An Appraisal Among Wired, Hybrid and Wireless Smart Homes to Mitigate Electromagnetic Radiation
- Architectural Engineering Department, College of Engineering, United Arab Emirates University, Al Ain, United Arab Emirates
The global Covid-19 pandemic caused a rapid transitioning to remote work settings, one likely to linger post-pandemic, resulting on people spending more time at home for work or study. The globalpandemic defined a new normal that is expected to be digital and heavily relying on technology. Smart buildings which are envisioned to be the next paradigm shift in the built environment are also foreseen as a response solution to aid in situations like pandemic. However, such a move yields benefits as well as risks, prompting wide debates on the priority to safeguard building occupants health, safety and well-being. Researchers, designers and engineers are seeking solutions to incorporate or modify design features in the indoor environment that prioritize the dwellers’ health and wellness. Though benefits of smart and IoT devices aid in monitoring health and wellness, radiation from these wireless devices may cause harm to human health, especially those with weaker health, as indicated by several research findings. Some of the negative impacts from wireless radiation include cell damage, cancer, tumor, change in hormonal levels, and neurological damage. Thus, this study seeks to determine the difference in radiation level inside a wired, hybrid and a wireless smart home through Computer Simulation Technology (CST) simulation. Such a quantification can help designers develop strategies to design smart buildings that cause low radiation for its occupants. Antenna field source was imported to CST to create the wireless and hybrid design scenario. The measurement for wired and hybrid were evaluated keeping the wired design as baseline. The results revealed that wireless produced 26.55% more radiation than wired scenario at 2.45 GHz, taken as baseline measurement. Further, the total Electromagnetic Radiation (EMR) and radiation patterns are dependent on several factors like proximity of IoT and smart devices to building walls and interior furnishings, frequency of operation. In order to create a safer indoor environment, this study recommends the use of both wired and hybrid design in lieu of totally wireless smart buildings.
Introduction
In general, people spend nearly 90% of their time indoors (American Society of Heating, Refrigerating and Air-Conditioning Engineers, 2011); now, with the global pandemic and shift towards the digital world, time spent in an indoor environment increased even more. This is a drastic change by which home and office border has blurred made people rely on smartphones and laptops to work effectively. Such an unprecedented change is believed to remain due to the onset of the fourth Industrial Revolution in which remote working, virtual learning, and virtual entertainment are the new norms (Petrillo et al., 2018; Peña-Cabrera et al., 2019). This trend is also visible in the new building design where Internet of Things (IoT) are used for creating virtual realities, security and surveillance (Minoli et al., 2017), health monitoring (Abdelgawad et al., 2016), while the fundamental prerequisite for all the aforementioned possibilities are rooted to ‘internet’ and its effective utilization in the general wellness of the community. However, similar to all previous industrial endeavors that affected the built environment, the virtual services, wireless IoT and smart devices also carry with them negative risks such as cyber-security problems, electromagnetic radiation (EMR),e-waste accumulation, and an increase in datacenters (Raveendran and Kheira, 2021). These worldwide threats to human health, particularly those generated from e-waste accumulation and the radiation from the extensive use of billions of high frequency IoT devices, require to be holistically addressed and realistic strategies determined (Atlam et al., 2018; Russell,2018).
Typically, an indoor environment such as a residential or commercial building is subjected to two types of EMR pollution, one from extremely low frequency (ELF) and the other from microwave and radio frequencies (Meyers, 2013). Electromagnetic Radiation (EMR) from the former occurs due to the operation of household appliances such as hairdryers, televisions (IARC Working Group on the Evaluation of Carcinogenic Risks toWorld Health Organizationand International Agency for Research on Cancer, 2002; Mark et al., 2015) and the latter are from wireless devices such as baby monitors, mobile phones, cordless phones and Bluetooth devices (Bhattacharjee, 2014). In a smart home or smart commercial building, the amount of exposure from EMR will be higher due to two plausible reasons, one, a multitude of smart or IoT devices will be connected, filling up the indoor space with excessive radiation (Atlam, Walters, and Wills, 2018) and, second, due to the high range of frequencies used for 5G (Russell 2018), that include millimeter frequency. Scientists and researchers believe both of these EMR sources cause health problems like dizziness, nausea (Al-Khlaiwi and Meo, 2004), gene (Chen et al., 2008) and DNA alteration (Panagopoulos, 2019), immunity issues (Gridley et al., 2006) besides cancer and brain tumor (Pakhomov et al., 1997; Hardell, 2017; Prasad et al., 2017). The World Health organization (WHO) declared that EMR produced from radiofrequency sources caused an increased risk occurrence of glioma (a type of brain tumor) and classified it as group 2B (probable carcinogenic) (IARC Working Group on the Evaluation of Carcinogenic Risks toWorld Health Organizationand International Agency for Research on Cancer, 2002). However, there is no consensus among the scientific or research community about the health problems from radiation thus it becomes necessary to deepen the studies (Pockett, 2018).
