Biomaterials recycling: a promising pathway to sustainability
- 1Department of Polymer Technology, Faculty of Chemistry, Gdańsk University of Technology, Gdańsk, Poland
- 2Advanced Materials Center, Gdańsk University of Technology, Gdańsk, Poland
- 3Department of Chemical Engineering, Northeastern University, Boston, MA, United States
- 4Department of Bioengineering, Northeastern University, Boston, MA, United States
- 5Harvard John A. Paulson School of Engineering and Applied Sciences, Harvard University, Cambridge, MA, United States
- 6Biomechanics and Bioengineering (BMBI), UTC CNRS UMR 7338, University of Technology of Compiègne, Sorbonne University, Compiègne, France
Biomaterials undergo a transformative journey, from their origin as renewable resources to the manufacturing plants where they are processed and stored, until they fulfill their intended therapeutic or diagnostic purposes and become medical waste. However, during this life cycle, biomaterials can be susceptible to contamination and subsequent degradation through various mechanisms such as hydro-mechanical, thermal, or biochemical processes in water, soil, or air. These factors raise significant concerns regarding biological safety. Additional complexities arise from the potential amalgamation of biomaterials with other materials, either of the same kind or different types. Use of biomaterials influences their porosity, surface chemistry, and structural strength, and these factors affect biomaterials’ reusability. Given the multitude of materials, processing parameters, sustainability requirements, and the limitation of natural resources, the recycling of biomaterials becomes necessary. Unfortunately, this topic has received limited attention thus far. In this context, this perspective provides a brief overview, analysis, and classification of reports on biomaterials recycling, aiming to initiate a discussion on this frequently overlooked subject. We highlight the challenges related to energy consumption and environmental pollution. However, the lack of established protocols and reporting on biomaterials recycling prevents a comprehensive understanding of these challenges and potential solutions. Nevertheless, addressing these issues can lead to more efficient resource use and reduced environmental impact in the field of biomaterials.
1 Introduction
The term “biomaterial” refers to a substance, either natural or synthetic, which is involved in a complex material or system that interacts with a living tissue, organ, or system for diagnostic or therapeutic purposes. In general, biomaterials are designed to perform at least one of the following functions: replace a damaged tissue, transfer or receive signals from the surrounding cells, modulate immune cell behavior (Colombani et al., 2021a; Colombani et al., 2021b), or rely on a biological function (Rodrigo-Navarro et al., 2021; Whitaker et al., 2021). Advanced multifunctional biomaterials can regulate functions such as macrophage polarization (Mao et al., 2022), drug delivery (Khan et al., 2019; Servatan et al., 2020), and therapy and regeneration (Chen et al., 2020; Rana et al., 2023). The design of stimuli-responsive biomaterials is deeply rooted in chemistry (Rogers et al., 2020; Taghizadeh et al., 2022; Yazdi et al., 2022). However, advanced integrated approaches must be used to form the shape, construct the bioactivity, and adjust the cell viability of these biomaterials (Brokesh and Gaharwar, 2020; Chua et al., 2021; Fu et al., 2022). For example, additive manufacturing (3D printing) can facilitate development of function-shaped patient-specific implants (Jafari et al., 2022). Therefore, materials science, chemistry, engineering, biology, and manufacturing techniques can be considered the five main pillars of biomaterials engineering. Regardless of the type of biomaterial, the porosity, surface area, aspect ratio, and biodegradability are factors that affect a biomaterial’s efficacy (Gsib et al., 2020; Servatan et al., 2020).
