RE:SLAB—a load bearing system for open-ended component reuse in building structures
- 1Structural Xploration Lab SXL, School of Architecture, Civil and Environmental Engineering ENAC, Ecole Polytechnique Fédérale de Lausanne EPFL, Lausanne, Switzerland
- 2Aeternum Technologies, Zollikofen, Switzerland
- 3iTEC, Institute of Construction and Environmental Technology, University of Applied Sciences of Western Switzerland, Fribourg, Switzerland
The construction industry plays a major role in the high levels of greenhouse gas emissions, resource consumption, and waste generation observed nowadays. Key to the circular economy, structural component reuse arises as a promising solution to divert construction waste from landfilling and avoid the production of new components. In this context, this paper presents the conceptual design of a new slab-and-column system called “Re:Slab”, optimized for disassembly and open-ended reassembly over multiple building lifespans. Beyond conventional considerations of modular sizing and reversible connections, the proposed system provides designers with a minimum kit of parts that is capable of exceptionally diverse building layouts–e.g., related to floor geometry, span between supports, applied loads, and spatial transformations. Attention is given to easily operable assembly and disassembly techniques, embodied environmental impacts, and manufacturing costs. As a result, the proposed system reaches unprecedented high levels of versatility, making it capable of adapting to future functional design requirements that are hard to predict over long-term social developments. Options for increased economic viability are identified, which are necessary to promote widespread adoption of the system.
1 Introduction
Across all knowledge fields, humankind has experienced unprecedented industrial and technological development during the last decades. Most research efforts worldwide have focused on developing technological means for broader accessibility to higher life quality standards. Interestingly, this has led to most of the population’s day-to-day running on autopilot, fueling a widespread lack of awareness regarding the actual resources required for daily activities. Recent data show that humankind has a current resource deficit of 1.7 times the Earth’s regenerative capacity (Grooten et al., 2018), posing a significant threat to the welfare of forthcoming generations and the planet’s stability (Steffen et al., 2015; O’Neill et al., 2018), and with the trend expected to increase as high as 2.6 times by 2050 (Moore et al., 2012). Even though regulation policies at several levels have been enforced during the last years to slow down this tendency (Kinzig et al., 2013), 30 billion tons of materials are produced annually across different industries, reaching in the year 2020 that global human-made mass exceeds all living biomass on Earth (Elhacham et al., 2020).
The construction industry is a major player in this increasing resource consumption trend. It is responsible for 30%–50% of natural resource exploitation worldwide (WST, 2012; Herczeg et al., 2014), 36% of global energy use (United Nations, 2017), and 16% of annual water intake (Cheng et al., 2006). By the year 2020, the construction industry accounted for a gross global mass of 1,100 gigatons, the equivalent of 94% of all human-made production up to date, where concrete manufacturing amounted to 549 gigatons out of the total toll (Elhacham et al., 2020). Not only consumption, but the construction industry is also responsible for 39% of the world’s greenhouse emissions (United Nations, 2017) and accounts for a significant percentage of the global waste stream, with numbers going from one-third in Europe (European Commission, 2018) to two-thirds in developing economies such as Brazil (ABRELPE, 2018). With an urban population expected to increase up to 70% by 2050 (United Nations, 2017) and the inevitable rise of global resource deficiency during the upcoming years (Chen et al., 2010; Ellen MacArthur Foundation, 2013), several governments have turned towards running resource-efficient economies by introducing waste upcycling hierarchies (Ellen MacArthur Foundation, 2013) that aim at enhancing material efficiency through all economic sectors, the construction industry being the prime focus of the campaign.
Current construction practices conventionally follow a linear economic model of “take-make-dispose” (Ellen MacArthur Foundation, 2015), preventing any further reuse of components after the buildings’ lifespan. In this way, most of the waste stream produced by the industry (up to 50% (Kibert, 2016)) takes place at the end-of-life phase since a vast majority of current materials do not have a reuse potential and end up in landfill sites. In contrast, economic models based on Circular Economy (CE) principles aim to keep materials and components in a closed loop at their highest value (Ellen MacArthur Foundation, 2015), delaying obsolescence by stretching their lifespan across several uses before disposal. Nowadays, recycling is the most common CE strategy in the industry, being the primary means of diverting construction waste from permanent disposal. For instance, about 90% of the non-hazardous construction and demolition waste in the UK is recovered by recycling (DEFRA, 2019). However, the processes involved in recycling are resource- and energy-intensive (Rakhshan et al., 2020), have significant environmental impacts in terms of greenhouse gas emissions (Addis, 2012), and often lead to downgraded materials, thus decreasing the quality of subsequent products (Braungart et al., 2007). Besides adaptive reuse, i.e., the in situ adaptation of existing systems (Remøy and van der Voordt, 2014), another CE strategy that has gained traction during the last few years is component reuse, which directs materials and elements back to the supply chain after the end-of-life phase. With the development of new design methods such as Design for Deconstruction (DfD) (Akinade et al., 2017) and Design for Manufacture and Assembly (DfMA) (Kalyun and Wodajo, 2012), component reuse aims at decoupling economic growth from resource consumption by slowing, closing, and narrowing material loops in the construction industry (López Ruiz et al., 2020).