Moreover, the real problem with artificial radiation is more convoluted and often overlooked in most research investigations which do not consider that humans also have bio-energetic fields associated with them (Nbrega, 2006; Cifra and Pospíšil, 2014). Humans use a bio-electromagnetic frequency that utilizes bio-photos (Hossu and Rupert, 2006; Zhang et al., 2019) for numerous macro and microbiological processes. This finding was due to the advancement in quantum biology (Cifra, Fields, and Farhadi, 2011; Chaban et al., 2013; Farhadi, 2014) and the inference that essentially, humans use a natural frequency window of 0–30 Hz (Saliev et al., 2014; Hei et al., 2016; Choi et al., 2018). Cellular functions and tissue healing brain functions utilize this frequency range (Meyers, 2013; Saliev et al., 2014; Choi et al., 2018). Moreover, all materials, including building materials such as wood, concrete also have electromagnetic properties (Cuinas et al., 2000). The experiment by Kyoichi Nakagawa proved that people who lived away from natural magnetic and electric fields were affected by several ailments, including lethargy and dizziness (Meyers, 2013). Decades of research investigation also determined that natural frequencies are essential to human health and wellbeing (Becker, 1990), while artificial or man-made EMR sourced be it from 50 Hz or 50 GHz, is harmful (Panagopoulos and Chrousos, 2019).
As such, only a few researches investigated the EMR pollution inside a building as most of them focused and verified only signal loss path between the wireless devices (Ghassemzadeh et al., 2003a; Ghassemzadeh et al., 2003b; Tayebi et al., 2019). There are currently two research works published directly connecting buildings and radiation and both simulation works performed in CST used a plane wave excitation to study the distribution of EMR inside building space. However, both of these studies did not use real antenna source excitation nor interior furnishings to truly demonstrate the EMR inside the space (Vizi and Vandenbosch, 2016; Wahba et al., 2021).
This makes studies dedicated to computing and quantifying the EMR inside the indoor environment important as the radiation levels in smart buildings using 5G may become very high. Some of the strategies that examined reducing the radiation from wireless devices highlight that ‘wired’ smart indoor environment can be considered as a viable option instead of wireless to curb high-frequency radiation (Schulz et al., 2016; Clegg et al., 2020). Fundamentally, 99% of internet data transfer occurs via wired mode, and only 1% through satellite communications (Drew and Hopper, 2009; Kugler, 2019). Currently, wireless devices predominantly use 2.45 GHz, one of the frequencies covered under 4G LTE (Dahlman et al., 2013), but wireless internet connection is possible only for a few metres (Drew and Hopper, 2009), and hence, for a large building to get full signal coverage, Wi-Fi repeaters are required (ElShafee and Karim, 2012). Thus, the notion of having a complete wireless smart home or building is partially true.
Due to several health concerns, scientists and researchers advocate making the indoor environment wired for both smart devices and IoT devices (Clegg et al., 2020). To implement this concept, hybrid smart homes are proposed as a suitable option, with appropriate zoning for schools, universities, airports (Clegg et al., 2020) to reduce EMR. Research findings from the possible implementation of wired and wireless smart buildings can assist designers and architects in planning for a safer and smarter indoor space. Furthermore, clients and building end-users can be engaged at an earlier building design stage to take appropriate preventive measures to reduce overall radiation. However, research related to radiation and smart buildings remains scarce and, thus, forms the basis of this research study which seeks to explore the difference in total radiation levels in a smart indoor environment for three different scenarios: wired, hybrid and wireless at 2.45 GHz.
Methodology
The study seeks to determine EMR simulation inside indoor environment based on internet connectivity among wired, wireless and hybrid design scenarios to quantify the propagation of electric and magnetic fields. For this purpose, Computer Simulation Technology (CST) is employed, whose reliability in solving problems related to EMR has been proved by academicians and industrialists alike (Vizi and Vandenbosch, 2016). The research problem also requires 3 days modelling of the indoor environment and functioning of the modelled space with IoT devices (cisco packet tracer). Thus, the methodology consists of using four different software programs (cisco packet tracer, Sweethome 3D, CST and Antenna Magus) in juxtaposition to obtain the difference in the radiation levels in different indoor layouts of smart and IoT devices. Smart devices include smartphones, tablets and IoT devices are those that are ordinary devices transformed to collect data, make decision using artificial intelligence and internet connectivity. IoT devices can also be controlled by smart devices as per user’s needs. However, both use antennas for their functionality (Berte, 2018). The design comprises of three parts, firstly to design and visualize the different configurations of IoT and smart devices connected to a home gateway in cisco packet tracer. The intent behind such a process is to construct several scenarios for wired, hybrid and wireless with realistic algorithms used behind the smart design. The second part involved designing the model in sweethome 3D to model the interior layout with furnishings. Finally, these models are exported to CST and EMR is emulated for 2.45 GHz frequency in conjunction with Antenna Magus software.
Wood is selected as the building material (wall, floor, and ceiling) used for the indoor environment as it requires less mesh cells than concrete or glass, an essential condition to obtain a reliable accuracy with multiple IoT devices. The frequency used for simulation is 2.45 GHz, as the IoT devices use this ISM band, which is currently used for IoT devices. Moreover, the number of mesh cells and the accuracy of results are also tied to the simulation frequency. Thus, lowering the value of frequency reduces the number of mesh cells and overall simulation time.