Biomaterials can be classified based on their chemistry, functionality, biocompatibility, responsiveness, and sustainability. These classifications are at times interchangeably or mistakenly applied. However, from the standpoint of chemistry, biomaterials can be categorized into the main classes of ceramics, glasses, polymers, metals, and composites. To define and distinguish biomaterials based on their chemistry, terms such as chemical composition, chemical structure, chemical backbone, and surface chemistry are frequently used by researchers (Kargozar et al., 2019). Bioceramics can be classified as bioinert, bioresorbable, or bioactive. Bioinert ceramic implants become encapsulated by non-adherent fibrous tissue, bioresorbable implants can be resorbed and incorporated into the local tissue (e.g., bone), and bioactive ceramics allow for bond formation between implant and tissue (Punj et al., 2021). Biometals are known for their biocompatibility and load-bearing properties, among which titanium, cobalt, stainless steel, and their alloys, are best known, while magnesium and zinc alloys are biodegradable (Saini et al., 2015). In addition to biocompatibility and biodegradability concerns, the corrosivity of metallic biomaterials is an important consideration. Anodic or cathodic reactions arising from biological macromolecules can govern the corrosion rate of implants (Eliaz, 2019). Polymers make up the most numerous group of biomaterials because their flexibility for molecular design provides a wide range of properties and performance (Wiśniewska et al., 2023). They can be used in the form of thin films, foams, hydrogels, scaffolds, nanoplatforms, implants, orthoses, and prosthetic devices (Kalirajan et al., 2021; Kim et al., 2014; Rabiee et al., 2022; Rezaeeyazdi et al., 2022; Yazdi et al., 2020). In addition to the molecular architecture of polymeric biomaterials, their biodegradability, hydrophilicity, surface chemistry, and mechanisms of erosion are of vital importance. The performance of polymeric biomaterials can significantly be improved through surface and bulk modification with nanoparticles or supplementary biodegradable polymers (Saeedi et al., 2022; Seidi et al., 2021; Sun et al., 2020). In particular, 3D and 4D printing of polymeric biomaterials has progressively evolved over the last decade because of its usefulness in personalized medicine as well as its low cost and high design flexibility (Pugliese et al., 2021; Shokrani et al., 2022).
2 Perspective on biomaterials recycling
In recent years, the design, manufacturing, and commercialization of mission-specific biomaterials have accelerated as the field has matured. Consequently, the need for repair, replacement, and recycling of biomaterials has also increased. When implanted orally or within the body, biomaterials are not fully degradable. This requires that additional steps be taken to address concerns about sustainability and circularity. Nevertheless, current knowledge and available data on biomaterials recycling are limited. Recent analyses based on Life Cycle Assessment (LCA) have revealed some of the environmental impacts and carbon footprint consequences of biomaterials (Hjuler and Hansen, 2018; Soman and Ajitha, 2018). Nevertheless, the environmental impacts and recyclability of biomaterials have not been at the center of attention. This article aims to provide meaningful insights from limited sources of data and highlight the urgent necessity of recycling biomaterials utilized in medical devices, artificial tissues, organs, and other applications. The main findings of relevant studies are summarized and discussed to provide readers with a comprehensive background for future advancements.
2.1 Current strategies for recycling biomaterials
Biomaterials are advanced materials that provide unique and valuable functions in vivo and/or in vitro. The production of biomaterials has continuously been increasing in step with progress in biomaterials engineering and related fields of research and technology, especially in regenerative medicine (Chen et al., 2016). Currently, biomaterials are frequently used in diverse medical devices, such as implants, scaffolds, dental products, prostheses, stents, catheters, and artificial organs. Once these biomaterials have served their purpose, they are replaced or withdrawn. As biomaterials come to play an increasingly more prominent role in medicine, their collection and management in medical waste is becoming a more significant problem. Currently, waste biomaterials are most often landfilled or incinerated (Joseph et al., 2021). However, as they are potentially infectious waste, biomaterials discarded in landfills may present environmental hazards, including soil and water pollution resulting from waste degradation. On the other hand, inadequate incineration of biomaterials may lead to the release of toxic chemical compounds into the atmosphere (Joseph et al., 2021). Therefore, biomaterials recycling is an urgent issue to be addressed. Table 1 provides the most pertinent information extracted from published review articles relevant to biomaterials recycling.
