Cerium oxide nanoparticles in wound care: a review of mechanisms and therapeutic applications
- 1School of Nursing, Southwest Medical University, Luzhou, China
- 2Wound Healing Basic Research and Clinical Application Key Laboratory of Luzhou, School of Nursing, Luzhou, China
- 3Department of Pediatrics, West China Second Hospital, Sichuan University, West China School of Nursing, Sichuan University, Chengdu, China
- 4Key Laboratory of Birth Defects and Related Diseases of Women and Children, Ministry of Education, Chengdu, China
- 5School of Basic Medical Science, Southwest Medical University, Luzhou, China
- 6Department of Ophthalmology, The Affiliated Hospital of Southwest Medical University, Luzhou, China
- 7Department of Oncology, The Affiliated Hospital of Southwest Medical University, Luzhou, China
- 8Department of Nursing, The Affiliated Hospital of Southwest Medical University, Luzhou, China
- 9Department of Transfusion, The Affiliated Hospital of Southwest Medical University, Luzhou, China
- 10Department of Radiology, The Affiliated Hospital of Southwest Medical University, Luzhou, China
- 11Department of Psychiatric, The Zigong Affiliated Hospital of Southwest Medical University, Zigong, China
- 12Zigong Psychiatric Research Center, Zigong, China
Skin wound healing is a complex and tightly regulated process. The frequent occurrence and reoccurrence of acute and chronic wounds cause significant skin damage to patients and impose socioeconomic burdens. Therefore, there is an urgent requirement to promote interdisciplinary development in the fields of material science and medicine to investigate novel mechanisms for wound healing. Cerium oxide nanoparticles (CeO2 NPs) are a type of nanomaterials that possess distinct properties and have broad application prospects. They are recognized for their capabilities in enhancing wound closure, minimizing scarring, mitigating inflammation, and exerting antibacterial effects, which has led to their prominence in wound care research. In this paper, the distinctive physicochemical properties of CeO2 NPs and their most recent synthesis approaches are discussed. It further investigates the therapeutic mechanisms of CeO2 NPs in the process of wound healing. Following that, this review critically examines previous studies focusing on the effects of CeO2 NPs on wound healing. Finally, it suggests the potential application of cerium oxide as an innovative nanomaterial in diverse fields and discusses its prospects for future advancements.
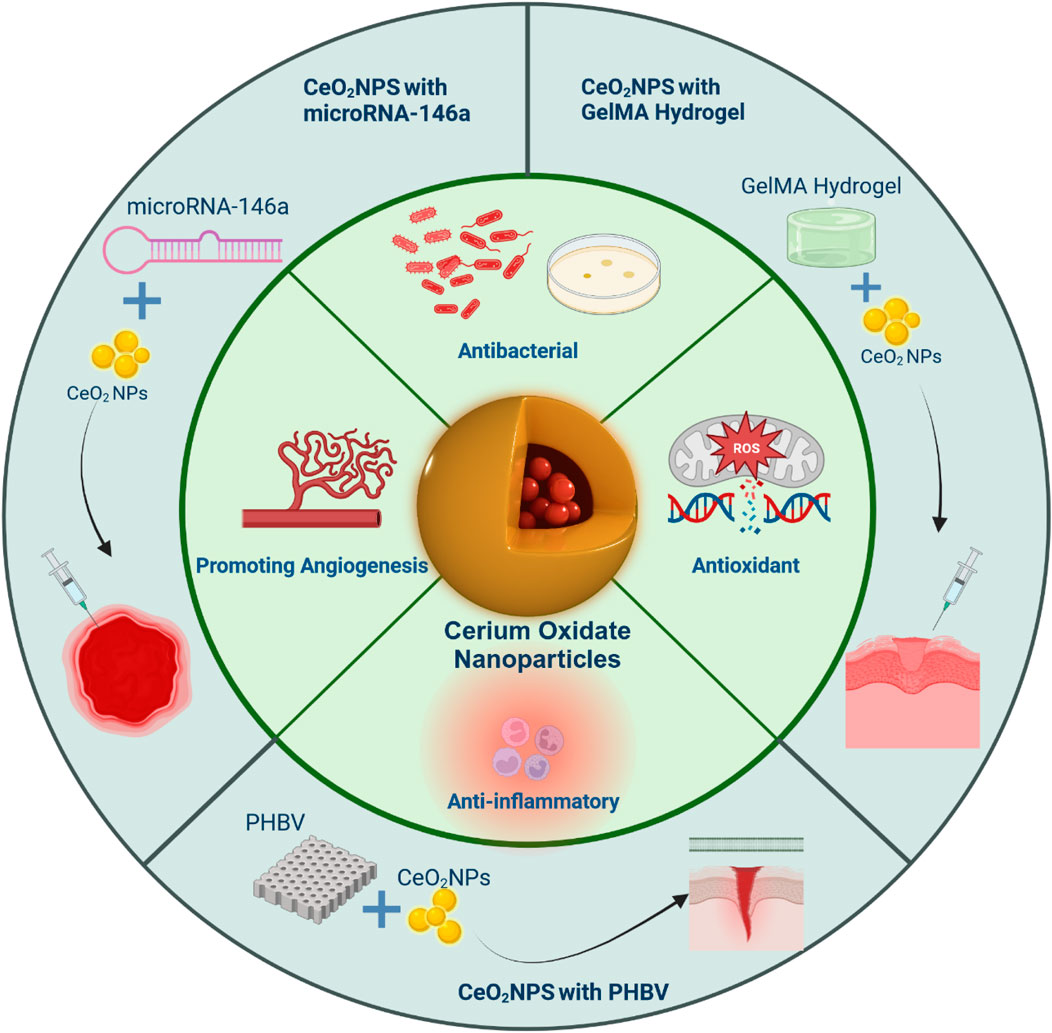
GRAPHICAL ABSTRACT | This article demonstrates the therapeutic mechanism of CeO2 NPs in wound healing. Then, the application of cerium oxide as a new nanomaterial in wound healing and the prospect of its future development are presented.
1 Introduction
Chronic and acute wounds pose significant health challenges globally. According to recent statistics from the World Health Organization, millions of people worldwide suffer from acute or chronic wounds each year resulting from accidents, trauma, or chronic diseases (Burgess et al., 2021). These wounds not only significantly impact individuals’ quality of life but also place a heavy burden on healthcare systems. It is estimated that global medical expenses related to wound care amount to tens of billions of dollars annually (Piipponen et al., 2020). Alarmingly, delays or deterioration in the wound healing process can lead to more severe complications such as infections, tissue necrosis, limb loss, and even life-threatening situations. Therefore, it is crucial to provide timely and effective wound care to protect wounds, promote healing, prevent infections and complications, and enhance patients’ comfort and psychological recovery.
Skin wound healing is a complex process that requires precise regulation of various cellular and molecular events. Numerous diseases can affect the different stages of wound healing. Consequently, discovering new therapeutic strategies that can enhance the healing of both acute and chronic wounds has always been a crucial area of research in the medical field. There is a wide range of treatments available for the healing of both acute and chronic wounds. Standard clinical treatments for wounds encompass various approaches such as skin perfusion recovery, infection management, metabolic control, complication treatment, local wound care, debridement, administration of growth factors, pressure regulation, hyperbaric oxygen therapy, and physical therapy (Comino-Sanz et al., 2021; Wang et al., 2023). Conventional dressings, such as gauze, are extensively utilized in clinics due to their convenience, affordability, and ability to quickly stop bleeding. However, they may hinder wound care and healing by promoting bacterial infections and insufficient presence of endogenous growth factors around the wound (Guo and DiPietro, 2010). Additionally, the prolonged treatment process can lead to secondary harm and have negative effects on the mental and physical wellbeing of patients. Therefore, further research on novel wound dressings that accelerate wound healing is necessary. In recent years, nanotechnology has become a promising field for developing innovative methods for wound healing due to advances in tissue engineering and regenerative medicine. The distinctive properties of nanomaterials, including their small size, high surface area-to-volume ratio, and customizable surface chemistry, make them promising candidates for wound healing applications. Nanotechnology-based methods have shown significant potential in improving wound healing outcomes through the promotion of cell proliferation and migration, inflammation reduction, angiogenesis induction, and broad-spectrum antibacterial activity. Various novel nanomaterials, including zinc oxide (Lin et al., 2017), silicon dioxide (Yang et al., 2022), gold and silver nanoparticles (Sharma et al., 2019), and cerium oxide, have been widely investigated for their potential in wound healing (Thakur et al., 2019). These nanomaterials have demonstrated positive effects on wound healing, such as excellent biocompatibility, re-epithelialization, antibacterial properties, and reduced scar formation (Baptista-Silva et al., 2021). Among them, CeO2 NPs are particularly noteworthy due to their outstanding antioxidant, anti-inflammatory, antibacterial, and angiogenic abilities, making them one of the most promising materials in this field.
CeO2 NPs exhibit advantageous physicochemical properties. CeO2 NPs have found extensive applications across diverse fields (Li et al., 2020), particularly in biomedicine, demonstrating potential for antioxidant therapy, cancer treatment, and bioimaging. Furthermore, owing to the thermal stability, favorable mechanical properties, exceptional oxygen storage capacity, and high retention rate of conjugated enzymes, the utilization of CeO2 NPs exhibits tremendous potential in wound healing (Lindholm and Searle, 2016; Singh et al., 2020).
Studies indicate that CeO2 NPs have the potential to positively impact biological processes, including inflammatory responses, cell proliferation, cell migration, and cell differentiation, thereby enhancing wound healing. Firstly, as potent antioxidants, CeO2 NPs can alleviate damage to wound tissue caused by inflammatory responses and oxidative stress (Hu et al., 2014). Secondly, they can modulate multiple cell signaling pathways, promoting cell proliferation and migration, thereby accelerating the wound healing process (Xu and Qu, 2014). Additionally, CeO2 NPs are believed to promote tissue regeneration and repair, further facilitating wound healing (Li et al., 2021; Sangha et al., 2024). These findings offer robust theoretical support for the utilization of CeO2 NPs in the realm of wound healing.
Although some promising results have been obtained, a comprehensive and in-depth understanding of the mechanisms of action and applications of CeO2 NPs in wound healing is still lacking. This review thus centers on the mechanisms of action of CeO2 NPs in wound healing and their potential applications. Through synthesizing existing research findings, this review aims to comprehensively elucidate the potential therapeutic mechanisms of CeO2 NPs in wound treatment and healing, offering a significant reference for future research and clinical applications.
2 The process and mechanism of wound healing
2.1 Hemostasis
Hemostasis is the first step in wound healing and aims to prevent bleeding. When the skin is damaged, platelets come into contact with collagen, leading to activation and aggregation, and the central thrombin triggers the formation of a fibrin mesh, which strengthens the platelet cohesive mass into a stable clot, effectively stopping bleeding (Velnar et al., 2009; Broughton et al., 2006). The most dominant feature of the hemostatic phase is the platelet, which is the main contributor to hemostasis and clotting. Platelet cytoplasm contains growth factors and cytokines such as platelet-derived growth factor (PDGF), transforming growth factor-β (TGF-β), epidermal growth factor and insulin-like growth factor. These factors play a catalytic role in the wound healing cascade by activating neutrophils, and subsequently macrophages, endothelial cells and fibroblasts (Ellis et al., 2018). Platelets also contain vasoactive amines, such as histamine and 5-hydroxytryptamine (5-HT), which cause vasodilation and increased vascular permeability (Cañedo-Dorantes and Cañedo-Ayala, 2019). This phase lasts for minutes to hours and leads to an inflammatory phase.
2.2 Inflammatory phase
The purpose of the inflammatory response is to establish an immune barrier system against microbial invasion, removal of injury-causing factors and necrotic tissue. The inflammatory phase is characterized by infiltration of immune cells such as neutrophils, macrophages and lymphocytes (Wilkinson and Hardman, 2020). Neutrophils clean cellular debris and bacteria, providing a well infiltrated wound site for wound healing (Futosi et al., 2013). Secondly, highly abundant macrophages destroy and remove bacteria, foreign particles and damaged tissue. The phagocytosis products trigger the release of cytokines, growth factors, reactive oxygen species (ROS) and protein hydrolases, which in turn attract more immune cells to the site of injury (Zhao et al., 2016). During wound healing, the wound is populated by two macrophage subpopulations, pro-inflammatory (M1) and anti-inflammatory (M2) (Koh and DiPietro, 2011). The pro-inflammatory or anti-inflammatory phenotype of macrophages is tightly controlled by signaling pathways (Wilkinson and Hardman, 2020). Finally, the cells that enter the wound are lymphocytes, which are attracted to interleukin-1 (IL-1), complement components, and immunoglobulin G (IgG) breakdown products 72 h after injury (Wang et al., 2014). IL-1 plays an important role in the regulation of collagenase, which plays an important role in collagen remodeling, production of extracellular matrix components and their degradation. This phase begins immediately after injury, usually lasts four to 6 days, and is often accompanied by edema, erythema (reddening of the skin), inflammation, and pain.
2.3 Proliferative phase
The proliferative phase is characterized by the accumulation of large numbers of cells and connective tissue leading to epithelial closure, reconstruction of damaged areas, and tissue regeneration (Midwood et al., 2004). Epidermal growth factor (EGF) initiates re-epithelialization of the epidermis, stimulating keratin formation and cell protrusion, adhesion, contraction, and detachment (Ilina and Friedl, 2009). Angiogenesis is the formation of new capillaries in blood vessels, which grow mainly from small veins near the injury. Angiogenesis contributes to cell proliferation, migration, and collagen production (Betz et al., 2016). Stimulation by vascular endothelial growth factor (VEGF) involves three major steps, namely, endothelial cell movement, differentiation and maturation. First, in response to a vascular stimulus, endothelial cells produce certain proteases that degrade to the basement membrane of the vessel on the stimulated side. After about 24 h, the endothelial cells move across the basement membrane toward the stimulus and begin to divide and proliferate, forming solid bundles of cell strips. As a result of the maturation of the endothelial cells and the impact of blood flow, the middle portion of the neoplastic cell strip bundles opens up, and blood flow enters therefrom, forming newborn capillaries (Sorg et al., 2017). Together, the new capillaries and proliferating fibroblasts are known as granulation tissue, which is necessary for connective tissue repair. Fibroblasts and myofibroblasts migrate to the wound site and rebuild new tissue. Fibroblasts are responsible for synthesizing extracellular matrix (ECM) and collagen, which is an essential protein for maintaining the structural integrity of tissues (Wilkinson and Hardman, 2020). Myofibroblasts are specialized in migrating cells that help bring the edges of injured skin closer together. The proliferative phase usually lasts for days and weeks.