In order to represent three design scenarios for EMR evaluation, the wireless and hybrid are directly computed using CST. EMR for the wired and hybrid (using some wired connections) is taken as zero (0) by using Shielded Twisted Pair (STP) Category 7 (CAT 7) or CAT 8, the preferred wired RJ-45 cable that produces almost 0 EMR (Sarma and Sarma, 2013) (also demonstrated in the validation section).
Fiber optics or STP is better than Unshielded Twisted Pair (UTP) in diminishing unwanted EMF (Mardiguian and Raimbourg, 2001). To differentiate the change in the overall EMR when smart devices are operated wirelessly and in wired mode, it is assumed that when all three devices are given simultaneous excitation, the resultant EMR produced from simulation equates to a wireless scenario.
Hence, when the number of devices operated wirelessly is one or two, in the simulation run, the other devices are presumed to be functioning via wired mode RJ-45 cable (Wani and Chaudhari, 2012) (RJ- 45 is an internet cable that can be used for multiple devices), thus aiding to estimate the increase in EMR. Even smartphones and tablets can be connected using appropriate RJ-connectors which allow the user to connect to the internet in wired mode.
A validation process was conducted to validate the reliability of the results from CST simulation and also to verify radiation from the wired device. Real measurements are taken in a real small concrete room and the size was kept small because, firstly, the model has to be reconstructed in CST to verify the results-a larger room would require high computational resources and time. Secondly, the only requirement for the experimental part was to validate and see the difference in results from EMF device and CST simulation results.
Modelling in “Sweet Home 3D”
An indoor environment depicting a one-bedroom apartment space is modelled in Sweet Home 3D, using wood as wall, flooring and ceiling material. The total size of the layout is 7 m × 5 m × 3 m, equivalent to a small living space of 35 m2. Small size was modelled to reduce the computational resources and get the reasonable accuracy required for electromagnetic simulation, used later in CST simulation. The interior space consists of an open space comprising of a kitchen, living room, and office space, and an en-suite bedroom. Twelve IoT and smart devices, of the most recurrently occurring ones, were selected, including one laptop and one smartphone, which is the control point of the IoT devices and can even be monitored remotely by the user. IoT devices used in the model are identical to those used in a real indoor environment. Table 1 summarizes the different IoT and smart devices connected to the home gateway. Figure 1 depict the model developed in Sweet Home 3D with furnishings.
Layout and Visualization in Cisco Packet Tracer
A general layout of a smart home can be visualized and designed in cisco packet tracer for the three scenarios. Cisco packet tracer helps designers verify the smart home design reliably by simulating several smart and IoT devices’ operation. In reality, any object or furniture can be transformed into a smart object by sensors and actuators. Moreover, a specific algorithm can be created for each device operation. For instance, the functioning of a thermostat can be set at a priorly specified temperature to determine when to turn it on/off, and it can be done using smartphone IoT monitor option (Alfarsi et al., 2019). Each interior space has been provided with 2–3 IoT devices totaling 12. Figure 2 depicts the design of a smart home connecting several smart and IoT devices to the smartphone and home gateway. While 7 of the IoT devices are connected wirelessly to the home gateway, other devices are connected through ethernet cable, thus operating in wired mode.
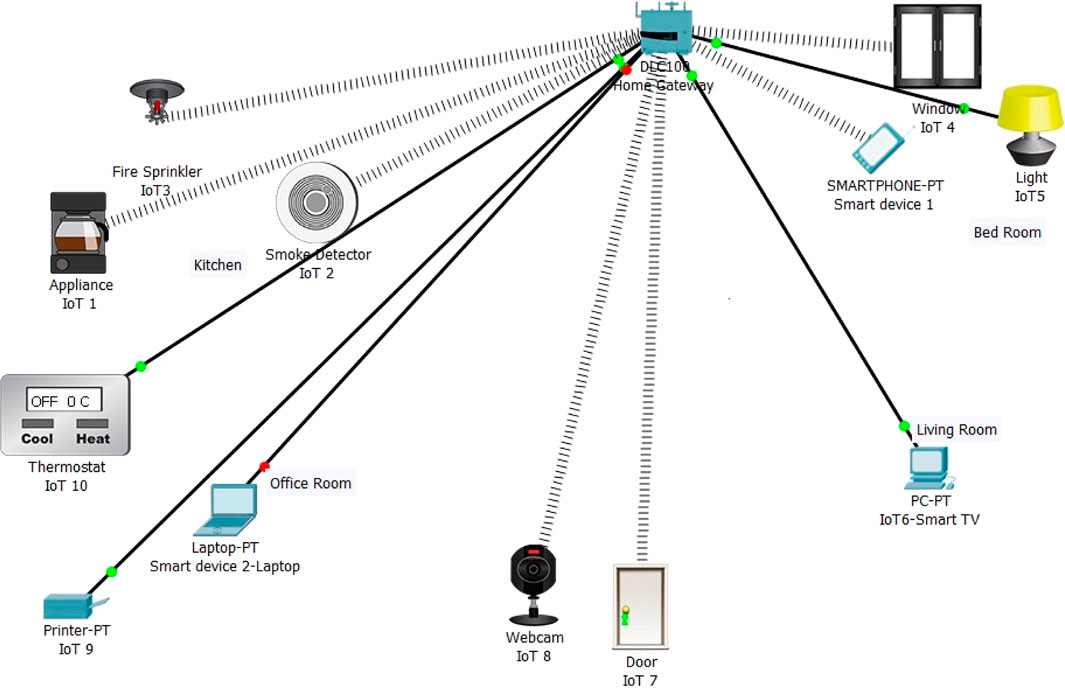
FIGURE 2. Configuration of IoT and smart devices in Cisco packet tracer connected to home gateway for the simulated model.
