Precise healing of oral and maxillofacial wounds: tissue engineering strategies and their associated mechanisms
- 1Hospital of Stomatology, The First Affiliated Hospital of Jinan University, Guangzhou, China
- 2Department of Stomatology, The Sixth Affiliated Hospital of Jinan University, Dongguan, China
- 3School of stomatology, Jinan University, Guangzhou, China
- 4Department of Stomatology, Baoan Central Hospital of Shenzhen, Shenzhen, China
Precise healing of wounds in the oral and maxillofacial regions is usually achieved by targeting the entire healing process. The rich blood circulation in the oral and maxillofacial regions promotes the rapid healing of wounds through the action of various growth factors. Correspondingly, their tissue engineering can aid in preventing wound infections, accelerate angiogenesis, and enhance the proliferation and migration of tissue cells during wound healing. Recent years, have witnessed an increase in the number of researchers focusing on tissue engineering, particularly for precise wound healing. In this context, hydrogels, which possess a soft viscoelastic nature and demonstrate exceptional biocompatibility and biodegradability, have emerged as the current research hotspot. Additionally, nanofibers, films, and foam sponges have been explored as some of the most viable materials for wound healing, with noted advantages and drawbacks. Accordingly, future research is highly likely to explore the application of these materials harboring enhanced mechanical properties, reduced susceptibility to external mechanical disturbances, and commendable water absorption and non-expansion attributes, for superior wound healing.
1 Introduction
The healing process for oral and maxillofacial wounds is identical to that of skin wounds and involves four distinct phases: hemostasis, inflammation, proliferation, and remodeling (Figure 1). To preserve its structural integrity, an organism initiates the wound healing process by first establishing hemostasis. It then induces key cells involved in healing, such as neutrophils, monocytes, endothelial cells, and fibroblasts, to interact with signaling molecules that regulate cell synthesis. In addition, it eliminates damaged or necrotic tissues and microorganisms, and stimulates the cells to release cytokines that promote the growth of new blood vessels (angiogenesis), the formation of granulation tissue, the deposition of collagen, and the re-growth of epithelial cells. Thus, the overall process facilitates rapid wound healing.
Owing to the high blood flow in the oral and maxillofacial regions, post-incision bleeding is observed to be more frequent and hematoma formation is facilitated. At the same time, strong tissue regeneration and repair capabilities are also demonstrated allowing the rapid healing of wounds. Nonetheless, the oral cavity mucosa is a multimicrobial habitat, and the wound is surrounded by a moist and bacterial environment due to the presence of salivary glands. In addition, facial wounds bleed more profusely, and direct exposure increases the risk of infection and scarring. Tissue engineering for oral and maxillofacial wound healing has thus far encountered the difficulty of developing adhesive tissues that can withstand humid environments and combat infections. Healing oral and maxillofacial wounds can be challenging due to various factors. The oral cavity serves as the entryway to the respiratory and digestive systems; actions such as coughing, sneezing, chewing, and swallowing can, therefore, result in mechanical interference with the wound. This interference may impede the proper fixation of the wound dressing, consequently hindering the process of wound healing. Additionally, oral movements involving the tongue and teeth may undermine the protective properties of the wound dressing, potentially resulting in its displacement and subsequent wound exposure (Wu et al., 2022). Moreover, the oral cavity is a dynamic, unstable, and moist environment that is constantly flushed with saliva and exposed to exogenous food stimuli. Sustaining attachment and retention of wound dressings is complicated by these factors, which can impede the healing of oral and maxillofacial wounds (Sun et al., 2023). When contrasting the oral mucosa and the skin, it is important to note that the functionalities of every stratum of the oral mucosa are analogous to those of the skin layers. However, the oral mucosa is equipped with salivary glands and is situated in a more moist, friction-prone, and bacterial-rich environment (Glim et al., 2013). Thus, in the context of restoring traumatic injuries to the oral and maxillofacial regions, it is imperative to develop dressings and medications that exhibit favorable adhesion, in addition to possessing effective antimicrobial and anti-inflammatory properties that can operate efficiently in humid environments.
The present article provides a comprehensive overview of tissue engineering strategies designed to promote wound healing, including the underlying mechanisms that facilitate the healing process. In particular, this review explores hydrogels, films, nanofibers, and foam sponges (Figure 2). Hydrogels can be described as shaped polymer network systems that resemble the extracellular matrix in nature (Zhang et al., 2015; Raina et al., 2022; Liu et al., 2023). These scaffolds function as three-dimensional porous networks and absorb surplus tissue exudate from the site of injury, thereby maintaining a moist wound environment that inhibits the proliferation of malignant tissues (Zhao et al., 2017). In addition, they allow oxygen to penetrate and cool the wound surface, thus alleviating patients’ discomfort and pain. By providing a physical barrier and expediting the healing process of wounds, hydrogels can function as a scaffold or temporary replacement for cell proliferation (Tomar et al., 2023). On the other hand, nanofibers have been reported to harbor anti-infective properties; thus, when loaded with chitosan, poly (ethylene oxide), and VEGF they can regulate the inflammatory response in the wound area more effectively (Xie et al., 2013). Furthermore, nanofibers can promote in vitro hemostasis and inhibit bacterial growth to prevent wound infections. Additionally, nanofibers can serve as a carrier for drug delivery, enabling the sustained release of drugs to locally act in the wound (Liu et al., 2017). Conversely, films effectively function as semi-permeable dressings and barriers to shield the trauma site from the effects of the external environment due to their thinness and elasticity. Furthermore, films offer a high degree of permeability to oxygen and water while simultaneously limiting the passage of bacteria, thus, providing a cellular barrier for wound healing (Liang et al., 2022). However, films lack a strong absorption capacity, thus rendering them suitable for mildly exuding wounds only (Liang et al., 2022). Foams, on the other hand, are comprised of polyurethane or silicone-based materials and facilitate a high degree of absorption and insulation. Not only are foams semipermeable, functioning as cellular barrier-like films (Shi et al., 2020), they also operate on a porous structure, thus, facilitating the ingress of moderate wound exudates, offering great filling capacity, and commendable shape memory (Landsman et al., 2017). In contrast, the interconnected porous structure of sponges renders them capable of absorbing large amounts of wound exudate and offer thermal insulating properties that increase tissue growth via hydrophilic and cellular interactions (Feng et al., 2019).
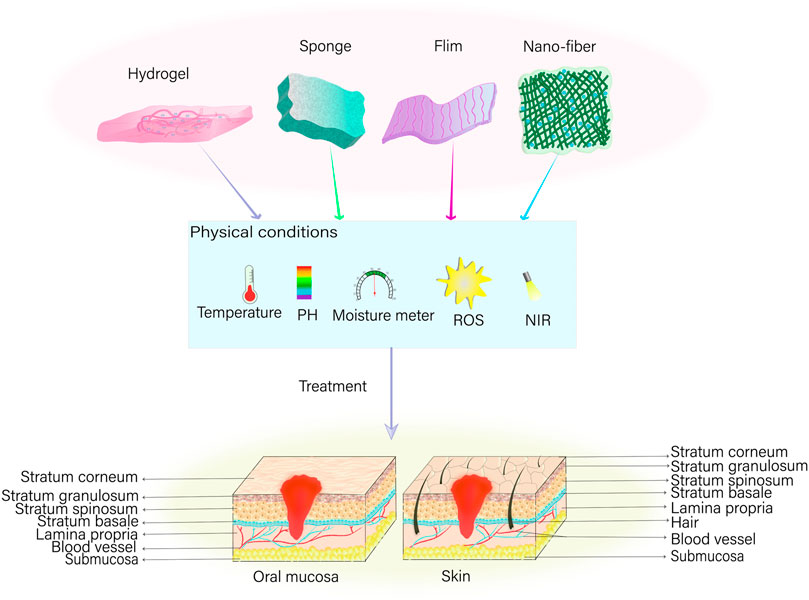
Figure 2. Tissue engineering strategies for wound healing*. In instances of oral mucous membrane or skin trauma, various dressings—including hydrogel, sponge, film, and nano-fiber—can be employed to precisely repair the affected area. This is feasible under suitable physical conditions encompassing temperature, potential of hydrogen (pH), humidity, and the concentration of reactive oxygen species (ROS), in addition to the implementation of near infrared (NIR) light-assisted irradiation on the wound.
2 Hydrogels
2.1 Polysaccharide-based hydrogels
Chitin, the most abundant animal polysaccharide, contains a high amount of hydroxyl functional groups. By synthesizing hydroxypropyl chitin and combining it with tannic acid (TA) and Fe3+, composite hydrogels have been fabricated previously (Mehrabani et al., 2018). The resulting hydrogel scaffolds, enriched with hydroxyl groups, enhance the hydrophilicity of the material, thereby allowing for better absorption of exudate from the wound area. In addition, hydrogels have been reported to facilitate the sustained release of tannic acid (TA) and Fe3+, which promotes cell proliferation and migration at the wound site (Ma et al., 2020). Furthermore, these hydrogels have been observed to aid in increasing the deposition of collagen, thereby accelerating the wound healing process. In hydrogels composed of chitin-derived chitosan (CS) (Song et al., 2019), the amino group on the surface of CS interacts electrostatically with the negative charges on the surface of platelets and erythrocytes. This interaction promotes erythrocyte aggregation and activates platelets to release PDGF and TGF, thereby enhancing platelet aggregation and inducing the hemostatic process (Shen et al., 2006). At the same time, free amino groups engage with bacteria possessing negatively charged surfaces, resulting in the disintegration and rupture of the bacterial cell wall membrane. This process eradicates the bacteria by causing the leakage of the cell contents (Sahariah et al., 2014; Raina et al., 2022). In addition, snail glycosaminoglycan (the A. fulica glycosaminoglycan, AFG) and gelatin methacrylate (GelMA)-bonded dual-network hydrogels, which is inspired by the structure of snail mucus, releases AFG to reduce the production of inflammatory substances by activating the TLR4/NF-κB signaling pathway. Furthermore, it enhances the anti-inflammatory properties of macrophages by promoting their polarization towards the M2 type. The use of such hydrogels in the repair of wounds has been observed to significantly influence the early stage of wound healing, thereby increasing collagen deposition and neovascularization for rapid recovery of the wounded area (Zhou et al., 2023). Table 1.