In pre-industrial times, reuse has been a common habit for construction practitioners due to time and cost advantages compared to new production (Fivet and Brütting, 2020). With the advent of industrial developments and decreased production costs, reuse became occasional and feasible just for specific boundary conditions. Nevertheless, several component reuse applications and case studies can be found in the current literature in projects of different natures (Addis, 2012; Ellen MacArthur Foundation, 2016; Benachio et al., 2020; Fivet and Brütting, 2020; Rakhshan et al., 2020). Present-day reuse techniques rely on hunting and the procurement of components from to-be-demolished structures so as to develop a material stock for designers to work with. Recently, this task has been partially facilitated by the creation of material banks and passports (BAMB, 2017b; BAMB, 2017a) aiming at generating a continuous offer/demand for reusable components with guaranteed properties. However, there are still a number of barriers of different nature that hinder the widespread adoption of component reuse.
As identified by previous investigations (Fivet and Brütting, 2020; Rakhshan et al., 2020), uncertainties about deconstruction times, scarce know-how of reuse techniques, and limited stocks of reusable components might threaten the tight schedules of the construction industry. Besides, the lack of standard components designed for deconstruction leads to increased cost overheads during disassembly and reassembly phases. Moreover, although new computational tools have recently been developed to assist practitioners during the design stage (Brütting et al., 2019; Brütting et al., 2020), limitations in element length, cross-section, and allowable load, along with uncertainties in the mechanical properties and availability of reusable components, poses new challenges of engineering and architecture.
Component reuse will have a relevant role in reducing the construction industry’s emissions and consumption only if it reaches widespread implementation on an industrial scale. In order to do so, the barriers and drawbacks described above must be acknowledged, understood, and overcome. Stemming from this thought development, this paper presents a new slab-and-column structural system called Re:Slab, designed for deconstruction and open-ended reuse over several lifespans, aiming at maximizing the reuse potential of building components. By employing reversible connections and modular elements, the Re:Slab system enhances the efficiency of assembling/disassembling processes, simultaneously allowing a large spectrum of feasible configurations. Moreover, it provides designers with a versatile framework regarding floor geometries, spans, load conditions, and element distribution, paving the way for an open-ended reuse framework, resulting in buildings being deconstructed and reassembled in different configurations as required by users over several life cycles, as Figure 1 schematically shows. Finally, the system has been conceived to have a high prefabrication level so as to expedite its market entry and use among practitioners and construction companies. It is expected that an extensive implementation of the solution herein proposed will contribute to reducing the resource consumption of the construction industry and decreasing its overall environmental footprint.
2 Literature review: current paradigms in the construction industry
Designing for deconstruction and open-ended reuse requires a throughout disruption of the status quo in structural engineering and the construction industry, so as to make room for new CE paradigms in the short and long term. Following the principle of “form follows function”, strategies such as long-term adaptability, modularity, or reversibility, are considered key factors to turn current structural practices towards sustainable reuse scenarios. Interestingly, such strategies have already been on the discussion table among researchers and practitioners, paving the path for new developments in the field.
2.1 Long term-adaptability
Designing for long-term adaptability has been studied by previous researchers as a concept that allows buildings to meet future unforeseen spatial, technical, or functional requirements (Fivet, 2019). Its origins date back to the early 70s and the work of Dutch architects Frans van der Werf and John Habraken, with the development of the Open Building concept (Habraken, 2003). As an attempt to take into account the building’s permanent need to change and adapt during its lifetime, the Open Building philosophy makes a clear distinction between the base building and the fit-out, where the base building exerts as a collective facility, and the fit-out is specific for each spatial unit (Habraken, 2003; John Habraken, 2008). In this way, subsystems can be modified to adapt to future social and technological changes as required by the final user while the main system’s lifespan is extended. Several successful implementations of this design concept have been observed worldwide during the last decades (Kendall and Teicher, 2010).