2.4 Remodeling phase
Remodeling is the final phase of wound healing and is responsible for the development of new epithelium and eventual scar tissue formation. The remodeling phase is characterized by the deposition of collagen, rearrangement of fibers and contraction of scar tissue (Tracy et al., 2016). The remodeling phase requires a precise balance between apoptosis of existing cells and the production of new cells. Extracellular matrix synthesis during the proliferation and remodeling phases is initiated concurrently with granulation tissue development and can last for several years (Velnar and Bailey, 2009). The gradual degradation of large amounts of extracellular matrix (ECM) and immature type III collagen during the remodeling phase and the gradual formation of mature type I collagen lead to an increase in the tensile strength of the newly formed tissue (Darby et al., 2014; de Oliveira Gonzalez et al., 2016; Wang et al., 2024). The newly formed fibers and collagen structures are relatively heterogeneous and may take years to reorganize correctly. In particular, the differentiation of fibroblasts into myofibroblasts contributes to the reduction of wound size. Once wound contraction is complete, apoptosis occurs in the population of immune cells, blood vessels and myofibroblasts (Hinz and Gabbiani, 2003; Di Pietro, 2013).
The remodeling phase of the healing process is very complex, and any aberrations at this stage can lead to excessive wound healing or chronic wounds (Gabbiani, 2003). It is often influenced by local and systemic factors, including infection, local maceration, age, nutritional status, and body size (whole body), all of which have a relationship with healing (Růžička et al., 2021). Bacterial infections often lead to the formation of exudates and biofilms that exhibit persistent abnormal inflammation and the development of a number of post-infectious complications, thus hindering the wound healing process. Hypoxia inhibits the wound healing process and increases the risk of infection by blocking fibroblast proliferation, collagen production, and capillogenesis. This phase lasts for months or years.
3 Properties and synthesis of CeO2 NPs
3.1 Properties of CeO2 NPs
CeO2 generally occurs as the fluorite phase, with a face-centered cubic (fcc) crystal structure (Figure 1). Each cerium ion coordinates with eight oxygen ions. The electronic structure of cerium ([Xe]4f15d16s2) enables the reversible charge transfer between Ce4+ and Ce3+ states (Mehta et al., 2020). The properties of CeO2 are mainly influenced by its cubic fluorite lattice structure, which has many oxygen vacancies. These vacancies enable Ce oxides to possess both reduced CeO2 (Ce4+) and oxidized CeO2 (Ce3+) states (LI et al., 2018; Özkan et al., 2020). Ce3+ and Ce4+ can undergo interconversion during redox reactions, exhibiting activities that mimic superoxide dismutase (SOD) and catalase (CAT) (Celardo et al., 2011a; Kargozar et al., 2018). A high surface ratio of Ce3+/Ce4+ effectively catalyzes SOD mimic activity, whereas a low surface ratio of Ce3+/Ce4+ catalyzes CAT mimic activity. By controlling the Ce4+/Ce3+ ratio on the surface of CeO2 nanoparticles, it is possible to eliminate reactive oxygen species (ROS), which protects cells from oxidative stress damage and maintains a balance between macrophage anti-inflammatory and pro-inflammatory cytokines, thereby creating an anti-inflammatory microenvironment (Wang et al., 2015).
3.2 Synthesis of CeO2 NPs
The synthesis of CeO2 can be classified according to various principles. For instance, depending on the conditions of powder synthesis, the methods can be divided into gas-phase, solid-phase, and liquid-phase methods. Furthermore, they can be classified as physical or chemical methods based on the underlying reaction mechanisms. Physical methods the milling methods, detonation, microwave, melting, and combustion, while chemical methods involve precipitation, sol-gel, hydrothermal, and electrochemical methods (Darroudi et al., 2014a; Charbgoo et al., 2017a; Nadeem et al., 2020). The method of synthesizing the nanoparticles is represented in Figure 2. Each method has its own advantages and limitations, which should be carefully considered when selecting a synthesis method (Nosrati et al., 2023). The milling methods is a fast, environmentally friendly, repeatable, and cost-effective executable technology, but there are also some problems, such as the possibility of environmental pollution, the tendency of nanoparticles to agglomerate, the formation of irregularly shaped nanoparticles, and the long time required for milling and cleaning (Naidi et al., 2021). Precipitation is the most commonly used method for the synthesis of CeO2 NPs, which produces nanoparticles with smaller size distributions and higher purity, but is also more complex and time-consuming (Sun et al., 2021). The sol-gel method is a versatile technique that can be used to synthesize CeO2 NPs of various sizes and shapes. It also allows precise control of the composition and purity of the nanoparticles. However, the process can be time-consuming and requires careful control of reaction conditions (Hosseini et al., 2020). The combustion method is a quick and easy method. However, this method requires careful control of experimental conditions to avoid unnecessary side reactions and obtain nanoparticles with the desired properties (Ghahramani et al., 2020).
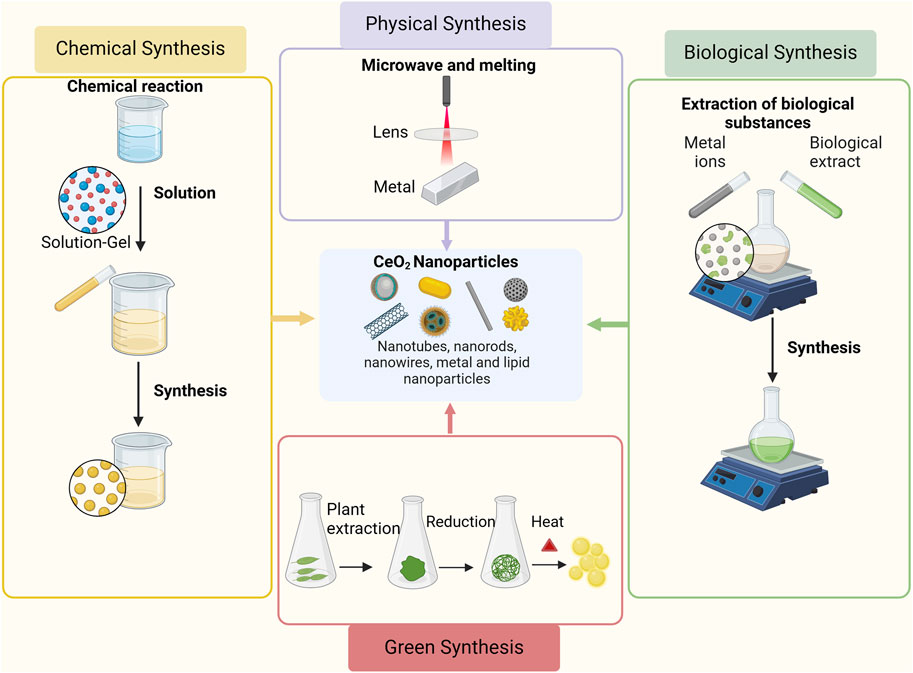
Figure 2. Synthesis methods of CeO2 NPs. Adapted from “Basic Methods of Nanoparticle Synthesis”, by BioRender.com (2020).
CeO2 NPs synthesized using different methods exhibit distinct characteristics. The unique morphologies (e.g., nanocubes, nanorods, octahedra) and exposed crystal faces (e.g (111), (110), (100)) of nanoceria enable convenient synthesis and investigation of the structure-activity relationships in catalysts (Mai et al., 2005; Xu et al., 2021). Studies indicate that rod-shaped CeO2 NPs possess greater safety and efficacy as antioxidants (Ozkan et al., 2020). Additionally, research suggests that the crystal morphology and size of nanoceria may play pivotal roles in its cytotoxicity (de Oliveira et al., 2020). Certain sizes of CeO2 NPs demonstrate elevated reactivity and interact strongly with the nanostructure and metal substrates (Castano et al., 2015; Huang et al., 2018). Size additionally impacts the enhancement of electronic conductivity, pressure-induced phase transitions, size-induced lattice relaxation, and blue shift observed in the ultraviolet absorption spectra of CeO2 NPs (Singh et al., 2018). Size can either restrict or enhance the cellular uptake of CeO2 NPs while influencing biological parameters such as biologic half-life, diffusivity, immunogenicity, as well as in vivo and in vitro toxicity (Dahle and Arai, 2015; Eriksson et al., 2018a).
The choice of synthesis conditions has a significant impact on the final product. This means that nanomaterials produced using different methods will possess distinct physical and chemical properties. It is essential to identify the synthesis methods, particularly when considering their applications in medical fields, as the properties of nanoparticles directly influence their interactions with biological interfaces. Conventional physical and chemical methods used for CeO2 synthesis often involve the use of toxic solvents or reagents, as well as the addition of external stabilizers or capping agents, which can reduce the biosafety of CeO2. For instance, in chemical synthesis methods, toxic substances may adsorb onto the surface of nanoparticles, potentially causing adverse effects in the biological environment. Therefore, the adoption of novel synthesis methods for obtaining CeO2 is particularly crucial for its biomedical applications.
The green synthesis of CeO2 NPs has attracted attention due to their abundance, biocompatibility, and environmental friendliness (Charbgoo et al., 2017a). The main methods for green synthesis of CeO2 NPs include plant-mediated, fungus-mediated, polymer-mediated, and nutrient-mediated synthesis (Darroudi et al., 2014a). In plant-mediated methods, plant extracts function as stabilizers and capping agents, resulting in the production of relatively larger CeO2 NPs (Eriksson et al., 2018b; Darroudi et al., 2014b; Nosrati et al., 2023), which are currently unsuitable for biomedical applications (Charbgoo et al., 2017b). The plant-mediated methods demonstrates the advantages of high speed, High speed, eco-friendly, pollutant- and toxicity-free, more cost-effective (no cost for culture media and microorganism isolation), simple handling, stable and non-aggregated NPs, scalability, while also not required performing genetic manipulation like microorganisms (Zuhrotun et al., 2023). Fungus-mediated methods (fungal synthesis) address this problem by producing smaller CeO2 NPs (Kargar et al., 2015), which exhibit higher stability, water dispersibility, and fluorescence properties (Nyoka et al., 2020). Polymer-mediated CeO2 NPs offer advantages in terms of manageability, cost-effectiveness, and the utilization of less time-consuming and energy-consuming techniques. Biologically oriented synthesis of CeO2 using natural substrates as stabilizers provides a safer approach to preparing CeO2 NPs, thereby alleviating concerns regarding biocompatibility (Naidi et al., 2021). An example of this is the production of water-dispersible nanopowders using PEG in aqueous solutions (Sun et al., 2021). The synthesis of CeO2 NPs using nutrients is highly cost-effective (Charbgoo et al., 2017a; Seal et al., 2020). For example, when egg white is used as a substrate for synthesizing CeO2 NPs, the egg white proteins act as stabilizers, resulting in controlled isotropic growth of small CeO2 NPs (Johnson and Wilgus, 2014). The development of various methods for the green synthesis of CeO2 NPs enables them to enhance both the speed and quality of wound healing. In particular, CeO2 NPs synthesized through green methods have demonstrated both promising antibacterial and excellent antioxidative potential in vitro (Das et al., 2014). However, the exact mechanisms, toxicity, in vivo studies, and environmental concerns related to these nanoparticles remain unresolved despite their promising potential.
4 General properties of CeO2 nanoparticles for wound healing
CeO2 NPs have been shown to exhibit various biological properties, which contribute to their extensive utilization in the field of biomedical applications. CeO2 NPs have been shown to facilitate wound healing through the mitigation of inflammation, reduction of oxidative stress responses, mitigation of infection risks, and promotion of angiogenesis throughout the wound healing process (Walkey et al., 2015). These attributes render CeO2 NPs a promising candidate for application in wound healing.
4.1 Promoting angiogenesis
CeO2 NPs can both promote and inhibit the new blood vessels (Das et al., 2012a; Chigurupati et al., 2013; Cheng et al., 2021a). Studies have shown that CeO2 NPs with diameters ranging from 3–5 nm and a higher surface Ce3+/Ce4+ ratio can stimulate the formation of vascular endothelial cell tubes in vitro. Smaller nanoparticles (≤15 nm) and higher surface Ce3+/Ce4+ ratios strongly induce angiogenesis (Figure 3). It has been observed that CeO2 NPs induce angiogenesis by modulating the intracellular oxygen environment. A correlation was found between the ability of nanoparticles to decrease intracellular oxygen levels and their potential to induce angiogenesis (Popova et al., 2020). Researchers have further investigated the impact of CeO2 NPs on the wound healing process due to their ability to modulate angiogenesis. Furthermore, CeO2 NPs promote tissue regeneration by alleviating oxidative stress. Importantly, CeO2 NPs may exhibit anti-angiogenic properties depending on the microenvironment, possibly due to their pH-dependent activity (Descamps and Emanueli, 2012).
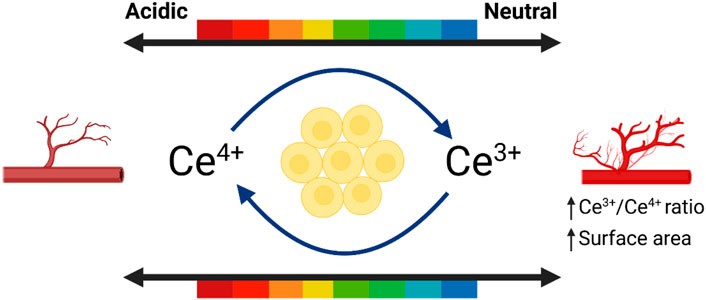
Figure 3. The influence of environment on promoting angiogenesis. Referenced and reproduced with permission (Xue et al., 2024).
