B., Liu, N., Li, Y., Jing, W., Fan, J., Li, D., et al. (2014). Reduction of Selenite to Red Elemental Selenium by Rhodopseudomonas palustris Strain N. PLoS One 9 (4), e95955. doi:10.1371/journal.pone.0095955
Li, M., Hou, F., Wu, T., Jiang, X., Li, F., Liu, H., et al. (2019). Recent Advances of Metabolic Engineering Strategies in Natural Isoprenoid Production Using Cell Factories. Nat. Prod. Rep. 37 (1), 80–99. doi:10.1039/c9np00016j
Li, M., Nian, R., Xian, M., and Zhang, H. (2018). Metabolic Engineering for the Production of Isoprene and Isopentenol by Escherichia coli. Appl. Microbiol. Biotechnol. 102 (18), 7725–7738. doi:10.1007/s00253-018-9200-5
Li, Z., Yang, J., and Loh, X. J. (2016). Polyhydroxyalkanoates: Opening Doors for a Sustainable Future. NPG Asia Mater 8 (4), e265. doi:10.1038/am.2016.48
Liao, Q., Wang, Y.-J., Wang, Y.-Z., Zhu, X., Tian, X., and Li, J. (2010). Formation and Hydrogen Production of Photosynthetic Bacterial Biofilm under Various Illumination Conditions. Bioresour. Technol. 101 (14), 5315–5324. doi:10.1016/j.biortech.2010.02.019
Liu, S., Daigger, G. T., Kang, J., and Zhang, G. (2019). Effects of Light Intensity and Photoperiod on Pigments Production and Corresponding Key Gene Expression of Rhodopseudomonas palustris in a Photobioreactor System. Bioresour. Technol. 294, 122172. doi:10.1016/j.biortech.2019.122172
Liu, S., Zhang, G., Zhang, J., Li, X., and Li, J. (2016). Performance, Carotenoids Yield and Microbial Population Dynamics in a Photobioreactor System Treating Acidic Wastewater: Effect of Hydraulic Retention Time (HRT) and Organic Loading Rate (OLR). Bioresour. Technol. 200, 245–252. doi:10.1016/j.biortech.2015.10.044
Liu, Y., Ghosh, D., and Hallenbeck, P. C. (2015). Biological Reformation of Ethanol to Hydrogen by Rhodopseudomonas palustris CGA009. Bioresour. Technol. 176, 189–195. doi:10.1016/j.biortech.2014.11.047
Llorens, I., Untereiner, G., Jaillard, D., Gouget, B., Chapon, V., and Carriere, M. (2012). Uranium Interaction with Two Multi-Resistant Environmental Bacteria: Cupriavidus Metallidurans CH34 and Rhodopseudomonas palustris. PLoS One 7 (12), e51783. doi:10.1371/journal.pone.0051783
Luo, X., Zhang, D., Zhou, X., Du, J., Zhang, S., and Liu, Y. (2018). Cloning and Characterization of a Pyrethroid Pesticide Decomposing Esterase Gene, Est3385, from Rhodopseudomonas palustris PSB-S. Sci. Rep. 8 (1), 7384. doi:10.1038/s41598-018-25734-9
Luo, Y., Ge, M., Wang, B., Sun, C., Wang, J., Dong, Y., et al. (2020). CRISPR/Cas9-deaminase Enables Robust Base Editing in Rhodobacter sphaeroides 2.4.1. Microb. Cell. Fact. 19 (1), 93. doi:10.1186/s12934-020-01345-w
Madigan, M. T., and Jung, D. O. (2009). “An Overview of Purple Bacteria: Systematics, Physiology, and Habitats,” in The Purple Phototrophic Bacteria. Editors C. N. Hunter, F. Daldal, M. C. Thurnauer, and J. T. Beatty (Dordrecht: Springer Netherlands), 1–15. doi:10.1007/978-1-4020-8815-5_1
McAdam, B., Brennan Fournet, M., McDonald, P., and Mojicevic, M. (2020). Production of Polyhydroxybutyrate (PHB) and Factors Impacting its Chemical and Mechanical Characteristics. Polymers 12 (12), 2908. doi:10.3390/polym12122908
McKinlay, J. B., and Harwood, C. S. (2011). Calvin Cycle Flux, Pathway Constraints, and Substrate Oxidation State Together Determine the H 2 Biofuel Yield in Photoheterotrophic Bacteria. mBio 2 (2), e00323–10. doi:10.1128/mBio.00323-10
