Building thermal resilience framework (BTRF): A novel framework to address the challenge of extreme thermal events, arising from climate change
- Division of Sustainable Development, College of Science and Engineering, Hamad Bin Khalifa University, Qatar Foundation, Doha, Qatar
Over the past 2 decades, many parts of the world have experienced unprecedented record-breaking temperatures; these extremes fall on both ends of the temperature spectrum ranging from excessively hot to freezing low. Moreover, recently, the rate and the impacts of these extremes have increased, despite all the mitigation efforts, necessitating a resilience-based approach to address these challenges stemming from the accelerated global warming and the advent of climate change. Examples of such extremes include the 2003 and 2022 heatwaves in Europe, claiming approximately 4,000 and 12,000 lives, respectively as well as the 2021 heatwave in the Pacific Northwest region of North America and the deep freeze in Southeast Texas. In this paper, we reflect on previous studies, identifying both internal and external aspects that contribute to a building’s thermal performance. We then incorporate these factors into a proposed framework, covering the important phases of a building’s life cycle, to reflect its thermal resilience. During each phase, an associated Building Thermal Resilience Profile (BTRP), taken from accumulated data of previous phases, provides the needed assessment of the building, and is regularly adapted to changes in the building and its surroundings. BTRP will be a valuable tool for the resilience evaluation of different design options. Furthermore, during the operation phase, it will contribute to real-time monitoring and assessment, facilitating disaster management and response, at both the buildings and city scale, reducing the causalities of extreme events. Thus, the BTRF has the potential to expand into various fields such as healthcare, green and resilient buildings rating systems, and even to improve the municipal regulations. Nevertheless, the prime aim of this paper is to address the challenge of extreme thermal events, arising from climate change, and pave the way for the adoption of effective thermal resilience in building design and operation practices.
Introduction
Since the start of the new millennium, the world has witnessed a drastic increase in the impacts and rates of natural hazards attributed to climate change and accelerated urbanization. During the past twenty years, the world has witnessed several devastating natural disasters that have brought far greater damage than in previous years; this trend is set to increase due to global warming, climate change, and rapid urbanization (Salimi and Al-Ghamdi, 2020; Tahir et al., 2021; Chen et al., 2022; Ma et al., 2022). The impact of climate change on the world includes a wide spectrum of natural disasters, such as unusual precipitation (in arid areas) and unusual extreme heatwaves, accompanied by record-breaking high temperatures (Tahir et al., 2021), (Attia et al., 2021), (García-Herrera et al., 2010). Generally, the global warming is considered as one aspect of climate change (NASA, 2022), (U.S. Geological Survey, 2022). The increase in heatwaves rate and impact is particularly associated with global warming; as such, increased emissions, as demonstrated in several simulated scenarios, are expected to elevate the temperatures globally and prolong the excessive-heat periods, thus increasing the mortality rates and other destructive impacts to the built environment associated with them (Chen et al., 2022), (Perkins-Kirkpatrick and Lewis, 2020). For example, in 2003 and 2022, despite all government precautions and efforts, Europe witnessed extreme heatwaves that claimed the lives of around 4,000 and 12,000 people, respectively (García-Herrera et al., 2010). These continuing extreme climate events have affected various development plans, and threaten the success of international sports events, like the upcoming FIFA World Cup 2022 in Qatar, that pushed organizers to delay the event by several months over concerns regarding the health of the athletes (Serdar et al., 2021a), (Serdar and Al-Ghamdi, 2021). Furthermore, the world is suffering from unusual extremes in temperature from both ends of the temperature spectrum, presenting increasingly threatening and deadly challenges. For example, in 2021, the Pacific Northwest region of North America experienced a devastating heatwave, while in the same year, Southeast Texas experienced a deep freeze; together, these unprecedented events, claimed approximately 2,000 lives at a staggering cost of USD$200 billion (Busby et al., 2021), (Schiermeier, 2021). Moreover, it is important to realize that such events increase electrical supply demand to excessive levels. In turn, this leads to failures and prolonged outages, increasing the damage of the disasters (Attia et al., 2021), (Serdar et al., 2021b; Busby et al., 2021; Schiermeier, 2021). The severity, and cost of these events, provides evidence of the limitations in using a conventional thermal design approach with respect to buildings, and highlights the need to adopt a resilience-based approach to face this existential threat, even for the world’s most advanced countries.