CeO2 NPs can also induce vasoconstriction, activate thrombin, and facilitate platelet aggregation. CeO2 NPs regulate multiple signaling pathways, including PI3K/Akt, ERK/MAPK, and Wnt/β-catenin, to activate cell proliferation and growth. CeO2 NPs promote cell proliferation and growth through the regulation of these signaling pathways. Moreover, they enhance the activities of catalase (CAT) and superoxide dismutase (SOD), which safeguards cells from damage and facilitates wound healing. Additionally, they stimulate angiogenesis and endothelial cell proliferation, while enhancing nutrient supply to the wound site (Das et al., 2007). Research has demonstrated that CeO2 NPs facilitate the transportation of oxygen and nutrients to the injured site through vascular endothelial growth factor (VEGF) (Gopinath et al., 2015). The mediation of CeO2 NPs is facilitated by Ref-1/APEI signaling and the HIF-1α pathway. The decrease in oxygen levels temporarily triggers the nuclear translocation of hypoxia-inducible factor 1α (HIF-1α). Consequently, this stimulation leads to the expression of numerous proteins implicated in angiogenesis. Through its association with HIF-1α activation, the Ref-1/APEI signaling pathway provides direct support for the angiogenic effect of CeO2 NPs. The viability and angiogenesis of HUVECs were observed to be correlated with the upregulation of HIF-1α expression (Ludin et al., 2014; Abdal Dayem et al., 2017). In addition, CeO2 NPs counteract high levels of oxidative stress by eliminating ROS and promoting the survival of endothelial cells. Over time, this process fosters cellular generation and various endothelial cell functions, ultimately facilitating tissue repair, regeneration, and expediting the wound healing process (Damle et al., 2019; Ahmad et al., 2023). The mechanism by which cerium oxide nanoparticles promote angiogenesis is shown in Figure 4.
4.2 Redox activity
CeO2 NPs demonstrate excellent antioxidant properties, which aid in alleviating oxidative stress and inflammatory responses, thus promoting the wound healing process. Antioxidation safeguards cells from oxidative stress by allowing Ce3+ and Ce4+ oxidation states to fulfill antioxidative and pro-oxidative roles. The antioxidative effects of CeO2 NPs primarily stem from their redox capability, which is attributed to the abundance of Ce3+ and Ce4+ ions on their surface. These ions continuously interconvert, absorbing or releasing oxygen molecules as part of a redox cycle. Consequently, a redox bipolar layer forms on the surface of CeO2 NPs, offering cellular protection during oxidative stress and inflammatory responses (Cheng et al., 2021b). Additionally, CeO2 NPs can activate the Nrf2 signaling pathway to enhance the production and release of cellular antioxidants, thereby increasing cellular antioxidant capacity. CeO2 NPs also act on endothelial cells. In endothelial cells, CeO2 NPs did not affect cell viability, reduced oxidative stress, and inhibited mRNA-TF expression, VCAM-1 expression and cytokine release (Del Turco et al., 2019). All these remarkable features rely on the Ce3+/Ce4+ redox reaction (Naganuma, 2017).
Additionally, CeO2 NPs demonstrate outstanding enzymatic mimicry. They possess the ability to eliminate ROS (such as superoxide anion radicals) and reactive nitrogen species (such as nitric oxide radicals). Studies have demonstrated that the enzymatic activities of CeO2 NPs mimic antioxidants in a concentration-dependent manner, as evaluated by the DPPH radical scavenging test, superoxide dismutase (SOD) mimic activity test, and catalase mimic activity test. Catalase mimic activity measurements revealed that the activity increased until a concentration of 1,000 μg/mL of CeO2 NPs was reached (Ighodaro and Akinloye, 2018). Catalase (CAT) and superoxide dismutase (SOD) are enzymes with antioxidative properties, and one of the capabilities of CeO2 NPs is to eliminate ROS. Through the increase in catalase (CAT) mimic activity using Ce4+ ions and superoxide dismutase (SOD) mimic activity using Ce3+ ions (Burello and Worth, 2011; Kaarniranta et al., 2019; Lu et al., 2016), ROS can be eliminated. ROS, as signaling mediators, regulate cell growth, proliferation, differentiation, apoptosis, and autophagy (Nelson et al., 2016; Dutta et al., 2022). However, elevated levels of ROS can induce oxidative stress responses and contribute to pathogenesis (Datta et al., 2020). Additionally, CeO2 NPs can scavenge ROS at high concentrations and acidic pH, while reducing radicals at low concentrations and neutral pH (Khurana et al., 2018). Therefore, the antioxidant properties of CeO2 NPs depend on the concentration and pH value. Moreover, CeO2 NPs can undergo self-renewal and switch flexibly between antioxidative and pro-oxidative states (Liu et al., 2020). Pro-oxidative properties dominate at lower pH values (Clark et al., 2011). Pro-oxidative systems increase oxidative stress, which ultimately leads to cell apoptosis (Celardo et al., 2011b). Numerous experimental data show that CeO2 NPs protect against cell apoptosis induced by oxidative stress (Augustine et al., 2020; Purohit et al., 2020). In fact, the antioxidant system serves as the foundation for anti-apoptotic and pro-survival actions. The antioxidative properties of CeO2 NPs catalyze the decomposition of ROS, promoting rapid wound healing (Zholobak et al., 2016; Sadidi et al., 2020). The antioxidant mechanism of cerium oxide nanoparticles is shown in Figure 5.
4.3 Antibacterial properties
CeO2 NPs exhibit antibacterial activity by inhibiting wound infections and bacterial growth, thereby reducing infection risks. The main antibacterial mechanism of CeO2 NPs involves direct contact with the bacterial membrane. Firstly, positively charged nanoparticles adsorb onto the negatively charged membrane of both Gram-negative and Gram-positive bacteria, causing them to adhere to the bacterial surface. This adsorption prevents the nanoparticles from penetrating the bacterial membrane, enabling them to persist on the bacterial surface for an extended period of time. Subsequently, these nanoparticles alter the viscosity of the membrane, disrupt the function of specific ion pumps, and significantly impact the exchange of substances between bacterial cells and the environment, thereby ultimately hindering bacterial growth (Babenko et al., 2012). Secondly, once CeO2 NPs are adsorbed onto the outer membrane of bacterial cells, they exert detrimental effects on proteins. One consequence of this interaction might be the release of cerium ions, which could disturb the bacterial electron flow and respiration (Li et al., 2012). Furthermore, these released ions might react with thiol groups (-SH) or be absorbed by transporters and/or pore proteins, potentially impeding nutrient transport (Das et al., 2012b). Moreover, the irregular shapes and rough edges of CeO2 NPs may induce physical damage to the bacterial membrane, particularly in Gram-positive bacteria. The main cause of nanomaterial toxicity in certain biological systems is the generation of ROS, which leads to oxidative stress (Park et al., 2020). ROS can induce the chemical breakdown of different organic components in microbes, leading to significant harm to bacteria. CeO2 NPs can also increase ROS levels in bacterial cells, potentially resulting in damage to DNA, RNA, proteins, lipids, and polysaccharides. Studies demonstrate the indirect interaction between CeO2 NPs and microbes enclosed in polysaccharides or forming biofilms, as CeO2 NPs are unable to directly penetrate the cell membrane (Chen et al., 2017). In this particular mechanism, the release of cerium ions from nanoparticle dissolution and the formation of ROS on the particle surface are of vital importance. ROS can target nucleic acids, proteins, polysaccharides, lipids, and other biomolecules, resulting in functional impairment and ultimately leading to the death and decomposition of bacteria. CeO2 NPs induce oxidative stress in the presence of bacteria (E. coli) by interacting with lipids or proteins within the microbial cells (Hirst et al., 2009). Hence, the antibacterial mechanism of CeO2 NPs is primarily mediated through the induction of oxidative stress by intracellular reactive oxygen species (ROS) (Sharma et al., 2020). The mechanism of antimicrobial activity of cerium oxide nanoparticles is shown in Figure 6.
4.4 Anti-inflammatory effects
The excessive and prolonged generation of ROS leads to oxidative stress, which contributes to the progression of inflammation and tissue damage. Therefore, because of their ability to scavenge ROS, CeO2 NPs are considered as anti-inflammatory agents. Studies have shown that CeO2 NPs can quench ROS in J774A cells and inhibit the production of iNOS. Hence, CeO2 NPs have potential therapeutic applications in the treatment of inflammatory diseases (Hirst et al., 2009). CeO2 NPs possess the ability to clear ROS, suppress inflammation, reduce cytokine levels, and protect cells both in vivo and in vitro. By inhibiting oxidative stress and activating the NF-κB signaling pathway, CeO2 NPs can reduce apoptosis and the release of inflammatory mediators, thereby lowering inflammation. Additionally, CeO2 NPs can inhibit the activity of epigenetic modifying enzymes, further reducing inflammatory responses. The anti-inflammatory effect of cerium oxide nanoparticles on endothelial cells plays a key role in wound healing and promotes rapid wound repair, Gojova A et al. incubated with CeO2 NPs at different concentrations (0.001–50 μg/mL) for 4 h, and subsequently measured the mRNA levels of three inflammatory markers, intercellular ad HAECs hesion molecule 1 (ICAM-1), interleukin (IL)-8, and monocyte chemotactic protein (MCP-1), using real-time polymerase chain reaction (PCR). (IL)-8 and monocyte chemotactic protein (MCP-1) mRNA levels. Even at the highest dose, CeO2 NPs rarely induced an inflammatory response in HAEC. This material is apparently harmless compared to Y (2)O (3) and ZnO nanoparticles that we have previously studied. These results suggest that the inflammatory response of HAEC after acute exposure to metal oxide nanoparticles is highly dependent on the particle composition. CeO2 NPs demonstrated excellent anti-inflammatory effects (Gojova et al., 2009). In another study, J. Ribera et al. evaluated the effects of albumin-coated 4 nm CeO2 NPs on primary endothelial cells isolated from the portal vein of cirrhotic rats and found that treatment with CeO2NPs reduced the pro-inflammatory state of endothelial cells, promoted an M2-like phenotype in macrophages in endothelial cell-conditioned media (antioxidant/regenerative), and reduced M1 polarization (pro-oxidant/defensive) (Ribera et al., 2019).
Research suggests that the distinctive valence state of CeO2 NPs serves as a scavenger for free radicals, enabling them to eliminate ROS or free radicals and suppress the generation of inflammatory mediators in macrophages (Saleh et al., 2020). They are also capable of downregulating the gene expression of pro-inflammatory cytokines (TSLP, Leukemia Inhibitory Factor (LIF), interleukin three and interleukin 7 (IL3 and IL7)), while increasing the expression of anti-inflammatory IL-6 and IL-13 (Kyosseva et al., 2013). Previous studies have demonstrated that CeO2 NPs do not induce an inflammatory response, even at high concentrations, such as 50 μg/mL (Wei et al., 2021). Some experimental data also indicate that CeO2 NPs have the potential to alleviate inflammation both in vitro and in vivo, thereby reducing the production of ROS in inflammatory conditions (Wang et al., 2018). Researchers have conducted in vivo studies, revealing that CeO2 NPs deposited in mouse tissues are non-pathogenic (Gagnon and Fromm, 2015; Bai et al., 2020; Ezhilarasu et al., 2020). Therefore, CeO2 NPs can alleviate inflammatory responses and decrease ROS production in inflammatory conditions.
The anti-inflammatory mechanism of cerium oxide nanoparticles is demonstrated in Figure 7.
5 Applications of CeO2 in wound healing
Cerium oxide nanoparticles are often combined with other biomaterials for healing efficacy investigations. This integration is crucial for effectively releasing nanoparticles, extending drug release duration, and accelerating wound healing. Ultimately, the goal of this strategy is to expand the utilization of CeO2 in wound healing and improve treatment efficiency. In this article, we explore recent and relevant studies demonstrating the potential of CeO2 NPs conjugated polymeric scaffolds in wound healing applications. Specifically, the potential use of CeO2 NPs in repairing diabetic wounds has a significant impact on wound healing (Barbu et al., 2021; Chen et al., 2021; Stan et al., 2021).
5.1 CeO2 NPs with GelMA hydrogel
A hydrogel patch made of biodegradable gelatin methacryloyl (GelMA), which contains CeO2 NPs, was developed to enhance the healing of diabetic wounds. A composite, injectable hydrogel consisting of cerium-containing bioactive glass (Ce-BG) and GelMA was prepared for the treatment of diabetic skin wounds. This composite hydrogel demonstrated multifunctional properties, including the inhibition of bacterial growth and enhancement of angiogenic activity, leading to an acceleration in the healing process of diabetic skin wounds (Augustine et al., 2021). Additionally, a wound healing patch composed of gelatin methacryloyl hydrogel loaded with CeO2 NPs was developed (Sener et al., 2020; Dewberry et al., 2022). The morphology, physical-mechanical properties, radical scavenging activity, in vitro cell proliferation, and in vivo diabetic wound healing activity of this patch were extensively analyzed. The highly porous and biodegradable patch exhibited excellent capability in absorbing exudate. In conclusion, the results indicate that the GelMA hydrogel loaded with CeO2 NPs has significant potential as a material for the development of therapeutic patches for the treatment of diabetic wounds, demonstrating good efficacy and promising prospects in wound healing. (Figure 8).
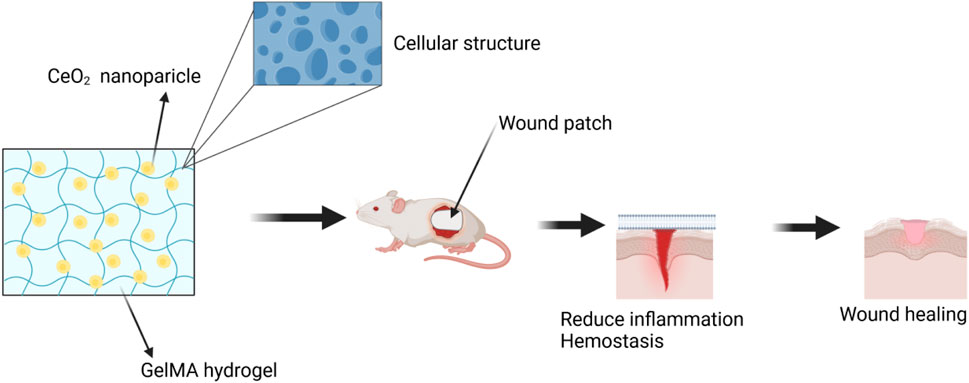
Figure 8. The wound was treated with a combination of CeO2 NPs and GelMA hydrogel. Referenced and reproduced with permission (Kyosseva et al., 2013).