McKinlay, J. B., and Harwood, C. S. (2010a). Carbon Dioxide Fixation as a Central Redox Cofactor Recycling Mechanism in Bacteria. Proc. Natl. Acad. Sci. U.S.A. 107 (26), 11669–11675. doi:10.1073/pnas.1006175107
McKinlay, J. B., and Harwood, C. S. (2010b). Photobiological Production of Hydrogen Gas as a Biofuel. Curr. Opin. Biotechnol. 21 (3), 244–251. doi:10.1016/j.copbio.2010.02.012
McKinlay, J. B., Oda, Y., Rühl, M., Posto, A. L., Sauer, U., and Harwood, C. S. (2014). Non-growing Rhodopseudomonas palustris Increases the Hydrogen Gas Yield from Acetate by Shifting from the Glyoxylate Shunt to the Tricarboxylic Acid Cycle. J. Biol. Chem. 289 (4), 1960–1970. doi:10.1074/jbc.M113.527515
Mekjinda, N., and Ritchie, R. J. (2015). Breakdown of Food Waste by Anaerobic Fermentation and Non-Oxygen Producing Photosynthesis Using a Photosynthetic Bacterium. Waste Manag. 35, 199–206. doi:10.1016/j.wasman.2014.10.018
Melis, A. (2009). Solar Energy Conversion Efficiencies in Photosynthesis: Minimizing the Chlorophyll Antennae to Maximize Efficiency. Plant Sci. 177 (4), 272–280. doi:10.1016/j.plantsci.2009.06.005
Monroy, I., and Buitrón, G. (2020). Production of Polyhydroxybutyrate by Pure and Mixed Cultures of Purple Non-Sulfur Bacteria: A Review. J. Biotechnol. 317, 39–47. doi:10.1016/j.jbiotec.2020.04.012
Mougiakos, I., Orsi, E., Ghiffary, M. R., Post, W., de Maria, A., Adiego-Perez, B., et al. (2019). Efficient Cas9-Based Genome Editing of Rhodobacter sphaeroides for Metabolic Engineering. Microb. Cell. Fact. 18 (1), 204. doi:10.1186/s12934-019-1255-1
Muzziotti, D., Adessi, A., Faraloni, C., Torzillo, G., and De Philippis, R. (2017). Acclimation Strategy of Rhodopseudomonas palustris to High Light Irradiance. Microbiol. Res. 197, 49–55. doi:10.1016/j.micres.2017.01.007
Nair, S., Joshi-Saha, A., Singh, S., V., R., Singh, S., Thorat, V., et al. (2012). Evaluation of Transgenic Tobacco Plants Expressing a Bacterial Co-ni Transporter for Acquisition of Cobalt. J. Biotechnol. 161 (4), 422–428. doi:10.1016/j.jbiotec.2012.07.191
Noh, U., Heck, S., and Kohring, G.-W. (2002). Phototrophic Transformation of Phenol to 4-Hydroxyphenylacetate by Rhodopseudomonas palustris. Appl. Microbiol. Biotechnol. 58 (6), 830–835. doi:10.1007/s00253-002-0954-3
Novak, R. T., Gritzer, R. F., Leadbetter, E. R., and Godchaux, W. (2004). Phototrophic Utilization of Taurine by the Purple Nonsulfur Bacteria Rhodopseudomonas palustris and Rhodobacter sphaeroides. Microbiology 150 (6), 1881–1891. doi:10.1099/mic.0.27023-0
Obranić, S., Babić, F., and Maravić-Vlahoviček, G. (2013). Improvement of pBBR1MCS Plasmids, a Very Useful Series of Broad-Host-Range Cloning Vectors. Plasmid 70 (2), 263–267. doi:10.1016/j.plasmid.2013.04.001
Oda, Y., Samanta, S. K., Rey, F. E., Wu, L., Liu, X., Yan, T., et al. (2005). Functional Genomic Analysis of Three Nitrogenase Isozymes in the Photosynthetic Bacterium Rhodopseudomonas palustris. J. Bacteriol. 187 (22), 7784–7794. doi:10.1128/JB.187.22.7784-7794.2005
Oelze, J. X. R., and Klein, G. (1996). Control of Nitrogen Fixation by Oxygen in Purple Nonsulfur Bacteria. Archives Microbiol. 165 (4), 219–225. doi:10.1007/s002030050319
Okada, S., Devarenne, T. P., and Chappell, J. (2000). Molecular Characterization of Squalene Synthase from the Green Microalga Botryococcus braunii, Race B. Archives Biochem. Biophys. 373 (2), 307–317. doi:10.1006/abbi.1999.1568
Orsi, E., Folch, P. L., Monje-López, V. T., Fernhout, B. M., Turcato, A., Kengen, S. W. M., et al. (2019). Characterization of Heterotrophic Growth and Sesquiterpene Production by Rhodobacter sphaeroides on a Defined Medium. J. Ind. Microbiol. Biotechnol. 46 (8), 1179–1190. doi:10.1007/s10295-019-02201-6
