An investigation of tendon strains in jersey finger injury load cases using a finite element neuromuscular human body model
- 1Institute for Modelling and Simulation of Biomechanical Systems, University of Stuttgart, Stuttgart, Germany
- 2Stuttgart Center for Simulation Science, University of Stuttgart, Stuttgart, Germany
Introduction: A common hand injury in American football, rugby and basketball is the so-called jersey finger injury (JFI), in which an eccentric overextension of the distal interphalangeal joint leads to an avulsion of the connected musculus flexor digitorum profundus (FDP) tendon. In the field of automotive safety assessment, finite element (FE) neuromuscular human body models (NHBMs) have been validated and are employed to evaluate different injury types related to car crash scenarios. The goal of this study is to show, how such a model can be modified to assess JFIs by adapting the hand of an FE-NHBM for the computational analysis of tendon strains during a generalized JFI load case.
Methods: A jersey finger injury criterion (JFIC) covering the injury mechanisms of tendon straining and avulsion was defined based on biomechanical experiments found in the literature. The hand of the Total Human Model for Safety (THUMS) version 3.0 was combined with the musculature of THUMS version 5.03 to create a model with appropriate finger mobility. Muscle routing paths of FDP and musculus flexor digitorum superficialis (FDS) as well as tendon material parameters were optimized using literature data. A simplified JFI load case was simulated as the gripping of a cylindrical rod with finger flexor activation levels between 0% and 100%, which was then retracted with the velocity of a sprinting college football player to forcefully open the closed hand.
Results: The optimization of the muscle routing node positions and tendon material parameters yielded good results with minimum normalized mean absolute error values of 0.79% and 7.16% respectively. Tendon avulsion injuries were detected in the middle and little finger for muscle activation levels of 80% and above, while no tendon or muscle strain injuries of any kind occurred.
Discussion: The presented work outlines the steps necessary to adapt the hand model of a FE-NHBM for the assessment of JFIs using a newly defined injury criterion called the JFIC. The injury assessment results are in good agreement with documented JFI symptoms. At the same time, the need to rethink commonly asserted paradigms concerning the choice of muscle material parameters is highlighted.
1 Introduction
Many sporting activities put great stress on the upper appendages with around 25% of sports related injuries involving the hand or the wrist (Amadio, 1990; Rettig, 2003). A common hand injury in American football, rugby and basketball is the so-called jersey finger injury (JFI). This type of injury is caused by an eccentric overextension of the distal interphalangeal (DIP) joint, as can occur during the forceful release of one player’s grip on another player’s jersey or a finger getting caught on the rim of a basketball hoop, and leads to an avulsion of the connected musculus flexor digitorum profundus (FDP) tendon (Murphy and Mass, 2005; Gaston and Loeffler, 2015; Avery et al., 2016). This injury has been studied extensively in clinical studies, for example, in the work of Tempelaere et al. (Tempelaere et al., 2017), while computational investigations have thus far been focused on the modelling of general hand models (Joaquin et al., 2011), singular digits (Wu et al., 2008; Vigouroux et al., 2009; Fok and Chou, 2010; Wu et al., 2010) or the finger pulley system (Roloff et al., 2006; Vigouroux et al., 2008). In the field of automotive safety assessment, finite element (FE) neuromuscular human body models (NHBMs) created for the use with the FE-solver LS-DYNA (Ansys, Canonsburg, PA, United States) such as the Global Human Body Models Consortium (GHBMC) (Devane et al., 2019) or the Total Human Model for Safety (THUMS) (Kato et al., 2017; Kato et al., 2018) have been validated and are mainly employed to evaluate a host of different injury types related to car crash scenarios (GHBMC, 2016; Toyota Motor Corporation, and Toyota Central R&D Labs. Inc, 2021). Examples of these validation efforts are given by Kato et al. (Kato et al., 2018) where the THUMS model of version 6.0 was validated against several sets of test data derived from post-mortem human subjects (Cavanaugh et al., 1986; Cesari and Bouquet, 1990; Bolte et al., 2003; Foster et al., 2006; Rupp et al., 2008; Kroell et al., 2009; Shaw et al., 2009; Viano, 2009). The goal of this study is to show how a FE-NHBM can be modified to assess injuries not only found in car crashes, specific to the automotive domain, by adapting the hand of the THUMS AM50 occupant model of academic version 3.0 (Iwamoto et al., 2007) for the computational analysis of tendon strains during generalized JFI load cases. To this end, a Jersey Finger Injury Criterion (JFIC) covering the injury mechanisms of tendon straining and avulsion is first defined based on biomechanical experiments found in the literature. Next, FE-NHBM choice and necessary modification steps, including the routing and parameter tuning of newly introduced Hill-type muscles, will be outlined. Finally, a simulation study is performed to ensure both a sensible model behavior, and to tackle the question of how varying muscle activation levels and resulting maximum muscle forces impact the risk of sustaining a JFI in a representative injury scenario.