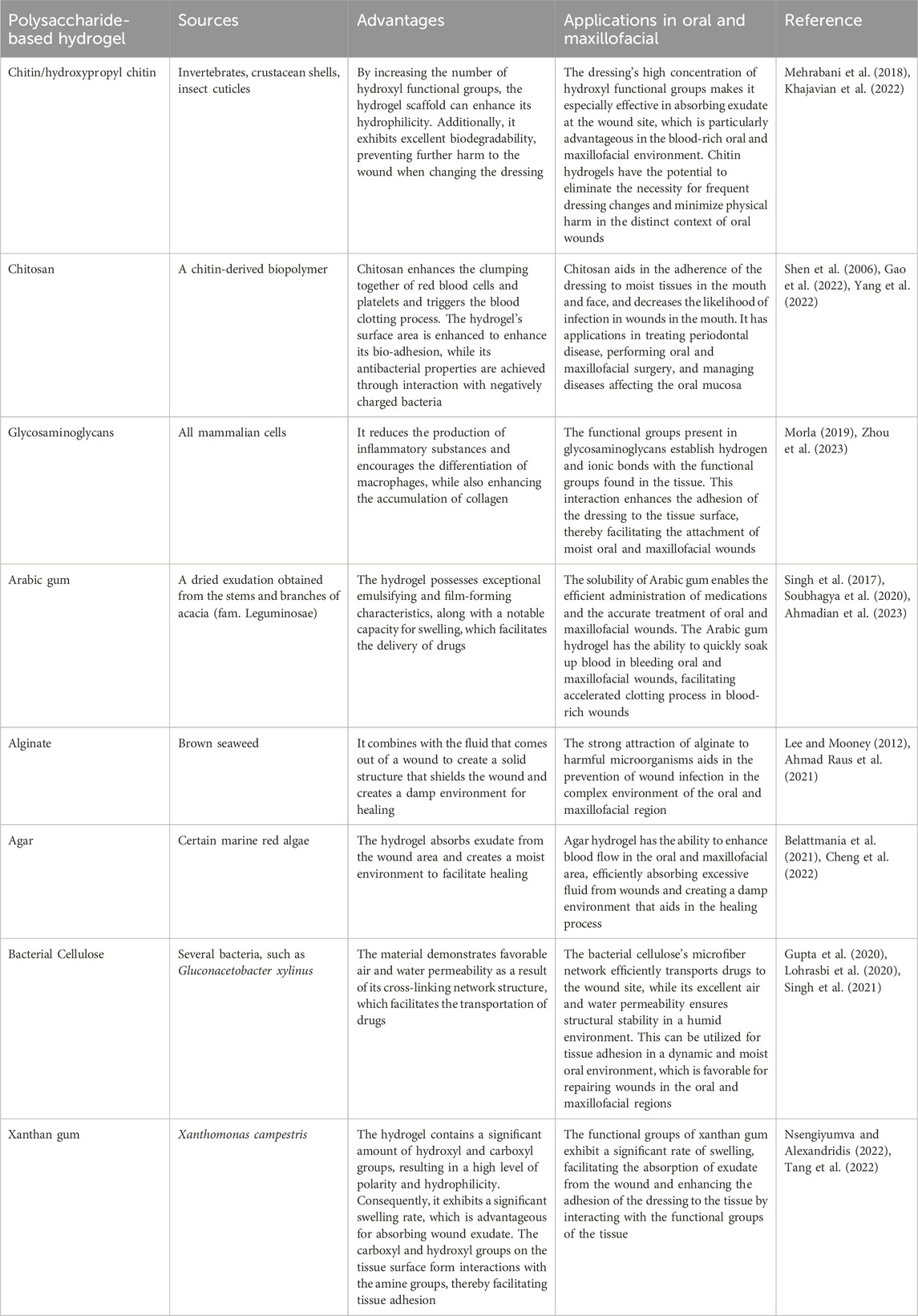
Table 1. Sources of various polysaccharide hydrogels, their advantages, and their application in oral and maxillofacial wound healing.
Among plant polysaccharides, Arabic gum (AG) polysaccharide is known for its exceptional emulsification and film-forming properties (Soubhagya et al., 2020). Similarly, pectin (PEC) is recognized for its remarkable adhesive properties to mucous membranes and its ability to reduce inflammation (Soubhagya et al., 2020). Accordingly, AG and PEC have been used as raw materials, while Naringin was incorporated to fabricate hydrogels (Alsakhawy et al., 2022). The AG-based hydrogel utilizes the tissue adhesion of PEC to release Naringin in a sustainable manner within the trauma region. This process effectively reduces the expression of inflammatory factors and controls the inflammatory response. Additionally, the hydrogel scavenges free radicals and prevents lipid peroxidation due to its antioxidant properties (Cavia-Saiz et al., 2010). It also enhances cell proliferation and differentiation by up-regulating the expression level of TGF-β mRNA. Furthermore, the hydrogel induces wound contraction, leading to faster healing, and promotes the expression of vascular endothelial growth factor, which stimulates neovascularization (Santos et al., 2021; Alsakhawy et al., 2022). This increased blood supply provides nutrients to the tissues at the trauma site and facilitates tissue regeneration.
Alginate anionic polysaccharides, which are obtained from algae, produce a gel matrix capable of protecting wounds and averting infection by interacting with cations in wound exudates and dissolving a fraction of them (Lee and Mooney, 2012). In addition, they have the potential to facilitate wound healing by creating a moist environment (Ahmad Raus et al., 2021). Accordingly, hydrogels composed of sodium alginate (SA) and gelatin have been reported to undergo a reduction in their equilibrium solubility, preventing re-injury caused by physical pressure on the wound at the time of application due to swelling (Aderibigbe and Buyana, 2018; Deng et al., 2022). Furthermore, SA adheres to tissues via the oxidation of functional groups of the molecular chain, which results in the formation of imines (Han et al., 2017; Reakasame and Boccaccini, 2018; Gao et al., 2019). Moreover, Agar polysaccharides (AGAR), which are extracted from seaweed (Belattmania et al., 2021), have been infused with fumaric acid (FA) to modify and synthesize silver nanoparticles (Ag NPs) resulting in the development of AA-Ag-FA hydrogels (Basha et al., 2020). FA promotes the angiogenic pathway to stimulate neovascularization, increase granulation tissue formation, and remodel collagen, thus accelerating wound healing. In addition, the fabrication of AGAR@PVA-TA hydrogels by mixing Agar and polyvinyl alcohol (PVA), followed by the subsequent introduction of tannic acid (TA) have been reported (Cheng et al., 2022). The use of TA improves the mechanical strength of the hydrogel owing to hydrogen bonding between the phenolic hydroxyl group of TA and the hydroxyl groups of Agar and PVA. At the same time, it enhances the ability of the hydrogel to capture erythrocytes while absorbing bleeding from the wound and promotes the production of thrombin, which expedites the hemostatic process (Ge et al., 2019; He et al., 2020; Cheng et al., 2022).
In addition to the above, bacterial cellulose (BC), derived from microorganisms, can be transformed into a hydrogel that can be loaded with drugs and specifically delivered to wound sites, promoting the healing process (Gupta et al., 2020; Lohrasbi et al., 2020). The addition of collagen to the BC-based hydrogel creates a porous structure with a larger pore size, which allows the cellulose nano fibers (CNFs) to intertwine with collagen, forming a denser network of fibers. This promotes the inward proliferation and growth of fibroblasts, as observed in studies by Moraes et al. (2016) and Pasaribu et al. (2023) (Moraes et al., 2016; Pasaribu et al., 2023). In addition, Chen et al. (2023) added Glucan and Xylan to BC hydrogel in order to enhance its ductility, improve tissue adhesion, and promote wound healing (Chen et al., 2023). Xanthan gum (XG) comprises another polysaccharide obtained from microorganisms. By combining the XG network with a covalent polyacrylamide network, the fabrication of a porous and stable hydrogel through hydrogen bonding was reported (Tang et al., 2022). This interaction relied on the abundant carboxyl and hydroxyl groups of XG to non-covalently interact with the amine groups on the tissue surface, enabling adhesion with the tissues at the wound site.
2.2 Carrier hydrogels
Nanomaterials possess the capacity to inflict damage upon bacteria by directly engaging with the bacterial cell wall membrane via their sharp edges (Akhavan and Ghaderi, 2010; Lu et al., 2022). Alternatively, they possess the capability to disturb cell membranes and provoke oxidative stress through the production of reactive oxygen species (ROS), interfering with the glycolysis process of bacteria, and disrupting bacterial DNA, among other antimicrobial effects (Kumar et al., 2011; Lakshmi Prasanna and Vijayaraghavan, 2015). Nanoparticles typically exert their antimicrobial effect through three mechanisms: the release of metal ions, the induction of oxidative stress, and non-oxidative mechanisms (Wang et al., 2017a; Barczikai et al., 2021). These mechanisms allow nanoparticles to enter bacterial membranes and interfere with the formation of bacterial biofilms. Controlled release of nanoparticles to specific locations can be achieved by utilizing the three-dimensional network structure of hydrogel (Gao et al., 2016). In this context, the synthesis of water-soluble polyethylene glycol (PEG) polymers containing catechol was achieved by imitating the adhesive properties of mussel proteins, which was then oxidized using silver nitrate to form a hydrogel (Fullenkamp et al., 2012). In addition, the incorporation of copper oxide nanoparticles (CuO NPs) (Ounkaew et al., 2020; Abdollahi et al., 2021) and silver nanoparticles (Ag NPs) (Yu et al., 2020), into carboxymethylated starch-based hydrogels have been reported. Furthermore, curcumin-cyclodextrin-characterized Ag NPs have also been incorporated into BC hydrogels (Gupta et al., 2020; Lyu et al., 2020) resulting in bactericidal action via release of nanoparticles that hinder the formation of biofilms and generate ROS. This disrupts the permeability of bacterial membranes, impairs bacterial proteins and DNA, and results in the leakage of cellular contents. Moreover, the nanoparticles incorporated into the hydrogel create a rough surface, which enhances the ability of cells to attach, multiply, and move, ultimately promoting the healing of wounds. Figure 3; Table 2.