Resilience is a relatively new and vague concept and is typically reflected through a balance between several qualities and capacities of the described system. The concept of resilience is relatively new in engineering fields; thus, it is sometimes represented through various definitions affected by the assessment method and threats under investigation (Hosseini et al., 2016; Serdar et al., 2022a; Serdar et al., 2022b). However, the general concept of resilience revolves around a system’s ability to absorb a disturbance and recover from it swiftly, which is interconnected with various qualities of a system, such as robustness, and vulnerabilities (Serdar et al., 2022a), (Al-Humaiqani and Al-Ghamdi, 2022), (Faturechi and Miller-Hooks, 2015). Furthermore, in the literature, these qualities are related to the thermal resilience of a building, through a group of capacities, such as absorptive, adaptive, and restorative, in addition to recovery rates, readers can expand on the thermal resilience, especially during heatwaves, by referring to (Attia et al., 2021), (Zhang et al., 2021). However, in this paper, we will consider the building’s thermal resilience to be its capacity to accommodate thermal demands and preserve the lives of its occupants during thermal stresses caused by disturbances, such as heatwaves, blizzards, and power outages.
Nevertheless, the resilience qualities and capacities can be reflected in the performance representation of a system by plotting it against time, which facilitates quantifying the resilience of a system through mathematical integration, as suggested by Bruneau et al. (2003) and later adopted by many researchers (Serdar et al., 2022a), (Faturechi and Miller-Hooks, 2015). Recent reviews, of the published literature, show limited progress has been achieved in the field of thermal resilience of buildings, with the majority of efforts focused on evaluating the contribution of cooling strategies against heatwaves and power outages, using a qualitative approach (Attia et al., 2021), (Zhang et al., 2021), (Rahif et al., 2022; mailto Attia et al., 2021; Homaei and Hamdy, 2021). Moreover, some researchers focused on the threat of elevated temperature to the urban built environment within the context of “SLow-Onset Disasters (SLOD)” which also includes air pollution (Blanco Cadena et al., 1308), (Blanco Cadena et al., 1186). Additionally, some researchers have attempted to evaluate the balance between sustainability and resilience considerations in buildings, while adhering to green building rating system requirements, and highlighted the need to avoid achieving energy savings on the expanse of a building’s resilience (Sun et al., 2020; Gholami Rostam and Abbasi, 2021; Abedrabboh et al., 2022).
However, throughout the literature, we can observe several approaches to improve the performance resilience of a building and subsequently its resilience, whether it is passive, active, or related to the building’s surroundings (Zhang et al., 2021), (De Wit, 1997; Zhivov, 2021; Schünemann et al., 2022). Some approaches focus on the building design, starting from the building’s site characteristics, as a building’s location and orientation can increase its thermal load (Azarnejhad and Mahdavi, 2019; Felicioni et al., 2020; Schünemann et al., 2022). For example, a study indicates that the heat island effect can create a temperature difference as high as 11°C during a heatwave (Hong et al., 2021). Moreover, urban planning best practices, such as providing open urban areas, can improve thermal resilience, even against future climate changes (Gherri et al., 2021), (D’Amico et al., 2021). Additionally, the choice of building and insulation materials can improve thermal stability and comfort. For example, utilizing Phase Changing Material (PCM) as thermal energy storage, can protect against rapid temperature changes, by ensuring the comfort of occupants, during prolonged outages (Kudabayev et al., 2022). Building design, that aims to improve natural ventilation potential and integrate thermal mass into a building, contributes significantly to its thermal resilience during power outages, but its efficiency differs depending on if the weather is hot or cold (Heracleous et al., 2021; Su et al., 2022; Zeng et al., 2022). During operation, occupant behavior and occupancy rates can affect thermal load, thus, opening the way for demand-side management practices (Schünemann et al., 2021). Electrical load shedding, during disasters, can also play an important role in managing available resources and protecting vulnerable communities (Zeng et al., 2022), (Wang et al., 1088). Despite all efforts to evaluate different conditioning strategies, on the thermal performance of buildings, a holistic evaluation approach, regarding building thermal resilience, is still lacking.
In this paper, we suggest a framework, which spans across different phases in the life cycle of a building, to assess a building’s thermal resilience. This framework allows for different factors, which affect thermal performance capacities and qualities, to be considered. Moreover, this framework facilitates quantifying a building’s thermal resilience. This innovative framework permits different design options to be compared, during the design phase, as well as allows for the application of smart home and city concepts, facilitating disaster management, planning, and response efforts to save the lives of occupants.
Methods and discussion
To determine a building’s thermal resilience, we need to deconstruct the challenge into several steps and components, as many factors can affect the overall performance of a building. The thermal performance of any building is related to several external and internal factors. External factors, such as climate, power supply, expected downtime, location, and orientation, can radically change the level of required thermal loads, and increase direct solar heat gain, exposing a building to the heat island effect (mailto Attia et al., 2021), (Felicioni et al., 2020). Other external factors such as occupancy schedule, types of clothing, cold beverage consumption, and thermal experience, can be attributed to the perception and culture of the occupants, significantly changing both thermal comfort and thermal demand (mailto Attia et al., 2021), (Schünemann et al., 2021), (Aljawabra and Nikolopoulou, 2018). Internal factors, such as envelop materials, natural ventilation potential, HVAC systems, and integrated thermal mass, are related to a building’s design and its systems (Zhang et al., 2021).