2 Materials and methods
2.1 Definition of a jersey finger injury criterion
JFI scenarios are characterized by the forced opening of an otherwise closed grip resulting in two distinct injury mechanisms. The main injury mechanism of the JFI is the avulsion of the FDP tendon caused by a hyperextension of the DIP joint (Gaston and Loeffler, 2015; Avery et al., 2016). Measurements of the forces necessary to induce such an injury are described in both the works of Holden and Northmore-Ball (Holden and Northmore-Ball, 1975) as well as those of Manske and Lesker (Manske and Lesker, 1978). To err on the side of caution, the lowest reported avulsion load of 10.8 kg (Manske and Lesker, 1978) was converted to Newtons and set as the resulting avulsion force threshold of 105.91 N. While not classically associated with the JFI, it is well known that eccentric muscle contraction can cause considerable damage to the affected tissue, with injuries ranging from minor strains to the complete rupture of the muscle-tendon-unit (MTU) (Noonan and Garrett, 1999; Maffulli, 2005). The eccentric lengthening of the finger flexor muscle groups was thus identified as a secondary injury mechanism to be represented in the JFIC. Studies of tendon material properties have shown that the severity of a sustained tendon strain injury can be linked to the deformation stages of the tendon’s stress-strain curve (Maffulli, 2005; Wang, 2006). Consequently, three distinct tendon strain injury thresholds were defined, with the minor injury threshold set at the start of the strain hardening region, the major injury threshold at the start of the necking region, and rupture threshold at the point of material failure. Tendon strains appropriate for the deformation regions of positional tendons as defined by Kaya et al. (Kaya et al., 2019) were derived from the literature (Maganaris et al., 2004; Wang, 2006; Stauber et al., 2019) and there thus used to define a secondary injury criterion called the Tendon Strain Injury Criterion (TSIC). A summary of the avulsion injury and TSIC threshold values, which together form the JFIC, is given in Table 1. The occurrence and severity of muscle strain injury was assessed using the Muscle Strain Injury Criterion (MSIC) analogously defined by Nölle et al. (Nölle et al., 2022b). An injury assessment using the JFIC and MSIC was performed for the FDP and the musculus flexor digitorum superficialis (FDS). All abbreviations used in the paper are listed in Supplementary Table S1.
2.2 Model selection and modification
The main selection criterion for the choice of an FE-NHBM was deemed to be the maximally achievable finger mobility, as simulating a gripping motion prior to the forced eccentric opening of the hand was considered to be essential for the reconstruction of JFI load cases. After evaluating the hand models of the THUMS AM50 occupant models version 3.0 (Iwamoto et al., 2007), version 4.1 (Shigeta et al., 2009), version 5.03 (Iwamoto and Nakahira, 2015) and version 6.1 (Kato et al., 2018), we arrived at the conclusion that no single model would be able to deliver a sufficient range of finger motion in their default states, as the mesh geometry and hand structures of all models limited the finger mobility to flexion movements only. Consequently, we decided to combine the properties of multiple models into one. THUMS version 3.0 was chosen as the base model because of the detailed modelling of the finger bone structure, while the muscle elements necessary for the generation of a flexion movement as well as the overall kinematic modelling approach were adopted from THUMS version 5.03. Both models were acquired under academic license from DYNAmore Gesellschaft für FEM Ingenieurdienstleistungen mbH, Stuttgart, Germany. All model modifications described in the following were performed on the right hand of the THUMS version 3.0. As a first modification step, the interphalangeal ligaments were removed to ensure appropriate finger movement capabilities. Second, the phalanges, metacarpals and carpals of the fingers were made rigid to allow for the insertion of kinematic revolute joints between the phalanges and metacarpals. To increase numerical stability, joint stiffness values taken from THUMS version 5.03 and joint range of motion limits described by Hirt et al. (Hirt, 2016) were implemented in a third step. Relevant flexor and extensor muscles of the hand were added based on the muscle modelling present in THUMS version 5.03 (Iwamoto and Nakahira, 2015) (Supplementary Table S2). Originally, these muscles are modelled using truss elements with the default LS-DYNA Hill-type muscle material *MAT_MUSCLE (LSTC, 2016b), while tendons are represented by seatbelt elements. To allow for a better assessment of tendon strain injury severity, *MAT_MUSCLE was replaced with a more biophysiological Hill-type muscle material developed by (Günther et al., 2007) and Haeufle et al. (Haeufle et al., 2014), which is available in LS-DYNA as a user-defined material named the extended Hill-type material (EHTM). The EHTM was initially implemented in LS-DYNA by Kleinbach et al. (Kleinbach et al., 2017) and updated to its most current version by Kleinbach et al. (Kleinbach, 2019), Martynenko et al. (Martynenko et al., 2023) and Wochner et al. (Wochner et al., 2022). Compared to *MAT_MUSCLE, the EHTM material has the additional benefit of including the tendon as a distinct element called the serial elastic element (SEE) (Haeufle et al., 2014), eliminating the need for combining muscle and seatbelt elements to form the MTU. However, one limitation of this modelling approach is that MTUs with a single muscle body and multiple connected tendons, as is the case for many muscles in the hand and lower arm, cannot be modelled as such but instead need be split up into discrete truss elements for each separate tendon path. For example, the FDP, a muscle with tendons reaching into fingers 2 to 5, was modelled as 4 parallel truss elements. A comparison of the original THUMS version 3.0 hand model and the modified version presented in this work is given in Figure 1.
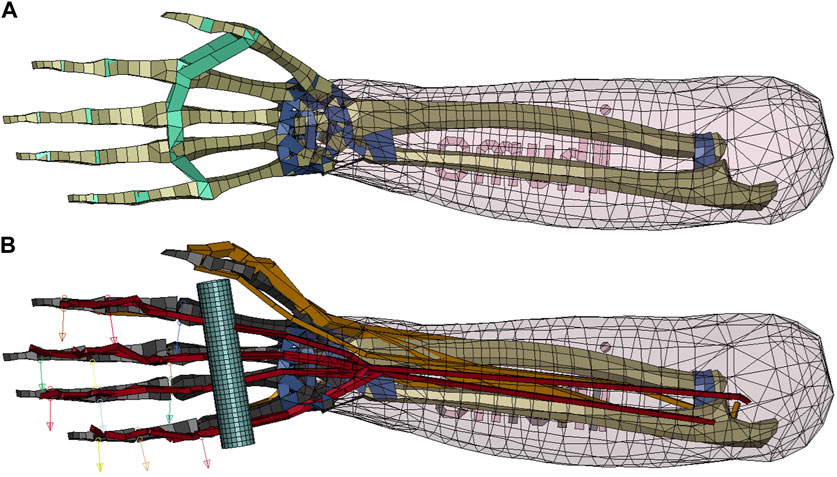
FIGURE 1. Comparison of the original and the modified THUMS version 3.0 hand models. (A) Original THUMS version 3.0 hand model, removed ligaments marked in green, kept ligaments marked in dark blue; (B) Modified THUMS version 3.0 hand model, rigid bones marked in black, manually routed muscles marked in orange, muscles with optimized routing marked in red, rod marked in light blue and revolute joint axes in the DIP, PIP and MCP joints marked with arrows.