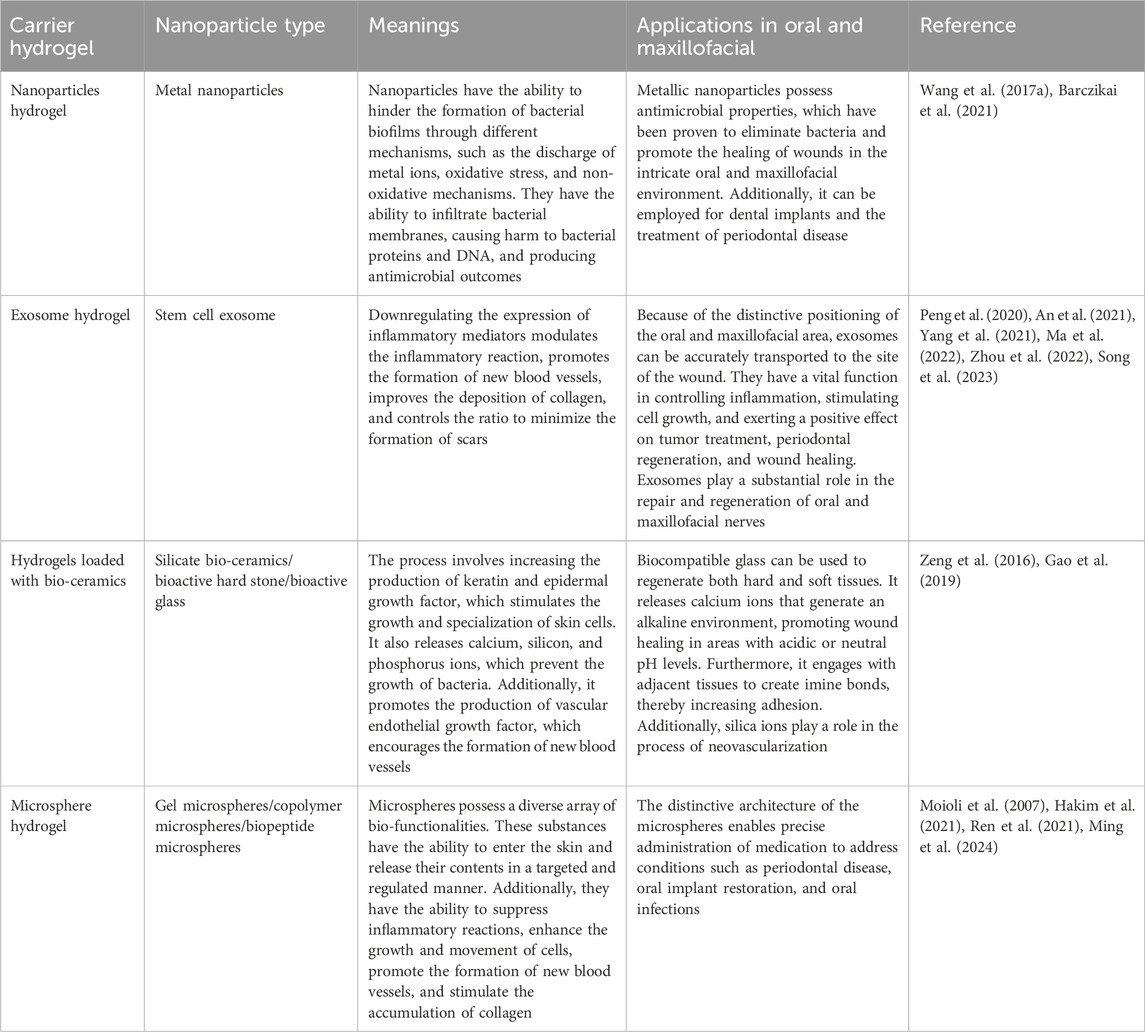
Table 2. Types of nanoparticles loaded in various hydrogels, as well as their significance and applications in the oral and maxillofacial wound healing.
The integration of exosomes into a hydrogel offers a means to transport and maintain exosomes by leveraging their three-dimensional architecture and compatibility with living organisms (Liu et al., 2017). Some examples of this include the use of adipose-derived mesenchymal stem cell exosome (ADSCs-EXO) loaded into Pluronic F-127 hydrogel (Zhou et al., 2022). These exosomes are enriched with miRNAs, which have the ability to regulate inflammatory responses by reducing the expression of TNF-a and IL-6 (Zhou et al., 2022). Additionally, they activate the PTEN/PI3K/AKT signaling pathway (Ma et al., 2022) and increase the expression of CD31 levels (Zhou et al., 2022) in order to promote neovascularization. In addition, the uptake and internalization of ADSCs-EXOs by fibroblasts can lead to an increase in the expression of N-cadherin, cyclin-1, and PCNA (Hu et al., 2016). This effect is achieved through the activation of the PI3K/AKT pathway and the Wnt/β-catenin pathway (An et al., 2021; Ma et al., 2022; Song et al., 2023), as well as the high expression of a-SMA (Zhou et al., 2022). These mechanisms ultimately promote fibroblast proliferation and migration. Activation of the TGF-β/Smad signaling pathway, as demonstrated by Liu et al. (2022) (Liu et al., 2022)promotes the production of collagen, which in turn enhances the process of re-epithelialization and accelerates wound healing. Regulating the proportion of type I and type III collagen and increasing the ratio of TGF-β3 to TGF-β1 during the later stages of wound healing has the potential to facilitate the remodeling of the extracellular matrix and minimize scar formation (Wang et al., 2017b). In addition, exosomes derived from mesenchymal stem cells (MSCs) present in human umbilical cord blood has the capacity to enhance cell proliferation for wound healing and suppress scar formation by inhibiting the differentiation of myofibroblasts (Zhang et al., 2021). This is achieved through the suppression of the TGF-β1 signaling pathway-induced overexpression of a-SMA and type I collagen (Zhang et al., 2021).
Silicate bio-ceramics have the ability to upregulate keratin, integrin β1, and epidermal growth factor, which in turn stimulates the proliferation and differentiation of epidermal cells (Wang et al., 2020; Fan et al., 2022). This effect is achieved by regulating the Wnt/β-catenin and ERK signaling pathways. One of these materials, known as bioactive hard stone (HS), facilitates the gradual release of Ca, Si, and Zn (Li et al., 2017). Another type of bio-ceramic material is bioactive glass (BG), which has the ability to release Ca, Si, and P (Wang and Tang, 2022). The hydrogel synthesis involves combining BG coated with gelatin, CS, and PDA with silver nanoparticles that are capped with curcumin (Cur-Ag NPs) (Tomar et al., 2023). The combined effect of BG and Cur-Ag NPs promotes the growth and movement of fibroblasts towards the wound site, leading to increased levels of VEGF, COL1a, and TGF-β (Tomar et al., 2023). This, in turn, enhances angiogenesis and accelerates the wound healing process. In addition, the incorporation of HS or BG into an SA-based hydrogel (Lansdown et al., 2007; Brauer, 2015; Gao et al., 2019), can lead to the release of ionic cross-linking of SA, resulting in the formation of a porous network scaffold (Lansdown et al., 2007; Brauer, 2015; Gao et al., 2019). This inhibits bacterial growth, while also promoting the multiplication and movement of keratinocytes in the area of the wound and increasing angiogenesis and re-epithelialization through the enhancement of intracellular signaling pathways. In addition, the use of lithium calcium silicate bioactive ceramic wound dressings has been shown to impact the inflammatory response in wound healing by promoting the polarization of macrophages towards the anti-inflammatory M2 type (Huang et al., 2018; Waasdorp et al., 2021; Fan et al., 2022). This is achieved by reducing the expression of IL-1β, IL-6, and TNF-a, while increasing the expression of IL-10. For example, BG has the ability to release Si ions, which can stimulate the increased expression of VEGF and bFGF. This, in turn, promotes the growth of vascular endothelial cells and fibroblasts in the wound area and enhances angiogenesis, thus expediting wound healing (Yu et al., 2016; Li et al., 2017; Zhou et al., 2018; Gao et al., 2019).
In addition, hydrogels have been employed as carriers to encapsulate pro-healing substances in the form of microspheres for the purpose of wound repair. For instance, a manganese-doped dopamine-derived hollow carbon sphere (MnOx/HNCS) were synthesized using dopamine and manganese acetylacetonate in combination with SiO2 microspheres as templates, which is cellulose-based gel microspheres (Lu et al., 2022). The gel microspheres promoted wound healing by suppressing inflammatory reactions and inducing the expression of healing-associated protein factors through the use of photothermal effects and the release of Mn2+ ions. In addition, they enhanced the growth, attachment, and movement of keratinocytes and fibroblasts (Tenaud et al., 1999; Lu et al., 2022), which in turn promoted the accumulation of collagen and facilitated the process of re-epithelialization, thereby expediting wound healing. Moreover, platelet-derived growth factor receptor (PDGF) has also been embedded in polylactic acid-glycolic acid copolymer (PLGA) (Xu et al., 2020). These microspheres were then combined with a solution containing CS/β-GF to create a PDGF-PLGA hydrogel. The PDGF was dispersed within the porous structure of the PLGA microspheres and subsequently released into the wound site via the characteristics of the CS hydrogel. This release aimed to stimulate the growth of fibroblasts and endothelial cells, thereby promoting the development of granulation tissue and neovascularization, resulting in wound healing. The production of CS-C/OPM/β-GP hydrogel has also been demonstrated by incorporating functionalized catechol chitosan (CS-C) into integral active oyster peptide microspheres (OPM) and sodium β-glycerophosphate (β-GP) (Zhang et al., 2021; Raina et al., 2022). The highly compact and consistent surface of the OPM facilitated the gradual release of oyster peptide, effectively reducing inflammatory responses by decreasing the expression of TNF-a and IL-6. In addition, it promoted the formation of new blood vessels by increasing the expression of VEGF and Ki-67, as well as the deposition of collagen fibers, which are crucial for wound healing (Zhang et al., 2021).
3 Nanofibers
3.1 Inorganic nanofibers
Carbon nanotubes (CNTs) have been employed as frameworks for precise drug delivery by binding with hydrophilic CS, enveloping CS around carbon nanotubes to augment the adhesive characteristics. The hydrophilic part of CS is exposed to the surroundings in order to facilitate the absorption of exudate from the wound area and maintain a moist microenvironment (Kittana et al., 2018). This moist environment stimulates the growth and movement of fibroblasts, increases collagen deposition, and aids in re-epithelialization.
By leveraging the structure of the nanofiber to imitate the extracellular matrix, CNTs were employed to improve the scaffold’s mechanical strength and structure, enhance adhesion between the biomaterial scaffold and tissue cells, and stimulate cell proliferation (Eivazzadeh-Keihan et al., 2019). By means of interaction with bacteria, disruption of bacterial cell membranes, and subsequent leakage of cellular contents, the integration of CNTS into the fibrous structural network of BC impedes cell proliferation and restricts activity, thereby exerting an antimicrobial effect (Kang et al., 2008; Akasaka and Watari, 2009). Furthermore, by facilitating cell adhesion and proliferation to form granulation and ensuring air-liquid exchange at the wound site via its porous structure, CNTs promote wound closure and re-epithelialization. Additionally, by stimulating neovascularization and increasing capillary density to advance tissue vascularization, BC and CNTs promote high VEGF expression in a synergistic manner, thereby accelerating the wound healing process (Khalid et al., 2022).
3.2 Organic nanofibers
Biopolymeric nanofibers synthesized via electrostatic spinning demonstrate excellent biocompatibility and biodegradability, and demonstrate significant potential for use as scaffolds when doped with drugs to stimulate cellular adhesion and proliferation (Farhaj et al., 2023). This can enhance the process of tissue regeneration and repair (Alavarse et al., 2017). By incorporating polysaccharide-based materials into nanofiber scaffolds, the healing process of wounds can be enhanced. This is achieved by delivering functional polysaccharide biopolymers directly to the wound site, controlling inflammatory reactions, promoting the growth and movement of fibroblasts, and increasing the deposition of collagen (Iacob et al., 2020).