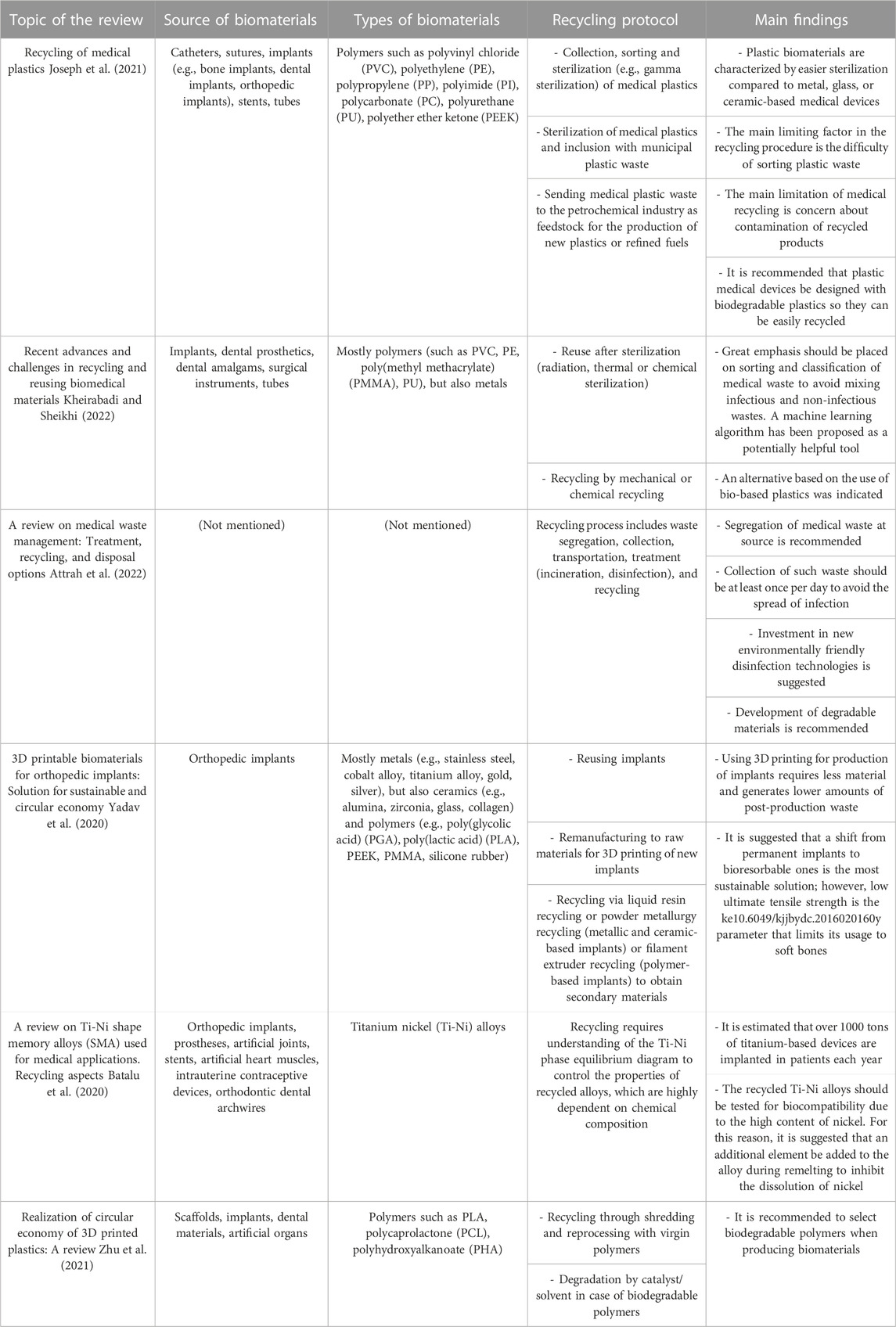
TABLE 1. Overview of biomaterials recycling strategies. This table presents a comprehensive compilation of biomaterials recycling strategies. It summarizes the proposed recycling approaches and their primary outcomes in managing various types of biomaterials. The strategies discussed are inspired and derived from available data in various review articles.
Considering all available reports, the recommended first step to successful recycling of biomaterials is segregation by the type of material (polymer, metal, or ceramic) (Kheirabadi and Sheikhi, 2022; Yadav et al., 2020), specific composition (Lee et al., 2002; Joseph et al., 2021), waste source (Attrah et al., 2022; Lee et al., 2002), and infection chance (Kheirabadi and Sheikhi, 2022; Yadav et al., 2020). Figure 1 illustrates an exemplary composition of biomaterials waste, highlighting the chemical diversity of the materials.
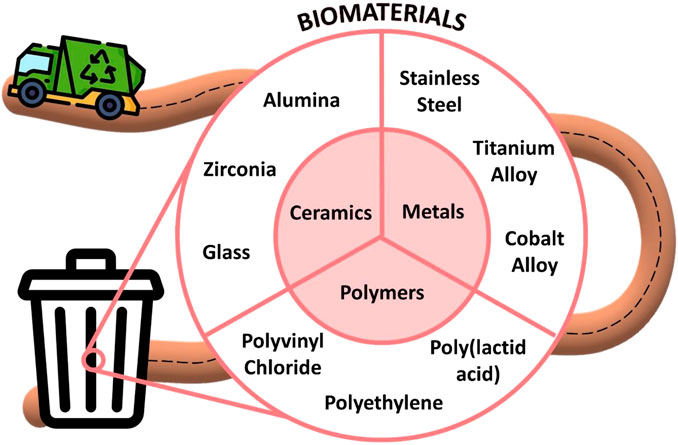
FIGURE 1. Composition of biomaterials waste. This schematic illustration depicts a diverse range of materials associated with biomaterials such as ceramics, metals, and polymers. Therefore, it is essential to ensure proper segregation for successful and sustainable recycling.