Further, using a smartphone, specific algorithms can be set and modified any time. Figure 3 exhibits the algorithm to activate the process of locking and unlocking the main door is automated by sensing the occupant through the security camera. Apart from this, users can also program using python scripts to control them via remote server instead of a home gateway (Sihombing et al., 2018).
Numerical Simulation in CST
CST is a viable option to get reliable results for problems related to electromagnetism as proven by both academia and industry (Vizi and Vandenbosch, 2016).
Time domain solver is used by CST to solve high frequency problems related to antennas, filters and is more accurate than frequency solver. It implements Finite Integration Technique (FIT) or transient solver to compute Maxwell’s equation on each of the hexahedral meshes. Maxwells’ equation are generally represented in partial differential equations and form the basis of classical electromagnetism (CST studio suite Manual, 2020). One of the significant consequence of Maxwell’s equation is that it demonstrates the fluctuating electric and magnetic fields propagating at the speed of light. This equation have universal applicability and different version of this equation is used for solving different problems. This numerical method uses an universal spatial discretizational scheme for the electromagnetic problem that is solved in time or frequency domain (CST manual, 2020). FIT also utilizes the integral form of Maxwell’s equation rather than the differential one. Numerical simulations are initiated by developing the finite calculation domain that encloses the application problem (This is followed by meshing, either automatic or manual (CST manual, 2020). CST uses perfect Boundary Approximation (PBA) to discretize the mesh cells to evaluate the necessary parameters like E-field, power density. Finally, Maxwells’ equation are performed on each of the cells till a suitable accuracy is reached.
Maxwell’equation in integral form:
Where, E is the electric field, H is the magnetic field, J is the electric current density, D and B are electric and magnetic flux densities respectively.
Determination of EMR in CST
Due to the requirement of a lower number of mesh cells, wood is provided as the material for ceiling, flooring and walls. It has also been shown from a research study that at lower frequency (2.45 GHz) that total EMR produced by different common building materials like concrete, wood and glass is nearly the same though the rate of dissipation may vary. Besides, wood is the preferred sustainable and eco-friendly material compared to concrete. A rectangular edge fed patch antenna is initially designed in a separate software called antenna magus to provide a wireless internet connection inside this smart indoor environment. The antenna is then run in CST to extract the field source which is used as an excitation source for IoT devices. Hence, different field sources are provided for the wireless and hybrid modes to depict the radiation from these wireless IoT devices. Figure 4 shows the power radiated from antenna at 30 dBm.
EMR can be measured at any point or even at a plane inside the interior space; however, to depict a realistic situation, measurements are taken at points where the occupant is likely to spend long hours in the indoor environment, one such point in the office room and the other in the bedroom as shown in Figure 5. For the wired scenario, no measurement is made inside the simulation space as it is assumed that a shielded ethernet wiring (CAT 7) do not cause measurable EMR in the space (Sarma and Sarma, 2013) which is later verified in the validation section below. Thus, for the simulation, devices without wireless field sourced excitation are presumed to be working in a wired mode in each scenario. Moreover, for the simulation simulataneous excitation is chosen, but in reality the EMR generated will be lower due to intermittent working of the devices.
Validation
Validation was conducted for checking the result of wireless and wired scenario tested in a real concrete room.
Figure 6 (A) shows the room plan and the smartphone device used to check the generated EMF using wintact WT3121 instrument (Figure 7).
1) Smartphone operating in wireless mode; the electric and magnetic field measured at 15 cm from the device was 26 V/m and 0.05 A/m, and the electric and magnetic field measured using CST were 27.769 V/m and 0.068 A/m respectively.
2) Smartphone operating in wired mode; to measure the EMF in wired mode, CAT 7 STP ethernet RJ-45 wire is used to operate the laptop in wired internet mode. Initially, the electric field read 4 V/m for video streaming; after a few seconds, it reduced to 0 and maintained zero electric field while magnetic field value was 0 from the beginning (Figure 6B).
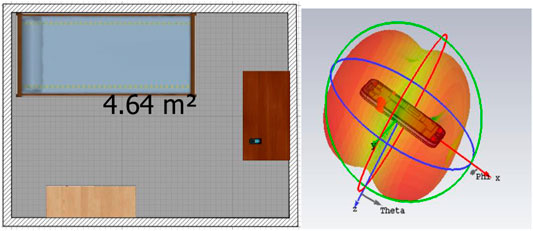
FIGURE 6. (A) Plan of real room where actual EMR measurements were taken (B) Far-field pattern of the smartphone designed in CST.
Results and Discussion
The visualization and value of electric field distribution varied as more devices were added to the simulation to run in wireless mode. While Figure 8 depicts the E-field distribution for hybrid design (with 5 devices operating wirelessly and the other devices operating via wired mode), Figure 9 shows the more intense radiation distribution when all devices are operated wirelessly. In both figures, the ceiling was used for simulation, but hidden in the figures for better visualization. Table 2 indicates the summary results of maximum E-field, maximum H-field, far-field directivity and E-field at measuring points where the occupant is likely to spend more time.