Hyaluronic acid (HA) nanofibers consist of glucosamine, glucuronic acid, and functional groups including polyhydroxy and polycarboxylate surface groups. These groups have the ability to interact with receptors on the cell surface in order to promote the movement and proliferation of fibroblasts and endothelial cells towards the site of the wound (Li et al., 2018; Debele and Su, 2022). In order to control the bleeding, HA absorbs the blood at the site of the wound via the nanofibers’ structure and acts as an adhesive framework for platelets to promote coagulation (Astaneh and Fereydouni, 2023). Furthermore, HA stimulates the polarization of macrophages towards the M2 subtype and inhibits the release of growth factors from the cells, thereby promoting fibroblast and endothelial cell proliferation and facilitating granulation and re-epithelialization.
Chitosan nanofibers, eucalyptus oil, and cellulose acetate nanofibers have also been used to prepare wound dressings (Elbhnsawi et al., 2023). In this context, the arrangement of fibers is crucial in attracting bacterial cells and ensuring their adhesion to the nanofiber surface; on the other hand, the inclusion of 1,8-eucalyptin in eucalyptus oil enhances the permeability of bacterial cytoplasmic membranes (Aldoghaim et al., 2018). Subsequently, the disruption of the cell membranes results in the lysis of bacterial cells and the release of cellular contents, ultimately resulting in bactericidal action. In addition, the dressing stimulates the development of granulation tissue and the deposition of collagen, as well as the growth of fibroblasts, endothelial cells, and keratinocytes. This is achieved by increasing the levels of mRNA expression of TGF-β1, type I and type III collagen, which in turn leads to the formation of new blood vessels and the promotion of the regrowth of epithelial cells, facilitating the healing of wounds.
4 Biofilms
4.1 Silk fiber membranes
Silk fiber membranes (SF membranes) enhance cell migration and proliferation and facilitate wound healing by activating the NF-jB signaling pathway, which is characteristic of the wound healing process, both in vitro and in vivo, thereby inducing the expression of multiple growth factors (Li et al., 2022). In this context, SF membranes created by enclosing surface aminated liposomes (NH2-LIPs) containing leptin have been reported previously, which were subsequently submerged in a polydopamine (PDA) solution (Qian et al., 2020; Schäfer et al., 2022). The NH2-LIPs were then grafted onto the surface of the SF membranes through a reaction between the amino group and the catechol moiety of PDA. The application of PDA increases the water-attracting properties of the fibers and improves the ability of fibroblasts to stick to the fiber membranes. In contrast, the NH2-LIPs inhibits the degradation of leptin bioactivity caused by the organic solvent and oral enzymes, while also quickly releasing leptin into the damaged tissues. Moreover, it induces a notable upregulation of angiogenic factors via pathways like STAT3 and MAPK23, and enhances the growth and formation of blood vessels by promoting the proliferation of endothelial cells, thus facilitating the regeneration of oral mucosal tissue.
Moreover, flat silk cocoons (FSCs) have been reported to be able to generate a novel form of natural SF membrane that can be used directly without the requirement of a lysis and regeneration procedure (Li et al., 2022). This membrane exhibits controllable dimensions and possesses a porous layer structure, in addition to displaying exceptional mechanical properties. FSC has the ability to attract fibroblasts to the dermis and stimulate the production of growth factors, collagen, and other components of the extracellular matrix. This process enhances the speed at which damaged tissues can be repaired.
4.2 Collagen base
Collagen, a prominent constituent of the extracellular matrix, possesses the capacity to create intricate networks and merge together. It serves as both a carrier for drug delivery and a scaffold for cell growth by cross-linking and self-aggregating to create durable fibers that enhance tissue repair or regeneration at the wound site. Accordingly, collagen films serve as barriers at the wound site to prevent infection, while also gradually releasing the drug into the wound (Chattopadhyay and Raines, 2014). The biodegradable collagen film acts as a scaffold for fibroblasts, promoting cell growth and collagen synthesis. In addition, the degradation products promote the growth of fibroblasts and vascular endothelial cells, as well as facilitate the movement of keratinocytes. These cells generate and release a range of growth factors to enhance the development of extracellular matrix, angiogenesis, and re-epithelialization of tissues.
4.3 Covalent organic framework films
metal organic frameworks (MOFs) are categorized as porous polymeric substances with antimicrobial, tissue regeneration-enhancing, and drug-carrying properties (Chen et al., 2018). In the context of wound healing, Peng et al. (2022) (Peng et al., 2022) developed a bimetallic organic frameworks (BMOFs)-based dressing (BMOF-DMR) using Cu/Zn-rich metal organic frameworks (MOFs) and glucose oxidase (GOx). However, zeolitic imidazolate framework-8 (ZIF-8) comprises the most extensively utilized MOF for wound repair. In acidic wound conditions, the discharge of Zn2+ stimulates the production of ROS within the bacterial cells, resulting in the onset of oxidative stress, which subsequently disrupts the synthesis of the disorganization of bacterial cell genes, and ultimately leading to the death of the bacteria. In addition, the introduction of Cu2+ into the BMOF-DMR and the continuous release of Cu2+ stimulates the expression of VEGF and CD34, which facilitates angiogenesis, accelerates wound healing, and facilitates tissue regeneration.
Covalent organic frameworks (COFs), which may take the form of porous crystalline polymers, also serve as drug delivery vehicles due to their exceptional biocompatibility and high porosity (Zou et al., 2022; Bukhari et al., 2023). The formation of Cur @COF/PCL NFM wound dressings has been documented to occur when Cur is loaded with COFs and doped into polycaprolactone (PCL) nanofiber films via electrostatic spinning (Zou et al., 2022; Mohajer et al., 2023). Cur is released in response to PH at the wound site and interferes with Fts Z, an essential protein for bacterial cell division (Kaur et al., 2010), which inhibits bacterial proliferation. Concurrently, it promotes rapid wound healing by assisting in the reduction of TNF-a levels, which in turn decrease the concentration of inflammatory cells at the site of the wound, and by increasing VEGF expression, which stimulates angiogenesis and enhances fibroblast proliferation and migration into the wound.
By utilizing cyclodextrin metal organic framework (CD-MOF) as a template, the synthesis of a porous fiber platform containing ultrafine silver nanoparticles was also facilitated in an earlier study. Meanwhile, in a study conducted by He et al. (2023) (He et al., 2023) Ag @MOF @PDA dressings were produced by encapsulating PDA on the surface of the framework. By enhancing the water stability of the scaffolds through the synergistic action of PDA on the surface and internal cyclodextrins, CD-MOF becomes less susceptible to collapse. This, in turn, facilitates the sustained release of Ag+, which is essential for sustaining the long-term effective concentration. Furthermore, when near-infrared light irradiation is applied to the wound site, the adhesion between PDA and bacteria initially binds to the bacteria at the wound site. The disruption of the bacterial cell wall membrane by the subsequent release of a substantial quantity of Ag+ causes the cell contents to escape and the bacteria to perish.
5 Foam/sponge dressings
Polyurethane foam (PUF) has a high capacity to absorb, along with strong mechanical stability and flexibility (Sahraro et al., 2016). This makes it effective in absorbing excessive exudate at the wound site and promoting tissue growth. In addition, lignin-based polyurethane foams (LPUFs) as antimicrobial PUF dressings can be acquired by incorporating Ag NPs that are capped with lignin (Morena et al., 2020; Li et al., 2022). The phenolic hydroxyl groups in lignin interfere with the movement of protons across the cell membrane by reducing the pH within the membrane (Li et al., 2022). This leads to the breakdown and rupture of the cell membrane, causing the release of cellular contents. In addition, Ag NPs adhere to the cell membrane via electrostatic interactions, releasing Ag+ ions that interact with bacterial cell proteins and DNA residues, resulting in antimicrobial effects. The imidazole bromide was synthesized by combining a mixture of bromoethanol and imidazole in a solution of acetonitrile. The mixture obtained was employed to generate cationic polyurethane foam at room temperature (Ding et al., 2019). Moreover, the imidazole cation interacts electrostatically with the negative charge of the bacterial cell wall, leading to a decrease in the production of inflammatory cells in the wound area. Additionally, it regulates the expression levels of TNF-a, IL-1, and IL-6 to control the inflammatory response.
Earlier studies also report the use of sericin to synthesize Ag NPs, which were then combined with curcumin (Cur) to create an antimicrobial agent: sericin-Ag NPs/Cur (Se-Ag/Cur) (Jiang et al., 2023). The antimicrobial agent was enclosed within SA-CS (SC) and immersed in a CaCl2 solution to create a composite sponge called SC/Se-Ag/Cur. Sericin exhibits favorable biocompatibility, minimal immunogenicity, and promotes cell adhesion (Guo et al., 2023). These properties enhance the hydrophilicity and solubility of the composite sponge, facilitating the absorption of wound exudate. In addition, it forms a three-dimensional interconnected porous structure by utilizing the electrostatic interactions between SA and CS, as well as the ionic interactions between SA and Ca2+. This structure effectively prevents dehydration and the accumulation of exudate during the wound healing process, while also promoting the delivery of nutrients. The composite sponge, formed by the combination of Ag NPs and Cur, effectively suppressed inflammation by decreasing the expression of TNF-a and enhanced the formation of new blood vessels by promoting early CD31 expression in the wound tissue (Jiang et al., 2023). Additionally, Cur facilitated the development of granulation tissue, tissue remodeling, and collagen formation (Table 3).