5.2 CeO2 NPs with microRNA-146a
The CNP-miR146a conjugate, consisting of CeO2 NPs and microRNA (miR)-146a, was found to enhance diabetic wound healing. CeO2 NPs, a divalent metal oxide, functions as an antioxidant by scavenging free radicals, and miR146a inhibits the pro-inflammatory NFκB pathway. Consequently, CNP-miR146a exerts a synergistic effect in regulating both oxidative stress and inflammation. Intradermal injection of CNP-miR146a was found to promote collagen production, enhance angiogenesis, reduce inflammation and oxidative stress, ultimately resulting in accelerated closure of diabetic wounds (Stager et al., 2022). Additionally, a biomaterial system based on an amphoteric ionic cryogel (gel formed at sub-zero temperatures) incorporating CNP-miR146a was developed. This system is suitable for local injection, self-healing, and provides sustained release of therapeutic molecules (Zgheib et al., 2019). The researchers utilized CeO2 NPs tagged with microRNA-146a (CNP-miR146a) to address oxidative stress and inflammation. In an in vivo diabetic mouse wound healing model, both the amphoteric ionic hydrogel alone and the hydrogel loaded with CNP-miR146a conjugate demonstrated significant improvements in diabetic wound healing rates (Niemiec et al., 2020). A dose of 100 ng of CNP-miR146a was found to improve diabetic wound healing without compromising the biomechanical properties of the healed skin (Rivera-Briso and Serrano-ArocaÁ, 2018). Moreover, research has shown that nano-silk can enhance the strength of diabetic skin, delivering CNP-miR146a to improve wound healing. The nano-fiber solution not only enhances the biomechanical properties of diabetic skin but also successfully delivers CNP-miR146a, thereby improving diabetic wound healing through the inhibition of pro-inflammatory gene signaling and the promotion of fibrogenic processes (Augustine et al., 2020). In conclusion, this nanotechnology-based therapy shows promise, and future research should focus on translating it into clinical applications (Figure 9).
5.3 CeO2 NPs with PHBV (Poly (3-hydroxybutyrate-co-3-hydroxyvalerate))
PHBV(Poly (3-hydroxybutyrate-co-3-hydroxyvalerate)) is a biodegradable, non-toxic, and biocompatible polyhydroxyalkanoate polymer (Luo et al., 2023). Cell membranes made of PHBV and encapsulating CeO2 NPs have shown potential in the treatment of complications in diabetic wound healing. In in vivo experiments on diabetic rats, this membrane demonstrated the ability to repair wounds. We developed a novel membrane made of electrospun poly (3-hydroxybutyrate-co-3-hydroxyvalerate) (PHBV) containing CeO2 for applications in diabetic wound healing. We evaluated the membrane’s potential for cell proliferation, angiogenesis, and wound healing through in vitro cell adhesion studies, chick embryo angiogenesis assays, and in vivo experiments on diabetic wound healing. The results showed that the PHBV membrane containing CeO2 promoted cell proliferation and adhesion when used as a wound dressing, leading to improved healing of diabetic wounds (Aalapati et al., 2014). We prepared nanofibers using the electrospinning method, which were based on PHBV and CeO2 NPs. The electrospinning process resulted in the formation of highly porous nanofiber matrices, enabling the free diffusion of oxygen and nutrients. This facilitates rapid and successful wound healing (Sadidi et al., 2020). CeO2 NP containing PHBV promotes wound healing in Figure 10.
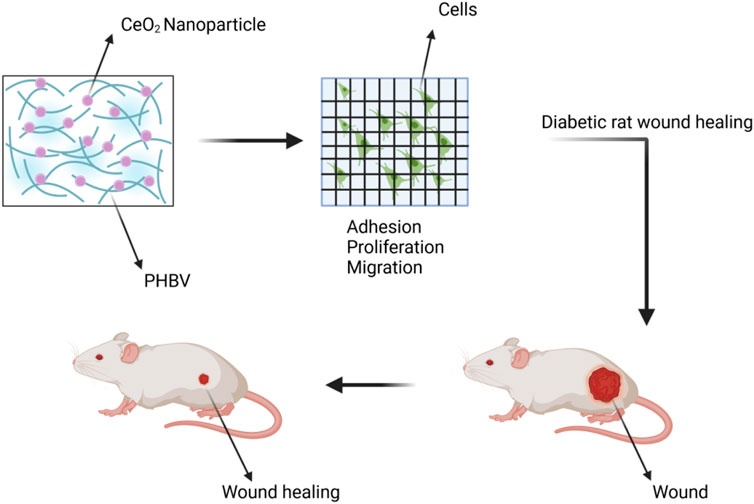
Figure 10. The wound was treated with a combination of CeO2 NPs and PHBV (Poly (3-hydroxybutyrate-co-3-hydroxyvalerate)).
6 Recent advancements
In order to have a deeper understanding of CeO2 NP, researchers have actively studied and summarized new research progress on CeO2 NPs. Firstly, Pandey S et al. found that different temperature ranges had a strong effect on the redox activity of CeO2 NPs, and observed that the superoxide dismutase mimetic activity decreased with decreasing temperature. CeO2 NP were also found to inhibit α-amylase activity by up to 60% at a concentration of 1 mM, suggesting their potential application in anti-diabetic wound healing therapy. In conclusion, CeO2 NP have found application in mitigating oxidative stress-related diseases exhibited by their high antioxidant, antimicrobial and antidiabetic behavior (Pandey et al., 2024). Secondly, Bai Y et al. state that due to their excellent catalytic activities, CeO2 NP have promise as biological nanoenzymes. A redox reaction occurs between Ce3+ ions and Ce4+ ions during which they undergo conversion by acquiring or losing electrons as well as forming oxygen vacancies (or defects) in the lattice structure, which can act as antioxidant enzymes and simulate various enzyme activities. A number of CeO2 NPs have been engineered with multienzyme activities, including catalase, superoxide oxidase, peroxidase, and oxidase mimetic properties. CeO2 NPs have nitric oxide radical clearing and radical scavenging properties and have been widely used in a number of fields of biology, including biomedicine, disease diagnosis, and treatment (Bai et al., 2024). In addition, new advances have also been made in the molecular pathways involved in wound healing with CeO2 NP. He S et al. found NLRP3 pathway-targeting ability to mediate mitochondrial function recovery. DEGs significantly enriched in immune, metabolic and complement cascade related pathways, particularly in NLRP3 pathway. TNFR2 non-canonical NF-kB pathway and TNFs binding their physiological receptors pathways were also enriched. Specifically, though treated with CeO2–Y@ZIF-8@Gel, the Ox-mtDNA was either repaired or less escaped within mitochondria via mPTP-dependent channels to inhibit cytosolic NLRP3 inflammasome (a key sensor and effector of tissue damage) initiation and IL-1β secretion leading Macrophages polarization. Meanwhile, cGAS-STING pathway activation in Macrophages was reduced as less mtDNA was uptaken and Macrophages ultimately exhibited polarization to the anti-inflammatory phenotype (He et al., 2024). Conventional nanozymes have limitations in preventing the continuous production of ROS, including the most oxidizing reactive hydroxyl radical (·OH), although they can remove pre-existing ROS. Zhu Z et al. found that a novel antioxidant nanoplatform addresses this challenge by incorporating JSH-23 into the mesoporous of cupric-doped cerium oxide nanozymes. Additionally, for rapid wound adaptability and durable tissue adhesion, a nanozyme hydrogel spray consisting of oxidized sodium alginate and methacrylate gelatin is constructed, named OG@CCJs. This platform resurrects Nrf2 transcriptional activity of macrophages in vitro, curbing the production of ROS at its source, particularly ·OH, while enabling the nanozymes to scavenge previously generated ROS. OG@CCJs significantly alleviate oxidative stress in diabetic wounds in vivo, promoting wound healing. Overall, the proposed nanozyme-hydrogel spray with enhanced ·OH-scavenging activity uses a “two-track” antioxidant strategy to rebuild the antioxidant defense barrier of macrophages (Zhu et al., 2024). Carvajal S et al. showed that CeO2 NPs reverted the H2O2-mediated increase in the phosphorylation of peptides related to cellular proliferation, stress response, and gene transcription regulation, and interfered with H2O2 effects on mTOR, MAPK/ERK, CK2A1, and PKACA signaling pathways (Carvajal et al., 2019).
In conclusion, these latest researches will push the research of CeO2 NPs to be more perfect, and together they will promote the development of biomedicine, which will be more favorable to the development of nanoparticles in the future, and push more researchers to carry out researches to improve the nanoparticles.
7 Challenges and prospects
7.1 Toxicity and safety of CeO2 NPs
Although the potential of CeO2 NP in various biomedical applications has been demonstrated, there are still shortcomings in the existing studies (Rzigalinski et al., 2017; Maccarone et al., 2020). In terms of toxicity considerations, the toxicity of CeO2 NPs is affected by a variety of factors, including particle size, synthesis method, cell type, dose/concentration, exposure time and exposure route (Li et al., 2023). First, in terms of dose and concentration, CeO2 NPs are generally not toxic in vivo at therapeutic doses. However, excessive release of cerium ions and at high doses (>1 10th of a milligram of CeO2 per kilogram of animal) show toxicity. The rapid release of CeO2 NPs has the following potential toxicity issues: first, the rapidly released cerium oxide nanoparticles may have direct toxic effects on cells and tissues around the wound. These toxic effects may include oxidative stress, apoptosis, DNA damage, etc., leading to damage of cell structure and function. These damages may delay the wound healing process and even trigger complications such as inflammation and infection. Second, cerium oxide nanoparticles may also interact with biomolecules in the wound and interfere with normal biological processes. For example, they may bind to biomolecules such as proteins and DNA, affecting their function and expression. These interferences may negatively affect the wound healing process, such as affecting cell proliferation, migration and differentiation. In addition, rapidly released cerium oxide nanoparticles may trigger an immune response, leading to inflammation and activation of immune cells. Although a moderate immune response can help wound healing, an excessive immune response may lead to tissue damage and increased inflammation, thereby affecting wound healing. Therefore, the potential risks of cerium oxide nanoparticles in wound healing should be carefully evaluated in practical applications and appropriate protective measures should be taken to ensure patient safety. So determining the ideal concentration of CeO2 NPs that stimulates cell growth in natural tissues and accelerates the healing process is challenging and requires precise control of the surface chemistry, particle size, physicochemical properties, route of administration, and synthesis method of CeO2 NPs. The identification of the optimal concentration is similar to its consideration of the factors influencing the toxicity of CeO2 NPs. In terms of morphology, different morphologies have different toxicities; Ji et al. observed that CeO2 NPs (ranging in length from a few hundred nanometers to a few micrometers) induced a gradual increase in IL-1β production by producing lysosomal damage, whereas CeO2 nanospheres and shorter nanorods did not show significant toxicity (Ji et al., 2012). The time and route of exposure play an important role in the toxicity of CeO2 NPs, as shown by Carlander et al., who administered CeO2 NPs of different sizes, coatings, and dosages to rats via different routes of exposure, which showed that the biokinetics of CeO2 NPs are not only dependent on the properties of the NPs (size and coatings), but also on the conditions of the exposure (routes and dosages) (Carlander et al., 2018). Mackevica et al. found that extrapolations of results obtained under high exposure conditions may not be applicable to real-world environmental conditions, as higher NP concentrations may lead to increased NP-to-cell ratios, which can cause testing errors (Mackevica et al., 2023). Surface modifiers can also affect NPs, Fisichella et al. found that uncoated CeO2 NPs downregulated key genes involved in metabolic activity, whereas ammonium citrate-coated CeO2 NPs did not show any detrimental effects at the same concentration (Fisichella et al., 2014). From a cost consideration, CeO2 NPs, as a rare earth oxide metal, are expensive and costly to use compared to other wound healing materials and do not offer a price advantage, which economically hinders the possibility of nanomedicine becoming a reality. From a standardization point of view, CeO2 NPs research lacks uniform standards and there may be differences between studies that make it difficult to make comparisons. Cerium dioxide nanoparticles have similar safety concerns as other insoluble nanomaterials. For example, the incorporation of CeO2 into polymer matrices such as 3D scaffolds may improve biocompatibility, but may also reduce therapeutic efficacy (Singh et al., 2021; He et al., 2023). Therefore, harmonization of standards will help to improve the potential and safety of cerium oxide nanoparticles. A major drawback from intellectual and technical considerations is that the safety of nanomaterials is still a widely debated topic. Currently, cerium oxide nanoparticles have shown excellent wound healing ability in animal experiments. For example, animal experiments conducted by Zhao R et al. on a rat whole skin wound model showed that alginate hydrogel-based wound dressings were effective in accelerating the wound healing process. This study demonstrated the safety and reliability of CeO2 NPs (Zhao et al., 2023). However, despite the medical advantages of nanomaterials and the impressive research results, few nanomaterials can be used in clinical applications. Clinical trials related to cerium oxide nanoparticles are still blank. There is also a need to understand the precise evolution and biodistribution (ADME characterization) of NPs in the human body if they are to be used safely and effectively. In this context, the development of reproducible and reliable analytical methods for the dynamic characterization of the evolution of nanomaterials in biological environments is considered to be an important way to perform nanosafety studies. Therefore, for the future development of cerium oxide nanoparticles, in addition to innovations in animal experiments, more breakthroughs in human trials are needed in the future to better demonstrate the wound healing ability of cerium oxide nanoparticles.
7.2 Potential effects of cerium oxide nanoparticle composites
Difficulties arise in the use of single nanoparticles for wound healing, so investigating composite biomaterials for wound healing and addressing the challenges encountered with individual nanomaterials in the healing process could elucidate their unique advantages in promoting wound repair (Veith et al., 2019; Zeng et al., 2022). Efforts have been made to improve the effectiveness of CeO2 NPs at various stages of the wound healing process. Cheng et al. investigated and designed cerium-nitrogen-phosphorus-striped peelable graphene nanocomposites (abbreviated as ACGNCs) to enhance wound healing by promoting the inflammatory and proliferative phases. The ACGNCs exerted the following roles to promote wound healing: (1) in the inflammatory phase, the pH value was still acidic (∼6. 0), ACG NCs received white light irradiation and produced large amounts of ROS to scavenge bacteria due to the effective separation of electron-hole space; (2) as the wound healing process proceeded to the proliferative phase, the pH was naturally elevated at a neutral level, and the folded single-stranded DNA variant of i-motif DNA was transformed into an unfolded form, which facilitated the opening of the pore of the hollow cerium dioxide NPs to release parameters that It can be decomposed into urea with the help of arginase overexpression site in the wound; (3) the isolated cerium dioxide NPs can freely enter into the fibroblasts, thus destroying the intracellular ROS and promoting cell proliferation, and the stealth peptide prevents the graphene from being absorbed by the macrophage, prolongs the retention time of graphene as a scaffold in the wound site, and thus promotes the migration of fibroblasts. Finally, during the remodeling phase, MMP, which is highly expressed in the extracellular matrix, cleaves the GPLGLAG peptide and promotes the internalization of graphene by macrophages through endocytosis, which leads to the biodegradation of ACG nc at the wound site (Cheng et al., 2019). In the study of Ma et al. hollow CeO2 NPs with porous shells and rough surfaces were synthesized and L-arginine was added to them to promote various stages of wound healing. In the hemostatic stage, the modified CeO2 NPs acted as nanobridges within the tissue to achieve rapid hemostasis. In the inflammatory stage, these nanoparticles generate ROS under simulated sunlight irradiation to eliminate bacteria and prevent infection. In the proliferative phase, modified CeO2 NPs can scavenge excess ROS generated at the wound site due to SOD and catalase activities. In addition, the released L-arginine can be converted to nitric oxide in macrophages, thus promoting cell proliferation (Ma et al., 2019).