Further developments of the Open Building idea have led to the advent of the Shearing Layers concept. Coined by Frank Duffy and later elaborated by Stewart Brand, the Shearing Layers concept regards buildings as a set of subsystems that evolve in different timescales, proposing a hierarchical organization based on their life expectancy (Brand, 1995). Six layers are identified by Brand (Brand, 1995): site, structure, skin, services, space plan, and stuff. In this way, fast-changing components or spaces can be easily replaced/modified within a layer without affecting others, allowing flexible buildings that adapt over time according to user needs, technological developments, or social needs (Leupen, 2006; Karimah and Paramita, 2020). This is consistent with the requirements of designing for deconstruction and reuse, where buildings should be conceived to suit a wide spectrum of possible unknown scenarios. However, although the adaptability of shallow layers (services or space plan) is greatly facilitated (Schneider and Till, 2007), modifications to long-lasting layers (structure or skin) might not be straightforward or even possible. Therefore, significant changes to the building’s main skeleton are currently only carried out through partial or complete demolition.
2.2 Modularity
Modular construction is re-emerging as an alternative methodology to boost prefabrication and reduce costs and timelines. By manufacturing 1D, 2D, and 3D components offsite and assembling them on-site, modular construction processes are estimated to accelerate project schedules by 20%–50% (Bertram et al., 2019), realize budget savings of 20% (Bertram et al., 2019), and reduce the on-site waste generation to as low as 1% (Generalova et al., 2016). The adoption of new materials, digital technologies, and manufacturing procedures, along with the increasing population growth and housing deficit, welcomes a fresh wave for the modular industry, with its market value expected to reach $130 billion in Europe and the US by 2030 (Bertram et al., 2019). Besides, it presents an opportunity to tackle the high environmental impact of the construction industry by reducing the emissions caused by construction operations (Gann, 1996; Lu and Liska, 2008).
Modularity has been successfully implemented in construction projects of different natures worldwide (Lawson et al., 2014), and particular applications for specific construction requirements have also been developed (Lawson et al., 2012). Interestingly, modular solutions compatible with the philosophy of design for deconstruction and reuse can also be found in the current literature. For instance, Liberman (Liberman, 2014) presents a methodology for assembling and disassembling modular units made up of concrete columns and slabs. By employing steel receptacles and access ports embedded in concrete, the different elements of the module can be put together to create 3D spatial units. Likewise, components can be easily disassembled to be reused or recycled. Segal (Segall, 2018) presents a methodology for assembling single-level relocatable habitat units. It employs a set of panels, corner posts, horizontal beams, and male/female connectors located at the peripheries. Simple hand-operated tools assemble the units and can be further disassembled for relocation or reuse of single components. Wilson and Wilson (Wilson and Wilson, 2017) developed a multi-story reusable rack for modular housing units. Remarkably, the proposed structural rack also allows for reusing subsystems such as the electrical, water, or wastewater ones. The housing units are fixed to the rack by means of reversible connections, so further replacements or changes to individual units can be carried out. Likewise, the structure of the rack itself can be disassembled and reconstructed in different configurations if required by modifications of the housing units. Modular construction is compatible with deconstruction and reuse as long as reversible connectors join modular units together. If monolithic connections are employed instead (such as welding or grouting), disassembly of modules might require demolition, thus threatening the integrity of components and preventing them from further high-quality reuse.
Three-dimensional volumetric modular systems (i.e., container type) are the market-dominant solution because of their construction advantages and low operational costs. For projects that require a high level of repeatability, 3D systems result in 24% cost savings when compared to traditional construction methodologies (Bertram et al., 2019) and allow up to 95% offsite prefabrication (Chourasia et al., 2023). Besides, they can be manufactured employing different materials and in combination with other structural solutions to meet specific load requirements (Chourasia et al., 2023). However, modular 3D systems are constrained to fixed spans and volumes, making them case-specific and hindering future reuse of components for different applications.
2.3 Reversibility
Reversibility in building design refers to conceiving structural systems and subsystems that can be easily disassembled or deconstructed, and whose parts can be added and removed without damaging the building and its components (Durmisevic, 2006; Donovan et al., 2023). It emphasizes the capability of buildings and components to get back to an earlier or initial status. In this way, buildings can be designed so that their components follow material loops, ease structural changes, allow changing user requirements, and keep high resource efficiency (Durmisevic, 2019a; Durmisevic, 2019b). Durmisevic (Durmisevic, 2006) proposes several principles to bring this philosophy into practice, such as the generality of spaces, upgradability and adaptability of assemblies, durability and compatibility of building components, and the reversibility of connections.