2.2 Dental and orthopedic implants
One study estimated that direct collection and appropriate recycling of metal alloys used in dental restorations and silver amalgam alloys, from 300 dental colleges, could recover approximately 500 kg of Co-Cr and Ni-Cr alloys, 500 kg of silver, and 850 kg of mercury (Thopegowda et al., 2013). In another study, Yadav et al. (2020) proposed effective ways to recycle orthopedic implants. They claimed that extrusion can be used for recycling of polymeric implants, while powder metallurgy or liquid resin recycling methods would be suitable for metallic and ceramic-based implants. According to the authors, following these paths would allow these recycled products to be reused in the manufacturing of subsequent implants or other biomaterials. Moreover, they highlighted that the crucial role in the fate of the waste implant is played by the doctor who, based on the condition of the implant, may decide to reuse, remanufacture, or recycle it. Regarding practical research, Wang et al. (2020) showed that there is no significant difference in the interfacial morphology of Pd-Cu-Ga and Au-Pt metal-ceramic dental alloys after recasting up to 3 times. Nevertheless, it is worth noting that in some cases, even collection at source and recycling of a specific type of biomaterial may not ensure similar characteristics to the original material. For example, recasting of some metallic alloys could potentially change the corrosion properties and thus the cytotoxicity of these alloys. Tripuraneni and Namburi (2008) demonstrated that Ni-Cr dental casting alloys prepared from their recycled form induced considerable genotoxicity, especially those with a higher content of recycled material. However, if recycling is not taken into consideration during the removal of biomaterials from the human body, managing them becomes significantly more challenging. For instance, the recycling of mixed polymeric biomaterials becomes complicated due to the wide range of components used in their production and their diverse behavior during processing.
2.3 Medical plastic waste
According to Kheirabadi and Sheikhi (2022), the recycling of medical plastic waste, including polymeric biomaterials, can theoretically undergo mechanical (secondary) and chemical (tertiary) recycling methods commonly used for typical polymers. However, it is essential to sterilize waste biomaterials before including them with municipal plastic waste (Joseph, et al., 2021). In practical terms, limitations in waste sorting may result in materials made from recycled polymers having weak interfacial adhesion and low mechanical properties. The utilization of advanced tools such as machine learning to enhance sorting efficiency may help to overcome this challenge (Kheirabadi and Sheikhi, 2022). Although this approach represents a conventional solution to tackle the problem, there are other possible strategies for biomaterials recycling, as depicted in Figure 2.
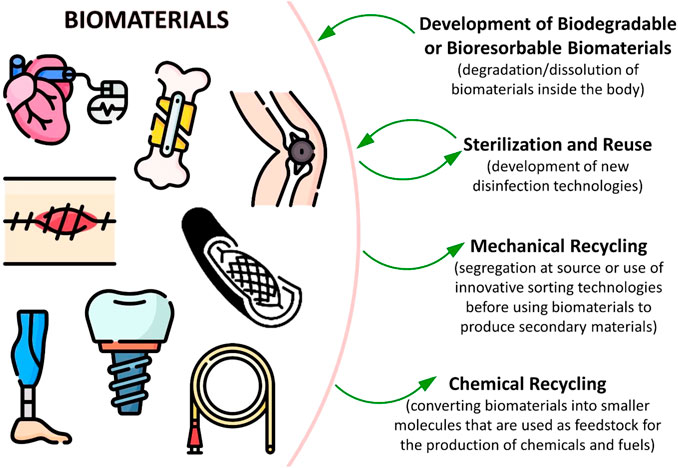
FIGURE 2. An overview of various strategies employed in biomaterials recycling. The direction of the arrows highlights that recycling strategies require specific actions to be taken during the biomaterial production stage.
2.4 Sterilization and reuse of biomaterials
Based on the conclusions drawn by other scientists (Attrah et al., 2022; Kheirabadi and Sheikhi, 2022; Yadav et al., 2020) and our own experience, greater attention should be given to the reuse of biomaterials after adequate treatment and sterilization. Several studies have reported successful application of this strategy, demonstrating that material properties can be maintained without compromise. For example, Estelita et al. (2014) compared the mechanical properties of unused mini-implants with mini-implants inserted and removed from pig iliac bone with and without additional treatment (ultrasonic cleaning, autoclave sterilization, and/or sandblasting). It was found that regardless of the treatment/sterilization technique used, the torsional strength of screws was unchanged. On the other hand, it was revealed that for some cases, the sterilization process may affect the final behavior of the biomaterial. For instance, Sayed et al. (2018) found that orthodontic archwires composed of Ni-Ti and Cu-Ni-Ti alloys experienced deterioration in ultimate tensile strength after two cycles of autoclave sterilization (134°C, 18 min) or chemical disinfection (1% hydrogen peroxide solution, 30 min). In addition, after just one cycle, some surface irregularities were observed on the archwires. Similarly, it was reported that flaming or flaming with ultrasonic cleaning resulted in noticeable reduction in shear bond strength of stainless steel orthodontic brackets (Khanal et al., 2021). However, applying flame treatment along with sandblasting provided satisfactory strength for clinical use, which was almost indistinguishable from the original product. Therefore, the field urgently needs novel, environmentally friendly disinfection technologies that would allow greater reuse of biomaterials without the fear of deterioration of properties (Attrah et al., 2022).