2.3 Muscle routing and validation through moment arm optimization
The finger extensor muscle groups as well as the musculus flexor pollicis longus were manually routed using the via-point method (Delp et al., 1990; Hoy et al., 1990; Günther and Ruder, 2003) along anatomical landmarks, while special attention was given to the most injury-relevant flexors, FDP and FDS, whose routing paths across the DIP, proximal interphalangeal (PIP) and metacarpophalangeal (MCP) joint were additionally validated by adjusting them to fit moment arm curves compiled by Boots et al. (Boots et al., 2020) to ensure physiologically valid grip strength production and finger flexion mobility. Moment arms of FDP and FDS for the index finger were originally measured by Fowler et al. (Fowler et al., 2001), while data on the middle to ring finger were taken from Koh et al. (Koh et al., 2006). The nodal positions defining the muscle routing paths were optimized using the least-squares optimization functionality “lsqcurvefit” provided in the MATLAB R2022a Optimization Toolbox (Mathworks, Natick, MA, United States). The boundary conditions for the optimization were defined such that the routing nodes were placed on a plane which is normal to the revolute joint axes and intersects with the joint center. This condition was implemented to ensure that the force generated by the muscle elements could fully contribute to the resulting joint torque instead of partially dissipating by acting on a degree of freedom locked by the revolute joint. Additionally, nodes needed be placed on the medial palm side of the hand to avoid an overlap of the muscle trusses and the finger bones. The quality of the moment arm curves resulting from the optimized node placement was evaluated using the mean absolute error (Willmott and Matsuura, 2005) (Eq. (1)) normalized to the mean of the measured moment arm data (Eq. (2)).
where
A total number of 20 moment arm curves, with the FDP spanning 4 digits over 3 joints and the FDS spanning 4 digits over 2 joints, were derived with the help of the routing path optimization. All optimized moment arm curves are shown in Supplementary Figures S1, S2. A detailed description of the moment arm optimization methodology is provided in Supplementary Chapter S3.
2.4 Tendon material parameter optimization
An assessment of JFI severity can only be reliably performed if the material parameters of the tendons are set within sensible bounds. On the one hand, overly compliant tendons would be a poor fit for the deformation characteristics of positional tendons and would limit finger movement capabilities, as the contractions of the muscles located in the lower arm could not be mechanically transferred to the fingers through the tendons but would instead be compensated for by an elongation of the tendon itself (Maffulli, 2005). On the other hand, overly stiff tendons would lead to an overestimation of MTU forces, which, in turn, would trigger the defined avulsion injury threshold (Table 1) erroneously. To avoid these issues, experimental data on the stress-strain characteristics of unembalmed human tendons collected by Benedict et al. (Benedict et al., 1968) were used for the manual tuning of the material parameters defining the tendon properties of the EHTM. These parameters are the force at the non-linear linear transition point
where
To account for the fact that the fingers of the THUMS version 3.0 model are straightened in its initial position and deviate from a relaxed hand position (Mount et al., 2003) in which the condition outlined in Eq. 3 could be assumed, we introduced an additional scaling factor
where
Additionally, the maximum isometric force of the muscles was scaled in cases where complex geometries of singular muscles had to be recreated with multiple parallel elements (Eq. (5)).
where
A complete list of all EHTM material parameters used in the presented model can be found in Supplementary Tables S2, S3.
2.5 Simulated load case
A simplified JFI load case was defined by substituting the opponent’s jersey with a cylindrical rigid rod of 100 mm length and a diameter of 20 mm placed in the palm of the THUMS version 3.0 hand (Figure 1). Each JFI simulation included two consecutive stages (Supplementary Figure S3). In the first stage, covering the time interval of 0–100 ms, FDP and FDS were activated and given time to reach their flexion state in order to grab the rigid rod. The interaction between the hand and the rod was modelled using the an automatic surface-to-surface contact with static and dynamic friction values of 0.4 and 0.3 respectively (LSTC, 2016a). Additionally, a tied surface-to-surface contact with the same friction values (LSTC, 2016a) was activated after 80 ms to ensure that the rection forces of the surface-to-surface contact would not push the fingers apart to loosen the grip before the retraction of the rod. Once the rod gripping movement was completed, the ulna and radius were fully constrained in space to eliminate noise factors such as elbow extension or shoulder rotation during the rod pulling stage and to make sure that the entire stress caused by the JFI load case is placed on the finger flexors. In the second stage from
Eight JFI simulations at FDP and FDS muscle activation levels 0%, 20%, 40%, 60%, 70%, 80%, 90% and 100% were performed (simulations 1 to 8 in Table 3). An additional model check simulation (simulation 9 in Table 3) was done at 100%, in which the rod was not retracted, to ensure that the maximal muscle contraction alone did not cause injury by itself, for a total of 9 simulations. Muscle activation levels for all other muscles were kept at the minimum activation level defined by (Günther, 1997) to reflect their relaxed state. The effectiveness of the muscle material parameter dependent transfer between muscle activation
where
All simulations were performed with a user-compiled double precision (DP) symmetric multiprocessing (SMP) version of LS-DYNA R9.3.1 (Ansys, Canonsburg, PA, United States) including EHTM version 3.2.04 (Nölle et al., 2022a). The simulations were run on a high-performance workstation equipped with an AMD Ryzen Threadripper 3990X 64-core processor (AMD, Santa Clara, CA, United States) using 32 SMP threads. The timestep size was automatically calculated with the timestep size for mass scaled solutions set to −6.000e-07 s. Simulation runtimes ranged between 4 h 31 m 25 s for simulation 5 and 7 h 12 m 58 s ± 3 m 16 s for all other simulations.