Özgür, E., Mars, A. E., Peksel, B., Louwerse, A., Yücel, M., Gündüz, U., et al. (2010). Biohydrogen Production from Beet Molasses by Sequential Dark and Photofermentation. Int. J. Hydrogen Energy 35 (2), 511–517. doi:10.1016/j.ijhydene.2009.10.094
Pakpour, F., Najafpour, G., Tabatabaei, M., Tohidfar, M., and Younesi, H. (2014). Biohydrogen Production from CO-rich Syngas via a Locally Isolated Rhodopseudomonas palustris PT. Bioprocess Biosyst. Eng. 37 (5), 923–930. doi:10.1007/s00449-013-1064-6
Pankan, A. O., Yunus, K., and Fisher, A. C. (2020). Mechanistic Evaluation of the Exoelectrogenic Activity of Rhodopseudomonas palustris under Different Nitrogen Regimes. Bioresour. Technol. 300, 122637. doi:10.1016/j.biortech.2019.122637
Patel, S. K. S., Kumar, P., and Kalia, V. C. (2012). Enhancing Biological Hydrogen Production through Complementary Microbial Metabolisms. Int. J. Hydrogen Energy 37 (14), 10590–10603. doi:10.1016/j.ijhydene.2012.04.045
Peirong, Z., and Wei, L. (2013). Use of Fluidized Bed Biofilter and Immobilized Rhodopseudomonas palustris for Ammonia Removal and Fish Health Maintenance in a Recirculation Aquaculture System. Aquac. Res. 44 (3), 327–334. doi:10.1111/j.1365-2109.2011.03038.x
Phillips, C. J. C., Pines, M. K., Latter, M., Muller, T., Petherick, J. C., Norman, S. T., et al. (2010). The Physiological and Behavioral Responses of Steers to Gaseous Ammonia in Simulated Long-Distance Transport by Ship. J. Anim. Sci. 88 (11), 3579–3589. doi:10.2527/jas.2010-3089
Piskorska, M., Soule, T., Gosse, J. L., Milliken, C., Flickinger, M. C., Smith, G. W., et al. (2013). Preservation of H2production Activity in Nanoporous Latex Coatings of Rhodopseudomonas palustris CGA009 During Dry Storage at Ambient Temperatures. Microb. Biotechnol. 6 (5), 515–525. doi:10.1111/1751-7915.12032
Pott, R. W. M., Howe, C. J., and Dennis, J. S. (2013). Photofermentation of Crude Glycerol from Biodiesel Using Rhodopseudomonas palustris: Comparison with Organic Acids and the Identification of Inhibitory Compounds. Bioresour. Technol. 130, 725–730. doi:10.1016/j.biortech.2012.11.126
Pott, R. W. M., Howe, C. J., and Dennis, J. S. (2014). The Purification of Crude Glycerol Derived from Biodiesel Manufacture and its Use as a Substrate by Rhodopseudomonas palustris to Produce Hydrogen. Bioresour. Technol. 152, 464–470. doi:10.1016/j.biortech.2013.10.094
Prévoteau, A., Carvajal-Arroyo, J. M., Ganigué, R., and Rabaey, K. (2020). Microbial Electrosynthesis from CO2: Forever a Promise? Curr. Opin. Biotechnol. 62, 48–57. doi:10.1016/j.copbio.2019.08.014
Qiang, S., Su, A. P., Li, Y., Chen, Z., Hu, C. Y., and Meng, Y. H. (2019). Elevated β-Carotene Synthesis by the Engineered Rhodobacter Sphaeroides with Enhanced CrtY Expression. J. Agric. Food Chem. 67 (34), 9560–9568. doi:10.1021/acs.jafc.9b02597
Quandt, J., and Hynes, M. F. (1993). Versatile Suicide Vectors Which Allow Direct Selection for Gene Replacement in Gram-Negative Bacteria. Gene 127 (1), 15–21. doi:10.1016/0378-1119(93)90611-6
Rabaey, K., Girguis, P., and Nielsen, L. K. (2011). Metabolic and Practical Considerations on Microbial Electrosynthesis. Curr. Opin. Biotechnol. 22 (3), 371–377. doi:10.1016/j.copbio.2011.01.010
Ramana, C. V., Arunasri, K., and Sasikala, C. (2002). Photobiodegradation of Pyridine by Rhodopseudomonas palustris JA1. Indian J. Exp. Biol. 40 (8), 967–970.