Secondly, it was also shown that combining CeO2 with polymers such as polycaprolactone (PCL), polyvinyl alcohol and chitosan does not affect the catalytic ability of enzymes. These composites have potential applications in wound healing, as confirmed by intracellular experiments. Based on the enzyme-catalyzing ability of CeO2, dressings with antimicrobial, anti-inflammatory and antioxidant properties have been developed. For example, when tested by Plocon C et al. using human osteoblasts, the composite scaffolds showed very good biocompatibility, with samples consisting of polymers and cerium-doped calcium phosphate responding better (Plocon et al., 2023). Sanmugam A et al. found that the fabricated scaffolds completely facilitated the complete closure of the wounds after 14 days, which suggests that the developed scaffolds have high swelling, good degradability and permeability, which facilitated the absorption of wound exudates, thus consolidating the healing effect (Sanmugam et al., 2024). Kamalipooya S et al. found that the nanocomposite with 0.1% CeO2-CSNPs exhibited high antibacterial performance against S. aureus (<58.59 μg/mL). The results of this research suggest that PCL/CA nanofiber mats functionalized with CeO2-CSNPs have the potential to be highly effective in treating diabetes-related wounds. The use of CeO2 NPs in diabetic wound repair substances has shown promising results (Kamalipooya et al., 2024). The popularity of combining nanoparticles with hydrogels is due to the highwater retention capacity of hydrogels, which provides a favorable microenvironment for cell proliferation, enabling the loading of bioactives and providing a soft texture and excellent wound healing properties. Hydrogels (including chitosan hydrogels, alginate hydrogels (Raja and Fathima, 2018) and silk-based hydrogels (Augustine et al., 2021)) have favorable physical properties and chemical structures. Numerous studies have shown significant progress in the utilization of hydrogels for sutureless wound closure, antimicrobial, hemostasis and angiogenesis. Hydrogels based on natural polymers have great potential for cell proliferation due to the presence of biomolecules and peptides, in addition to their general advantages. ZC-QPP hydrogels provide an avenue for the development of a multifunctional synergistic therapeutic platform that combines enzyme nanomaterials with hydrogels to synergize antimicrobial and antioxidant properties and promote wound healing (Zhang et al., 2023). CeO2 NPs contain Ce3+ ions and produce oxygen vacancies. The Ce3+ ions on the surface are the site of catalytic reactions, while the generated oxygen vacancies contribute to the conversion and migration of active substances in the system. All these factors will be favorable for biosensors (Soni et al., 2018; Domínguez-Aragón et al., 2021). The redox activity of cerium oxide can be used as a sensing platform to generate color in the presence of oxidative enzymes acting on the corresponding substrates to produce H2O2. CeO2 particles can be used both as a high specific surface area support for immobilizing highly loaded enzymes and as a reagent for generating color (Karimi et al., 2016).
In summary, CeO2 NPs, both naked and functionalized, show excellent ability to accelerate acute and chronic wound healing. CeO2 NPs can be used in wound dressings, drug delivery systems and biomedical nanotechnology to promote wound healing. They have antioxidant and antimicrobial properties that help reduce the risk of infection and promote tissue regeneration and repair. In addition, CeO2 NPs can be used as drug carriers to control the release of drugs to promote wound healing. They can also be used to fabricate biosensors to monitor biomarkers and inflammatory responses during wound healing for real-time diagnosis and therapeutic feedback (Sadidi et al., 2020; Xue et al., 2024). In addition to their wide range of applications in wound healing, CeO2 NPs are mainly used in the treatment of skin, cardiac, neurological and ophthalmic tissues. Other soft tissues may also be evaluated in future experimental studies. Thin films, hydrogels and nanofiber scaffolds containing CeO2 NPs have been shown to be highly suitable alternatives with satisfactory results in the repair and regeneration of soft tissue injuries and defects. Mesenchymal stem cell-derived extracellular vesicles (EVs) carried with CeO2 NPs shows promising in the field of regenerative medicine as they have a powerful role in promoting tissue repair and regeneration (Sadidi et al., 2020). However, the fragile lipid membrane limits their function in oxidative stress microenvironments. Cerium nanocerium is an antioxidant nanoenzymes; herein, we reveal that cerium nanocerium-loaded EVs extracted from MSCs promote skin wound healing in aged mice. Gao L et al. further demonstrated that the DG-CeO2 EVsHyp are biocompatible and have antioxidant and pro-angiogenic effects during skin wound healing in both young and aged mice (Gao et al., 2023). Yildizbakan L et al. found that synthetic chitosan-cerium oxide porous scaffolds are an effective solution to complications associated with bone injury by promoting tissue regeneration and reducing the risk of infection. All scaffold variants inhibited the bacterial growth of Staphylococcus aureus and Escherichia coli strains (Yildizbakan et al., 2023).
7.3 Potential of CeO2 NPs for interdisciplinary applications
We are looking forward to the interdisciplinary field of CeO2 NPs, which is widely used as a nanomaterial in wound dressings or other biomedical applications, a novel approach that has revolutionized the therapeutic regimen and management of wound healing. The unrivaled advantages of CeO2 NPs in wound care compared with other materials mainly include (1) high reactivity and large specific surface area with higher catalytic and gas production/loading efficiencies, and (2) control of the conductivity and penetration depth of the nanomaterials by changing the nanomaterials’ size and shape. However, the use of nanomaterials for wound healing is far from commercialization and clinical application. Major obstacles include: (1) nanomaterials have toxic effects, mainly in the form of oxidative stress and inflammation in cells, and in many cases, it is difficult to gather sufficient information about the expected behavior and toxicity of nanosystems in the human body; (2) nanomaterials have a single function in wound healing and prevention of infections, and insufficient ability in hemostasis, keeping wounds moist, isolating wounds, and promoting wound healing; (3) Nanomaterials are mainly injected into wounds in situ, producing liquids that are not only easy to spill out of wounds, but also pose challenges to clinical care.
In order to accelerate the adoption of cerium oxide nanomaterial-based wound care in clinical settings, there is a need to develop more degradable and absorbable green nanomaterials. In addition, there is a need to extend the functionality of nanomaterials by using gauze and hydrogels as carriers. This combination approach not only prevents acute toxicity caused by direct contact between nanomaterials and wounds, but also greatly expands the range of applications of nanomaterials in wound care, such as hemostasis, moisturization, and isolation from the external environment. And researchers have made several efforts to develop smart dressings with real-time monitoring and diagnostic capabilities, including detection and monitoring of wound-related bacteria, pH, temperature, movement, physiological signals, glucose, ROS, and uric acid. An example is the Bacterial Response Therapy Dressing. Early detection of wound infections will provide substantial benefits to physicians and patients by providing effective interventions. pH monitoring therapeutic dressing. pH is an important indicator of wound status as it is closely related to many physiologic processes including bacterial infection, angiogenesis and collagen formation. Temperature monitoring of therapeutic dressings. This is because it affects a range of chemical and enzymatic actions. Movement and physiologic signals monitor the therapeutic dressing. Because frequent movement at the wound site, compression and stretching of the wound can affect the growth of skin and muscle tissue, which can severely impact wound healing.
8 Conclusion
This article provides a review of the distinctive chemical structure and physicochemical properties of CeO2 NPs, as well as the recent progress in their application for wound healing. Furthermore, it discusses the growing significance of employing green synthesis methods for CeO2 NPs in wound healing applications. Effective and precise control over the surface chemical structure, particle size, and physicochemical properties of CeO2 NPs is necessary. Moreover, there have been recent advancements in utilizing CeO2 NPs in combination with other substances for wound healing. For example, precise control over the rate of drug release and dosage can lead to targeted therapy and enhanced efficacy. The feasibility and scientific validity of CeO2 NPs in clinical applications are still under investigation. In the future, it is expected that CeO2 will overcome additional challenges and thrive in interdisciplinary nanomedicine, thereby promoting its application in skin wound healing.
Author contributions
SC: Writing–original draft, Writing–review and editing. YW: Writing–original draft, Writing–review and editing. SB: Writing–original draft. LY: Writing–original draft. XF: Writing–original draft. YY: Writing–original draft. HL: Writing–original draft. HP: Writing–review and editing. SG: Writing–review and editing. HZ: Writing–review and editing. PZ: Writing–original draft. YZ: Writing–original draft, Writing–review and editing.
Funding
The author(s) declare that financial support was received for the research, authorship, and/or publication of this article. This work was supported by Sichuan Provincial Medical Research Project Plan (No. S21004), Gulin County People’s Hospital-Affiliated Hospital of Southwest Medical University Science and Technology Strategic Cooperation Program (No. 2022GLXNYDFY05), Key-funded Project of the National College Student Innovation and Entrepreneurship Training Program (No. 202310632001), Luzhou Science and Technology Program (No. 2021-JYJ-102), Luzhou Municipal Science and Technology Project (No. 2022-SYF-98), Sichuan Provincial Medical Research Youth Innovation Project (No.Q17080), National College Student Innovation and Entrepreneurship Training Program (No. 202310632028), National College Student Innovation and Entrepreneurship Training Program (No. 202310632036) and National College Student Innovation and Entrepreneurship Training Program (No. 202310632093), The Sichuan Association of Healthcare and Health Promotion Scientific Research Project (KY2023SJ0059), Sichuan Science and Technology Program (2022YFS0611).
Acknowledgments
All figures created with biorender.com.
Conflict of interest
The authors declare that the research was conducted in the absence of any commercial or financial relationships that could be construed as a potential conflict of interest.
Publisher’s note
All claims expressed in this article are solely those of the authors and do not necessarily represent those of their affiliated organizations, or those of the publisher, the editors and the reviewers. Any product that may be evaluated in this article, or claim that may be made by its manufacturer, is not guaranteed or endorsed by the publisher.
References
Aalapati, S., Ganapathy, S., Manapuram, S., Anumolu, G., and Prakya, B. M. (2014). Toxicity and bio-accumulation of inhaled cerium oxide nanoparticles in CD1 mice. Nanotoxicology 8, 786–798. doi:10.3109/17435390.2013.829877
Abdal Dayem, A., Hossain, M. K., Lee, S. B., Kim, K., Saha, S. K., Yang, G.-M., et al. (2017). The role of reactive oxygen species (ROS) in the biological activities of metallic nanoparticles. Int. J. Mol. Sci. 18, 120. doi:10.3390/ijms18010120
Ahmad, A., Javed, M. S., Khan, S., Almutairi, T. M., Mohammed, A. A. A., and Luque, R. (2023). Green synthesized Ag decoratedCeO2 nanoparticles: efficient photocatalysts and potential antibacterial agents. Chemosphere 310, 136841. doi:10.1016/j.chemosphere.2022.136841
Augustine, R., Hasan, A., Patan, N. K., Dalvi, Y. B., Varghese, R., Antony, A., et al. (2020). Cerium oxide nanoparticle incorporated electrospun poly(3-hydroxybutyrate-Co-3-Hydroxyvalerate) membranes for diabetic wound healing applications. ACS Biomater. Sci. Eng. 6, 58–70. doi:10.1021/acsbiomaterials.8b01352
Augustine, R., Zahid, A. A., Hasan, A., Dalvi, Y. B., and Jacob, J. (2021). Cerium oxide nanoparticle-loaded gelatin methacryloyl hydrogel wound-healing patch with free radical scavenging activity. ACS Biomater. Sci. Eng. 7, 279–290. doi:10.1021/acsbiomaterials.0c01138
Babenko, L. P., Zholobak, N. M., Shcherbakov, A. B., Voychuk, S. I., Lazarenko, L. M., and Spivak, M. Y. (2012). Antibacterial activity of cerium colloids against opportunistic microorganisms in vitro. Mikrobiol. Z 74, 54–62.