From a technical point of view, reversibility is accomplished through careful design of demountable connections between systems, subsystems, and components. Steel structures with bolted joints are reversible by default, and several successful case studies of deconstruction and reuse of steel structures are present in the current literature (Ellen MacArthur Foundation, 2016; Fivet and Brütting, 2020; Rakhshan et al., 2020). Bolted connections are usually employed with mass timber solutions as well, allowing easy disassembly of walls, columns, beams, and slabs (Erman, 2002; Hairstans et al., 2018; Estrella et al., 2021a; Orellana et al., 2021). Reversible connections have also been developed for concrete and hybrid structures. For instance, Kozma et al. (Kozma et al., 2019) proposed a novel shear connector for demountable composite beams. The solution employs standard M20 bolts, steel plates, and L-shaped steel beams cast into concrete. For easy assembly and disassembly, bolts are accessible through concrete pockets from the top of the slab. Uy et al. (Uy et al., 2017) developed a set of demountable solutions for beam-to-beam, column-to-column, and beam-to-slab joints. The designs use high-strength bolts steel plates, and were validated through non-linear finite element models. Similar developments have been proposed for seismic-resistant structures under cyclic loads, such as those presented by Aninthaneni and Dhakal (Aninthaneni, et al. 2017) or Cia et al. (Cai et al., 2019). Under this load condition, reversible connections must meet the ductility requirements of building codes (Estrella et al., 2020; Estrella et al., 2021b).
2.4 Design for disassembly
Designing for deconstruction and reuse requires the successful implementation of the concepts described above (i.e., long-term adaptability, modularity, and reversibility) so as to guarantee efficient assembly/disassembly procedures and component adaptability through several reuses. However, since the requirements of future life cycles are unknown at the time of design, reusable structures should also be devised under open-ended principles and criteria. Designing for open-ended reusability implies that structures can adapt to future unforeseen needs not only at the fit-out level, but the main building skeleton can be modified to make room for new load conditions, spans, inter-story heights, support layouts, among others. In this way, the possibilities for future reuse scenarios become limitless. Likewise, the lack of open-ended design criteria might hinder the reuse potential of structures even if they were initially conceived for long-term adaptability, modularity, and reversibility, as reported by previous investigations (Fivet, 2019). The open-ended design philosophy is yet to be embraced by the construction industry since its adoption is mainly restrained by the deterministic design approach that assumes that future user needs will be the same as current ones. Although this latter might be true for short timespans, structures that seek to be reused over several life cycles should be projected for long-term, hard-to-anticipate timelines (∼200 years). Bringing this concept into a novel structural system is the primary purpose of the investigation presented in this paper.
3 Materials and methods
This investigation employs a Research-through-Design (RtD) approach as a methodological tool for the development of the Re:Slab system. In this way, new knowledge is generated by systematic and iterative evaluations of design results and feedback (Zimmerman and Forlizzi, 2014). By mixing theoretical concepts with design and development, RtD methods allow exploratory ideas and status quo disruption under a scientific controlled environment. The RtD framework for the investigation in this paper is shown in Figure 2. As part of the workflow, six main stages are identified (Grooten et al., 2018): goals and functional requirements definition (O’Neill et al., 2018), iterative design sessions (Steffen et al., 2015), conceptual design (Moore et al., 2012), structural implementation (Kinzig et al., 2013), feasibility analysis, and (Elhacham et al., 2020) full-scale validation.
The decision-making methodology along the workflow is here based on a three-scale hierarchy (Grooten et al., 2018): open-ended reuse (O’Neill et al., 2018), environmental footprint, and (Steffen et al., 2015) economic cost. Therefore, although environmental impacts or construction costs are not left behind, decisions are, in priority, made to favour open-ended reusability. This strategy fosters originality compared to conventional design approaches and allows for meeting the system goals and requirements outlined in Sections 3.1 and 3.2 more straightforwardly.