Ranaivoarisoa, T. O., Singh, R., Rengasamy, K., Guzman, M. S., and Bose, A. (2019). Towards Sustainable Bioplastic Production Using the Photoautotrophic Bacterium Rhodopseudomonas palustris TIE-1. J. Ind. Microbiol. Biotechnol. 46 (9), 1401–1417. doi:10.1007/s10295-019-02165-7
Ray, D. E., and Fry, J. R. (2006). A Reassessment of the Neurotoxicity of Pyrethroid Insecticides. Pharmacol. Ther. 111 (1), 174–193. doi:10.1016/j.pharmthera.2005.10.003
Read, B. A., and Tabita, F. R. (1994). High Substrate Specificity Factor Ribulose Bisphosphate Carboxylase/oxygenase from Eukaryotic Marine Algae and Properties of Recombinant Cyanobacterial Rubisco Containing “algal” Residue Modifications. Arch. Biochem. Biophys. 312 (1), 210–218. doi:10.1006/abbi.1994.1301
Rengasamy, K., Ranaivoarisoa, T., Singh, R., and Bose, A. (2018). An Insoluble Iron Complex Coated Cathode Enhances Direct Electron Uptake by Rhodopseudomonas palustris TIE-1. Bioelectrochemistry 122, 164–173. doi:10.1016/j.bioelechem.2018.03.015
Rey, F. E., Heiniger, E. K., and Harwood, C. S. (2007). Redirection of Metabolism for Biological Hydrogen Production. Appl. Environ. Microbiol. 73 (5), 1665–1671. doi:10.1128/aem.02565-06
Romagnoli, S., and Tabita, F. R. (2007). Phosphotransfer Reactions of the CbbRRS Three-Protein Two- Component System from Rhodopseudomonas Palustris CGA010 Appear to Be Controlled by an Internal Molecular Switch on the Sensor Kinase. J. Bacteriol. 189 (2), 325–335. doi:10.1128/jb.01326-06
Ryu, M.-H., Hull, N. C., and Gomelsky, M. (2014). Metabolic Engineering of Rhodobacter sphaeroides for Improved Hydrogen Production. Int. J. Hydrogen Energy 39 (12), 6384–6390. doi:10.1016/j.ijhydene.2014.02.021
Sabourin-Provost, G., and Hallenbeck, P. C. (2009). High Yield Conversion of a Crude Glycerol Fraction from Biodiesel Production to Hydrogen by Photofermentation. Bioresour. Technol. 100 (14), 3513–3517. doi:10.1016/j.biortech.2009.03.027
Sakpirom, J., Kantachote, D., Nunkaew, T., and Khan, E. (2017). Characterizations of Purple Non-Sulfur Bacteria Isolated from Paddy Fields, and Identification of Strains with Potential for Plant Growth-Promotion, Greenhouse Gas Mitigation and Heavy Metal Bioremediation. Res. Microbiol. 168 (3), 266–275. doi:10.1016/j.resmic.2016.12.001
Sakpirom, J., Kantachote, D., Siripattanakul-Ratpukdi, S., McEvoy, J., and Khan, E. (2019). Simultaneous Bioprecipitation of Cadmium to Cadmium Sulfide Nanoparticles and Nitrogen Fixation by Rhodopseudomonas palustris TN110. Chemosphere 223, 455–464. doi:10.1016/j.chemosphere.2019.02.051
Sangani, A. A., McCully, A. L., LaSarre, B., and McKinlay, J. B. (2019). Fermentative Escherichia coli Makes a Substantial Contribution to H2 Production in Coculture with Phototrophic Rhodopseudomonas palustris. FEMS Microbiol. Lett. 366 (14), fnz162. doi:10.1093/femsle/fnz162