Bai, Q., Han, K., Dong, K., Zheng, C., Zhang, Y., Long, Q., et al. (2020). Potential applications of nanomaterials and technology for diabetic wound healing. Int. J. Nanomedicine 15, 9717–9743. doi:10.2147/ijn.s276001
Bai, Y., Li, Y., Li, Y., and Tian, L. (2024). Advanced biological applications of cerium oxide nanozymes in disease related to oxidative damage. ACS Omega 9 (8), 8601–8614. doi:10.1021/acsomega.3c03661
Baptista-Silva, S., Borges, S., Costa-Pinto, A. R., Costa, R., Amorim, M., Dias, J. R., et al. (2021). In situ forming silk sericin-based hydrogel: a novel wound healing biomaterial. ACS Biomater. Sci. Eng. 7 (4), 1573–1586. doi:10.1021/acsbiomaterials.0c01745
Barbu, A., Neamtu, B., Zăhan, M., Iancu, G. M., Bacila, C., and Mireșan, V. (2021). Current trends in advanced alginate-based wound dressings for chronic wounds. J. Pers. Med. 11 (9), 890. doi:10.3390/jpm11090890
Betz, C., Lenard, A., Belting, H.-G., and Affolter, M. (2016). Cell behaviors and dynamics during angiogenesis. Development 143, 2249–2260. doi:10.1242/dev.135616
Broughton, G., Janis, J. E., and Attinger, C. E. (2006). The basic science of wound healing. Plast. Reconstr. Surg. 117 (7 Suppl. l), 12S–34S. doi:10.1097/01.prs.0000225430.42531.c2
Burello, E., and Worth, A. P. (2011). A theoretical framework for predicting the oxidative stress potential of oxide nanoparticles. Nanotoxicology 5, 228–235. doi:10.3109/17435390.2010.502980
Burgess, J. L., Wyant, W. A., Abdo Abujamra, B., Kirsner, R. S., and Jozic, I. (2021). Diabetic wound-healing science. Medicina 57 (10), 1072. doi:10.3390/medicina57101072
Cañedo-Dorantes, L., and Cañedo-Ayala, M. (2019). Skin acute wound healing: a comprehensive review. Int. J. Inflam. 2019, doi:10.1155/2019/3706315
Carlander, U., Moto, T. P., Desalegn, A. A., Yokel, R. A., and Johanson, G. (2018). Physiologically based pharmacokinetic modeling of nanoceria systemic distribution in rats suggests dose- and route-dependent biokinetics. Int. J. Nanomedicine 13, 2631–2646. doi:10.2147/ijn.s157210
Carvajal, S., Perramón, M., Casals, G., Oró, D., Ribera, J., Morales-Ruiz, M., et al. (2019). Cerium oxide nanoparticles protect against oxidant injury and interfere with oxidative mediated kinase signaling in human-derived hepatocytes. Int. J. Mol. Sci. 20 (23), 5959. doi:10.3390/ijms20235959
Castano, C. E., O’Keefe, M. J., and Fahrenholtz, W. G. (2015). Cerium-based oxide coatings. Curr. Opin. Solid State Mater Sci. 19 (2), 69–76. doi:10.1016/j.cossms.2014.11.005
Celardo, I., De Nicola, M., Mandoli, C., Pedersen, J. Z., Traversa, E., and Ghibelli, L. (2011a). Ce³+ ions determine redox-dependent anti-apoptotic effect of cerium oxide nanoparticles. ACS Nano 5 (6), 4537–4549. doi:10.1021/nn200126a
Celardo, I., De Nicola, M., Mandoli, C., Pedersen, J. Z., Traversa, E., and Ghibelli, L. (2011b). Ce3+ ions determine redox-dependent anti-apoptotic effect of cerium oxide nanoparticles. ACS Nano 5, 4537–4549. doi:10.1021/nn200126a
Charbgoo, F., Ahmad, M. B., and Darroudi, M. (2017a). Cerium oxide nanoparticles: green synthesis and biological applications. Int. J. Nanomedicine 12, 1401–1413. doi:10.2147/ijn.s124855
Charbgoo, F., Ahmad, M. B., and Darroudi, M. (2017b). Cerium oxide nanoparticles: green synthesis and biological applications. Int. J. Nanomed. 12, 1401–1413. doi:10.2147/ijn.s124855
Chen, L., Deng, H., Cui, H., Fang, J., Zuo, Z., Deng, J., et al. (2017). Inflammatory responses and inflammation-associated diseases in organs. Oncotarget 9, 7204–7218. doi:10.18632/oncotarget.23208
Chen, Y. H., Rao, Z. F., Liu, Y. J., Liu, X. S., Liu, Y. F., Xu, L. J., et al. (2021). Multifunctional injectable hydrogel loaded with cerium-containing bioactive glass nanoparticles for diabetic wound healing. Biomolecules 11 (5), 702. doi:10.3390/biom11050702
Cheng, H., Shi, Z., Yue, K., Huang, X., Xu, Y., Gao, C., et al. (2021a). Sprayable hydrogel dressing accelerates wound healing with combined reactive oxygen species-scavenging and antibacterial abilitiesActa. Biomater 124, 219–232. doi:10.1016/j.actbio.2021.02.002
Cheng, H., Shi, Z., Yue, K., Huang, X., Xu, Y., Gao, C., et al. (2021b). Sprayable hydrogel dressing accelerates wound healing with combined reactive oxygen species-scavenging and antibacterial abilities. Acta Biomater. 124, 219–232. doi:10.1016/j.actbio.2021.02.002
Cheng, Y., Chang, Y., Feng, Y., Jian, H., Wu, X., Zheng, R., et al. (2019). Hierarchical acceleration of wound healing through intelligent nanosystem to promote multiple stages. ACS Appl. Mater Interfaces 11 (37), 33725–33733. doi:10.1021/acsami.9b13267
Chigurupati, S., Mughal, M. R., Okun, E., Das, S., Kumar, A., McCaffery, M., et al. (2013). Effects of cerium oxide nanoparticles on the growth of keratinocytes, fibroblasts and vascular endothelial cells in cutaneous wound healing. Biomaterials 34 (9), 2194–2201. doi:10.1016/j.biomaterials.2012.11.061
Clark, A., Zhu, A., Sun, K., and Petty, H. R. (2011). Cerium oxide and platinum nanoparticles protect cells from oxidant-mediated apoptosis. J. Nanoparticle Res. 13, 5547. doi:10.1007/s11051-011-0544-3
Comino-Sanz, I. M., López-Franco, M. D., Castro, B., and Pancorbo-Hidalgo, P. L. (2021). The role of antioxidants on wound healing: a review of the current evidence. J. Clin. Med. 10 (16), 3558
Wang, Y., Chen, S., Bao, S., Yao, L., Wen, Z., Xu, L., et al. (2024). Deciphering the fibrotic process: mechanism of chronic radiation skin injury fibrosis. Front. immunol. 15, 1338922
Dahle, J. T., and Arai, Y. (2015). Environmental geochemistry of cerium: applications and toxicology of cerium oxide nanoparticles. Int. J. Environ. Res. Public Health 12 (2), 1253–1278. doi:10.3390/ijerph120201253
Damle, M. A., Jakhade, A. P., and Chikate, R. C. (2019). Modulating pro- and antioxidant activities of nanoengineered cerium dioxide nanoparticles against Escherichia coli. ACS Omega 4, 3761–3771. doi:10.1021/acsomega.8b03109
Darby, I. A., Laverdet, B., Bonté, F., and Desmoulière, A. (2014). Fibroblasts and myofibroblasts in wound healing. Clin. Cosmet. Investig. Dermatol. 7, 301–311. doi:10.2147/CCID.S50046
Darroudi, M., Sarani, M., Oskuee, R. K., Zak, A. K., and Amiri, M. S. (2014b). Nanoceria: gum mediated synthesis and in vitro viability assay. Ceram. Int. 40, 2863–2868. doi:10.1016/j.ceramint.2013.10.026
Darroudi, M., Sarani, M., Oskuee, R. K., Zak, A. K., Hosseini, H. A., and Gholami, L. (2014a). Green synthesis and evaluation of metabolic activity of starch mediated nanoceria. Ceram. Int. 40, 2041–2045. doi:10.1016/j.ceramint.2013.07.116
Das, M., Patil, S., Bhargava, N., Kang, J. F., Riedel, L. M., Seal, S., et al. (2007). Auto-catalytic ceria nanoparticles offer neuroprotection to adult rat spinal cord neurons. Biomaterials 28, 1918–1925. doi:10.1016/j.biomaterials.2006.11.036
Das, S., Chigurupati, S., Dowding, J., Munusamy, P., Baer, D. R., McGinnis, J. F., et al. (2014). Therapeutic potential of nanoceria in regenerative medicine. MRS Bull. 39 (11), 976–983. doi:10.1557/mrs.2014.221
Das, S., Singh, S., Dowding, J. M., Oommen, S., Kumar, A., Sayle, T. X., et al. (2012b). The induction of angiogenesis by cerium oxide nanoparticles through the modulation of oxygen in intracellular environments. Biomaterials 33, 7746–7755. doi:10.1016/j.biomaterials.2012.07.019
Das, S., Singh, S., Dowding, J. M., Oommen, S., Kumar, A., Sayle, T. X., et al. (2012a). The induction of angiogenesis by cerium oxide nanoparticles through the modulation of oxygen in intracellular environments. Biomaterials 33 (31), 7746–7755. doi:10.1016/j.biomaterials.2012.07.019
Datta, A., Mishra, S., Manna, K., Saha, K. D., Mukherjee, S., and Roy, S. (2020). Pro-oxidant therapeutic activities of cerium oxide nanoparticles in colorectal carcinoma cells. ACS Omega 5, 9714–9723. doi:10.1021/acsomega.9b04006
Del Turco, S., Ciofani, G., Cappello, V., Parlanti, P., Gemmi, M., Caselli, C., et al. (2019). Effects of cerium oxide nanoparticles on hemostasis: coagulation, platelets, and vascular endothelial cells. J. Biomed. Mater Res. A 107 (7), 1551–1562. doi:10.1002/jbm.a.36669
de Oliveira, R. C., Amoresi, R. A. C., Marama, N. L., Zaghete, M. A., Chiquito, A. J., Sambrano, J. R., et al. (2020). Influence of synthesis time on the morphology and properties of CeO2 nanoparticles. An experimental—theoretical study. Cryst. Growth Des. 20, 5031–5042. doi:10.1021/acs.cgd.0c00165
de Oliveira Gonzalez, A. C., Costa, T. F., de Araújo Andrade, Z., and Medrado, A. R. A. P. (2016). Wound healing—a literature review. An. Bras. Dermatol. 91, 614–620. doi:10.1590/abd1806-4841.20164741
Descamps, B., and Emanueli, C. (2012). Vascular differentiation from embryonic stem cells: novel technologies and therapeutic promises. Vasc. Pharmacol. 56, 267–279. doi:10.1016/j.vph.2012.03.007
Dewberry, L. C., Niemiec, S. M., Hilton, S. A., Louiselle, A. E., Singh, S., Sakthivel, T. S., et al. (2022). Cerium oxide nanoparticle conjugation to microRNA-146a mechanism of correction for impaired diabetic wound healing. Nanomedicine 40, 102483. doi:10.1016/j.nano.2021.102483
Di Pietro, L. A. (2013). Angiogenesis and scar formation in healing wounds. Curr. Opin. Rheumatol. 25, 87–91. doi:10.1097/bor.0b013e32835b13b6
Domínguez-Aragón, A., Dominguez, R. B., and Zaragoza-Contreras, E. A. (2021). Simultaneous detection of dihydroxybenzene isomers using electrochemically reduced graphene oxide-carboxylated carbon nanotubes/gold nanoparticles nanocomposite. Biosensors 11, 321. doi:10.3390/bios11090321
Dutta, D., Mukherjee, R., Ghosh, S., Patra, M., Mukherjee, M., and Basu, T. (2022). Cerium oxide nanoparticles as antioxidant or pro-oxidant agents. ACS Appl. Nano Mat. 5, 1690–1701. doi:10.1021/acsanm.1c04518
Ellis, S., Lin, E. J., and Tartar, D. (2018). Immunology of wound healing. Curr. Dermatol. Rep. 7, 350–358. doi:10.1007/s13671-018-0234-9
Eriksson, P., Tal, A. A., Skallberg, A., Brommesson, C., Hu, Z., Boyd, R. D., et al. (2018b). Cerium oxide nanoparticles with antioxidant capabilities and gadolinium integration for MRI contrast enhancement. Sci. Rep. 8, 6999. doi:10.1038/s41598-018-25390-z
Eriksson, P., Tal, A. A., Skallberg, A., et al. (2018a). Cerium oxide nanoparticles with antioxidant capabilities and gadolinium integration for MRI contrast enhancement. Sci. Rep. 8 (1), 6999. doi:10.1038/s41598-018-25390-z
Ezhilarasu, H., Vishalli, D., Dheen, S. T., Bay, B. H., and Srinivasan, D. K. (2020). Nanoparticle-based therapeutic approach for diabetic wound healing. Nanomaterials 10, 1234. doi:10.3390/nano10061234
Fisichella, M., Berenguer, F., Steinmetz, G., Auffan, M., Rose, J., and Prat, O. (2014). Toxicity evaluation of manufactured CeO2 nanoparticles before and after alteration: combined physicochemical and whole-genome expression analysis in Caco-2 cells. BMC Genomics 15 (1), 700. doi:10.1186/1471-2164-15-700
Futosi, K., Fodor, S., and Mócsai, A. (2013). Neutrophil cell surface receptors and their intracellular signal transduction pathways. Int. Immunopharmacol. 17, 638–650. doi:10.1016/j.intimp.2013.06.034
Gabbiani, G. (2003). The myofibroblast in wound healing and fibrocontractive diseases. J. Pathol. 200, 500–503. doi:10.1002/path.1427
Gagnon, J., and Fromm, K. (2015). Toxicity and protective effects of cerium oxide nanoparticles (nanoceria) depending on their preparation method, particle size, cell type, and exposure route. Eur. J. Inorg. Chem. 2015, 4510–4517. doi:10.1002/ejic.201500643
Gao, L., Feng, Q., Cui, B., Mao, Y., Zhao, Z., Liu, Z., et al. (2023). Loading nanoceria improves extracellular vesicle membrane integrity and therapy to wounds in aged mice. ACS Biomater. Sci. Eng. 9 (2), 732–742. doi:10.1021/acsbiomaterials.2c01104
Ghahramani, Z., Arabi, A. M., Shafiee Afarani, M., and Mahdavian, M. (2020). Solution combustion synthesis of cerium oxide nanoparticles as corrosion inhibitor. Int. J. Appl. Ceram. Technol. 17 (3), 1514–1521. doi:10.1111/ijac.13365
Gojova, A., Lee, J. T., Jung, H. S., Guo, B., Barakat, A. I., and Kennedy, I. M. (2009). Effect of cerium oxide nanoparticles on inflammation in vascular endothelial cells. Inhal. Toxicol. 21 (Suppl. 1), 123–130. doi:10.1080/08958370902942582
Gopinath, K., Karthika, V., Sundaravadivelan, C., Gowri, S., and Arumugam, A. (2015). Mycogenesis of cerium oxide nanoparticles using Aspergillus Niger culture filtrate and their applications for antibacterial and larvicidal activities. J. Nanostruct. Chem. 5, 295–303. doi:10.1007/s40097-015-0161-2
Guo, S., and DiPietro, L. A. (2010). Factors affecting wound healing. J. Dent. Res. 89, 219–229. doi:10.1177/0022034509359125
He, S., Li, Z., Wang, L., Yao, N., Wen, H., Yuan, H., et al. (2024). A nanoenzyme-modified hydrogel targets macrophage reprogramming-angiogenesis crosstalk to boost diabetic wound repair. Bioact. Mater 35, 17–30. doi:10.1016/j.bioactmat.2024.01.005
He, S., Wu, H., Huang, J., Li, Q., Huang, Z., Wen, H., et al. (2023). 3-D tissue-engineered epidermis against human primary keratinocytes apoptosis via relieving mitochondrial oxidative stress in wound healing. J. Tissue Eng. 14, 20417314231163168. doi:10.1177/20417314231163168
Hinz, B., and Gabbiani, G. (2003). Cell-matrix and cell-cell contacts of myofibroblasts: role in connective tissue remodeling. Thromb. Haemost. 90 (6), 993–1002. doi:10.1160/TH03-05-0328
Hirst, S. M., Karakoti, A. S., Tyler, R. D., Sriranganathan, N., Seal, S., and Reilly, C. M. (2009a). Anti-inflammatory properties of cerium oxide nanoparticles. Small 5 (24), 2848–2856. doi:10.1002/smll.200901048
Hirst, S. M., Karakoti, A. S., Tyler, R. D., Sriranganathan, N., Seal, S., and Reilly, C. M. (2009b). Anti-inflammatory properties of cerium oxide nanoparticles. Small 5, 2848–2856. doi:10.1002/smll.200901048
Hosseini, M., Amjadi, I., Mohajeri, M., and Mozafari, M. (2020). Sol-gel synthesis, physico-chemical and biological characterization of cerium oxide/polyallylamine nanoparticles. Polym. (Basel) 12 (7), 1444. doi:10.3390/polym12071444
Hu, Y., Du, Y., Jiang, H., and Jiang, G.-S. (2014). Cerium promotes bone marrow stromal cells migration and osteogenic differentiation via smad1/5/8 signaling pathway. Int. J. Clin. Exp. Pathol. 7 (8), 5369–5378.