3.1 System goals
A set of goals was defined early in the development of the Re:Slab system so as to lay down its guiding design principles and soundly define the overall requirements to meet. Following a downstream component reuse philosophy (Fivet and Brütting, 2020) of designing structures for easy repair, replacement, disassembly, transport, and reassembly, the system’s goals aim at closing the gap between reusability and its widespread application. Furthermore, they intend to account for the inherent uncertainty of future scenarios and maximize the open-ended reuse potential of structures by including CE principles during the design phase. The system goal is to maximize open-ended reuse potential through:
• Reversibility of elements, connections, and assembling procedures.
• Transformability and adaptability to different scenarios and boundary conditions.
• Prefabrication and modularity as means to optimize manufacture, storage, and transportation.
3.2 Functional requirements
Designing for deconstruction and reuse (DfDR) is not a common habit among practitioners nowadays; therefore, current design guidelines and principles might not be suitable for structures aimed at being disassembled and reused. The main challenge upon DfDR relies on foreseeing all possible future scenarios for the to-be-deconstructed structure, such as changes in building purpose, engineering and architectural variations, different market interests, and further technological development. Since typical building lifespans are about 30–40 years (Liu et al., 2014; Muresan et al., 2020), the variables mentioned above are difficult to anticipate during early design stages, hindering a wide application of DfDR due to high uncertainty levels. Therefore, prior to developing the Re:Slab system, a set of functional requirements was established to provide a well-defined research framework. By looking into past, present, and future reuse practices, the requirements were put forward aiming at defining the niche and boundary conditions for the newly proposed system, guaranteeing the reuse appeal (both technical and economic) of the structure under several future scenarios, and allowing open-ended reusability of the structural system and its components. Because of its originality when compared to the functional features of existing conventional and less-conventional structural systems, the chosen set of functional requirements also guaranteed that any system fulfilling them all would have unprecedented features.
As described in the following subsections, functional requirements were sorted out into three groups so as to have a better implementation along the conceptual design phase (Grooten et al., 2018): structural (O’Neill et al., 2018), assembly and disassembly, and (Steffen et al., 2015) versatility and modularity.
3.2.1 Structural requirements
Structural requirements determine the intended scope of the proposed system in terms of its applications, expected performance, and inherent potential market. Structural requirements are to:
• To provide a load-bearing structural system for low-to mid-rise office or residential buildings.
• To transfer vertical loads down to the foundation system. The system is not intended to resist horizontal loads and should be designed along with a lateral-resistant system (e.g., core walls, bracing system, among others).
• To comply with variable spans and different load levels.
• To employ robust and durable materials for components and connections.
• To provide an effortless installation/removal of vertical shafts, pipes, and other non-structural elements.
3.2.2 Assembly and disassembly requirements
Assembly and disassembly requirements intend to establish a set of design constraints in order to maximize the structure’s reuse potential under unknown future scenarios. By defining simple design requirements, effortless assembly and disassembly procedures can be guaranteed. The requirements are:
• To employ reversible and readily accessible mechanical connections upon assembling elements and components.
• To maximize the use of standard connections across different system levels (substructure, superstructure, flooring, and non-structural components).
• To employ low-tech tools and procedures for assembling and disassembling. Likewise, to provide means for easy handling and transportation of components.
• To favour dry construction procedures.
3.2.3 Versatility and adaptability requirements
Versatility and adaptability requirements aim at providing the basis to secure open-ended reusability of the proposed system. In this way, the solution and its detailing allow high flexibility in designing new architectural layouts and structural configurations for unknown reuse scenarios, further expanding the functional and technical properties of the system. The requirements are:
• To allow open-ended configurations and solutions by allowing a wide range of feasible (re-)arrangements of structural and non-structural components.
• To employ interchangeable modular elements and connections, facilitating any further reorganization and replacement of system components.
• To allow inclusion and removal of openings across the floor layout, providing room for new staircases, elevators, shafts, technical installations, or extensions.
• Not to restrict the inner partitioning layout and to facilitate parallel disassembly if modifications are required.
The requirements above act as conceptual design rules during the development of the Re:Slab system; therefore, the component reuse principles of durability, versatility, reversibility, modularity, and transformability proposed by previous investigations (Fivet and Brütting, 2020) are fully met by the proposed system. On the other hand, even though some of the discussed requirements are specific for the purpose and scope of the project described in this paper, they can be adapted and employed for developing other structural systems intended for deconstruction and reuse. If different construction niches are pursued, such requirements are meant to act as reference benchmarks in order to maximize the reuse potential of structural solutions at early design stages.
4 Results: conceptual development of the Re:Slab system
This section presents the load-bearing structural system conceived for deconstruction and reuse employing the design guidelines outlined in Sections 3.1 and 3.2. The following subsections discuss the conceptual development of the system and its components, as well as the technical and engineering features that allow its open-ended reuse over several lifespans.