Scott, H. N., Laible, P. D., and Hanson, D. K. (2003). Sequences of Versatile Broad-Host-Range Vectors of the RK2 Family. Plasmid 50 (1), 74–79. doi:10.1016/S0147-619X(03)00030-1
Sharma, N., Doerner, K. C., Alok, P. C., and Choudhary, M. (2015). Skatole Remediation Potential of Rhodopseudomonas palustris WKU-KDNS3 Isolated from an Animal Waste Lagoon. Lett. Appl. Microbiol. 60 (3), 298–306. doi:10.1111/lam.12379
Slate, A. J., Whitehead, K. A., Brownson, D. A. C., and Banks, C. E. (2019). Microbial Fuel Cells: An Overview of Current Technology. Renew. Sustain. Energy Rev. 101, 60–81. doi:10.1016/j.rser.2018.09.044
Su, H., Cheng, J., Zhou, J., Song, W., and Cen, K. (2009a). Combination of Dark- and Photo-Fermentation to Enhance Hydrogen Production and Energy Conversion Efficiency. Int. J. Hydrogen Energy 34 (21), 8846–8853. doi:10.1016/j.ijhydene.2009.09.001
Su, H., Cheng, J., Zhou, J., Song, W., and Cen, K. (2009b). Improving Hydrogen Production from Cassava Starch by Combination of Dark and Photo Fermentation. Int. J. Hydrogen Energy 34 (4), 1780–1786. doi:10.1016/j.ijhydene.2008.12.045
Su, P., Feng, T., Zhou, X., Zhang, S., Zhang, Y., Cheng, J. e., et al. (2015). Isolation of Rhp-PSP, a Member of YER057c/YjgF/UK114 Protein Family with Antiviral Properties, from the Photosynthetic Bacterium Rhodopseudomonas palustris Strain JSC-3b. Sci. Rep. 5, 16121. doi:10.1038/srep16121
Su, P., Tan, X., Li, C., Zhang, D., Cheng, J. e., Zhang, S., et al. (2017). Photosynthetic Bacterium Rhodopseudomonas palustris GJ-22 Induces Systemic Resistance Against Viruses. Microb. Biotechnol. 10 (3), 612–624. doi:10.1111/1751-7915.12704
Swingley, W. D., Blankenship, R. E., and Raymond, J. (2009). “Evolutionary Relationships Among Purple Photosynthetic Bacteria and the Origin of Proteobacterial Photosynthetic Systems,” in The Purple Phototrophic Bacteria. Editors C. N. Hunter, F. Daldal, M. C. Thurnauer, and J. T. Beatty (Dordrecht: Springer Netherlands), 17–29. doi:10.1007/978-1-4020-8815-5_2
Tabita, F. R. (1999). Microbial Ribulose 1,5-bisphosphate Carboxylase/Oxygenase: A Different Perspective. Photosyn. Res. 60 (1), 1–28. doi:10.1023/A:1006211417981
Tabita, F. R., Satagopan, S., Hanson, T. E., Kreel, N. E., and Scott, S. S. (2008). Distinct Form I, II, III, and IV Rubisco Proteins from the Three Kingdoms of Life Provide Clues about Rubisco Evolution and Structure/function Relationships. J. Exp. Bot. 59 (7), 1515–1524. doi:10.1093/jxb/erm361
Takaichi, S. (2004). “Carotenoids and Carotenogenesis in Anoxygenic Photosynthetic Bacteria,” in The Photochemistry of Carotenoids. Editors H. A. Frank, A. J. Young, G. Britton, and R. J. Cogdell (Dordrecht: Springer), 39–69.