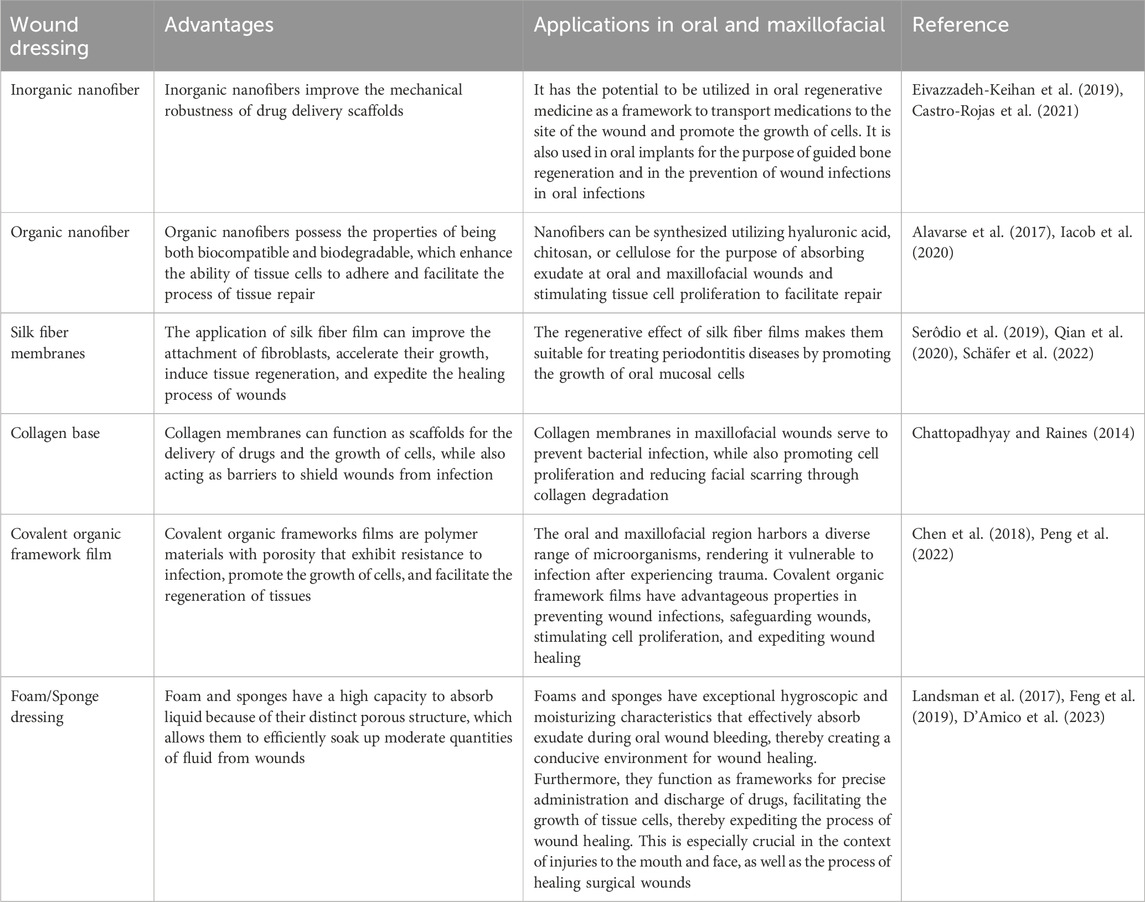
Table 3. Advantages of Nanofibers, Biofilms, and Foam Sponges for Oral and Maxillofacial wound healing.
6 Conclusion and perspectives
Hydrogels have become an intense topic of interest in modern society, where difficulties and uncertainties permeate every aspect. By capitalizing on the exceptional water absorption and retention properties of the three-dimensional structure, exudate from the wound is absorbed and a moist environment is maintained; this simultaneously lowers the temperature of the wound site and promotes the healing process. Additionally, the porous structure of the hydrogel facilitates the ingress of oxygen and water, which promotes the proliferation and growth of tissue cells in the vicinity of the wound. Adhesion of wound dressings to tissues in the oral and maxillofacial region is significantly hindered by the moist environment of the mucosa in the oral cavity and the blood of superficial skin wounds. Therefore, by capitalizing on the hydrogel’s gentle viscoelasticity and the biomaterials’ functional groups incorporated in its synthesis, an exceptional adhesion with the tissues can be achieved. However, despite possessing commendable biocompatibility and biodegradability, hydrogels fail to exhibit satisfactory mechanical properties. Despite having a network structure, hydrogels are susceptible to the impact of physiological processes occurring in the oral environment, including mastication and swallowing. Furthermore, when employed to absorb exudate from wounds, hydrogels that consist of porous hydrophilic polymer networks comprised of polymers experience an increase in mass and volume due to the presence of a considerable number of hydrophilic groups in the polymer backbone. This expansion leads to a further degradation of the hydrogels mechanical properties and stability. Additionally, the swelling hydrogel obstructs and compresses the tissue adjacent to the site of the wound, thereby impeding the tissue’s ability to heal precisely (Wu et al., 2022). In light of the aforementioned drawbacks of hydrogel, it is expected that forthcoming research will explore the application of hydrogel for wound healing, which would possess enhanced mechanical properties, decreased vulnerability to external mechanical disturbances, and superior water absorption and non-expansion characteristics.
Author contributions
QZ: Conceptualization, Funding acquisition, Writing–original draft, Writing–review and editing. CL: Conceptualization, Writing–original draft, Writing–review and editing. ML: Investigation, Writing–review and editing. KC: Writing–review and editing. RW: Writing–review and editing. YZ: Writing–review and editing. TC: Writing–review and editing. CZ: Writing–review and editing. ZL: Project administration, Supervision, Writing–review and editing.
Funding
The author(s) declare financial support was received for the research, authorship, and/or publication of this article. This work was funding by Guangdong Basic and Applied Basic Research Foundation (Grant No. 2022A1515220047) and Science and Technology Projects in Guangzhou (Grant No. 202201020069).
Conflict of interest
The authors declare that the research was conducted in the absence of any commercial or financial relationships that could be construed as a potential conflict of interest.
Publisher’s note
All claims expressed in this article are solely those of the authors and do not necessarily represent those of their affiliated organizations, or those of the publisher, the editors and the reviewers. Any product that may be evaluated in this article, or claim that may be made by its manufacturer, is not guaranteed or endorsed by the publisher.
Abbreviations
a-SMA, Alpha-smooth muscle; bFGF, Basic fibroblast growth factor; CD31, Platelet endothelial cell adhesion molecule-1, PECAM-1/CD31; CD34, Recombinant cluster of differentiation 34; Col1a, Collagen, type I, alpha 1; IL-6, Interleukin- 6; IL-10, Interleukin- 10; IL-1β, Interleukin- 1β; PCNA, Proliferating cell nuclear antigen; PDGF, Platelet-derived growth factor; TGF-β, Transforming growth factor-β; TNF-a, Tumor necrosis factor alpha; VEGF, Vascular endothelial growth factor.
References
Abdollahi, Z., Zare, E. N., Salimi, F., Goudarzi, I., Tay, F. R., and Makvandi, P. (2021). Bioactive carboxymethyl starch-based hydrogels decorated with CuO nanoparticles: antioxidant and antimicrobial properties and accelerated wound healing in vivo. IJMS 22, 2531. doi:10.3390/ijms22052531
Aderibigbe, B., and Buyana, B. (2018). Alginate in wound dressings. Pharmaceutics 10, 42. doi:10.3390/pharmaceutics10020042
Ahmadian, Z., Jelodar, M. Z., Rashidipour, M., Dadkhah, M., Adhami, V., Sefareshi, S., et al. (2023). A self-healable and bioadhesive acacia gum polysaccharide-based injectable hydrogel for wound healing acceleration. DARU J. Pharm. Sci. 31, 205–219. doi:10.1007/s40199-023-00475-x
Ahmad Raus, R., Wan Nawawi, W. M. F., and Nasaruddin, R. R. (2021). Alginate and alginate composites for biomedical applications. Asian J. Pharm. Sci. 16, 280–306. doi:10.1016/j.ajps.2020.10.001
Akasaka, T., and Watari, F. (2009). Capture of bacteria by flexible carbon nanotubes. Acta Biomater. 5, 607–612. doi:10.1016/j.actbio.2008.08.014
Akhavan, O., and Ghaderi, E. (2010). Toxicity of graphene and graphene oxide nanowalls against bacteria. ACS Nano 4, 5731–5736. doi:10.1021/nn101390x
Alavarse, A. C., De Oliveira Silva, F. W., Colque, J. T., Da Silva, V. M., Prieto, T., Venancio, E. C., et al. (2017). Tetracycline hydrochloride-loaded electrospun nanofibers mats based on PVA and chitosan for wound dressing. Mater. Sci. Eng. C 77, 271–281. doi:10.1016/j.msec.2017.03.199
Aldoghaim, F., Flematti, G., and Hammer, K. (2018). Antimicrobial activity of several cineole-rich western Australian Eucalyptus essential oils. Microorganisms 6, 122. doi:10.3390/microorganisms6040122
Alsakhawy, M. A., Abdelmonsif, D. A., Haroun, M., and Sabra, S. A. (2022). Naringin-loaded Arabic gum/pectin hydrogel as a potential wound healing material. Int. J. Biol. Macromol. 222, 701–714. doi:10.1016/j.ijbiomac.2022.09.200
An, Y., Lin, S., Tan, X., Zhu, S., Nie, F., Zhen, Y., et al. (2021). Exosomes from adipose-derived stem cells and application to skin wound healing. Cell Prolif. 54, e12993. doi:10.1111/cpr.12993
Astaneh, M. E., and Fereydouni, N. (2023). A focused review on hyaluronic acid contained nanofiber formulations for diabetic wound healing. Int. J. Biol. Macromol. 253, 127607. doi:10.1016/j.ijbiomac.2023.127607
Barczikai, D., Kacsari, V., Domokos, J., Szabó, D., and Jedlovszky-Hajdu, A. (2021). Interaction of silver nanoparticle and commonly used anti-inflammatory drug within a poly(amino acid) derivative fibrous mesh. J. Mol. Liq. 322, 114575. doi:10.1016/j.molliq.2020.114575
Basha, S. I., Ghosh, S., Vinothkumar, K., Ramesh, B., Kumari, P. H. P., Mohan, K. V. M., et al. (2020). Fumaric acid incorporated Ag/agar-agar hybrid hydrogel: a multifunctional avenue to tackle wound healing. Mater. Sci. Eng. C 111, 110743. doi:10.1016/j.msec.2020.110743
Belattmania, Z., Bhaby, S., Nadri, A., Khaya, K., Bentiss, F., Jama, C., et al. (2021). Gracilaria gracilis (gracilariales, rhodophyta) from dakhla (southern Moroccan atlantic coast) as source of agar: content, chemical characteristics, and gelling properties. Mar. Drugs 19, 672. doi:10.3390/md19120672
Brauer, D. S. (2015). Bioactive glasses—structure and properties. Angew. Chem. Int. Ed. 54, 4160–4181. doi:10.1002/anie.201405310
Bukhari, S. N. A., Ahmed, N., Amjad, M. W., Hussain, M. A., Elsherif, M. A., Ejaz, H., et al. (2023). Covalent organic frameworks (COFs) as multi-target multifunctional frameworks. Polymers 15, 267. doi:10.3390/polym15020267
Castro-Rojas, M. A., Vega-Cantu, Y. I., Cordell, G. A., and Rodriguez-Garcia, A. (2021). Dental applications of carbon nanotubes. Molecules 26, 4423. doi:10.3390/molecules26154423
Cavia-Saiz, M., Busto, M. D., Pilar-Izquierdo, M. C., Ortega, N., Perez-Mateos, M., and Muñiz, P. (2010). Antioxidant properties, radical scavenging activity and biomolecule protection capacity of flavonoid naringenin and its glycoside naringin: a comparative study. J. Sci. Food Agric. 90, 1238–1244. doi:10.1002/jsfa.3959
Chattopadhyay, S., and Raines, R. T. (2014). Collagen-based biomaterials for wound healing. Biopolymers 101, 821–833. doi:10.1002/bip.22486
Chen, S.-Q., Liao, Q., Meldrum, O. W., Guo, L., Wang, K., Zhang, S., et al. (2023). Mechanical properties and wound healing potential of bacterial cellulose-xyloglucan-dextran hydrogels. Carbohydr. Polym. 321, 121268. doi:10.1016/j.carbpol.2023.121268