The E-field accumulation slowly builds up as wireless devices are added in the simulation one by one (Figure 10). The maximum E-field resulted in a 26.5% increase when the number of devices increased from one (109.12 V/m) to twelve (138.101 V/m) (from Table 2). However, E-field values remained constant at P1 and P2 for different hybrid setups; for instance, E-field at P1 is the same for hybrid scenarios using 6–10 IoT devices. Similarly, E-field at P2 is very low till Iot-10 (printer) is added to the simulation domain and reaches a maximum value of 9.725 V/m for the scenario where all devices are operating wirelessly. The non-variance of E-field value is related to the distance between the IoT device and the measuring point far away from the device. The increase in the value of E-field for P1 and P2 is observed when more number of IoT and smart devices are kept close to it, thus increasing the exposure. However, it is imperative to note that though the measuring points only recorded a maximum value of 9.725 V/m, if the occupant is very close as in the case of holding the mobile phone or working with the laptop, the value then increases to around 26 V/m.
Maximum E-field in the solution domain initially peaks up and steadily increases as wireless devices are added to the simulation until the seventh device (Figure 11). Afterwards, it slowly decreases until the last two devices are added to the scenario. This is due to the position of the seventh (main door) and eighth (security camera) wireless device that are closer to the wall, thereby dissipating the radiation energy through the wall. The maximum value increases again with the introduction the 10th device (thermostat) and finally reaching the maximum value of 138.164 V/m. Thus, it can be seen that the EMR inside the indoor environment is dependent on factors such as electromagnetic properties of interior and building materials, the position of the wireless device, proximity of the device and the occupant and the number of devices and also the frequency. Maximum H-field values are almost constant for all simulation runs, excluding wired scenario.
As in the case with maximum E-field, the far-field directivity also depicted a similar trend, initially rising and slowly dropping and finally peaking up with the addition of the last wireless device. The reason is also similar as noticed with the variation in E-field, as more wireless devices were added to the simulation scenario at different positions, especially those placed closer to the wall, back-scattering from the wall occurred, which made the directivity value drop slightly. Figure 12 reveals the shape of far-field directivity or the overall radiation pattern for hybrid (2 wireless) and wireless scenarios (all 12 devices). The radiation pattern of Figure 13 depicts the intense radiation compared to Figure 12 which has fewer operating devices.
Recommendations and Further Research Directions
All the simulation runs were performed with 2.45 GHz, and thus the EMR generated is small compared to the higher frequency range. Nonetheless, exposure from 2.45 GHz is also linked to health impairments (Al-Khlaiwi and Meo, 2004) and especially with the instruction of the governing authorities to increase the frequency to 28 and 58 GHz, the proliferation of EMR inside the smart indoor environment will be even more important. Since the wired simulation scenario using STP CAT 7 revealed almost zero EMR (validation section), it will be a viable option to consider while designing the indoor space. This is particularly a valid option for the spaces where an occupant spends long hours such as sleeping or working in an office. These spaces will benefit from an integrated wired option that enables the user to select it. Also, whenever wireless IoT and smart devices need to be operated, hybrid design can be provided with appropriate zoning separating both wired and wireless indoor spaces, as the simulation reveals for even comparatively low frequency of 2.45 GHz, radiation zone occurs in close proximity to the wireless device and drops significantly as distance increases as per inverse squarw law of far-field antenna radiation (Voudoukis and Oikonomidis, 2017). Using shielded cables and early design intervention to reduce the EMR in a building space, an appropriate selection of building materials that can effectively reduce radiation transmission can also be considered as a vital choice (Panagopoulos and Chrousos, 2019).
Even the shielded ethernet wires can be designed to be placed inside the walls instead of outside, reducing EMR further. Besides, special attention to design can be provided to counteract the radiation produced internally and externally as per the shielding effectiveness of building materials (Panagopoulos and Chrousos, 2019)
Further research directions can include using different materials for walls, floor and ceilings with different building shape and size. Similarly, higher frequency can be tested with multiple occupants to understand the increase and distribution of radiation inside smart buildings.
Conclusion
The rapid digital transformation occurring in the built environment, exacerbated by the pandemic crisis, has forced people to spend more time indoors, relying more on internet-based devices for work and entertainment. However, there are health concerns regarding the harmful EMR from artificial sources such as a wireless device that the occupant may be using for long hours. 5G revolution is proposing to use higher frequencies to connect billions of IoT devices may pose a health threat. In an effort to quantify and compare the radiation levels present inside a smart indoor environment using 2.45 GHz, this research study designed three scenarios-wired, hybrid and wireless using cisco packet tracer, Sweet home 3D and CST. It was found that while an STP based ethernet connectivity provides zero EMR, hybrid and wireless do cause EMR. The overall EMR produced is dependent on factors such as the proximity of occupant and the device, the indoor material and furnishings, frequency of operation. Further, it was observed that far-field and EMR generated may decrease depending on the back reflection from the wall or other interior material, and do not follow a direct proportionality relation to the number of the device itself. The study recommends applying options for hybrid or wired smart and IoT devices, especially in home zones where the occupants are likely to spend long hours, as an integrated early decisions with architects and designers to provide a safer and smarter building.