Tian, X., Liao, Q., Zhu, X., Wang, Y., Zhang, P., Li, J., et al. (2010). Characteristics of a Biofilm Photobioreactor as Applied to Photo-Hydrogen Production. Bioresour. Technol. 101 (3), 977–983. doi:10.1016/j.biortech.2009.09.007
Troost, K., Loeschcke, A., Hilgers, F., Özgür, A. Y., Weber, T. M., Santiago-Schübel, B., et al. (2019). Engineered Rhodobacter capsulatus as a Phototrophic Platform Organism for the Synthesis of Plant Sesquiterpenoids. Front. Microbiol. 10, 1998. doi:10.3389/fmicb.2019.01998
Venkidusamy, K., and Megharaj, M. (2016). A Novel Electrophototrophic Bacterium Rhodopseudomonas palustris Strain RP2, Exhibits Hydrocarbonoclastic Potential in Anaerobic Environments. Front. Microbiol. 7, 1071. doi:10.3389/fmicb.2016.01071
Vincenzini, M., Marchini, A., Ena, A., and De Philippis, R. (1997). H and Poly-β-Hydroxybutyrate, Two Alternative Chemicals from Purple Non Sulfur Bacteria. Biotechnol. Lett. 19 (8), 759–762. doi:10.1023/A:1018336209252
Vitousek, P. M., Aber, J. D., Howarth, R. W., Likens, G. E., Matson, P. A., Schindler, D. W., et al. (1997). Human Alteration of the Global Nitrogen Cycle: Sources and Consequences. Ecol. Appl. 7 (3), 737–750. doi:10.1890/1051-0761(1997)007[0737:haotgn]2.0.co;2
Wang, B., Jiang, Z., Yu, J. C., Wang, J., and Wong, P. K. (2019). Enhanced CO2 Reduction and Valuable C2+ Chemical Production by a CdS-Photosynthetic Hybrid System. Nanoscale 11 (19), 9296–9301. doi:10.1039/c9nr02896j
Welander, P. V., Hunter, R. C., Zhang, L., Sessions, A. L., Summons, R. E., and Newman, D. K. (2009). Hopanoids Play a Role in Membrane Integrity and pH Homeostasis in Rhodopseudomonas palustris TIE-1. J. Bacteriol. 191 (19), 6145–6156. doi:10.1128/jb.00460-09
Woude, B. J., Boer, M., Put, N. M. J., Geld, F. M., Prins, R. A., and Gottschal, J. C. (1994). Anaerobic Degradation of Halogenated Benzoic Acids by Photoheterotrophic Bacteria. FEMS Microbiol. Lett. 119 (1-2), 199–207. doi:10.1111/j.1574-6968.1994.tb06889.x
Wu, P., Chen, Z., Zhang, Y., Wang, Y., Zhu, F., Cao, B., et al. (2019). Rhodopseudomonas palustris Wastewater Treatment: Cyhalofop-Butyl Removal, Biochemicals Production and Mathematical Model Establishment. Bioresour. Technol. 282, 390–397. doi:10.1016/j.biortech.2018.11.087
Wu, S. C., Liou, S. Z., and Lee, C. M. (2012). Correlation between Bio-Hydrogen Production and Polyhydroxybutyrate (PHB) Synthesis by Rhodopseudomonas palustris WP3-5. Bioresour. Technol. 113, 44–50. doi:10.1016/j.biortech.2012.01.090
Xia, S., Zhang, L., Davletshin, A., Li, Z., You, J., and Tan, S. (2020). Application of Polysaccharide Biopolymer in Petroleum Recovery. Polymers 12 (9), 1860. doi:10.3390/polym12091860
Xiao, L., Zhao, Z., Ma, Z., Chen, J., and Song, Z. (2019). Immobilization of Rhodopseudomonas Palustris P1 on Glass Pumice to Improve the Removal of NH4+ ‐N and NO2− ‐N from Aquaculture Pond Water. Biotechnol. Appl. Biochem. 67 (3), 323–329. doi:10.1002/bab.1863
Xing, D., Zuo, Y., Cheng, S., Regan, J. M., and Logan, B. E. (2008). Electricity Generation by Rhodopseudomonas palustris DX-1. Environ. Sci. Technol. 42 (11), 4146–4151. doi:10.1021/es800312v
Xu, Q. Q., Yan, H., Liu, X. L., Lv, L., Yin, C. H., and Wang, P. (2014). Growth Performance and Meat Quality of Broiler Chickens Supplemented with Rhodopseudomonas palustris in Drinking Water. Br. Poult. Sci. 55 (3), 360–366. doi:10.1080/00071668.2014.903326
Xu, W., Chai, C., Shao, L., Yao, J., and Wang, Y. (2016). Metabolic Engineering of Rhodopseudomonas palustris for Squalene Production. J. Ind. Microbiol. Biotechnol. 43 (5), 719–725. doi:10.1007/s10295-016-1745-7