Chen, W.-H., Luo, G.-F., Vázquez-González, M., Cazelles, R., Sohn, Y. S., Nechushtai, R., et al. (2018). Glucose-Responsive metal–organic-framework nanoparticles act as “smart” sense-and-treat carriers. ACS Nano 12, 7538–7545. doi:10.1021/acsnano.8b03417
Cheng, C., Peng, X., Xi, L., Wan, C., Shi, S., Wang, Y., et al. (2022). An agar–polyvinyl alcohol hydrogel loaded with tannic acid with efficient hemostatic and antibacterial capacity for wound dressing. Food Funct. 13, 9622–9634. doi:10.1039/D2FO02251F
D’Amico, E., Pierfelice, T. V., Lepore, S., Iezzi, G., D’Arcangelo, C., Piattelli, A., et al. (2023). Hemostatic collagen sponge with high porosity promotes the proliferation and adhesion of fibroblasts and osteoblasts. IJMS 24, 7749. doi:10.3390/ijms24097749
Debele, T. A., and Su, W.-P. (2022). Polysaccharide and protein-based functional wound dressing materials and applications. Int. J. Polym. Mater. Polym. Biomaterials 71, 87–108. doi:10.1080/00914037.2020.1809403
Deng, P., Yao, L., Chen, J., Tang, Z., and Zhou, J. (2022). Chitosan-based hydrogels with injectable, self-healing and antibacterial properties for wound healing. Carbohydr. Polym. 276, 118718. doi:10.1016/j.carbpol.2021.118718
Ding, Y., Sun, Z., Shi, R., Cui, H., Liu, Y., Mao, H., et al. (2019). Integrated endotoxin adsorption and antibacterial properties of cationic polyurethane foams for wound healing. ACS Appl. Mat. Interfaces 11, 2860–2869. doi:10.1021/acsami.8b19746
Eivazzadeh-Keihan, R., Maleki, A., De La Guardia, M., Bani, M. S., Chenab, K. K., Pashazadeh-Panahi, P., et al. (2019). Carbon based nanomaterials for tissue engineering of bone: building new bone on small black scaffolds: a review. J. Adv. Res. 18, 185–201. doi:10.1016/j.jare.2019.03.011
Elbhnsawi, N. A., Elwakil, B. H., Hassanin, A. H., Shehata, N., Elshewemi, S. S., Hagar, M., et al. (2023). Nano-Chitosan/Eucalyptus oil/cellulose acetate nanofibers: manufacturing, antibacterial and wound healing activities. Membranes 13, 604. doi:10.3390/membranes13060604
Fan, C., Xu, Q., Hao, R., Wang, C., Que, Y., Chen, Y., et al. (2022). Multi-functional wound dressings based on silicate bioactive materials. Biomaterials 287, 121652. doi:10.1016/j.biomaterials.2022.121652
Farhaj, S., Conway, B. R., and Ghori, M. U. (2023). Nanofibres in drug delivery applications. Fibers 11, 21. doi:10.3390/fib11020021
Feng, Y., Li, X., Zhang, Q., Yan, S., Guo, Y., Li, M., et al. (2019). Mechanically robust and flexible silk protein/polysaccharide composite sponges for wound dressing. Carbohydr. Polym. 216, 17–24. doi:10.1016/j.carbpol.2019.04.008
Fullenkamp, D. E., Rivera, J. G., Gong, Y., Lau, K. H. A., He, L., Varshney, R., et al. (2012). Mussel-inspired silver-releasing antibacterial hydrogels. Biomaterials 33, 3783–3791. doi:10.1016/j.biomaterials.2012.02.027
Gao, H., Wu, N., Wang, N., Li, J., Sun, J., and Peng, Q. (2022). Chitosan-based therapeutic systems and their potentials in treatment of oral diseases. Int. J. Biol. Macromol. 222, 3178–3194. doi:10.1016/j.ijbiomac.2022.10.090
Gao, L., Zhou, Y., Peng, J., Xu, C., Xu, Q., Xing, M., et al. (2019). A novel dual-adhesive and bioactive hydrogel activated by bioglass for wound healing. NPG Asia Mater 11, 66. doi:10.1038/s41427-019-0168-0
Gao, W., Zhang, Y., Zhang, Q., and Zhang, L. (2016). Nanoparticle-hydrogel: a hybrid biomaterial system for localized drug delivery. Ann. Biomed. Eng. 44, 2049–2061. doi:10.1007/s10439-016-1583-9
Ge, W., Cao, S., Shen, F., Wang, Y., Ren, J., and Wang, X. (2019). Rapid self-healing, stretchable, moldable, antioxidant and antibacterial tannic acid-cellulose nanofibril composite hydrogels. Carbohydr. Polym. 224, 115147. doi:10.1016/j.carbpol.2019.115147
Glim, J. E., Van Egmond, M., Niessen, F. B., Everts, V., and Beelen, R. H. J. (2013). Detrimental dermal wound healing: what can we learn from the oral mucosa? Wound Repair Regen. 21, 648–660. doi:10.1111/wrr.12072
Guo, Y., Xie, B., Jiang, M., Yuan, L., Jiang, X., Li, S., et al. (2023). Facile and eco-friendly fabrication of biocompatible hydrogel containing CuS@Ser NPs with mechanical flexibility and photothermal antibacterial activity to promote infected wound healing. J. Nanobiotechnol 21, 266. doi:10.1186/s12951-023-02035-6
Gupta, A., Briffa, S. M., Swingler, S., Gibson, H., Kannappan, V., Adamus, G., et al. (2020). Synthesis of silver nanoparticles using curcumin-cyclodextrins loaded into bacterial cellulose-based hydrogels for wound dressing applications. Biomacromolecules 21, 1802–1811. doi:10.1021/acs.biomac.9b01724
Hakim, L. K., Yazdanian, M., Alam, M., Abbasi, K., Tebyaniyan, H., Tahmasebi, E., et al. (2021). Biocompatible and biomaterials application in drug delivery system in oral cavity. Evidence-Based Complementary Altern. Med. 2021, 1–12. doi:10.1155/2021/9011226
Han, Y., Li, Y., Zeng, Q., Li, H., Peng, J., Xu, Y., et al. (2017). Injectable bioactive akermanite/alginate composite hydrogels for in situ skin tissue engineering. J. Mat. Chem. B 5, 3315–3326. doi:10.1039/C7TB00571G
He, X., Liu, X., Yang, J., Du, H., Chai, N., Sha, Z., et al. (2020). Tannic acid-reinforced methacrylated chitosan/methacrylated silk fibroin hydrogels with multifunctionality for accelerating wound healing. Carbohydr. Polym. 247, 116689. doi:10.1016/j.carbpol.2020.116689
He, Y., Wang, X., Zhang, C., Sun, J., Xu, J., and Li, D. (2023). Near-infrared light-mediated cyclodextrin metal–organic frameworks for synergistic antibacterial and anti-biofilm therapies. Small 19, 2300199. doi:10.1002/smll.202300199
Hu, L., Wang, J., Zhou, X., Xiong, Z., Zhao, J., Yu, R., et al. (2016). Exosomes derived from human adipose mensenchymal stem cells accelerates cutaneous wound healing via optimizing the characteristics of fibroblasts. Sci. Rep. 6, 32993. doi:10.1038/srep32993
Huang, Y., Wu, C., Zhang, X., Chang, J., and Dai, K. (2018). Regulation of immune response by bioactive ions released from silicate bioceramics for bone regeneration. Acta Biomater. 66, 81–92. doi:10.1016/j.actbio.2017.08.044
Iacob, A.-T., Drăgan, M., Ionescu, O.-M., Profire, L., Ficai, A., Andronescu, E., et al. (2020). An overview of biopolymeric electrospun nanofibers based on polysaccharides for wound healing management. Pharmaceutics 12, 983. doi:10.3390/pharmaceutics12100983
Jiang, M., Li, S., Ming, P., Guo, Y., Yuan, L., Jiang, X., et al. (2023). Rational design of porous structure-based sodium alginate/chitosan sponges loaded with green synthesized hybrid antibacterial agents for infected wound healing. Int. J. Biol. Macromol. 237, 123944. doi:10.1016/j.ijbiomac.2023.123944
Kang, S., Mauter, M. S., and Elimelech, M. (2008). Physicochemical determinants of multiwalled carbon nanotube bacterial cytotoxicity. Environ. Sci. Technol. 42, 7528–7534. doi:10.1021/es8010173
Kaur, S., Modi, N. H., Panda, D., and Roy, N. (2010). Probing the binding site of curcumin in Escherichia coli and Bacillus subtilis FtsZ – a structural insight to unveil antibacterial activity of curcumin. Eur. J. Med. Chem. 45, 4209–4214. doi:10.1016/j.ejmech.2010.06.015
Khajavian, M., Vatanpour, V., Castro-Muñoz, R., and Boczkaj, G. (2022). Chitin and derivative chitosan-based structures — preparation strategies aided by deep eutectic solvents: a review. Carbohydr. Polym. 275, 118702. doi:10.1016/j.carbpol.2021.118702
Khalid, A., Madni, A., Raza, B., Islam, M. U., Hassan, A., Ahmad, F., et al. (2022). Multiwalled carbon nanotubes functionalized bacterial cellulose as an efficient healing material for diabetic wounds. Int. J. Biol. Macromol. 203, 256–267. doi:10.1016/j.ijbiomac.2022.01.146
Kittana, N., Assali, M., Abu-Rass, H., Lutz, S., Hindawi, R., Ghannam, L., et al. (2018). Enhancement of wound healing by single-wall/multi-wall carbon nanotubes complexed with chitosan. IJN 13, 7195–7206. doi:10.2147/IJN.S183342
Kumar, A., Pandey, A. K., Singh, S. S., Shanker, R., and Dhawan, A. (2011). Engineered ZnO and TiO2 nanoparticles induce oxidative stress and DNA damage leading to reduced viability of Escherichia coli. Free Radic. Biol. Med. 51, 1872–1881. doi:10.1016/j.freeradbiomed.2011.08.025
Lakshmi Prasanna, V., and Vijayaraghavan, R. (2015). Insight into the mechanism of antibacterial activity of ZnO: surface defects mediated reactive oxygen species even in the dark. Langmuir 31, 9155–9162. doi:10.1021/acs.langmuir.5b02266
Landsman, T. L., Touchet, T., Hasan, S. M., Smith, C., Russell, B., Rivera, J., et al. (2017). A shape memory foam composite with enhanced fluid uptake and bactericidal properties as a hemostatic agent. Acta Biomater. 47, 91–99. doi:10.1016/j.actbio.2016.10.008
Lansdown, A. B. G., Mirastschijski, U., Stubbs, N., Scanlon, E., and Ågren, M. S. (2007). Zinc in wound healing: theoretical, experimental, and clinical aspects. Wound Repair Regen. 15, 2–16. doi:10.1111/j.1524-475X.2006.00179.x