Data Availability Statement
The original contributions presented in the study are included in the article, further inquiries can be directed to the corresponding author.
Author Contributions
Conceptualization, RR and KA; methodology, RR and KA; writing—original draft preparation, RR; writing—review and editing, KA visualization, RR and KA; funding acquisition, KA. All authors have read and agreed to the published version of the manuscript.
Funding
This research was funded by the National Water and Energy Center at the United Arab Emirates University, Grant Number 31R102.
Conflict of Interest
The authors declare that the research was conducted in the absence of any commercial or financial relationships that could be construed as a potential conflict of interest.
Publisher’s Note
All claims expressed in this article are solely those of the authors and do not necessarily represent those of their affiliated organizations, or those of the publisher, the editors and the reviewers. Any product that may be evaluated in this article, or claim that may be made by its manufacturer, is not guaranteed or endorsed by the publisher.
References
Abdelgawad, A., Kumar, Y., and Ahmed, K. (2016). “IoT-Based Health Monitoring System for Active and Assisted Living,” in International Conference on Smart Objects and Technologies for Social Good (Springer), 11–20.
Al-Khlaiwi, T., and Meo, S. A. (2004). Association of Mobile Phone Radiation with Fatigue, Headache, Dizziness, Tension and Sleep Disturbance in Saudi Population. Saudi Med. J. 25 (6), 732–736.
Alfarsi, G., Jabbar, J., Tawafak, R. M., Malik, S. I., Alsidiri, A., and Alsinani, M. (2019). Using Cisco Packet Tracer to Simulate Smart Home. Int. J. Eng. Res. Technology. 8 (12), 670–674. doi:10.30935/cedtech/10879
American Society of Heating, Refrigerating and Air-Conditioning Engineers (2011). ASHRAE Guideline 10-2011, Interactions Affecting the Achievement of Acceptable Indoor Environments. ASHRAE.
Atlam, H. F., Walters, R. J., and Wills, G. B. (2018). “Intelligence of Things: Opportunities & Challenges,” in 2018 3rd Cloudification of the Internet of Things (CIoT) (IEEE), 1–6. doi:10.1109/ciot.2018.8627114
Becker, R. O. (1990). Cross Currents: The Startling Effects Of Electro-Magnetic Radiation On Your Health. Bloomsbury.
Berte, D.-R. (2018). Defining the Iot. Proc. Int. Conf. Business Excell. 12 (1), 118–128. doi:10.2478/picbe-2018-0013
Bhattacharjee, S. (2014). Protective Measures to Minimize the Electromagnetic Radiation. Electron. Electric Eng. 4 (4), 375–380.
Chaban, V. V., Cho, T., Reid, C. B., and Norris, K. C. (2013). Physically Disconnected Non-Diffusible Cell-To-Cell Communication between Neuroblastoma SH-Sy5y and DRG Primary Sensory Neurons. Am. J. Transl Res. 5 (1), 69–79.
Chen, Q., Lu, D. Q., Jiang, H., and Xu, Z. P. (2008). Effects of Millimeter Wave on Gene Expression in Human Keratinocytes. Zhejiang Da Xue Xue Bao Yi Xue BanMedical Sci. 37 (1), 23–28.
Choi, H. M. C., CheingCheing, A. K. K., Ng, G. Y. F., and CheingCheing, G. L. Y. (2018). Effects of Pulsed Electromagnetic Field (PEMF) on the Tensile Biomechanical Properties of Diabetic Wounds at Different Phases of Healing. PLoS One. 13 (1), e0191074. doi:10.1371/journal.pone.0191074
Cifra, M., FieldsFields, J. Z., and Farhadi, A. (2011). Electromagnetic Cellular Interactions. Prog. Biophys. Mol. Biol. 105 (3), 223–246. doi:10.1016/j.pbiomolbio.2010.07.003
Cifra, M., and Pospíšil, P. (2014). Ultra-Weak Photon Emission from Biological Samples: Definition, Mechanisms, Properties, Detection and Applications. J. Photochem. Photobiol. B: Biol. 139, 2–10. doi:10.1016/j.jphotobiol.2014.02.009
Clegg, F. M., Sears, M., Friesen, M., Scarato, T., Metzinger, R., Russell, C., et al. (2020). Building Science and Radiofrequency Radiation: What Makes Smart and Healthy Buildings. Building Environ. 176, 106324. doi:10.1016/j.buildenv.2019.106324
Cuinas, I., Pugliese, J-P., Hammoudeh, A., and Garcia Sanchez, M. (2000). “Comparison of the Electromagnetic Properties of Building Materials at 5.8 GHz and 62.4 GHz,” in In Vehicular Technology Conference Fall 2000. IEEE VTS Fall VTC2000. 52nd Vehicular Technology Conference (Cat. No. 00CH37152) (IEEE), 780–785.
Dahlman, E., Parkvall, S., and Skold, J. (2013). 4G: LTE/LTE-Advanced for Mobile Broadband. Paris, France: Academic Press.
Drew, S. C., and Hopper, A. G. (2009). Fishing and Submarine Cables Working Together. The International Cable Protection. Committee Second Edi (Catch fish, not cables!).