Yang, C.-F., and Lee, C.-M. (2011). Enhancement of Photohydrogen Production Using phbC Deficient Mutant Rhodopseudomonas palustris Strain M23. Bioresour. Technol. 102 (9), 5418–5424. doi:10.1016/j.biortech.2010.09.078
Yang, Z.-Y., Moure, V. R., Dean, D. R., and Seefeldt, L. C. (2012). Carbon Dioxide Reduction to Methane and Coupling with Acetylene to Form Propylene Catalyzed by Remodeled Nitrogenase. Proc. Natl. Acad. Sci. U.S.A. 109, 19644–19648. doi:10.1073/pnas.1213159109
Zhan, P. R., and Liu, W. (2013). Immobilization and Ammonia Removal of Photosynthetic Bacteria. Adv. Mat. Res. 610–613, 311–314. doi:10.4028/www.scientific.net/AMR.610-613.311
Zhang, P., Sun, F., Cheng, X., Li, X., Mu, H., Wang, S., et al. (2019). Preparation and Biological Activities of an Extracellular Polysaccharide from Rhodopseudomonas palustris. Int. J. Biol. Macromol. 131, 933–940. doi:10.1016/j.ijbiomac.2019.03.139
Zhang, S., Luo, X., Cheng, J. e., Peng, J., Zhang, D., and Liu, Y. (2014). Genome Sequence of Pyrethroid-Degrading Bacterium Rhodopseudomonas palustris Strain JSC-3b. Genome Announc. 2 (1), e01228–13. doi:10.1128/genomeA.01228-13
Zhao, C., Zhang, Y., Chan, Z., Chen, S., and Yang, S. (2015). Insights into Arsenic Multi-Operons Expression and Resistance Mechanisms in Rhodopseudomonas palustris CGA009. Front. Microbiol. 6, 986. doi:10.3389/fmicb.2015.00986
Zhao, J., Wei, Q., Wang, S., and Ren, X. (2021). Progress of Ship Exhaust Gas Control Technology. Sci. Total Environ. 799, 149437. doi:10.1016/j.scitotenv.2021.149437
Zhao, L., Zhao, C., Han, D., Yang, S., Chen, S., and Yu, C.-P. (2011). Anaerobic Utilization of Phenanthrene by Rhodopseudomonas palustris. Biotechnol. Lett. 33 (11), 2135–2140. doi:10.1007/s10529-011-0681-x
Zheng, Y., Harris, D. F., Yu, Z., Fu, Y., Poudel, S., Ledbetter, R. N., et al. (2018). A Pathway for Biological Methane Production Using Bacterial Iron-Only Nitrogenase. Nat. Microbiol. 3 (3), 281–286. doi:10.1038/s41564-017-0091-5
Zheng, Y., and Harwood, C. S. (2019). Influence of Energy and Electron Availability on In Vivo Methane and Hydrogen Production by a Variant Molybdenum Nitrogenase. Appl. Environ. Microbiol. 85 (9), e02671–02618. doi:10.1128/AEM.02671-18
Keywords: Rhodopseudomonas palustris, biopolymer, biofuel, wastewater treatment, microbial cell factory, photoautotrophic
Citation: Li M, Ning P, Sun Y, Luo J and Yang J (2022) Characteristics and Application of Rhodopseudomonas palustris as a Microbial Cell Factory. Front. Bioeng. Biotechnol. 10:897003. doi: 10.3389/fbioe.2022.897003
Received: 15 March 2022; Accepted: 27 April 2022;
Published: 12 May 2022.
Edited by:
Ka Yu Cheng, CSIRO Land and Water, AustraliaReviewed by:
Sanjay Kumar Singh Patel, Konkuk University, South KoreaJung Rae Kim, Pusan National University, South Korea
Copyright © 2022 Li, Ning, Sun, Luo and Yang. This is an open-access article distributed under the terms of the Creative Commons Attribution License (CC BY). The use, distribution or reproduction in other forums is permitted, provided the original author(s) and the copyright owner(s) are credited and that the original publication in this journal is cited, in accordance with accepted academic practice. No use, distribution or reproduction is permitted which does not comply with these terms.
*Correspondence: Jie Luo, qdluojie@126.com; Jianming Yang, yjming888@126.com
†These authors have contributed equally to this work and share first authorship