To ensure the effectiveness of the evaluation of a building’s thermal resilience, the assessment process should start from the design phase through to the decommissioning phase as well as consider the variability of contributing factors, across the various phases of a building’s life cycle. During the design phase, the evaluation requires forecasting occupancy rate and climate change impacts so that associated thermal loads can be determined, thereby, enabling comparison of different resilience improvement approaches. Towards the end of the construction phase and, exactly during the commissioning step, the structure’s resilience profile (which we will present later) needs to be updated to reflect the as-built characteristics of a building. Likewise, the resilience profile needs to be regularly updated, during operation and maintenance, to reflect actual performance and contribute effectively, during a disaster, to life safety, rescue operation, and allocation of emergency resources. To cover all these phases, we have suggested a Building Thermal Resilience Framework (BTRF), which oversees the development of a Building Thermal Resilience Profile (BTRP), that evolves at every phase by accumulating additional inputs, so that different resilience assessment goals, suitable to that phase, are addressed (See Figure 1.)
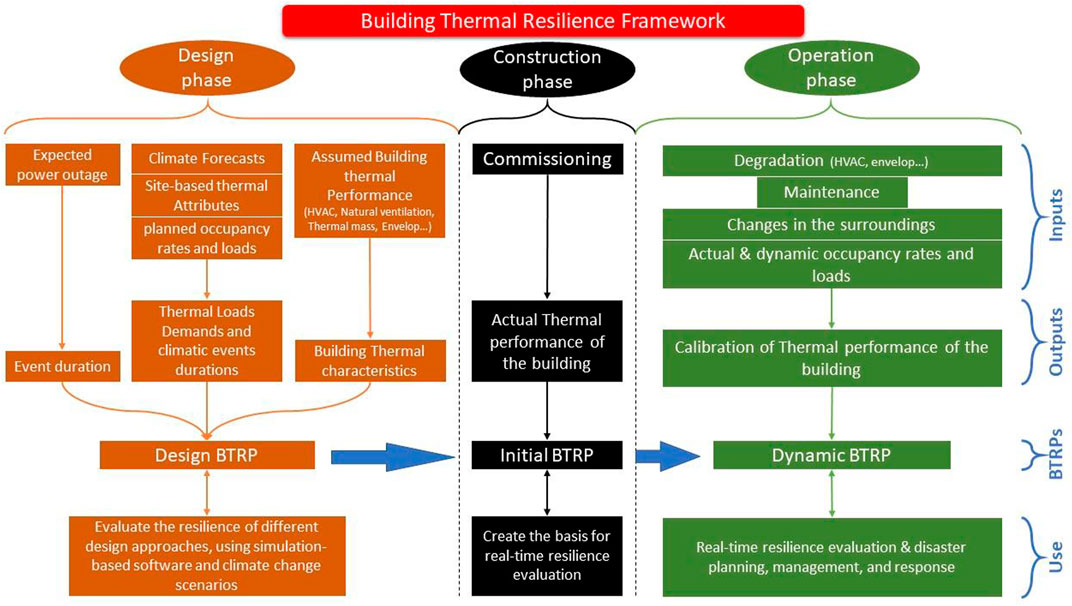
FIGURE 1. Building Thermal Resilience Framework (BTRF). During each phase of the building’s life cycle, a modified version of the Building Thermal Resilience Profile (BTRP) is created. These versions serve different needs at the associated phase and result from accumulating inputs from that phase with the ones from previous phases.
The BTRPs focus on the use of thermal loads and building thermal capacities, under different scenarios, to allow both forecasting and real-time monitoring of a building’s thermal performance. The BTRP reflects the different capacities of a building and their relationship with thermal load demands during both hot and cold climates, as presented in Figure 2. Depending on the stage of the building, different types of BTRP will be developed, including Design BTRP to compare different design options using simulation tools based on several factors to be presented later; Initial BTRP, which will result from the actual performance of the building in the as-built situation and present the starting point of the real-time assessment; and the Dynamic BTRP, which spans across the operation stage and dynamically changes according to the real occupancy at the moment as will further be explained later. The development of Design BTRP requires defining several inputs, including:
• The expected power outage: the expected power outage can yield the duration of power outages as well as be used for managing limited power supply, during disasters, through load shedding, while still avoiding threats to the lives of occupants.