3 Results
3.1 Moment arm curve fit quality
The optimization of the muscle routing node positions for FDP and FDS using moment arm data derived from the literature (Fowler et al., 2001; Koh et al., 2006; Boots et al., 2020) yielded good results with all moment arm curves showing
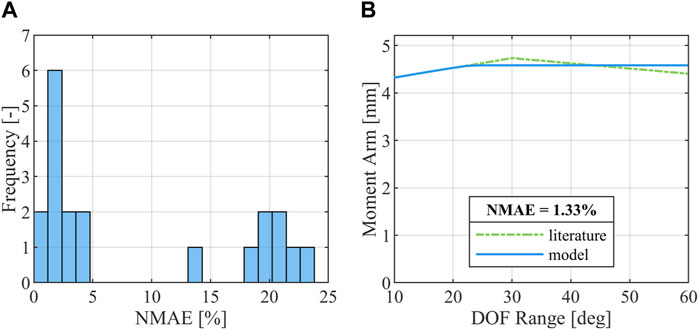
FIGURE 2. Results of the moment arm optimization. (A) Distribution of
3.2 Optimized tendon material parameters
The manual tuning of EHTM tendon material properties to curves reported by Benedict et al. (Benedict et al., 1968) resulted in a greatly improved curve fit for tendon strains of up to 3%, with the tuned EHTM tendon material parameters scoring
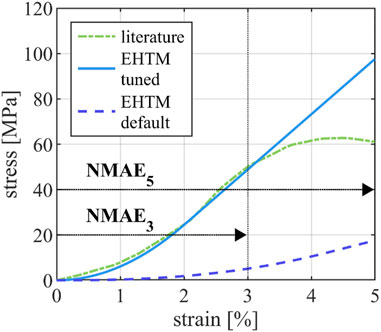
FIGURE 3. Comparison of the tendon stress-strain-curve reported in the literature (Benedict et al., 1968), the stress-strain curve of the EHTM tendon achieved through manual parameter fit and the stress-strain curve of the EHTM tendon using the default material parameters (Nölle et al., 2022a). Curve sections used for the calculation of
3.3 Tendon and muscle strain injury assessment
The analysis of the MTU forces
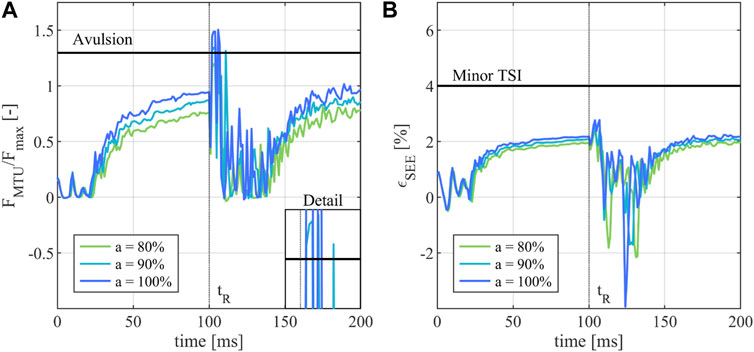
FIGURE 4. Injury assessment of the of the FDP in the middle finger during a JFI loading scenario. (A) Activity dependent normalized muscle force
4 Discussion
The simulation-based reconstruction of sports injuries such as a JFI and the definition of criteria to assess such injuries is a challenging task, as numerous methodological approaches (Krosshaug et al., 2005) such as motion analysis, cadaver studies or athlete interviews yield insufficiently detailed information necessary for the one-to-one reconstruction of an injury. Additionally, only few instances of sports injuries during biomechanical experiments are described in the literature (Zernicke et al., 1977; Barone et al., 1999; Heiderscheit et al., 2005; Schache et al., 2009) with, to the authors’ knowledge, no documented case of an in-vivo JFI occurring in an experimental setup. Likewise, tendon strains and avulsion are not currently tracked in publicly available injury databases (Compton, 2002), further limiting the pool of load cases useable to define an injury criterion by conventional statistical means. The JFIC is thus based on the biomechanical properties of the human tendon instead of the statistical derivation of a risk index from documented injury cases. The tendon-strain-based injury thresholds of the TSIC were defined with the aim to represent the properties of positional tendons (Kaya et al., 2019), which are comparatively stiff and serve to transfer forces from the muscle to the bone with minimal force dissipation. As functional requirements influence the material properties of the tendon (Quigley et al., 2018), TSIC threshold values may need to be adjusted if applied to tendons which are known to be subjected to larger strain ranges such as the Achilles tendon. Similarly, the force-based JFIC avulsion threshold is only valid when applied to human finger flexor MTUs as the underlying experiments by Manske and Lesker (Manske and Lesker, 1978) were limited to the FDP tendon insertion only. Given that the JFIC was defined for the use with Hill-type muscle models such as the EHTM, tendon properties such as fatigue (Ker et al., 2000) or tendon creep (Maganaris, 2002; Maganaris et al., 2004) were not included in the definition of the injury criterion, as the used muscle model is not able to reflect these effects properly. Numerical instabilities common to Hill-type muscles as described by Yeo et al. (Yeo et al., 2023) were mitigated in this study by not routing muscles in series and by keeping muscle co-contraction levels to a minimum as the finger extensor muscles were only activated with a minimum physiological activity level (Günther, 1997). The modified THUMS version 3.0 hand model itself is well suited for the assessment of MTU forces and strains as is necessary for the proposed JFI assessment. Other anatomical structures such as the finger pulley system, the joint capsules or the soft tissues surrounding the phalanges are however not represented in the current model and offer room for further improvement of the model quality in future studies. Additionally, the previous validation efforts of both THUMS version 3.0 (Iwamoto et al., 2007) and 5.03 (Iwamoto and Nakahira, 2015) did not include specific validation cases for the lower arm or hand regions. The validity of the FE-NHBMs used in this study is thus transitively assumed, as the models performed well in the described whole-body validation cases, whose outcomes partly depend on the correct behavior of the upper extremities. The quality of the moment arm curve fit was determined using the
4.1 Conclusion
The presented work outlines the steps necessary to successfully adapt the hand model of a FE-NHBMs for the assessment of JFIs using a newly defined injury criterion called the JFIC based on biomechanical data found in the literature, which, together with the previously established MSIC (Nölle et al., 2022b), forms a next step in creating a wholistic injury criterion for strain injuries of the MTU. The injury assessment results achieved with the JFIC are in good agreement with JFI symptoms as described in the medical field, showing a clear dependency between finger flexor activation, gripping force and JFI severity. At the same time, the need to rethink commonly asserted paradigms concerning the choice of muscle material parameters, in which only few parameters are assumed to be muscle specific, is highlighted, as material properties across all muscle structures need to be closely matched to the physiological demands of the muscle to ensure a reliable MTU injury assessment. Additionally, modelling choices and load case conditions should be representative of the real-life injury scenario, as to not subject the MTU to unrealistic loads. This work emphasizes the benefit of using neuromuscular human body models together with literature data and experiments to improve our understanding of how mechanical loads may cause tissue damage and thus, how to predict potential sources of injury. The authors hope to inspire further scientific cooperation between all fields of injury biomechanics with this interdisciplinary work of applying models commonly used in the automotive sector in a sports science context.