Huang, J., Yu, Y., Zhu, J., and Yu, R. (2018). Oxygen adatoms and vacancies on the (110) surface of CeO2. Sci. China Technol. Sci. 61 (1), 135–139. doi:10.1007/s11431-017-9154-9
Ighodaro, O. M., and Akinloye, O. A. (2018). First line defence antioxidants-superoxide dismutase (SOD), catalase (CAT) and glutathione peroxidase (GPX): their fundamental role in the entire antioxidant defence grid. Alex. J. Med. 54, 287–293. doi:10.1016/j.ajme.2017.09.001
Ilina, O., and Friedl, P. (2009). Mechanisms of collective cell migration at a glance. J. Cell Sci. 122, 3203–3208. doi:10.1242/jcs.036525
Ji, Z., Wang, X., Zhang, H., Lin, S., Meng, H., Sun, B., et al. (2012). Designed synthesis of CeO2 nanorods and nanowires for studying toxicological effects of high aspect ratio nanomaterials. ACS Nano 6 (6), 5366–5380. doi:10.1021/nn3012114
Johnson, K. E., and Wilgus, T. A. (2014). Vascular endothelial growth factor and angiogenesis in the regulation of cutaneous wound repair. Adv. Wound Care 3, 647–661. doi:10.1089/wound.2013.0517
Kaarniranta, K., Pawlowska, E., Szczepanska, J., Jablkowska, A., and Blasiak, J. (2019). Role of mitochondrial DNA damage in ROS-mediated pathogenesis of age-related macular degeneration (AMD). Int. J. Mol. Sci. 20, 2374. doi:10.3390/ijms20102374
Kamalipooya, S., Fahimirad, S., Abtahi, H., Golmohammadi, M., Satari, M., Dadashpour, M., et al. (2024). Diabetic wound healing function of PCL/cellulose acetate nanofiber engineered with chitosan/cerium oxide nanoparticles. Int. J. Pharm. 653, 123880. doi:10.1016/j.ijpharm.2024.123880
Kargar, H., Ghazavi, H., and Darroudi, M. (2015). Size-controlled and bio-directed synthesis of ceria nanopowders and their in vitro cytotoxicity effects. Ceram. Int. 41, 4123–4128. doi:10.1016/j.ceramint.2014.11.108
Kargozar, S., Baino, F., Hoseini, S. J., Hamzehlou, S., Darroudi, M., Verdi, J., et al. (2018). Biomedical applications of nanoceria: new roles for an old player. Nanomedicine 13 (23), 3051–3069. doi:10.2217/nnm-2018-0189
Karimi, A., Othman, A., and Andreescu, S. (2016). Portable enzyme-paper biosensors based on redox-active CeO2 nanoparticles. Methods Enzymol. 571, 177–195. doi:10.1016/bs.mie.2016.03.006
Khurana, A., Tekula, S., and Godugu, C. (2018). Nanoceria suppresses multiple low doses of streptozotocin-induced Type 1 diabetes by inhibition of Nrf2/NF-κB pathway and reduction of apoptosis. Nanomedicine 13, 1905–1922. doi:10.2217/nnm-2018-0085
Koh, T. J., and DiPietro, L. A. (2011). Inflammation and wound healing: the role of the macrophage. Expert Rev. Mol. Med. 13, e23. doi:10.1017/s1462399411001943
Kyosseva, S. V., Chen, L., Seal, S., and McGinnis, J. F. (2013). Nanoceria inhibit expression of genes associated with inflammation and angiogenesis in the retina of Vldlr null mice. Exp. Eye Res. 116, 63–74. doi:10.1016/j.exer.2013.08.003
Li, H. R., Xia, P., and Pan, S. (2020).The advances of ceria nanoparticles for biomedical applications in orthopaedics.Int. J. Nanomed 15, 7199–7214. doi:10.2147/ijn.s270229
Li, J., Wang, X., Yao, Z., Yuan, F., Liu, H., Sun, Z., et al. (2023). NLRP3-Dependent crosstalk between pyroptotic macrophage and senescent cell orchestrates trauma-induced heterotopic ossification during aberrant wound healing. Adv. Sci. (Weinh) 10 (19), e2207383. doi:10.1002/advs.202207383
Li, J. H., Wen, J., Li, B., Li, W., Qiao, W., Shen, J., et al. (2018). Valence state manipulation of cerium oxide nanoparticles on a titanium surface for modulating cell fate and bone formation. Adv. Sci(Weinh) 5 (2), 1700678. doi:10.1002/advs.201700678
Li, S., Renick, P., Senkowsky, J., Nair, A., and Tang, L. (2021). Diagnostics for wound infections. Adv. Wound Care (New Rochelle). 10 (6), 317–327. doi:10.1089/wound.2019.1103
Li, Y., Zhang, W., Niu, J., and Chen, Y. (2012). Mechanism of photogenerated reactive oxygen species and correlation with the antibacterial properties of engineered metal-oxide nanoparticles. ACS Nano 6, 5164–5173. doi:10.1021/nn300934k
Lin, P. H., Sermersheim, M., Li, H., Lee, P. H. U., Steinberg, S. M., and Ma, J. (2017). Zinc in wound healing modulation. Nutrients 10 (1), 16. doi:10.3390/nu10010016
Lindholm, C., and Searle, R. (2016). Wound management for the 21st century: combining effectiveness and efficiency. Int. Wound J. 13, 5–15. doi:10.1111/iwj.12623
Liu, Z., Wang, X., Xing, Z., Xu, P., and Sun, J. (2020). Nano-cerium oxide promotes proliferation of hepatoma cells and regulates mRNA expression of apoptosis-related genes bcl-2 and bax, as detected through real-time fluorescent quantitative polymerase chain reaction. J. Nanosci. Nanotechnol. 20, 7457–7463. doi:10.1166/jnn.2020.18718
Lu, M., Zhang, Y., Wang, Y., Jiang, M., and Yao, X. (2016). Insight into several factors that affect the conversion between antioxidant and oxidant activities of nanoceria. ACS Appl. Mat. Interfaces 8, 23580–23590. doi:10.1021/acsami.6b08219
Ludin, A., Gur-Cohen, S., Golan, K., Kaufmann, K. B., Itkin, T., Medaglia, C., et al. (2014). Reactive oxygen species regulate hematopoietic stem cell self-renewal, migration and development, as well as their bone marrow microenvironment. Antioxid. Redox Signal. 21, 1605–1619. doi:10.1089/ars.2014.5941
Luo, J., Liu, W., Xie, Q., He, J., and Jiang, L. (2023). Synthesis and characterisation of a novel poly(2-hydroxyethylmethacrylate)-chitosan hydrogels loaded cerium oxide nanocomposites dressing on cutaneous wound healing on nursing care of chronic wound. IET Nanobiotechnol 17 (4), 312–325. doi:10.1049/nbt2.12118
Ma, X., Cheng, Y., Jian, H., Feng, Y., Chang, Y., Zheng, R., et al. (2019). Hollow, rough, and nitric oxide-releasing cerium oxide nanoparticles for promoting multiple stages of wound healing. Adv. Healthc. Mater 8 (16), e1900256. doi:10.1002/adhm.201900256
Maccarone, R., Tisi, A., Passacantando, M., and Ciancaglini, M. (2020). Ophthalmic applications of cerium oxide nanoparticles. J. Ocul. Pharmacol. Ther. 36 (6), 376–383. doi:10.1089/jop.2019.0105
Mackevica, A., Hendriks, L., Meili-Borovinskaya, O., Baun, A., and Skjolding, L. M. (2023). Effect of exposure concentration and growth conditions on the association of cerium oxide nanoparticles with green algae. Nanomater. (Basel) 13 (17), 2468. doi:10.3390/nano13172468
Mai, H. X., Sun, L. D., Zhang, Y. W., Si, R., Feng, W., Zhang, H. P., et al. (2005). Shape-selective synthesis and oxygen storage behavior of ceria nanopolyhedra, nanorods, and nanocubes. J. Phys. Chem. B 109 (51), 24380–24385. doi:10.1021/jp055584b
Mehta, A., Scammon, B., Shrake, K., Bredikhin, M., Gil, D., Shekunova, T., et al. (2020). Nanoceria: metabolic interactions and delivery through PLGA-encapsulation. Mat. Sci. Eng. C 114, 111003. doi:10.1016/j.msec.2020.111003
Midwood, K. S., Williams, L. V., and Schwarzbauer, J. E. (2004). Tissue repair and the dynamics of the extracellular matrix. Int. J. Biochem. Cell Biol. 36, 1031–1037. doi:10.1016/j.biocel.2003.12.003
Nadeem, M., Khan, R., Afridi, K., Nadhman, A., Ullah, S., Faisal, S., et al. (2020). Green synthesis of cerium oxide nanoparticles (CeO2 NPs) and their antimicrobial applications: a review. Int. J. Nanomedicine 15, 5951–5961. doi:10.2147/ijn.s255784
Naganuma, T. (2017). Shape design of cerium oxide nanoparticles for enhancement of enzyme mimetic activity in therapeutic applications. Nano Res. 10, 199–217. doi:10.1007/s12274-016-1278-4
Naidi, S. N., Harunsani, M. H., Tan, A. L., and Khan, M. M. (2021). Green-synthesized CeO2 nanoparticles for photocatalytic, antimicrobial, antioxidant and cytotoxicity activities. J. Mater Chem. B 9 (28), 5599–5620. doi:10.1039/d1tb00248a
Nelson, B. C., Johnson, M. E., Walker, M. L., Riley, K. R., and Sims, C. M. (2016). Antioxidant cerium oxide nanoparticles in biology and medicine. Antioxidants 5, 15. doi:10.3390/antiox5020015
Niemiec, S. M., Louiselle, A. E., Hilton, S. A., Dewberry, L. C., Zhang, L., Azeltine, M., et al. (2020). Nanosilk increases the strength of diabetic skin and delivers CNP-miR146a to improve wound healing. Front. Immunol. 11, 590285. doi:10.3389/fimmu.2020.590285
Nosrati, H., Heydari, M., and Khodaei, M. (2023). Cerium oxide nanoparticles: synthesis methods and applications in wound healing. Mater Today Bio 23, 100823. doi:10.1016/j.mtbio.2023.100823
Nyoka, M., Choonara, Y. E., Kumar, P., Kondiah, P. P. D., and Pillay, V. (2020). Synthesis of cerium oxide nanoparticles using various methods: implications for biomedical applications. Nanomaterials 10, 242. doi:10.3390/nano10020242
Özkan, E., Cop, P., Benfer, F., Hofmann, A., Votsmeier, M., Guerra, J. M., et al. (2020). J. Phys. Chem. C 124 (16), 8736–8748.