2.5 Waste-collection changes will accelerate biomaterial recycling
Although the above recycling strategies shed light on the proper management of waste biomaterials, such approaches require major changes in the biomaterial waste collection system and the awareness of healthcare professionals. For instance, each type of biomaterial should be segregated and categorized at the source and, whenever possible, reused after sterilization. This will maximize the effectiveness of recycling and allow the production of valuable secondary products in accordance with the concept of sustainable development. However, the most promising alternative for achieving a sustainable, circular economy of biomaterials is the shift towards the development of biodegradable or bioresorbable biomaterials. This approach has been referenced multiple times in the literature (Attrah et al., 2022; Joseph et al., 2021; Yadav et al., 2020; Zhu et al., 2021). Such materials do not pose any burden to the environment, as they dissolve or degrade inside the body after a predefined period. Nevertheless, it is believed that low ultimate tensile strength is a key parameter that may limit their use to soft bone implants, among other applications (Yadav et al., 2020).
3 Concluding remarks and future challenges
In the medical field, recycling of biomaterials holds great potential for addressing sustainability challenges. Through this perspective, we have examined and emphasized the numerous benefits associated with biomaterials recycling. This includes significant advantages such as reducing waste generated, cost savings, and preserving valuable resources. Nevertheless, we must address the challenges ahead to maximize biomaterial recycling effectiveness. While we have established the positive impact of recycling, overcoming the existing barriers and hurdles within the recycling process is also essential.
To ensure safety and efficiency in biomaterials recycling, it is also crucial to develop robust collection, sorting, and processing methodologies that adhere to stringent quality control measures. Biomaterials’ contamination and degradation raise significant concerns regarding biological safety. Additionally, incorporating biomaterials into other materials can affect the biomaterials’ porosity, surface chemistry, and structural strength, which introduces complexities for reuse and recyclability. Biomaterials recycling becomes imperative when we consider the diverse nature of these materials and the urgent need to protect our limited natural resources.
Despite the relatively limited emphasis placed on biomaterials recycling, our article offers a concise compilation of up-to-date reports highlighting the inherent challenges associated with energy consumption and pollution in this critical endeavor. Energy-intensive procedures, such as sorting, processing, and reprocessing biomaterials, pose significant obstacles to sustainable recycling practices.
To fully harness the potential of biomaterials recycling and facilitate sustainable transformation in healthcare, it is essential to foster collaborative efforts among researchers, policymakers, and industry stakeholders. This collaborative approach can catalyze technical innovation, establish a regulatory framework, and cultivate a circular economy mindset within the biomedical sector. By addressing these challenges, we can create a sustainable ecosystem that enables efficient biomaterial reuse in therapeutic and diagnostic applications.
Author contributions
PW: Writing–original draft, Writing–review and editing. MS: Writing–original draft, Writing–review and editing, Conceptualization, Supervision. SB: Conceptualization, Supervision, Writing–original draft, Writing–review and editing.
Funding
The author(s) declare financial support was received for the research, authorship, and/or publication of this article. SAB gratefully acknowledges the financial support from the National Institutes of Health (NIH, 1R01EB027705) and the National Science Foundation (NSF CAREER, DMR 1847843). MRS thankfully acknowledges the financial support provided by the Gdańsk University of Technology, the DEC-1/2021/IDUB/I.1Pt grant under the Platinum Establishing Top-Class Research Teams—Excellence Initiative—Research University program.
Conflict of interest
The authors declare that the research was conducted in the absence of any commercial or financial relationships that could be construed as a potential conflict of interest. The authors (MRS) and (SAB) declared that they were editorial board members of Frontiers at the time of submission. This had no impact on the peer review process and the final decision.