• External factors: including climate forecast (based on climate change models and scenarios), site-based factors (location, orientation, and possible heat island effect), and planned/expected occupancy rates and loads.
• Internal factors: related to the design of the building and its equipment, such as the HVAC system, natural ventilation potential, envelope characteristics, and thermal mass.
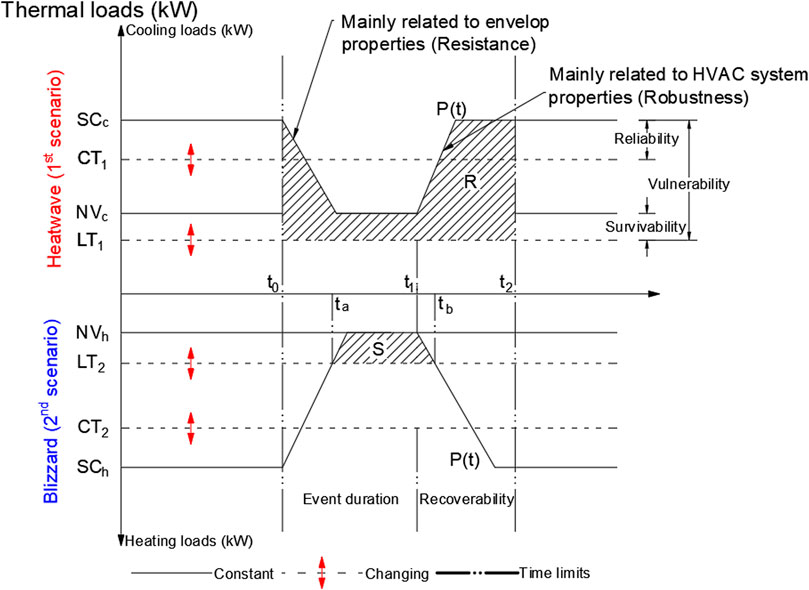
FIGURE 2. Building Thermal Resilience Profile (BTRP). The suggested BTRP covers both ends of thermal load (heating and cooling) and includes certain limits: System Capacity (SC); Comfort Threshold (CT); Natural Ventilation capacity (NV); Life-safety Threshold (LT); t0, t1, and t2 are: the moment event starts, the moment event ends, and the maximum allowed recovery duration, respectively; ta and tb are: the moment when the system loses the capacity to meet LT loads, and the moment the system regains capacity, respectively; c & h subscriptions refer to cooling and heating capacities, respectively. This figure shows two scenarios, the first one happens during a heatwave where the natural ventilation capacity (NV) still allows the system to overcome LT demands; thus, we can evaluate the system resilience (R). The second scenario is during cold weather, where the system fails to meet LT demands, and leads to calculating the situation severity index (S). In addition, some of the resilience qualities are presented in relation to these limits. CT & LT are continuously changing, reflecting the occupancy rates and thermal demands, while SC & NV are calibrated and modified only after regular maintenance.
Despite presenting the different resilience qualities in the BTRP, the main aim is to quantify a building’s resilience. The inputs mentioned above help in developing the Design BTRP, which will be used to compare different options during the design phase. This requires the use of several software packages, to conduct performance simulation, and develop the BTRP for each design and then compute each design’s thermal resilience index (R), based on Eq. 1:
The resulting (R) values, between different design options, will determine the optimal design option, from a resilience perspective. Furthermore, the performance should be checked if, at any point
where
During the commissioning step, the BTRP will undergo a calibration process based on the as-built conditions, which will serve as the basis for (initial) operational BTRP, during the early stages. However, in later stages of operation, (due to degradation, maintenance programs, changes in the surroundings, and building occupancy rates), the BTRP will be subjected to a regular calibration process to update some limits, in addition to continuous changes in the rest of the limits, giving the profile a dynamic characteristic. The accumulated changes in the regular calibration process, can be significant as some researchers have reported that degradation in thermal performance ranges from 20% to 30% over 20 years (Eleftheriadis and Hamdy, 2017). Thus, making the initial BTRP outdated if it is not subjected to regular updates. These regular updates will affect System Capacity (SC) and Natural Ventilation capacity (NV). On the other hand, the Dynamic BTRP will continuously change according to the changes in the momentary occupancy rates, which changes the Comfort Threshold (CT) and Life-safety Threshold (LT). The Dynamic BTRP could be connected to a central disaster management authority, which can direct the efforts, during disasters, to address specific situations as well as prevent loss of life. The designated authority may choose between several options, such as load shedding, evacuation, and allocation of emergency resources, based on the resilience evaluation of the different buildings (in a district) using live monitoring data and short-term forecasting. The Dynamic BTRP serves as an efficient application and can make an important contribution to societal resilience and sustainable resource management, as it has the potential to pave the way for smart buildings and cities. Additionally, the dynamic nature of the BTRP allows considering the efficiencies of different interventions and can be developed to suggest the optimal steps creating the potential for an Artificial-Intelligence (AI) driven system.