ElShafee, A., and Karim, A. H. (2012). Design and Implementation of a WIFI Based Home Automation System. World Acad. Sci. Eng. Technology. 68 363, 2177–2183.
Farhadi, A. (2014). Non-Chemical Distant Cellular Interactions as a Potential Confounder of Cell Biology Experiments. Front. Physiol. 5, 405. doi:10.3389/fphys.2014.00405
Ghassemzadeh, S. S., Greenstein, L. J., Kavcic, A., Sveinsson, T., and Tarokh, V. (2003a). “UWB Indoor Path Loss Model for Residential and Commercial Buildings,” in 2003 IEEE 58th Vehicular Technology Conference. VTC 2003-Fall (IEEE Cat. No. 03CH37484) (IEEE), 3115–3119. doi:10.1109/vetecf.2003.1286197
Ghassemzadeh, S. S., Greenstein, L. J., Kavcic, A., Sveinsson, T., and Tarokh, V. (2003b). An Empirical Indoor Path Loss Model for Ultra-wideband Channels. J. Commun. Netw. 5 (4), 303–308. doi:10.1109/jcn.2003.6596612
Gridley, D. S., Dutta-Roy, R., Andres, M. L., Nelson, G. A., and Pecaut, M. J. (2006). Acute Effects of Iron-Particle Radiation on Immunity. Part II: Leukocyte Activation, Cytokines and Adhesion. Radiat. Res. 165 (1), 78–87. doi:10.1667/rr3490.1
Hardell, L. (2017). World Health Organization, Radiofrequency Radiation and Health - A Hard Nut to Crack (Review). Int. J. Oncol. 51 (2), 405–413. doi:10.3892/ijo.2017.4046
Hei, W. H., Byun, S. H., Kim, J. S., Kim, S., Seo, Y. K., Park, J. C., et al. (2016). Effects of Electromagnetic Field (PEMF) Exposure at Different Frequency and Duration on the Peripheral Nerve Regeneration: In Vitro and In Vivo Study. Int. J. Neurosci. 126 (8), 739–748. doi:10.3109/00207454.2015.1054032
Hossu, M., and Rupert, R. (2006). Quantum Events of Biophoton Emission Associated with Complementary and Alternative Medicine Therapies: A Descriptive Pilot Study. J. Altern. Complement. Med. 12 (2), 119–124. doi:10.1089/acm.2006.12.119
IARC Working Group on the Evaluation of Carcinogenic Risks to, World Health Organization, and International Agency for Research on Cancer (2002). Non-Ionizing Radiation: Static and Extremely Low-Frequency (ELF) Electric and Magnetic Fields.
Mardiguian, M., and Raimbourg, J. (2001). “Shielded (STP) versus Unshielded (UTP) Twisted Pairs: An EMC Comparison,” in 2001 IEEE EMC International Symposium. Symposium Record. International Symposium on Electromagnetic Compatibility (Cat. No. 01CH37161) (IEEE), 43–46.
Mark, M., Vermeulen, R., Nijssen, P. C. G., Mulleners, W. M., Sas, A. M. G., van Laar, T., et al. (2015). Extremely Low-Frequency Magnetic Field Exposure, Electrical Shocks and Risk of Parkinson’s Disease. Int. Arch. Occup. Environ. Health 88 (2), 227–234. doi:10.1007/s00420-014-0949-2
Meyers, B. A. (2013). Pemf-the Fifth Element Of Health: Learn Why Pulsed Electromagnetic Field (Pemf) Therapy Supercharges Your Health like Nothing Else! BalboaPress.
Minoli, D., Sohraby, K., and Occhiogrosso, B. (2017). IoT Considerations, Requirements, and Architectures for Smart Buildings-Energy Optimization and Next-Generation Building Management Systems. IEEE Internet Things J. 4 (1), 269–283. doi:10.1109/JIOT.2017.2647881
Nbrega, C. (2006). Biophoton the Language of the Cells: What Can Living Systems Tell Us about Interaction? Technoetic Arts 4 (3), 193–201. doi:10.1386/tear.4.3.193/1
Pakhomov, A. G., Howard, K., Mathur, S. P., Akyel, Y., and Campbell, C. B. G.. (1997). Role of Field Intensity in the Biological Effectiveness of Millimeter Waves at a Resonance Frequency. Bioelectrochemistry Bioenerg. 43 (1): 27–33. doi:10.1016/S0302-4598(97)00022-6
Panagopoulos, D. J., and Chrousos, G. P. (2019). Shielding Methods and Products against Man-Made Electromagnetic Fields: Protection versus Risk. Sci. Total Environ. 667, 255–262. doi:10.1016/j.scitotenv.2019.02.344
Panagopoulos, D. J. (2019). Comparing DNA Damage Induced by Mobile Telephony and Other Types of Man-Made Electromagnetic Fields. Mutat. Research/Reviews Mutat. Res. 781, 53–62. doi:10.1016/j.mrrev.2019.03.003
Peña-Cabrera, M., Lomas, V., and Lefranc, G. (2019). “Fourth Industrial Revolution and its Impact on Society,” . in 2019 IEEE CHILEAN Conference on Electrical, Electronics Engineering, Information and Communication Technologies (CHILECON) (IEEE), 1–6.