Publisher’s note
All claims expressed in this article are solely those of the authors and do not necessarily represent those of their affiliated organizations, or those of the publisher, the editors and the reviewers. Any product that may be evaluated in this article, or claim that may be made by its manufacturer, is not guaranteed or endorsed by the publisher.
References
Attrah, M., Elmanadely, A., Akter, D., and Rene, E. R. (2022). A review on medical waste management: treatment, recycling, and disposal options. Environments 9, 146. doi:10.3390/environments9110146
Batalu, D., Guoqiu, H., Aloman, A., Coşmeleaţă, G., Xiaoshan, L., and Zhihua, Z. (2020). “A review on TiNi shape memory alloys (SMA) used for medical applications,” in Recycling aspects (Berlin, Germany: Springer).
Brokesh, A. M., and Gaharwar, A. K. (2020). Inorganic biomaterials for regenerative medicine. ACS Appl. Mater. interfaces 12, 5319–5344. doi:10.1021/acsami.9b17801
Chen, B., Xiang, H., Pan, S., Yu, L., Xu, T., and Chen, Y. (2020). Advanced theragenerative biomaterials with therapeutic and regeneration multifunctionality. Adv. Funct. Mater. 30, 2002621. doi:10.1002/adfm.202002621
Chen, Q., Ting, Y., and Chang, X. (2016). Research on development trends, mode comparison of enterprise product R&D management and its inspirat. Sci. Technol. Prog. Policy 33, 86–91.
Chua, K., Khan, I., Malhotra, R., and Zhu, D. (2021). Additive manufacturing and 3D printing of metallic biomaterials. Eng. Regen. 2, 288–299. doi:10.1016/j.engreg.2021.11.002
Colombani, T., Eggermont, L. J., Hatfield, S. M., Rogers, Z. J., Rezaeeyazdi, M., Memic, A., et al. (2021a). Oxygen-generating cryogels restore T cell mediated cytotoxicity in hypoxic tumors. Adv. Funct. Mater. 31, 2102234. doi:10.1002/adfm.202102234
Colombani, T., Eggermont, L. J., Rogers, Z. J., McKay, L. G., Avena, L. E., Johnson, R. I., et al. (2021b). Biomaterials and oxygen join forces to shape the immune response and boost COVID-19 vaccines. Adv. Sci. 8, 2100316. doi:10.1002/advs.202100316
Eliaz, N. (2019). Corrosion of metallic biomaterials: a review. Materials 12, 407. doi:10.3390/ma12030407
Estelita, S., Janson, G., Chiqueto, K., and Ferreira, E. S. (2014). Effect of recycling protocol on mechanical strength of used mini-implants. Int. J. Dent. 2014, 1–5. doi:10.1155/2014/424923
Fu, Z., Ouyang, L., Xu, R., Yang, Y., and Sun, W. (2022). Responsive biomaterials for 3D bioprinting: a review. Mater. Today 52, 112–132. doi:10.1016/j.mattod.2022.01.001
Gsib, O., Eggermont, L. J., Egles, C., and Bencherif, S. A. (2020). Engineering a macroporous fibrin-based sequential interpenetrating polymer network for dermal tissue engineering. Biomaterials Sci. 8, 7106–7116. doi:10.1039/d0bm01161d
Hjuler, S. V., and Hansen, S. B. (2018). LCA of Biofuels and Biomaterials. Life cycle assessment: theory and practice. Berlin, Germany: Springer, 755–782.