Emergency management, during disasters, can be divided into two parts, one related to resource allocation, like electrical power shedding (in a sequence that reduces the possible number of individuals exposed to prolonged thermal stresses), and the other to intervention, like directing community first responders (to evacuate buildings expected to fall under lethal thermal stress); both approaches can be employed in parallel, to maximize the efficiency of life-saving efforts. Moreover, the simulation of such scenarios can help in creating contingency plans, ahead of the impact of heatwaves or winter storms, for possible interventions and resources allocation (Zhou et al., 2020), (Kubilay et al., 2021). By providing a rapid and efficient way to quantify and simulate different scenarios and interventions, the suggested BTRP can play a vital role in overseeing and conducting planning and emergency management best practices.
It is important to understand that, despite the linear presentation of the various components of the BTRP in Figure 2, the actual representation of the system will, most likely, consist of non-linear slopes, especially during system resistance and robustness. Moreover, the actual CT and LT are continuously changing based on occupant activities, creating an irregular shape that can only be presented moment-by-moment. Thus, during the event, the use of a short average period is required for the evaluation, as well as the assumption it continues to be static. Finally, the developed BTRP will be unique for each building, reflecting its characteristics, based on the incorporated system and operational strategies. Yet, depending on the stage, it will be flexible enough to facilitate comparability of the results, between different designs or intervention options.
The suggested BTRP explicitly expresses different resilience qualities and important resilience capacities reported in the literature. As the BTRP is based on the concept agreed upon in the literature, it expresses several capacities that are necessary to reflect system resilience, such as absorptive, adaptive, and restorative capacities (Zhang et al., 2021). Furthermore, as highlighted in the resilience literature, it reflects various resilience qualities which characterize and describe system performance, such as robustness, vulnerability, and reliability (Attia et al., 2021), (Serdar et al., 2022a), (Al-Humaiqani and Al-Ghamdi, 2022). These qualities assure the framework’s alignment with the general concept of system resilience, determined in the resilience literature as well as the specific concept of thermal resilience, as defined and explained in the literature (Attia et al., 2021), (Zhang et al., 2021).
Conclusion
In recent decades, the world has witnessed several catastrophes associated with record-breaking extreme temperatures. These unusual events, both in nature and magnitude, have been associated to climate change. Additionally, the increasing density in urban areas is creating microclimates that magnify the impact of such events. The high cost of such events has clearly proven the limitations of the conventional thermal design approach and motivated the need for a resilience-based approach to buildings’ design and operation. In this paper, we suggested a holistic framework, that considers different phases of the building’s life cycle, in a bid to provide a dynamic and evolving solution for the challenge of thermal resilience in buildings. The framework, and the associated BTRP, can evaluate the efficiency of different design options. Later during the operational phase, the system is regularly calibrated and can provide valuable input for real-time assessment during disasters, thus, facilitating disaster management at various levels. The suggested perspective creates a holistic understanding of the building’s thermal performance in a way that is occupant centric; thus, it has the potential to be employed in health-oriented disaster management practices. The design BTRP can evaluate different options, so it can be embedded in the regulations as a mandatory step, to ensure buildings’ safety, in order to acquire a development license; this will facilitate achieving resilience by design concept on the urban scale. Moreover, as the framework stretches over different steps of the life cycle of the buildings, it can create the backbone for building resilience rating systems similar to green building rating systems, such as LEED which offers certificates for both design and operation; however, such step will require more development and the consideration of other threats to create a holistic resilience evaluation, or integrating specific aspects, such as thermal resilience into the new versions of green building rating systems. Finally, as suggested in the discussion, the BTRP can be used for real-time monitoring and to simulate possible intervention strategies, thus potentially being a valuable disaster management tool. The perspective solution provided in this paper acts as the first step, for an effective framework and further research, to address the challenges of thermal resilience in buildings and integrate them into professional practice, such as green and resilient building rating systems. Furthermore, the outputs of thermal resilience evaluation can be used as inputs, in developing and completing future research, of building resilience evaluation.
Data availability statement
The original contributions presented in the study are included in the article/Supplementary Material, further inquiries can be directed to the corresponding author.
Author contributions
MS and SA-G conceptualization and methodology; MS writing the original draft; MS, NM, and SA-G writing -review and editing; SA-G supervision and funding acquisition. All authors have read and agreed to the published version of the manuscript.