Lee, K. Y., and Mooney, D. J. (2012). Alginate: properties and biomedical applications. Prog. Polym. Sci. 37, 106–126. doi:10.1016/j.progpolymsci.2011.06.003
Li, C., Zhang, Q., Lan, D., Cai, M., Liu, Z., Dai, F., et al. (2022a). ε-Poly-l-lysine-modified natural silk fiber membrane wound dressings with improved antimicrobial properties. Int. J. Biol. Macromol. 220, 1049–1059. doi:10.1016/j.ijbiomac.2022.08.140
Li, H., Xue, Y., Jia, B., Bai, Y., Zuo, Y., Wang, S., et al. (2018). The preparation of hyaluronic acid grafted pullulan polymers and their use in the formation of novel biocompatible wound healing film. Carbohydr. Polym. 188, 92–100. doi:10.1016/j.carbpol.2018.01.102
Li, S., Zhang, Y., Ma, X., Qiu, S., Chen, J., Lu, G., et al. (2022b). Antimicrobial lignin-based polyurethane/Ag composite foams for improving wound healing. Biomacromolecules 23, 1622–1632. doi:10.1021/acs.biomac.1c01465
Li, Y., Han, Y., Wang, X., Peng, J., Xu, Y., and Chang, J. (2017). Multifunctional hydrogels prepared by dual ion cross-linking for chronic wound healing. ACS Appl. Mat. Interfaces 9, 16054–16062. doi:10.1021/acsami.7b04801
Liang, Y., Liang, Y., Zhang, H., and Guo, B. (2022). Antibacterial biomaterials for skin wound dressing. Asian J. Pharm. Sci. 17, 353–384. doi:10.1016/j.ajps.2022.01.001
Liu, M., Duan, X.-P., Li, Y.-M., Yang, D.-P., and Long, Y.-Z. (2017a). Electrospun nanofibers for wound healing. Mater. Sci. Eng. C 76, 1413–1423. doi:10.1016/j.msec.2017.03.034
Liu, S., Zhao, Y., Li, M., Nie, L., Wei, Q., Okoro, O. V., et al. (2023). Bioactive wound dressing based on decellularized tendon and GelMA with incorporation of PDA-loaded asiaticoside nanoparticles for scarless wound healing. Chem. Eng. J. 466, 143016. doi:10.1016/j.cej.2023.143016
Liu, X., Yang, Y., Li, Y., Niu, X., Zhao, B., Wang, Y., et al. (2017b). Integration of stem cell-derived exosomes with in situ hydrogel glue as a promising tissue patch for articular cartilage regeneration. Nanoscale 9, 4430–4438. doi:10.1039/C7NR00352H
Liu, Y., Liu, Y., Wu, M., Zou, R., Mao, S., Cong, P., et al. (2022). Adipose-derived mesenchymal stem cell-loaded β-chitin nanofiber hydrogel promote wound healing in rats. J. Mater Sci. Mater Med. 33, 12. doi:10.1007/s10856-021-06630-7
Lohrasbi, S., Mirzaei, E., Karimizade, A., Takallu, S., and Rezaei, A. (2020). Collagen/cellulose nanofiber hydrogel scaffold: physical, mechanical and cell biocompatibility properties. Cellulose 27, 927–940. doi:10.1007/s10570-019-02841-y
Lu, M., Li, S., Xiong, X., Huang, Z., Xu, B., Liu, Y., et al. (2022). A photo-responsive hollow manganese/carbon hybrid nanosphere for wound disinfection and healing. Adv. Funct. Mater. 32, 2208061. doi:10.1002/adfm.202208061
Lyu, Y., Yu, M., Liu, Q., Zhang, Q., Liu, Z., Tian, Y., et al. (2020). Synthesis of silver nanoparticles using oxidized amylose and combination with curcumin for enhanced antibacterial activity. Carbohydr. Polym. 230, 115573. doi:10.1016/j.carbpol.2019.115573
Ma, J., Yong, L., Lei, P., Li, H., Fang, Y., Wang, L., et al. (2022). Advances in microRNA from adipose-derived mesenchymal stem cell-derived exosome: focusing on wound healing. J. Mat. Chem. B 10, 9565–9577. doi:10.1039/D2TB01987F
Ma, M., Zhong, Y., and Jiang, X. (2020). Thermosensitive and pH-responsive tannin-containing hydroxypropyl chitin hydrogel with long-lasting antibacterial activity for wound healing. Carbohydr. Polym. 236, 116096. doi:10.1016/j.carbpol.2020.116096
Mehrabani, M. G., Karimian, R., Mehramouz, B., Rahimi, M., and Kafil, H. S. (2018). Preparation of biocompatible and biodegradable silk fibroin/chitin/silver nanoparticles 3D scaffolds as a bandage for antimicrobial wound dressing. Int. J. Biol. Macromol. 114, 961–971. doi:10.1016/j.ijbiomac.2018.03.128
Ming, P., Liu, Y., Yu, P., Jiang, X., Yuan, L., Cai, S., et al. (2024). A biomimetic Se-nHA/PC composite microsphere with synergistic immunomodulatory and osteogenic ability to activate bone regeneration in periodontitis. Small 20, 2305490. doi:10.1002/smll.202305490
Mohajer, F., Mohammadi Ziarani, G., Badiei, A., Iravani, S., and Varma, R. S. (2023). Recent advances in covalent organic frameworks (COFs) for wound healing and antimicrobial applications. RSC Adv. 13, 8136–8152. doi:10.1039/D2RA07194K
Moioli, E. K., Clark, P. A., Xin, X., Lal, S., and Mao, J. J. (2007). Matrices and scaffolds for drug delivery in dental, oral and craniofacial tissue engineering. Adv. Drug Deliv. Rev. 59, 308–324. doi:10.1016/j.addr.2007.03.019
Moraes, P. R. F. D. S., Saska, S., Barud, H., Lima, L. R. D., Martins, V. D. C. A., Plepis, A. M. D. G., et al. (2016). Bacterial cellulose/collagen hydrogel for wound healing. Mat. Res. 19, 106–116. doi:10.1590/1980-5373-MR-2015-0249
Morena, A. G., Stefanov, I., Ivanova, K., Pérez-Rafael, S., Sánchez-Soto, M., and Tzanov, T. (2020). Antibacterial polyurethane foams with incorporated lignin-capped silver nanoparticles for chronic wound treatment. Ind. Eng. Chem. Res. 59, 4504–4514. doi:10.1021/acs.iecr.9b06362
Morla, S. (2019). Glycosaminoglycans and glycosaminoglycan mimetics in cancer and inflammation. IJMS 20, 1963. doi:10.3390/ijms20081963
Nsengiyumva, E. M., and Alexandridis, P. (2022). Xanthan gum in aqueous solutions: fundamentals and applications. Int. J. Biol. Macromol. 216, 583–604. doi:10.1016/j.ijbiomac.2022.06.189
Ounkaew, A., Kasemsiri, P., Jetsrisuparb, K., Uyama, H., Hsu, Y.-I., Boonmars, T., et al. (2020). Synthesis of nanocomposite hydrogel based carboxymethyl starch/polyvinyl alcohol/nanosilver for biomedical materials. Carbohydr. Polym. 248, 116767. doi:10.1016/j.carbpol.2020.116767
Pasaribu, K. M., Ilyas, S., Tamrin, T., Radecka, I., Swingler, S., Gupta, A., et al. (2023). Bioactive bacterial cellulose wound dressings for burns with collagen in-situ and chitosan ex-situ impregnation. Int. J. Biol. Macromol. 230, 123118. doi:10.1016/j.ijbiomac.2022.123118
Peng, L., Yang, X., Wang, S., Chan, Y. K., Chen, Y., Yang, Z., et al. (2022). Bimetal metal–organic framework domino micro-reactor for synergistic antibacterial starvation/chemodynamic therapy and robust wound healing. Nanoscale 14, 2052–2064. doi:10.1039/D1NR07611F
Peng, Q., Yang, J., and Zhou, G. (2020). Emerging functions and clinical applications of exosomes in human oral diseases. Cell Biosci. 10, 68. doi:10.1186/s13578-020-00424-0
Qian, C., Xin, T., Xiao, W., Zhu, H., Zhang, Q., Liu, L., et al. (2020). Vascularized silk electrospun fiber for promoting oral mucosa regeneration. NPG Asia Mater 12, 39. doi:10.1038/s41427-020-0221-z
Raina, N., Pahwa, R., Thakur, V. K., and Gupta, M. (2022). Polysaccharide-based hydrogels: new insights and futuristic prospects in wound healing. Int. J. Biol. Macromol. 223, 1586–1603. doi:10.1016/j.ijbiomac.2022.11.115
Reakasame, S., and Boccaccini, A. R. (2018). Oxidized alginate-based hydrogels for tissue engineering applications: a review. Biomacromolecules 19, 3–21. doi:10.1021/acs.biomac.7b01331
Ren, B., Lu, J., Li, M., Zou, X., Liu, Y., Wang, C., et al. (2021). Anti-inflammatory effect of IL-1ra-loaded dextran/PLGA microspheres on Porphyromonas gingivalis lipopolysaccharide-stimulated macrophages in vitro and in vivo in a rat model of periodontitis. Biomed. Pharmacother. 134, 111171. doi:10.1016/j.biopha.2020.111171
Sahariah, P., Gaware, V., Lieder, R., Jónsdóttir, S., Hjálmarsdóttir, M., Sigurjonsson, O., et al. (2014). The effect of substituent, degree of acetylation and positioning of the cationic charge on the antibacterial activity of quaternary chitosan derivatives. Mar. Drugs 12, 4635–4658. doi:10.3390/md12084635
Sahraro, M., Yeganeh, H., and Sorayya, M. (2016). Guanidine hydrochloride embedded polyurethanes as antimicrobial and absorptive wound dressing membranes with promising cytocompatibility. Mater. Sci. Eng. C 59, 1025–1037. doi:10.1016/j.msec.2015.11.038
Santos, T. S., Santos, I. D. D. D., Pereira-Filho, R. N., Gomes, S. V. F., Lima-Verde, I. B., Marques, M. N., et al. (2021). Histological evidence of wound healing improvement in rats treated with oral administration of hydroalcoholic extract of vitis labrusca. CIMB 43, 335–352. doi:10.3390/cimb43010028
Schäfer, S., Smeets, R., Köpf, M., Drinic, A., Kopp, A., Kröger, N., et al. (2022). Antibacterial properties of functionalized silk fibroin and sericin membranes for wound healing applications in oral and maxillofacial surgery. Biomater. Adv. 135, 212740. doi:10.1016/j.bioadv.2022.212740
Serôdio, R., Schickert, S. L., Costa-Pinto, A. R., Dias, J. R., Granja, P. L., Yang, F., et al. (2019). Ultrasound sonication prior to electrospinning tailors silk fibroin/PEO membranes for periodontal regeneration. Mater. Sci. Eng. C 98, 969–981. doi:10.1016/j.msec.2019.01.055