Ozkan, E., Cop, P., Benfer, F., Hofmann, A., Votsmeier, M., Guerra, J. M., et al. (2020). Rational synthesis concept for cerium oxide nanoparticles: on the impact of particle size on the oxygen storage capacity. J. Phys. Chem. C 124, 8736–8748. doi:10.1021/acs.jpcc.0c00010
Pandey, S., Kumari, S., Manohar Aeshala, L., and Singh, S. (2024). Investigating temperature variability on antioxidative behavior of synthesized cerium oxide nanoparticle for potential biomedical application. J. Biomater. Appl. 38 (7), 866–874. doi:10.1177/08853282231226037
Park, I.-S., Mahapatra, C., Park, J. S., Dashnyam, K., Kim, J.-W., Ahn, J. C., et al. (2020). Revascularization and limb salvage following critical limb ischemia by nanoceria-induced Ref-1/APE1-dependent angiogenesis. Biomaterials 242, 119919. doi:10.1016/j.biomaterials.2020.119919
Piipponen, M., Li, D., and Landén, N. X. (2020). The immune functions of keratinocytes in skin wound healing. Ijms 21, 8790. doi:10.3390/ijms21228790
Plocon, C., Evanghelidis, A., Enculescu, M., Isopencu, G., Oprea, O., Bacalum, M., et al. (2023). Development and characterization of electrospun composites built on polycaprolactone and cerium-containing phases. Int. J. Mol. Sci. 24 (18), 14201. doi:10.3390/ijms241814201
Popova, N., Andreeva, V. V., Khohlov, N. V., Popov, A., and Ivanov, V. (2020). Fabrication of CeO2 nanoparticles embedded in polysaccharide hydrogel and their application in skin wound healing. Nanosyst. Phys. Chem. Math. 11, 99–109. doi:10.17586/2220-8054-2020-11-1-99-109
Purohit, S. D., Singh, H., Bhaskar, R., Yadav, I., Chou, C.-F., Gupta, M. K., et al. (2020). Gelatin—alginate—cerium oxide nanocomposite scaffold for bone regeneration. Mat. Sci. Eng. C 116, 111111. doi:10.1016/j.msec.2020.111111
Raja, I. S., and Fathima, N. N. (2018). Gelatin-cerium oxide nanocomposite for enhanced excisional wound healing. ACS Appl. Bio Mater 1 (2), 487–495. doi:10.1021/acsabm.8b00208
Ribera, J., Rodríguez-Vita, J., Cordoba, B., Portolés, I., Casals, G., Casals, E., et al. (2019). PLoS One 14, e0218716. doi:10.1371/journal.pone.0218716
Rivera-Briso, A. L., and Serrano-ArocaÁ, (2018). Poly(3-Hydroxybutyrate-co-3-Hydroxyvalerate): enhancement strategies for advanced applications. Polym. (Basel) 10 (7), 732. doi:10.3390/polym10070732
Růžička, J., Dejmek, J., Bolek, L., Beneš, J., and Kuncová, J. (2021). Hyperbaric oxygen influences chronic wound healing - a cellular level review. Physiol. Res. 70 (S3), S261–S273. doi:10.33549/physiolres.934822
Rzigalinski, B. A., Carfagna, C. S., and Ehrich, M. (2017). Cerium oxide nanoparticles in neuroprotection and considerations for efficacy and safety. Wiley Interdiscip. Rev. Nanomed Nanobiotechnol 9 (4). doi:10.1002/wnan.1444
Sadidi, H., Hooshmand, S., Ahmadabadi, A., Javad Hosseini, S., Baino, F., Vatanpour, M., et al. (2020). Cerium oxide nanoparticles (nanoceria): hopes in soft tissue engineering. Molecules 25 (19), 4559. doi:10.3390/molecules25194559
Saleh, H., Nassar, A. M., Noreldin, A. E., Samak, D., Elshony, N., Wasef, L., et al. (2020). Chemo-protective potential of cerium oxide nanoparticles against fipronil-induced oxidative stress, apoptosis, inflammation and reproductive dysfunction in male white albino rats. Molecules 25, 3479. doi:10.3390/molecules25153479
Sangha, M. S., Deroide, F., and Meys, R. (2024). Wound healing, scarring and management. Clin. Exp. Dermatol 49 (4), 325–336. doi:10.1093/ced/llad410
Sanmugam, A., Sellappan, L. K., Manoharan, S., Rameshkumar, A., Kumar, R. S., Almansour, A. I., et al. (2024). Development of chitosan-based cerium and titanium oxide loaded polycaprolactone for cutaneous wound healing and antibacterial applications. Int. J. Biol. Macromol. 256 (Pt 1), 128458. doi:10.1016/j.ijbiomac.2023.128458
Seal, S., Jeyaranjan, A., Neal, C. J., Kumar, U., Sakthivel, T. S., and Sayle, D. C. (2020). Engineered defects in cerium oxides: tuning chemical reactivity for biomedical, environmental. energy Appl. Nanoscale 12 (13), 6879–6899. doi:10.1039/d0nr01203c
Sener, G., Hilton, S. A., Osmond, M. J., Zgheib, C., Newsom, J. P., Dewberry, L., et al. (2020). Injectable, self-healable zwitterionic cryogels with sustained microRNA - cerium oxide nanoparticle release promote accelerated wound healing. Acta Biomater. 101, 262–272. doi:10.1016/j.actbio.2019.11.014
Sharma, G., Prema, D., Venkataprasanna, K. S., Prakash, J., Sahabuddin, S., and Devanand Venkatasubbu, G. (2020). Photo induced antibacterial activity of CeO2/GO against wound pathogens. Arab. J. Chem. 13, 7680–7694. doi:10.1016/j.arabjc.2020.09.004
Sharma, M., Yadav, S., Ganesh, N., Srivastava, M. M., and Srivastava, S. (2019). Biofabrication and characterization of flavonoid-loaded Ag, Au, Au-Ag bimetallic nanoparticles using seed extract of the plant Madhuca longifolia for the enhancement in wound healing bio-efficacy. Prog. Biomater. 8 (1), 51–63. doi:10.1007/s40204-019-0110-0
Singh, H., Purohit, S. D., Bhaskar, R., Yadav, I., Bhushan, S., Gupta, M. K., et al. (2021). Biomatrix from goat-waste in sponge/gel/powder form for tissue engineering and synergistic effect of nanoceria. Biomed. Mater 16 (2), 025008. doi:10.1088/1748-605x/abdb74
Singh, K. R., Nayak, V., Sarkar, T., and Singh, R. P. (2020). Cerium oxide nanoparticles:properties,biosynthesis and biomedical application. RSC Adv. 10 (45), 27194–27214. doi:10.1039/d0ra04736h
Singh, S., Ly, A., Das, S., Sakthivel, T. S., Barkam, S., and Seal, S. (2018). Cerium oxide nanoparticles at the nano-bio interface: size-dependent cellular uptake. Artif. Cells Nanomed Biotechnol. 46 (Suppl. 3), S956–S963. doi:10.1080/21691401.2018.1521818
Soni, S., Vats, V. S., Kumar, S., Dalela, B., Mishra, M., Meena, R. S., et al. (2018). Structural, optical and magnetic properties of Fe-doped CeO2 samples probed using X-ray photoelectron spectroscopy. J. Mat. Sci. Mat. Electron. 29, 10141–10153. doi:10.1007/s10854-018-9060-x
Sorg, H., Tilkorn, D. J., Hager, S., Hauser, J., and Mirastschijski, U. (2017). Skin wound healing: an update on the current knowledge and concepts. Eur. Surg. Res. 58 (1-2), 81–94. doi:10.1159/000454919
Stager, M. A., Bardill, J., Raichart, A., Osmond, M., Niemiec, S., Zgheib, C., et al. (2022). Photopolymerized zwitterionic hydrogels with a sustained delivery of cerium oxide nanoparticle-miR146a conjugate accelerate diabetic wound healing. ACS Appl. Bio Mater 5 (3), 1092–1103. doi:10.1021/acsabm.1c01155
Stan, D., Tanase, C., Avram, M., Apetrei, R., Mincu, N.-B., Mateescu, A. L., et al. (2021). Wound healing applications of creams and “smart” hydrogels. Exp. Dermatol. 30, 1218–1232. doi:10.1111/exd.14396
Sun, Z., Wu, B., Ren, Y., Wang, Z., Zhao, C. X., Hai, M., et al. (2021). Diverse particle carriers prepared by Co-precipitation and phase separation: formation and applications. Chempluschem 86 (1), 49–58. doi:10.1002/cplu.202000497
Thakur, N., Manna, P., and Das, J. (2019). Synthesis and biomedical applications of nanoceria, a redox active nanoparticle. J. Nanobiotechnol 17 (1), 84. doi:10.1186/s12951-019-0516-9
Tracy, L. E., Minasian, R. A., and Caterson, E. J. (2016). Extracellular matrix and dermal fibroblast function in the healing wound. Adv. Wound Care 5, 119–136. doi:10.1089/wound.2014.0561
Veith, A. P., Henderson, K., Spencer, A., Sligar, A. D., and Baker, A. B. (2019). Therapeutic strategies for enhancing angiogenesis in wound healing. Adv. Drug Deliv. Rev. 146, 97–125. doi:10.1016/j.addr.2018.09.010
Velnar, T., and Bailey, T. (2009). The wound healing process: an overview of the cellular and molecular mechanisms. J. Int. Med. Res. 37, 1528–1542. doi:10.1177/147323000903700531
Velnar, T., Bailey, T., and Smrkolj, V. (2009). The wound healing process: an overview of the cellular and molecular mechanisms. J. Int. Med. Red. 37, 1528–1542. doi:10.1177/147323000903700531
Walkey, C., Das, S., Seal, S., Erlichman, J., Heckman, K., Ghibelli, L., et al. (2015). Catalytic properties and biomedical applications of cerium oxide nanoparticles. Environ. Sci. Nano 2 (1), 33–53. doi:10.1039/c4en00138a
Wang, L., Ai, W., Zhai, Y., Li, H., Zhou, K., and Chen, H. (2015). Effects of nano-CeO with different nanocrystal morphologies on cytotoxicity in HepG2 cells. Int. J. Environ. Res. Public Health 12 (9), 10806–10819. doi:10.3390/ijerph120910806
Wang, N., Liang, H., and Zen, K. (2014). Molecular mechanisms that influence the macrophage M1-M2 polarization balance. Front. Immunol. 5, 1–9. doi:10.3389/fimmu.2014.00614
Wang, P. H., Huang, B. S., Horng, H. C., Yeh, C. C., and Chen, Y. J. (2018). Wound healing. J. Chin. Med. Assoc. 81 (2), 94–101. doi:10.1016/j.jcma.2017.11.002
Wang, Y., Yang, L., Liu, B., Liao, S., Fu, X., Zhou, Y., et al. (2023). Radiation skin injury care in radiotherapy for oncology: mechanisms, drug therapy and novel biomaterial application strategies. Adv. Therap. 6 (11), 2300024
Wei, F., Neal, C. J., Sakthivel, T. S., Kean, T., Seal, S., and Coathup, M. J. (2021). Multi-functional cerium oxide nanoparticles regulate inflammation and enhance osteogenesis. Mat. Sci. Eng. C 124, 112041. doi:10.1016/j.msec.2021.112041
Wilkinson, H. N., and Hardman, M. J. (2020a). Wound healing: cellular mechanisms and pathological outcomes: cellular mechanisms of wound repair. Open Biol. 10, 200223. doi:10.1098/rsob.200223
Wilkinson, H. N., and Hardman, M. J. (2020b). Wound healing: cellular mechanisms and pathological outcomes. Open Biol. 10 (9), 200223. doi:10.1098/rsob.200223
Xu, C., and Qu, X. (2014). Cerium oxide nanoparticle: a remarkably versatile rare earth nanomaterial for biological applications. NPG Asia Mat. 6 (3), e90. doi:10.1038/am.2013.88
Xu, Y., Mofarah, S. S., Mehmood, R., Cazorla, C., Koshy, P., and Sorrell, C. C. (2021). Design strategies for ceria nanomaterials: untangling key mechanistic concepts. Mat. Horiz. 8, 102–123. doi:10.1039/d0mh00654h
Xue, Y., Yang, F., Wu, L., Xia, D., and Liu, Y. (2024). CeO2 nanoparticles to promote wound healing: a systematic review. Adv. Healthc. Mater 13 (6), e2302858. doi:10.1002/adhm.202302858
Yang, B. Y., Zhou, Z. Y., Liu, S. Y., Shi, M. J., Liu, X. J., Cheng, T. M., et al. (2022). Porous Se@SiO2 nanoparticles enhance wound healing by ROS-PI3K/akt pathway in dermal fibroblasts and reduce scar formation. Front. Bioeng. Biotechnol. 10, 852482. doi:10.3389/fbioe.2022.852482
Yildizbakan, L., Iqbal, N., Ganguly, P., Kumi-Barimah, E., Do, T., Jones, E., et al. (2023). Fabrication and characterisation of the cytotoxic and antibacterial properties of chitosan-cerium oxide porous scaffolds. Antibiot. (Basel) 12 (6), 1004. doi:10.3390/antibiotics12061004
Zeng, Q., Qi, X., Shi, G., Zhang, M., and Haick, H. (2022). Wound dressing: from nanomaterials to diagnostic dressings and healing evaluations. ACS Nano 16 (2), 1708–1733. doi:10.1021/acsnano.1c08411
Zgheib, C., Hilton, S. A., Dewberry, L. C., Hodges, M. M., Ghatak, S., Xu, J., et al. (2019). Use of cerium oxide nanoparticles conjugated with MicroRNA-146a to correct the diabetic wound healing impairment. J. Am. Coll. Surg. 228 (1), 107–115. doi:10.1016/j.jamcollsurg.2018.09.017
Zhang, Z., Wang, J., Luo, Y., Li, C., Sun, Y., Wang, K., et al. (2023). A pH-responsive ZC-QPP hydrogel for synergistic antibacterial and antioxidant treatment to enhance wound healing. J. Mater Chem. B 11 (38), 9300–9310. doi:10.1039/d3tb01567j
Zhao, R., Liang, H., Clarke, E., Jackson, C., and Xue, M. (2016). Inflammation in chronic wounds. Int. J. Mol. Sci. 17, 2085. doi:10.3390/ijms17122085
Zhao, R., Zhao, C., Wan, Y., Majid, M., Abbas, S. Q., and Wang, Y. (2023). In vitro and in vivo evaluation of alginate hydrogel-based wound dressing loaded with green chemistry cerium oxide nanoparticles. Front. Chem. 11, 1298808. doi:10.3389/fchem.2023.1298808
Zholobak, N., Ivanov, V., and Shcherbakov, A. (2016) Interaction of nanoceria with microorganisms. New York, NY, USA: Elsevier Inc., 419–450. doi:10.1016/b978-0-323-42864-4.00012-9
Zhu, Z., Ding, J., Qin, M., Wang, L., Jiang, D., Zhao, J., et al. (2024). Enhanced ·OH-scavenging activity of Cu-CeOx nanozyme via resurrecting macrophage Nrf2 transcriptional activity facilitates diabetic wound healing. Adv. Healthc. Mater, doi:10.1002/adhm.202303229
Keywords: cerium oxide, wound healing, mechanism, composite materials, nanotechnology, wearable sensor, toxicity, interdisciplinary
Citation: Chen S, Wang Y, Bao S, Yao L, Fu X, Yu Y, Lyu H, Pang H, Guo S, Zhang H, Zhou P and Zhou Y (2024) Cerium oxide nanoparticles in wound care: a review of mechanisms and therapeutic applications. Front. Bioeng. Biotechnol. 12:1404651. doi: 10.3389/fbioe.2024.1404651
Received: 22 March 2024; Accepted: 29 April 2024;
Published: 20 May 2024.
Edited by:
Hamed Barabadi, Shahid Beheshti University of Medical Sciences, IranReviewed by:
Sisubalan Natarajan, Chiang Mai University, ThailandSerena Del Turco, National Research Council (CNR), Italy
Rakesh Bhaskar, Yeungnam University, Republic of Korea
Copyright © 2024 Chen, Wang, Bao, Yao, Fu, Yu, Lyu, Pang, Guo, Zhang, Zhou and Zhou. This is an open-access article distributed under the terms of the Creative Commons Attribution License (CC BY). The use, distribution or reproduction in other forums is permitted, provided the original author(s) and the copyright owner(s) are credited and that the original publication in this journal is cited, in accordance with accepted academic practice. No use, distribution or reproduction is permitted which does not comply with these terms.
*Correspondence: Haowen Pang, haowenpang@foxmail.com; Shengmin Guo, 2930773281@qq.com; Hongwei Zhang, zhanghongwei2020@swmu.edu.cn; Ping Zhou, zhouping11@swmu.edu.cn; Yun Zhou, yzhou@swmu.edu.cn
†These authors have contributed equally to this work and share first authorship