Funding
This research was supported by a scholarship from Hamad Bin Khalifa University (HBKU) a member of Qatar Foundation (QF). Any opinions, findings, and conclusion or recommendations expressed in this material are those of the author(s) and do not necessarily reflect the views of HBKU or QF.
Acknowledgments
The research team would like to also thank Mark Twain for his insight and valuable comments.
Conflict of interest
The authors declare that the research was conducted in the absence of any commercial or financial relationships that could be construed as a potential conflict of interest.
Publisher’s note
All claims expressed in this article are solely those of the authors and do not necessarily represent those of their affiliated organizations, or those of the publisher, the editors and the reviewers. Any product that may be evaluated in this article, or claim that may be made by its manufacturer, is not guaranteed or endorsed by the publisher.
References
Abedrabboh, O., Koç, M., and Biçer, Y. (2022). Sustainability performance of space-cooling technologies and approaches. Energy Sources Part A Recovery Util. Environ. Eff. 44, 9017–9042. doi:10.1080/15567036.2022.2127979
Al-Humaiqani, M. M., and Al-Ghamdi, S. G. (2022). The built environment resilience qualities to climate change impact: Concepts, frameworks, and directions for future research. Sustain. Cities Soc. 80, 103797. doi:10.1016/j.scs.2022.103797
Aljawabra, F., and Nikolopoulou, M. (2018). Thermal comfort in urban spaces: A cross-cultural study in the hot arid climate. Int. J. Biometeorol. 62 (10), 1901–1909. doi:10.1007/s00484-018-1592-5
Attia, S., Levinson, R., Ndongo, E., Holzer, P., Berk Kazanci, O., Homaei, S., et al. (2021). Resilient cooling of buildings to protect against heat waves and power outages: Key concepts and definition. Energy Build. 239, 110869. doi:10.1016/j.enbuild.2021.110869
Azarnejhad, A., and Mahdavi, A. (2019). On the impact of building façades’ color on thermal building performance and outdoor thermal comfort. Appl. Mech. Mat. 887, 189–195. doi:10.4028/www.scientific.net/AMM.887.189
Blanco Cadena, J. D., Moretti, N., Salvalai, G., Quagliarini, E., Re Cecconi, F., and Poli, T. A new approach to assess the built environment risk under the conjunct effect of critical slow onset disasters: A case study in milan, Italy. Appl. Sci. (Basel). 11 (3), 1186. doi:10.3390/app11031186
Blanco Cadena, J. D., Salvalai, G., Lucesoli, M., Quagliarini, E., and D’Orazio, M. Flexible workflow for determining critical hazard and exposure scenarios for assessing SLODs risk in urban built environments. Sustainability 13 (8), 4538. doi:10.3390/su13084538
Bruneau, M., Chang, S. E., Eguchi, R. T., Lee, G. C., O'Rourke, T. D., Reinhorn, A. M., et al. (2003). A framework to quantitatively assess and enhance the seismic resilience of communities. Earthq. Spectra 19 (4), 733–752. doi:10.1193/1.1623497
Busby, J. W., Baker, K., Bazilian, M. D., Gilbert, A. Q., Grubert, E., Rai, V., et al. (2021). Cascading risks: Understanding the 2021 winter blackout in Texas. Energy Res. Soc. Sci. 77, 102106. doi:10.1016/j.erss.2021.102106
Chen, H., Zhao, L., Cheng, L., Zhang, Y., Wang, H., Gu, K., et al. (2022). Projections of heatwave-attributable mortality under climate change and future population scenarios in China. Lancet Regional Health - West. Pac. 28, 100582. doi:10.1016/j.lanwpc.2022.100582
D’Amico, A., Russo, M., Angelosanti, M., Bernardini, G., Vicari, D., Quagliarini, E., et al. (2021). Built environment typologies prone to risk: A cluster Analysis of open spaces in Italian cities. Sustainability 13 (16), 9457. doi:10.3390/su13169457
De Wit, M. S. (1997). Identification of the important parameters in thermal building simulation models. J. Stat. Comput. Simul. 57 (1–4), 305–320. doi:10.1080/00949659708811814
Eleftheriadis, G., and Hamdy, M. (2017). Impact of building envelope and mechanical component degradation on the whole building performance: A review paper. Energy Procedia 132, 321–326. doi:10.1016/j.egypro.2017.09.739
Faturechi, R., and Miller-Hooks, E. (2015). Measuring the performance of transportation infrastructure systems in disasters: A comprehensive review. J. Infrastruct. Syst. 21 (1), 04014025. doi:10.1061/(ASCE)IS.1943-555X.0000212