Shen, E., Chou, T., Gau, C., Tu, H., Chen, Y., and Fu, E. (2006). Releasing growth factors from activated human platelets after chitosan stimulation: a possible bio-material for platelet-rich plasma preparation. Clin. Oral Implants Res. 17, 572–578. doi:10.1111/j.1600-0501.2004.01241.x
Shi, C., Wang, C., Liu, H., Li, Q., Li, R., Zhang, Y., et al. (2020). Selection of appropriate wound dressing for various wounds. Front. Bioeng. Biotechnol. 8, 182. doi:10.3389/fbioe.2020.00182
Singh, B., Sharma, S., and Dhiman, A. (2017). Acacia gum polysaccharide based hydrogel wound dressings: synthesis, characterization, drug delivery and biomedical properties. Carbohydr. Polym. 165, 294–303. doi:10.1016/j.carbpol.2017.02.039
Singh, J., Tan, N. C. S., Mahadevaswamy, U. R., Chanchareonsook, N., Steele, T. W. J., and Lim, S. (2021). Bacterial cellulose adhesive composites for oral cavity applications. Carbohydr. Polym. 274, 118403. doi:10.1016/j.carbpol.2021.118403
Song, R., Zheng, J., Liu, Y., Tan, Y., Yang, Z., Song, X., et al. (2019). A natural cordycepin/chitosan complex hydrogel with outstanding self-healable and wound healing properties. Int. J. Biol. Macromol. 134, 91–99. doi:10.1016/j.ijbiomac.2019.04.195
Song, Y., You, Y., Xu, X., Lu, J., Huang, X., Zhang, J., et al. (2023). Adipose-derived mesenchymal stem cell-derived exosomes biopotentiated extracellular matrix hydrogels accelerate diabetic wound healing and skin regeneration. Adv. Sci. 10, 2304023. doi:10.1002/advs.202304023
Soubhagya, A. S., Moorthi, A., and Prabaharan, M. (2020). Preparation and characterization of chitosan/pectin/ZnO porous films for wound healing. Int. J. Biol. Macromol. 157, 135–145. doi:10.1016/j.ijbiomac.2020.04.156
Sun, J., Chen, T., Zhao, B., Fan, W., Shen, Y., Wei, H., et al. (2023). Acceleration of oral wound healing under diabetes mellitus conditions using bioadhesive hydrogel. ACS Appl. Mat. Interfaces 15, 416–431. doi:10.1021/acsami.2c17424
Tang, S., Gong, Z., Wang, Z., Gao, X., and Zhang, X. (2022). Multifunctional hydrogels for wound dressings using xanthan gum and polyacrylamide. Int. J. Biol. Macromol. 217, 944–955. doi:10.1016/j.ijbiomac.2022.07.181
Tenaud, S.-M., Jumbou, L., Dréno, , Litoux, , and Dréno, (1999). In vitro modulation of keratinocyte wound healing integrins by zinc, copper and manganese. Br. J. Dermatology 140, 26–34. doi:10.1046/j.1365-2133.1999.02603.x
Tomar, S., Pandey, R., Surya, P., Verma, R., Mathur, R., Gangenahalli, G., et al. (2023). Multifunctional, adhesive, and PDA-coated bioactive glass reinforced composite hydrogel for regenerative wound healing. ACS Biomater. Sci. Eng. 9, 1520–1540. doi:10.1021/acsbiomaterials.2c01223
Waasdorp, M., Krom, B. P., Bikker, F. J., Van Zuijlen, P. P. M., Niessen, F. B., and Gibbs, S. (2021). The bigger picture: why oral mucosa heals better than skin. Biomolecules 11, 1165. doi:10.3390/biom11081165
Wang, F., Wang, X., Ma, K., Zhang, C., Chang, J., and Fu, X. (2020). Akermanite bioceramic enhances wound healing with accelerated reepithelialization by promoting proliferation, migration, and stemness of epidermal cells. Wound Repair Regen. 28, 16–25. doi:10.1111/wrr.12742
Wang, L., Hu, C., and Shao, L. (2017a). The antimicrobial activity of nanoparticles: present situation and prospects for the future. IJN 12, 1227–1249. doi:10.2147/IJN.S121956
Wang, L., Hu, L., Zhou, X., Xiong, Z., Zhang, C., Shehada, H. M. A., et al. (2017b). Exosomes secreted by human adipose mesenchymal stem cells promote scarless cutaneous repair by regulating extracellular matrix remodelling. Sci. Rep. 7, 13321. doi:10.1038/s41598-017-12919-x
Wang, X., and Tang, M. (2022). Bioceramic materials with ion-mediated multifunctionality for wound healing. Smart Med. 1, e20220032. doi:10.1002/SMMD.20220032
Wu, J., Pan, Z., Zhao, Z., Wang, M., Dong, L., Gao, H., et al. (2022). Anti-swelling, robust, and adhesive extracellular matrix-mimicking hydrogel used as intraoral dressing. Adv. Mater. 34, 2200115. doi:10.1002/adma.202200115
Xie, Z., Paras, C. B., Weng, H., Punnakitikashem, P., Su, L.-C., Vu, K., et al. (2013). Dual growth factor releasing multi-functional nanofibers for wound healing. Acta Biomater. 9, 9351–9359. doi:10.1016/j.actbio.2013.07.030
Xu, K., An, N., Zhang, H., Zhang, Q., Zhang, K., Hu, X., et al. (2020). Sustained-release of PDGF from PLGA microsphere embedded thermo-sensitive hydrogel promoting wound healing by inhibiting autophagy. J. Drug Deliv. Sci. Technol. 55, 101405. doi:10.1016/j.jddst.2019.101405
Yang, Y., Liang, Y., Chen, J., Duan, X., and Guo, B. (2022). Mussel-inspired adhesive antioxidant antibacterial hemostatic composite hydrogel wound dressing via photo-polymerization for infected skin wound healing. Bioact. Mater. 8, 341–354. doi:10.1016/j.bioactmat.2021.06.014
Yang, Z., Yang, Y., Xu, Y., Jiang, W., Shao, Y., Xing, J., et al. (2021). Biomimetic nerve guidance conduit containing engineered exosomes of adipose-derived stem cells promotes peripheral nerve regeneration. Stem Cell Res. Ther. 12, 442. doi:10.1186/s13287-021-02528-x
Yu, H., Peng, J., Xu, Y., Chang, J., and Li, H. (2016). Bioglass activated skin tissue engineering constructs for wound healing. ACS Appl. Mat. Interfaces 8, 703–715. doi:10.1021/acsami.5b09853
Yu, N., Wang, X., Qiu, L., Cai, T., Jiang, C., Sun, Y., et al. (2020). Bacteria-triggered hyaluronan/AgNPs/gentamicin nanocarrier for synergistic bacteria disinfection and wound healing application. Chem. Eng. J. 380, 122582. doi:10.1016/j.cej.2019.122582
Zeng, Q., Desai, M. S., Jin, H.-E., Lee, J. H., Chang, J., and Lee, S.-W. (2016). Self-healing elastin–bioglass hydrogels. Biomacromolecules 17, 2619–2625. doi:10.1021/acs.biomac.6b00621
Zhang, D., Ouyang, Q., Hu, Z., Lu, S., Quan, W., Li, P., et al. (2021a). Catechol functionalized chitosan/active peptide microsphere hydrogel for skin wound healing. Int. J. Biol. Macromol. 173, 591–606. doi:10.1016/j.ijbiomac.2021.01.157
Zhang, D., Zhou, W., Wei, B., Wang, X., Tang, R., Nie, J., et al. (2015). Carboxyl-modified poly(vinyl alcohol)-crosslinked chitosan hydrogel films for potential wound dressing. Carbohydr. Polym. 125, 189–199. doi:10.1016/j.carbpol.2015.02.034
Zhang, Y., Pan, Y., Liu, Y., Li, X., Tang, L., Duan, M., et al. (2021b). Exosomes derived from human umbilical cord blood mesenchymal stem cells stimulate regenerative wound healing via transforming growth factor-β receptor inhibition. Stem Cell Res. Ther. 12, 434. doi:10.1186/s13287-021-02517-0
Zhao, X., Wu, H., Guo, B., Dong, R., Qiu, Y., and Ma, P. X. (2017). Antibacterial anti-oxidant electroactive injectable hydrogel as self-healing wound dressing with hemostasis and adhesiveness for cutaneous wound healing. Biomaterials 122, 34–47. doi:10.1016/j.biomaterials.2017.01.011
Zhou, Y., Gao, L., Peng, J., Xing, M., Han, Y., Wang, X., et al. (2018). Bioglass activated albumin hydrogels for wound healing. Adv. Healthc. Mater. 7, 1800144. doi:10.1002/adhm.201800144
Zhou, Y., Zhang, X.-L., Lu, S.-T., Zhang, N.-Y., Zhang, H.-J., Zhang, J., et al. (2022). Human adipose-derived mesenchymal stem cells-derived exosomes encapsulated in pluronic F127 hydrogel promote wound healing and regeneration. Stem Cell Res. Ther. 13, 407. doi:10.1186/s13287-022-02980-3
Zhou, Z., Deng, T., Tao, M., Lin, L., Sun, L., Song, X., et al. (2023). Snail-inspired AFG/GelMA hydrogel accelerates diabetic wound healing via inflammatory cytokines suppression and macrophage polarization. Biomaterials 299, 122141. doi:10.1016/j.biomaterials.2023.122141
Keywords: wound healing, tissue engineering, hydrogel, nanofibers, tissue repair
Citation: Zhao Q, Leng C, Lau M, Choi K, Wang R, Zeng Y, Chen T, Zhang C and Li Z (2024) Precise healing of oral and maxillofacial wounds: tissue engineering strategies and their associated mechanisms. Front. Bioeng. Biotechnol. 12:1375784. doi: 10.3389/fbioe.2024.1375784
Received: 24 January 2024; Accepted: 01 April 2024;
Published: 18 April 2024.
Edited by:
Monireh Kouhi, Isfahan University of Medical Sciences, IranReviewed by:
Jaydee Cabral, University of Otago, New ZealandYunfan Kong, Harvard Medical School, United States
Copyright © 2024 Zhao, Leng, Lau, Choi, Wang, Zeng, Chen, Zhang and Li. This is an open-access article distributed under the terms of the Creative Commons Attribution License (CC BY). The use, distribution or reproduction in other forums is permitted, provided the original author(s) and the copyright owner(s) are credited and that the original publication in this journal is cited, in accordance with accepted academic practice. No use, distribution or reproduction is permitted which does not comply with these terms.
*Correspondence: Zejian Li, tlizejian@jnu.edu.cn
†These authors have contributed equally to this work