Data availability statement
The original contributions presented in the study are included in the article/Supplementary Material, further inquiries can be directed to the corresponding author.
Author contributions
LN: Conceptualization, Methodology, Software, Visualization, Writing–original draft. EA: Data curation, Software, Writing–review and editing. OM: Conceptualization, Funding acquisition, Supervision, Writing–review and editing. SS: Conceptualization, Funding acquisition, Supervision, Writing–review and editing.
Funding
The author(s) declare financial support was received for the research, authorship, and/or publication of this article. This work was supported by the Deutsche Forschungsgemeinschaft (DFG, German Research Foundation) under Germany’s Excellence Strategy—EXC 2075—390740016 (SimTech) and by the Federal Ministry for Economic Affairs and Climate Action of Germany (Bundesministerium für Wirtschaft und Klimaschutz) through the project “Artificial Intelligence for Real-Time Injury Prediction (ATTENTION),” grant number 19A21027D.
Conflict of interest
The authors declare that the research was conducted in the absence of any commercial or financial relationships that could be construed as a potential conflict of interest.
Publisher’s note
All claims expressed in this article are solely those of the authors and do not necessarily represent those of their affiliated organizations, or those of the publisher, the editors and the reviewers. Any product that may be evaluated in this article, or claim that may be made by its manufacturer, is not guaranteed or endorsed by the publisher.
Supplementary material
The Supplementary Material for this article can be found online at: https://www.frontiersin.org/articles/10.3389/fbioe.2023.1293705/full#supplementary-material
References
Amadio, P. C. (1990). Epidemiology of hand and wrist injuries in sports. Hand Clin. 6, 379–381. doi:10.1016/S0749-0712(21)00880-5
Avery, D. M., Rodner, C. M., and Edgar, C. M. (2016). Sports-related wrist and hand injuries: a review. J. Orthop. Surg. Res. 11, 99. doi:10.1186/s13018-016-0432-8
Barone, M., Senner, V., and Schaff, P. (1999). “ACL Injury Mechanism in Alpine Skiing: Analysis of an Accidental ACL Rupture” in Skiing trauma and safety: 12th volume: contains papers presented at the 12th International Symposium on Skiing Trauma and Safety held in Whistler Blackcomb on May 4–10, 1997. Eds R. J. Johnson, and R. J. Johnson (British Columbia, Canada: West Conshohocken: ASTM) 63-63-19.
Benedict, J. V., Walker, L. B., and Harris, E. H. (1968). Stress-strain characteristics and tensile strength of unembalmed human tendon. J. Biomech. 1, 53–63. doi:10.1016/0021-9290(68)90038-9
Bolte, J. H., Hines, M. H., Herriott, R. G., McFadden, J. D., and Donnelly, B. R. (2003). Shoulder impact response and injury due to lateral and oblique loading. Stapp Car Crash J. 47, 35–53. doi:10.4271/2003-22-0003
Boots, M. T., Hardesty, R., Sobinov, A., Gritsenko, V., Collinger, J. L., Fisher, L. E., et al. (2020). Functional and structural moment arm validation for musculoskeletal models: a study of the human forearm and hand. arXiv.
Brechue, W. F., Mayhew, J. L., and Piper, F. C. (2010). Characteristics of sprint performance in college football players. J. Strength Cond. Res. 24, 1169–1178. doi:10.1519/JSC.0b013e3181d68107
Bynum, D. K., and Gilbert, J. A. (1988). Avulsion of the flexor digitorum profundus: anatomic and biomechanical considerations. J. Hand Surg. Am. 13, 222–227. doi:10.1016/S0363-5023(88)80053-4
Cavanaugh, J. M., Nyquist, G. W., Goldberg, S. J., and King, A. I. (1986). “Lower abdominal tolerance and response,” in SAE technical paper series SAE International400 commonwealth drive (Warrendale, PA, United States: SAE International).
Cesari, D., and Bouquet, R. (1990). “Behaviour of human surrogates thorax under belt loading,” in SAE technical paper series SAE International400 commonwealth drive (Warrendale, PA, United States: SAE International).
Compton, C. P. (2002). “The use of public crash data in biomechanical research,” in Accidental injury: biomechanics and prevention. Editors A. M. Nahum, and J. W. Melvin (New York, NY: Springer New York), 40–71.