Felicioni, L., Lupíšek, A., and Hájek, P. (2020). Major European stressors and potential of available tools for assessment of urban and buildings resilience. Sustainability 12 (18), 7554. doi:10.3390/su12187554
García-Herrera, R., Díaz, J., Trigo, R. M., Luterbacher, J., and Fischer, E. M. (2010). A review of the European summer heat wave of 2003. Crit. Rev. Environ. Sci. Technol. 40 (4), 267–306. doi:10.1080/10643380802238137
Gherri, B., Maiullari, D., Finizza, C., Maretto, M., and Naboni, E. (2021). On the thermal resilience of Venetian open spaces. Heritage 4 (4), 4286–4303. doi:10.3390/heritage4040236
Gholami Rostam, M., and Abbasi, A. (2021). A framework for identifying the appropriate quantitative indicators to objectively optimize the building energy consumption considering sustainability and resilience aspects. J. Build. Eng. 44, 102974. doi:10.1016/j.jobe.2021.102974
Heracleous, C., Michael, A., Savvides, A., and Hayles, C. (2021). Climate change resilience of school premises in Cyprus: An examination of retrofit approaches and their implications on thermal and energy performance. J. Build. Eng. 44, 103358. doi:10.1016/j.jobe.2021.103358
Homaei, S., and Hamdy, M. (2021). Thermal resilient buildings: How to be quantified? A novel benchmarking framework and labelling metric. Build. Environ. 201, 108022. doi:10.1016/j.buildenv.2021.108022
Hong, T., Xu, Y., Sun, K., Zhang, W., Luo, X., and Hooper, B. (2021). Urban microclimate and its impact on building performance: A case study of san francisco. Urban Clim. 38, 100871. doi:10.1016/j.uclim.2021.100871
Hosseini, S., Barker, K., and Ramirez-Marquez, J. E. (2016). A review of definitions and measures of system resilience. Reliab. Eng. Syst. Saf. 145, 47–61. doi:10.1016/J.RESS.2015.08.006
Kubilay, A., Ferrari, A., Derome, D., and Carmeliet, J. (2021). Smart wetting of permeable pavements as an evaporative-cooling measure for improving the urban climate during heat waves. J. Build. Phys. 45 (1), 36–66. doi:10.1177/1744259120968586
Kudabayev, R., Suleimenov, U., Ristavletov, R., Kasimov, I., Kambarov, M., Zhangabay, N., et al. (2022). Modeling the thermal regime of a room in a building with a thermal energy storage envelope. Math. Model. Eng. Probl. 9 (2), 351–358. doi:10.18280/mmep.090208
Ma, H., Wang, Y., and Lin, Z. (2022). Future changes of summer heat waves over urban agglomerations in eastern China under 1.5°C and 2.0°C global warming. Front. Earth Sci. 10, 823286. doi:10.3389/feart.2022.823286
mailto Attia, S., Rahif, R., Corrado, V., Levinson, R., Wang, L., Sodagar, B., et al. (2021). Framework to evaluate the resilience of different cooling technologies,” sustainable building design lab PP - liege. Belgium: Université de Liège - ULiège > Département ArGEnCo > Techniques de construction des bâtiments.
NASA (2022). What’s the difference between climate change and global warming? – climate change: Vital signs of the planet. Available at: https://climate.nasa.gov/faq/12/whats-the-difference-between-climate-change-and-global-warming/(accessed Oct 29, 2022).Available at:
Perkins-Kirkpatrick, S. E., and Lewis, S. C. (2020). Increasing trends in regional heatwaves. Nat. Commun. 11 (1), 3357. doi:10.1038/s41467-020-16970-7
Rahif, R., Hamdy, M., Homaei, S., Zhang, C., Holzer, P., and Attia, S. (2022). Simulation-based framework to evaluate resistivity of cooling strategies in buildings against overheating impact of climate change. Build. Environ. 208, 108599. doi:10.1016/j.buildenv.2021.108599
Salimi, M., and Al-Ghamdi, S. G. (2020). Climate change impacts on critical urban infrastructure and urban resiliency strategies for the Middle East. Sustain. Cities Soc. 54, 101948. doi:10.1016/j.scs.2019.101948
Schiermeier, Q. (2021). Climate change made Europe's mega-heatwave five times more likely. Nature 571, 155. doi:10.1038/d41586-019-02071-z
Schünemann, C., Schiela, D., and Ortlepp, R. (2021). Guidelines to calibrate a multi-residential building simulation model addressing overheating evaluation and residents’ influence. Buildings 11 (6), 242. doi:10.3390/buildings11060242