Petrillo, A., De Felice, F., Cioffi, R., and Zomparelli, F. (2018). Fourth Industrial Revolution: Current Practices, Challenges, and Opportunities. Digital Transformation in Smart Manufacturing., 1–20. doi:10.5772/intechopen.72304
Pockett, S. (2018). Public Health and the Radio Frequency Radiation Emitted by Cellphone Technology, Smart Meters and WiFi. New Zealand Med. J. (Online). 131 (1487), 97.
Prasad, M., Kathuria, P., Nair, P., Amit, K., and Prasad, K. (2017). Mobile Phone Use and Risk of Brain Tumours: A Systematic Review of Association between Study Quality, Source of Funding, and Research Outcomes. Neurol. Sci. : Official J. Ital. Neurol. Soc. Ital. Soc. Clin. Neurophysiol. 38 (5), 797–810. doi:10.1007/s10072-017-2850-8
Raveendran, R., and Kheira, A. (2021). A Meta-Integrative Qualitative Study on the Hidden Threats of Smart Buildings/Cities and Their Associated Impacts on Humans and the Environment. Buildings. 11 (6), 251. doi:10.3390/buildings11060251
Russell, C. L. (2018). 5 G Wireless Telecommunications Expansion: Public Health and Environmental Implications. Environ. Res. 165 (April), 484–495. doi:10.1016/j.envres.2018.01.016
Saliev, T., Mustapova, Z., Kulsharova, G., Bulanin, D., and Mikhalovsky, S. (2014). Therapeutic Potential of Electromagnetic Fields for Tissue Engineering and Wound Healing. Cell Prolif. 47 (6), 485–493. doi:10.1111/cpr.12142
Sarma, M., and Sarma, S. K. (2013). “Effect of Ac Power Line on Utp cable: A Review,” in 2013 4th International Conference on Intelligent Systems, Modelling and Simulation, 549–552. doi:10.1109/isms.2013.79
Schulz, M., Klapper, P., Hollick, M., Tews, E., and Katzenbeisser, S. (2016). “Trust the Wire, They Always Told Me! on Practical Non-Destructive Wire-Tap Attacks against Ethernet,” in Proceedings of the 9th ACM Conference on Security & Privacy in Wireless and Mobile Networks, 43–48.
Sihombing, O., Zendrato, N., Yonata, L., Nababan, M., Sitanggang, D., Purba, W., et al. (2018). “Smart Home Design for Electronic Devices Monitoring Based Wireless Gateway Network Using Cisco Packet Tracer,” in Journal of Physics: Conference Series (IOP Publishing), 12021. doi:10.1088/1742-6596/1007/1/012021
Tayebi, A., Gomez, J., and Gutierrez, O. (2019). Development of a Web-Based Simulation Tool to Estimate the Path Loss in Outdoor Environments Using Openstreetmaps [Wireless Corner]. IEEE Antennas Propagation Mag. 61 (1), 123–129. doi:10.1109/map.2018.2883088
Vizi, N., and Vandenbosch, G. A. E. (2016). Building Materials and Electromagnetic Radiation: The Role of Material and Shape. J. Building Eng. 5, 96–103. doi:10.1016/j.jobe.2015.11.010
Voudoukis, N., and Oikonomidis, S. (2017). Inverse Square Law for Light and Radiation: A Unifying Educational Approach. Eur. J. Eng. Technology Res. 2 (11), 23–27. doi:10.24018/ejers.2017.2.11.517
Wahba, A. S., Elboushi, A., El-Sherbiny, Y. M., and El-Shazly, M. (2021). Electromagnetic Energy in Buildings: Analysis of the Effect of Roof Shape and Treated Materials. J. Building Perform. Simulatio 14 (5), 536–553. doi:10.1080/19401493.2021.1976276
Wani, S. A., and Chaudhari, R. P. (2012). “Ethernet Enabled Digital I/O Control in Embedded Systems,” in 2012 International Conference on Computing, Electronics and Electrical Technologies (ICCEET) (IEEE), 634–637. doi:10.1109/icceet.2012.6203907
Keywords: Internet of Things (IoT), indoor enviroment, electromagnetic radiation, human health, computer simulation technology
Citation: Raveendran R and Tabet Aoul KA (2022) An Appraisal Among Wired, Hybrid and Wireless Smart Homes to Mitigate Electromagnetic Radiation. Front. Built Environ. 7:764295. doi: 10.3389/fbuil.2021.764295
Received: 25 August 2021; Accepted: 17 December 2021;
Published: 28 March 2022.
Edited by:
Sahar Zahiri, Oxford Brookes University, United KingdomReviewed by:
Ana Karina Silverio, University of the West of England, United KingdomGaetano Licitra, Agenzia Regionale per la Protezione Ambientale della Toscana (ARPAT), Italy
Copyright © 2022 Raveendran and Tabet Aoul. This is an open-access article distributed under the terms of the Creative Commons Attribution License (CC BY). The use, distribution or reproduction in other forums is permitted, provided the original author(s) and the copyright owner(s) are credited and that the original publication in this journal is cited, in accordance with accepted academic practice. No use, distribution or reproduction is permitted which does not comply with these terms.
*Correspondence: Kheira Anissa Tabet Aoul, kheira.anissa@uaeu.ac.ae