Delp, S. L., Loan, J. P., Hoy, M. G., Zajac, F. E., Topp, E. L., and Rosen, J. M. (1990). An interactive graphics-based model of the lower extremity to study orthopaedic surgical procedures. IEEE Trans. Biomed. Eng. 37, 757–767. doi:10.1109/10.102791
Devane, K., Johnson, D., and Gayzik, F. S. (2019). Validation of a simplified human body model in relaxed and braced conditions in low-speed frontal sled tests. Traffic Inj. Prev. 20, 832–837. doi:10.1080/15389588.2019.1655733
Fok, K. S., and Chou, S. M. (2010). Development of a finger biomechanical model and its considerations. J. Biomech. 43, 701–713. doi:10.1016/j.jbiomech.2009.10.020
Foster, C. D., Hardy, W. N., Yang, K. H., King, A. I., and Hashimoto, S. (2006). High-speed seatbelt pretensioner loading of the abdomen. Stapp Car Crash J. 50, 27–51. doi:10.4271/2006-22-0002
Fowler, N., Nicol, A., Condon, B., and Hadley, D. (2001). Method of determination of three dimensional index finger moment arms and tendon lines of action using high resolution MRI scans. J. Biomech. 34, 791–797. doi:10.1016/S0021-9290(01)00021-5
Gaston, R. G., and Loeffler, B. J. (2015). Sports-specific injuries of the hand and wrist. Clin. Sports Med. 34, 1–10. doi:10.1016/j.csm.2014.09.003
GHBMC (2016). User manual: M50 detailed occupant version 4.5 for LS-DYNA. Winston-Salem NC: Elemance, LLC.
Günther, M. (1997). Computersimulationen zur Synthetisierung des muskulär erzeugten menschlichen Gehens unter Verwendung eines biomechanischen Mehrkörpermodells. Tübingen, Germany: Eberhard-Karls-Universität zu Tübingen.
Günther, M., and Ruder, H. (2003). Synthesis of two-dimensional human walking: a test of the lambda-model. Biol. Cybern. 89, 89–106. doi:10.1007/s00422-003-0414-x
Günther, M., Schmitt, S., and Wank, V. (2007). High-frequency oscillations as a consequence of neglected serial damping in Hill-type muscle models. Biol. Cybern. 97, 63–79. doi:10.1007/s00422-007-0160-6
Haeufle, D. F. B., Günther, M., Bayer, A., and Schmitt, S. (2014). Hill-type muscle model with serial damping and eccentric force-velocity relation. J. Biomech. 47, 1531–1536. doi:10.1016/j.jbiomech.2014.02.009
Hammer, M., Günther, M., Haeufle, D. F. B., and Schmitt, S. (2019). Tailoring anatomical muscle paths: a sheath-like solution for muscle routing in musculoskeletal computer models. Math. Biosci. 311, 68–81. doi:10.1016/j.mbs.2019.02.004
Heiderscheit, B. C., Hoerth, D. M., Chumanov, E. S., Swanson, S. C., Thelen, B. J., and Thelen, D. G. (2005). Identifying the time of occurrence of a hamstring strain injury during treadmill running: a case study. Clin. Biomech. 20, 1072–1078. doi:10.1016/j.clinbiomech.2005.07.005
Holden, C., and Northmore-Ball, M. (1975). The strength of the profundus tendon insertion. Hand 7, 238–240. doi:10.1016/0072-968x(75)90059-5
Hoy, M. G., Zajac, F. E., and Gordon, M. E. (1990). A musculoskeletal model of the human lower extremity: the effect of muscle, tendon, and moment arm on the moment-angle relationship of musculotendon actuators at the hip, knee, and ankle. J. Biomech. 23, 157–169. doi:10.1016/0021-9290(90)90349-8
Iwamoto, M., and Nakahira, Y. (2015). “Development and validation of the total HUman model for safety (THUMS) version 5 containing multiple 1D muscles for estimating occupant motions with muscle activation during side impacts,” in SAE technical paper series (SAE International400 commonwealth drive (Warrendale, PA, United States: SAE International).
Iwamoto, M., Nakahira, Y., Tamura, A., Kimpara, H., Watanabe, I., and Miki, K. (2007). “Development of advanced human models in THUMS” in Proc. 6th European LS-DYNA users’ conference (Toyota Central R&D Labs, Inc), 47–56.
Joaquin, L., Perez-Gonzalez, A., and Vergara, M. (2011). “Towards a realistic and selfcontained biomechanical model of the hand”, in Theoretical biomechanics. Editor V. Klika (London, United Kingdom: intechOpen).
Kato, D., Nakahira, Y., Atsumi, N., and Iwamoto, M. (2018). “Development of human-body model THUMS Version 6 containing muscle controllers and application to injury analysis in frontal collision after brake deceleration” in Proceedings of the IRCOBI conference (Athens, Greece: IRCOBI), 12–14.
Kato, D., Nakahira, Y., and Iwamoto, M. (2017). “A study of muscle control with two feedback controls for posture and reaction force for more accurate prediction of occupant kinematics in low-speed frontal impacts” in Proceedings of the 25th International technical conference on the enhanced safety of vehicles (ESV) (Detroit, USA: Toyota Central R&D Labs. Inc).
Kaya, M., Karahan, N., and Yılmaz, B. (2019). “Tendon structure and classification”, in Tendons (London, United Kingdom: IntechOpen).