Schünemann, C., Son, S., and Ortlepp, R. (2022). Heat resilience of apartment buildings in korea and Germany: Comparison of building design and climate. Int. J. Energy Environ. Eng. 13, 889–909. doi:10.1007/s40095-022-00476-7
Serdar, M. Z., and Al-Ghamdi, S. G. (2021). Resiliency assessment of road networks during mega sport events: The case of FIFA world Cup Qatar 2022. Sustainability 13 (22), 12367. doi:10.3390/su132212367
Serdar, M. Z., Koc, M., and Al-Ghamdi, S. G. (2021). Urban infrastructure resilience assessment during mega sport events using a multi-criteria approach. Front. Sustain. 2, 673797. doi:10.3389/frsus.2021.673797
Serdar, M. Z., and Al-Ghamdi, S. G. (2021). “Preparing for the unpredicted: A resiliency approach in energy system Assessment,” in Green energy and technology. Editor J. Ren (Cham: Springer International Publishing), 183–201. doi:10.1007/978-3-030-67529-5_9
Serdar, M. Z., Koç, M., and Al-Ghamdi, S. G. (2022). Urban transportation networks resilience: Indicators, disturbances, and assessment methods. Sustain. Cities Soc. 76, 103452. doi:10.1016/j.scs.2021.103452
Serdar, M. Z., Çağlayan, B. Ö., and Al-Ghamdi, S. G. (2022). A special characteristic of an earthquake response spectrum detected in Turkey. Mater. Today Proc. 62, 3589–3592. doi:10.1016/j.matpr.2022.04.407
Su, B., Jadresin Milic, R., McPherson, P., and Wu, L. (2022). Thermal performance of school buildings: Impacts beyond thermal comfort. Int. J. Environ. Res. Public Health 19 (10), 5811. doi:10.3390/ijerph19105811
Sun, K., Specian, M., and Hong, T. (2020). Nexus of thermal resilience and energy efficiency in buildings: A case study of a nursing home. Build. Environ. 177, 106842. doi:10.1016/j.buildenv.2020.106842
Tahir, F., Ajjur, S. B., Serdar, M. Z., Al-Humaiqani, M., Kim, D., Al-Thani, S. K., et al. (2021). Qatar climate change conference 2021 Editor Al-Ghamdi, and Al-Thani. Doha, Qatar: Hamad bin Khalifa University Press. doi:10.5339/conf_proceed_qccc2021
U.S. Geological Survey What is the difference between global warming and climate change? Available at: https://www.usgs.gov/faqs/what-difference-between-global-warming-and-climate-change (accessed Oct. 29, 2022).
Wang, Z., Hong, T., and Li, H. Informing the planning of rotating power outages in heat waves through data analytics of connected smart thermostats for residential buildings. Environ. Res. Lett. 16 (7), 074003. doi:10.1088/1748-9326/ac092f
Zeng, Z., Zhang, W., Sun, K., Wei, M., and Hong, T. (2022). Investigation of pre-cooling as a recommended measure to improve residential buildings’ thermal resilience during heat waves. Build. Environ. 210, 108694. doi:10.1016/j.buildenv.2021.108694
Zhang, C., Kazanci, O. B., Levinson, R., Heiselberg, P., Olesen, B. W., Chiesa, G., et al. (2021). Resilient cooling strategies – a critical review and qualitative assessment. Energy Build. 251, 111312. doi:10.1016/j.enbuild.2021.111312
Zhivov, A. M. (2021). Parameters for thermal energy systems resilience. E3S Web Conf. 246. doi:10.1051/e3sconf/202124608001
Keywords: thermal resilience, heatwave, built environment resiliency, cooling and heating load, disaster and risk management, climate change resilience and adaptation, disaster planning and preparedness
Citation: Serdar MZ, Macauley N and Al-Ghamdi SG (2022) Building thermal resilience framework (BTRF): A novel framework to address the challenge of extreme thermal events, arising from climate change. Front. Built Environ. 8:1029992. doi: 10.3389/fbuil.2022.1029992
Received: 28 August 2022; Accepted: 31 October 2022;
Published: 11 November 2022.
Edited by:
Fahim Tonmoy, Griffith University, AustraliaReviewed by:
Graziano Salvalai, Politecnico di Milano, ItalyCopyright © 2022 Serdar, Macauley and Al-Ghamdi. This is an open-access article distributed under the terms of the Creative Commons Attribution License (CC BY). The use, distribution or reproduction in other forums is permitted, provided the original author(s) and the copyright owner(s) are credited and that the original publication in this journal is cited, in accordance with accepted academic practice. No use, distribution or reproduction is permitted which does not comply with these terms.
*Correspondence: Sami G. Al-Ghamdi, salghamdi@hbku.edu.qa