Jafari, A., Ajji, Z., Mousavi, A., Naghieh, S., Bencherif, S. A., and Savoji, H. (2022). Latest advances in 3D bioprinting of cardiac tissues. Adv. Mater. Technol. 7, 2101636. doi:10.1002/admt.202101636
Joseph, B., James, J., Kalarikkal, N., and Thomas, S. (2021). Recycling of medical plastics. Adv. Industrial Eng. Polym. Res. 4, 199–208. doi:10.1016/j.aiepr.2021.06.003
Kalirajan, C., Dukle, A., Nathanael, A. J., Oh, T.-H., and Manivasagam, G. (2021). A critical review on polymeric biomaterials for biomedical applications. Polymers 13, 3015. doi:10.3390/polym13173015
Kargozar, S., Ramakrishna, S., and Mozafari, M. (2019). Chemistry of biomaterials: future prospects. Curr. Opin. Biomed. Eng. 10, 181–190. doi:10.1016/j.cobme.2019.07.003
Khan, M. S. J., Khan, S. B., Kamal, T., and Asiri, A. M. (2019). Agarose biopolymer coating on polyurethane sponge as host for catalytic silver metal nanoparticles. Polym. Test. 78, 105983. doi:10.1016/j.polymertesting.2019.105983
Khanal, P. P., Shrestha, B. K., Yadav, R., and Prasad Gupta, D. S. (2021). A comparative study on the effect of different methods of recycling orthodontic brackets on shear bond strength. Int. J. Dent. 2021, 1–7. doi:10.1155/2021/8844085
Kheirabadi, S., and Sheikhi, A. (2022). Recent advances and challenges in recycling and reusing biomedical materials. Curr. Opin. Green Sustain. Chem. 38, 100695. doi:10.1016/j.cogsc.2022.100695
Kim, J., Bencherif, S. A., Li, W. A., and Mooney, D. J. (2014). Cell-friendly inverse opal-like hydrogels for a spatially separated Co-culture system. Macromol. rapid Commun. 35, 1578–1586. doi:10.1002/marc.201400278
Lee, B.-K., Ellenbecker, M. J., and Moure-Eraso, R. (2002). Analyses of the recycling potential of medical plastic wastes. Waste Manag. 22, 461–470. doi:10.1016/s0956-053x(02)00006-5
Mao, J., Chen, L., Cai, Z., Qian, S., Liu, Z., Zhao, B., et al. (2022). Advanced biomaterials for regulating polarization of macrophages in wound healing. Adv. Funct. Mater. 32, 2111003. doi:10.1002/adfm.202111003
Pugliese, R., Beltrami, B., Regondi, S., and Lunetta, C. (2021). Polymeric biomaterials for 3D printing in medicine: an overview. Ann. 3D Print. Med. 2, 100011. doi:10.1016/j.stlm.2021.100011
Punj, S., Singh, J., and Singh, K. (2021). Ceramic biomaterials: properties, state of the art and future prospectives. Ceram. Int. 47, 28059–28074. doi:10.1016/j.ceramint.2021.06.238
Rabiee, N., Atarod, M., Tavakolizadeh, M., Asgari, S., Rezaei, M., Akhavan, O., et al. (2022). Green metal-organic frameworks (MOFs) for biomedical applications. Microporous Mesoporous Mater. 335, 111670. doi:10.1016/j.micromeso.2021.111670
Rana, D., Colombani, T., Saleh, B., Mohammed, H. S., Annabi, N., and Bencherif, S. A. (2023). Engineering injectable, biocompatible, and highly elastic bioadhesive cryogels. Mater. Today Bio 19, 100572. doi:10.1016/j.mtbio.2023.100572
Rezaeeyazdi, M., Colombani, T., Eggermont, L. J., and Bencherif, S. A. (2022). Engineering hyaluronic acid-based cryogels for CD44-mediated breast tumor reconstruction. Mater. Today Bio 13, 100207. doi:10.1016/j.mtbio.2022.100207
Rodrigo-Navarro, A., Sankaran, S., Dalby, M. J., del Campo, A., and Salmeron-Sanchez, M. (2021). Engineered living biomaterials. Nat. Rev. Mater. 6, 1175–1190. doi:10.1038/s41578-021-00350-8
Rogers, Z. J., Zeevi, M. P., Koppes, R., and Bencherif, S. A. (2020). Electroconductive hydrogels for tissue engineering: current status and future perspectives. Bioelectricity 2, 279–292. doi:10.1089/bioe.2020.0025
Saeedi, M., Vahidi, O., Moghbeli, M., Ahmadi, S., Asadnia, M., Akhavan, O., et al. (2022). Customizing nano-chitosan for sustainable drug delivery. J. Control. Release 350, 175–192. doi:10.1016/j.jconrel.2022.07.038
Saini, M., Singh, Y., Arora, P., Arora, V., and Jain, K. (2015). Implant biomaterials: a comprehensive review. World J. Clin. Cases WJCC 3, 52. doi:10.12998/wjcc.v3.i1.52
Sayed, Y., Aboulazm, K., and Fahmy, A. (2018). Evaluation of ultimate tensile strength and surface roughness of NiTi and CuNiTi orthodontic archwires after recycling procedures. Egypt. Orthod. J. 54, 47–57. doi:10.21608/eos.2018.77146
Seidi, F., Yazdi, M. K., Jouyandeh, M., Dominic, M., Naeim, H., Nezhad, M. N., et al. (2021). Chitosan-based blends for biomedical applications. Int. J. Biol. Macromol. 183, 1818–1850. doi:10.1016/j.ijbiomac.2021.05.003
Servatan, M., Zarrintaj, P., Mahmodi, G., Kim, S.-J., Ganjali, M. R., Saeb, M. R., et al. (2020). Zeolites in drug delivery: progress, challenges and opportunities. Drug Discov. Today 25, 642–656. doi:10.1016/j.drudis.2020.02.005
Shokrani, H., Shokrani, A., and Saeb, M. R. (2022). Methods for biomaterials printing: a short review and perspective. Methods 206, 1–7. doi:10.1016/j.ymeth.2022.07.016
Soman, S., and Ajitha, A. (2018). “Life cycle assessment of metallic biomaterials,” in Fundamental biomaterials: metals (United Kingdom: Woodhead Publishing), 411–423.