Ker, R. F., Dimery, N. J., and Alexander, R. M. (1986). The role of tendon elasticity in hopping in a wallaby (Macropus rufogriseus). J. Zool. 208, 417–428. doi:10.1111/j.1469-7998.1986.tb01904.x
Ker, R. F., Wang, X. T., and Pike, A. V. (2000). Fatigue quality of mammalian tendons. J. Exp. Biol. 203, 1317–1327. doi:10.1242/jeb.203.8.1317
Kleinbach, C., Martynenko, O., Promies, J., Haeufle, D. F. B., Fehr, J., and Schmitt, S. (2017). Implementation and validation of the extended Hill-type muscle model with robust routing capabilities in LS-DYNA for active human body models. Biomed. Eng. Online 16, 109. doi:10.1186/s12938-017-0399-7
Kleinbach, C. G. (2019). Simulation of occupant kinematics using active human body models. Dissertation. Düren: Universität Stuttgart.
Koh, S., Buford, W. L., Andersen, C. R., and Viegas, S. F. (2006). Intrinsic muscle contribution to the metacarpophalangeal joint flexion moment of the middle, ring, and small fingers. J. Hand Surg. Am. 31, 1111–1117. doi:10.1016/j.jhsa.2006.03.003
Kroell, C. K., Schneider, D. C., and Nahum, A. M. (2009). “Impact tolerance and response of the human thorax II,” in Electronic control module network and data link development and validation using hardware in the loop systems. Editors D. Williams, J. Allen, and R. Hukkeri (Warrendale, PA: SAE International).
Krosshaug, T., Andersen, T. E., Olsen, O.-E. O., Myklebust, G., and Bahr, R. (2005). Research approaches to describe the mechanisms of injuries in sport: limitations and possibilities. Br. J. Sports Med. 39, 330–339. doi:10.1136/bjsm.2005.018358
Leddy, J. P. (1985). Avulsions of the flexor digitorum profundus. Hand Clin. 1, 77–83. doi:10.1016/S0749-0712(21)01334-2
Leddy, J. P., and Packer, J. W. (1977). Avulsion of the profundus tendon insertion in athletes. J. Hand Surg. Am. 2, 66–69. doi:10.1016/S0363-5023(77)80012-9
LSTC (2016a). LS-DYNA® keyword USER'S manual volume I: LS-DYNA R9.0 08/29/16 (r:7883). Livermore, California Livermore Software Technology Corporation.
LSTC (2016b). LS-DYNA®KEYWORD USER'S MANUAL VOLUME II material models: LS-DYNA r9.008/31/16 (r:7893). Livermore, California Livermore Software Technology Corporation.
Lunn, P. G., and Lamb, D. W. (1984). Rugby finger"--avulsion of profundus of ring finger. J. Hand Surg. Br. 9, 69–71. doi:10.1016/0266-7681(84)90020-2
Maganaris, C. N. (2002). Tensile properties of in vivo human tendinous tissue. J. Biomech. 35, 1019–1027. doi:10.1016/S0021-9290(02)00047-7
Maganaris, C. N., Narici, M. V., Almekinders, L. C., and Maffulli, N. (2004). Biomechanics and pathophysiology of overuse tendon injuries: ideas on insertional tendinopathy. Sports Med. 34, 1005–1017. doi:10.2165/00007256-200434140-00005
Maganaris, C. N., Narici, M. V., and Maffulli, N. (2008). Biomechanics of the Achilles tendon. Disabil. Rehabil. 30, 1542–1547. doi:10.1080/09638280701785494
Manske, P. R., and Lesker, P. A. (1978). Avulsion of the ring finger flexor digitorum profundus tendon: an experimental study. Hand 10, 52–55. doi:10.1016/S0072-968X(78)80025-4
Martynenko, O. V., Kempter, F., Kleinbach, C., Nölle, L. V., Lerge, P., Schmitt, S., et al. (2023). Development and verification of a physiologically motivated internal controller for the open-source extended Hill-type muscle model in LS-DYNA. Biomech. Model Mechanobiol. 22, 2003–2032. doi:10.1007/s10237-023-01748-9
Morales-Orcajo, E., Becerro de Bengoa Vallejo, R., Losa Iglesias, M., and Bayod, J. (2016). Structural and material properties of human foot tendons. Clin. Biomech. (Bristol, Avon) 37, 1–6. doi:10.1016/j.clinbiomech.2016.05.014
Mount, F. E., Whitmore, M., and Stealey, S. L. (2003). Evaluation of neutral body posture on shuttle mission sts-57 (spacehab-1). Washington, DC NASA Headquarters.
Murphy, B. A., and Mass, D. P. (2005). Zone I flexor tendon injuries. Hand Clin. 21, 167–171. doi:10.1016/j.hcl.2004.12.004
Nölle, L. V., Lerge, P., Martynenko, O., Wochner, I., Kempter, F., Kleinbach, C., et al. (2022a). EHTM code and manual. Stuttgart, Germany University of Stuttgart. doi:10.18419/DARUS-1144
Nölle, L. V., Mishra, A., Martynenko, O. V., and Schmitt, S. (2022b). Evaluation of muscle strain injury severity in active human body models. J. Mech. Behav. Biomed. Mater 135, 105463. doi:10.1016/j.jmbbm.2022.105463
Noonan, T. J., and Garrett, W. E. (1999). Muscle strain injury: diagnosis and treatment. J. Am. Acad. Orthop. Surg. 7, 262–269. doi:10.5435/00124635-199907000-00006
Quigley, A. S., Bancelin, S., Deska-Gauthier, D., Légaré, F., Kreplak, L., and Veres, S. P. (2018). In tendons, differing physiological requirements lead to functionally distinct nanostructures. Sci. Rep. 8, 4409. doi:10.1038/s41598-018-22741-8
Rettig, A. C. (2003). Athletic injuries of the wrist and hand. Part I: traumatic injuries of the wrist. Am. J. Sports Med. 31, 1038–1048. doi:10.1177/03635465030310060801
Roloff, I., Schöffl, V. R., Vigouroux, L., and Quaine, F. (2006). Biomechanical model for the determination of the forces acting on the finger pulley system. J. Biomech. 39, 915–923. doi:10.1016/j.jbiomech.2005.01.028
Rupp, J. D., Miller, C. S., Reed, M. P., Madura, N. H., Klinich, K. D., and Schneider, L. W. (2008). Characterization of knee-thigh-hip response in frontal impacts using biomechanical testing and computational simulations. Stapp Car Crash J. 52, 421–474. doi:10.4271/2008-22-0017
Saraswat, P., Andersen, M. S., and Macwilliams, B. A. (2010). A musculoskeletal foot model for clinical gait analysis. J. Biomech. 43, 1645–1652. doi:10.1016/j.jbiomech.2010.03.005
Schache, A. G., Wrigley, T. V., Baker, R., and Pandy, M. G. (2009). Biomechanical response to hamstring muscle strain injury. Gait Posture 29, 332–338. doi:10.1016/j.gaitpost.2008.10.054
Scovil, C. Y., and Ronsky, J. L. (2006). Sensitivity of a Hill-based muscle model to perturbations in model parameters. J. Biomech. 39, 2055–2063. doi:10.1016/j.jbiomech.2005.06.005
Shaw, J. M., Herriott, R. G., McFadden, J. D., Donnelly, B. R., and Bolte, J. H. (2009). “Oblique and lateral impact response of the PMHS thorax,” in Electronic control module network and data link development and validation using hardware in the loop systems. Editors D. Williams, J. Allen, and R. Hukkeri (Warrendale, PA: SAE International).