Sun, W., Liu, W., Wu, Z., and Chen, H. (2020). Chemical surface modification of polymeric biomaterials for biomedical applications. Macromol. Rapid Commun. 41, 1900430. doi:10.1002/marc.201900430
Taghizadeh, A., Taghizadeh, M., Yazdi, M. K., Zarrintaj, P., Ramsey, J. D., Seidi, F., et al. (2022). Mussel-inspired biomaterials: from chemistry to clinic. Bioeng. Transl. Med. 7, e10385. doi:10.1002/btm2.10385
Thopegowda, N. B., Shenoy, K., Shankarnarayana, R. K., Kukkila, J., Vaddya, S. B., and Gingipalli, K. (2013). Recycling of materials used in dentistry with reference to its economical and environmental aspects. Int. J. Health Rehabil. Sci. 2, 140–145. doi:10.6049/kjjbydc.2016020160
Tripuraneni, S., and Namburi, S. (2008). Evaluation of genotoxicity of recycled Ni-Cr dental casting alloys: an in vitro study. J. Appl. Biomaterials Biomechanics 6, 47–54.
Wang, Y., Huang, H., Lin, H., Jiang, L., Pan, Y., Li, X., et al. (2020). The influence of recycling on the properties of interface between ceramic and dental alloys. BioMed Res. Int. 2020, 1–9. doi:10.1155/2020/3529781
Whitaker, R., Hernaez-Estrada, B., Hernandez, R. M., Santos-Vizcaino, E., and Spiller, K. L. (2021). Immunomodulatory biomaterials for tissue repair. Chem. Rev. 121, 11305–11335. doi:10.1021/acs.chemrev.0c00895
Wiśniewska, P., Haponiuk, J., Saeb, M. R., Rabiee, N., and Bencherif, S. A. (2023). Mitigating Metal-organic framework (MOF) toxicity for biomedical applications. Chem. Eng. J. 471, 144400. doi:10.1016/j.cej.2023.144400
Yadav, D., Garg, R. K., Ahlawat, A., and Chhabra, D. (2020). 3D printable biomaterials for orthopedic implants: solution for sustainable and circular economy. Resour. Policy 68, 101767. doi:10.1016/j.resourpol.2020.101767
Yazdi, M. K., Sajadi, S. M., Seidi, F., Rabiee, N., Fatahi, Y., Rabiee, M., et al. (2022). Clickable polysaccharides for biomedical applications: a comprehensive review. Prog. Polym. Sci. 133, 101590. doi:10.1016/j.progpolymsci.2022.101590
Yazdi, M. K., Vatanpour, V., Taghizadeh, A., Taghizadeh, M., Ganjali, M. R., Munir, M. T., et al. (2020). Hydrogel membranes: a review. Mater. Sci. Eng. 114, 111023. doi:10.1016/j.msec.2020.111023
Keywords: biomaterials, recycling, biowaste, sustainability, biomedical applications
Citation: Wiśniewska P, Saeb MR and Bencherif SA (2023) Biomaterials recycling: a promising pathway to sustainability. Front. Front. Biomater. Sci. 2:1260402. doi: 10.3389/fbiom.2023.1260402
Received: 17 July 2023; Accepted: 19 October 2023;
Published: 10 November 2023.
Edited by:
Virginia Brancato, University Milano Bicocca, ItalyReviewed by:
Jochen Salber, Ruhr-University Bochum, GermanySomasundaram Prasadh, Postdoctoral Research Associate/National Dental Center Singapore, Singapore
Copyright © 2023 Wiśniewska, Saeb and Bencherif. This is an open-access article distributed under the terms of the Creative Commons Attribution License (CC BY). The use, distribution or reproduction in other forums is permitted, provided the original author(s) and the copyright owner(s) are credited and that the original publication in this journal is cited, in accordance with accepted academic practice. No use, distribution or reproduction is permitted which does not comply with these terms.
*Correspondence: Mohammad Reza Saeb, mrsaeb2008@gmail.com; Sidi A. Bencherif, s.bencherif@northeastern.edu