Shigeta, K., Kitagawa, Y., and Yasuki, T. (2009). “Development of next generation human FE model capable of organ injury prediction,” in Proceedings of the 21st annual enhanced safety of vehicles. Washington, DC (National Highway Traffic Safety Administration), 15–18.
Stauber, T., Blache, U., and Snedeker, J. G. (2019). Tendon tissue microdamage and the limits of intrinsic repair. Matrix Biol. 85-86, 68–79. doi:10.1016/j.matbio.2019.07.008
Tempelaere, C., Brun, M., Doursounian, L., and Feron, J.-M. (2017). Traumatic avulsion of the flexor digitorum profundus tendon. Jersey finger, a 29 cases report. Hand Surg. Rehabil. 36, 368–372. doi:10.1016/j.hansur.2017.06.002
Toyota Motor Corporation, and Toyota Central R&D Labs. Inc (2021). Documentation total human model for safety (THUMS) AM50 occupant model. Version 6.1.
Viano, D. C. (2009). “Biomechanical responses and injuries in blunt lateral impact,” in Electronic control module network and data link development and validation using hardware in the loop systems. Editors D. Williams, J. Allen, and R. Hukkeri (Warrendale, PA: SAE International).
Vigouroux, L., Domalain, M., and Berton, E. (2009). Comparison of tendon tensions estimated from two biomechanical models of the thumb. J. Biomech. 42, 1772–1777. doi:10.1016/j.jbiomech.2009.03.052
Vigouroux, L., Quaine, F., Paclet, F., Colloud, F., and Moutet, F. (2008). Middle and ring fingers are more exposed to pulley rupture than index and little during sport-climbing: a biomechanical explanation. Clin. Biomech. (Bristol, Avon) 23, 562–570. doi:10.1016/j.clinbiomech.2007.12.009
Wang, J. H. C. (2006). Mechanobiology of tendon. J. Biomech. 39, 1563–1582. doi:10.1016/j.jbiomech.2005.05.011
Willmott, C. J., and Matsuura, K. (2005). Advantages of the mean absolute error (MAE) over the root mean square error (RMSE) in assessing average model performance. Clim. Res. 30, 79–82. doi:10.3354/cr030079
Wochner, I., Nölle, L. V., Martynenko, O. V., and Schmitt, S. (2022). Falling heads': investigating reflexive responses to head-neck perturbations. Biomed. Eng. Online 21, 25. doi:10.1186/s12938-022-00994-9
Wu, J. Z., An, K.-N., Cutlip, R. G., and Dong, R. G. (2010). A practical biomechanical model of the index finger simulating the kinematics of the muscle/tendon excursions. Biomed. Mater Eng. 20, 89–97. doi:10.3233/BME-2010-0618
Wu, J. Z., An, K.-N., Cutlip, R. G., Krajnak, K., Welcome, D., and Dong, R. G. (2008). Analysis of musculoskeletal loading in an index finger during tapping. J. Biomech. 41, 668–676. doi:10.1016/j.jbiomech.2007.09.025
Yeo, S.-H., Verheul, J., Herzog, W., and Sueda, S. (2023). Numerical instability of Hill-type muscle models. J. R. Soc. Interface 20, 20220430. doi:10.1098/rsif.2022.0430
Keywords: finite element analysis, injury criteria, jersey finger injury, muscle modelling, tendon strain injury
Citation: Nölle LV, Alfaro EH, Martynenko OV and Schmitt S (2023) An investigation of tendon strains in jersey finger injury load cases using a finite element neuromuscular human body model. Front. Bioeng. Biotechnol. 11:1293705. doi: 10.3389/fbioe.2023.1293705
Received: 13 September 2023; Accepted: 01 December 2023;
Published: 14 December 2023.
Edited by:
Jörg Miehling, Friedrich-Alexander-Universität Erlangen-Nürnberg, GermanyReviewed by:
Sigrid Leyendecker, University of Erlangen Nuremberg, GermanyMagnus Gislason, Reykjavík University, Iceland
Copyright © 2023 Nölle, Alfaro, Martynenko and Schmitt. This is an open-access article distributed under the terms of the Creative Commons Attribution License (CC BY). The use, distribution or reproduction in other forums is permitted, provided the original author(s) and the copyright owner(s) are credited and that the original publication in this journal is cited, in accordance with accepted academic practice. No use, distribution or reproduction is permitted which does not comply with these terms.
*Correspondence: Lennart V. Nölle, lennart.noelle@imsb.uni-stuttgart.de