4.1 Re:Slab system: overall description
Figure 3 shows a schematic representation of the Re:Slab system. As observed, the system comprises discrete slab modules arranged horizontally to form a lateral surface and column elements to transfer vertical loads to the foundations. Slab elements are horizontally connected by in-plane slab-to-slab connections to create a lateral tessellation, allow easy assembly and disassembly, and enable multiple floor plan configurations. Slab elements can also be vertically superimposed by employing out-of-plane slab-to-slab connections to increase structural depth, stiffness, and strength locally, allowing larger spans and higher vertical loads without oversizing base elements.
Floor tiles are installed on slab elements to create a finishing surface and provide non-structural functions. Column elements are attached to slab elements using column-to-slab connectors and can be placed at any required location throughout the floor plan in discrete positions, e.g., through slab openings or at beam crossings. Short column elements might also be employed to compensate for differences between column lengths and slab thicknesses. This arrangement of structural components enables a versatile spectrum of possible architectural and engineering configurations, enhances the system’s reuse capability, and favours industrialized and modular construction processes. Besides, parallel construction and deconstruction are possible; in other words, any sequence of element disassembly is feasible. This allows further local changes in the floor plan, e.g., for the creation of new large vertical shafts for technical ducts, stairs or lifts, or repairs of malfunctioning components.
4.2 Slab elements
Slab elements are made up of an arrangement of orthogonally intersecting beams that transfer vertical loads to adjacent slabs or columns. The beams’ discrete location leads to a uniform distribution of slab openings that make room for columns and small technical shafts, resulting in a low-weight solution compared to traditional continuous flooring systems. Slab elements can be laterally connected to one another at any position, and perimeter faces are not required to coincide; therefore, floor plans with multiple configurations and patterns can be designed and constructed with minimum additional complexity. Besides, the dense distribution of beams and openings allows for a straightforward installation of floor finishes and non-structural components. Slab elements can be designed and developed employing any long-lasting structural material (steel, timber, concrete, among others); however, low-impact solutions should be preferred so as to reduce the environmental footprint of the system.
By way of example, Figures 4A,B depict Re:Slab slab elements employing conventional IPE steel beams and glue-laminated timber (GLT) beams, respectively. Early pre-designs have defined the slab elements’ dimensions as 2,400 × 2,400 mm so as to allow easy handling, transportation, and storage, and to provide a versatile element for designers upon devising floor plans. Moreover, a beam spacing of 400 mm has been defined to develop a dense grid of openings and a reduced slab thickness. For instance, 120-mm-high steel beams (IPE 120) or 240-mm-high GLT beams are required for spans of up to 6 m under the loading conditions defined by the Swiss SIA 261 standard (Swiss Society of Engineers and Architects, 2003) for office and residential structures. If required by design, slab elements of different materiality might be employed across the same floor in order to satisfy the requirements of high-stress areas. Besides, slab elements are required to have a plane of symmetry at half thickness to provide uniform capacities for both positive and negative bending moments, with the aim of coping with the uncertainty of future reuses and loading conditions of the element.
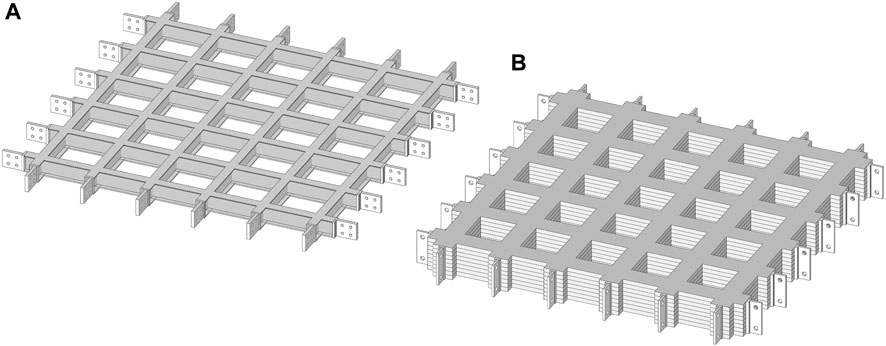
FIGURE 4. Re:Slab elements employing (A) IPE 120 steel beams and (B) layered glue-laminated timber (GLT) beams.
4.3 Slab-to-slab connections
Slab elements are assembled together by employing reversible connections for easy construction, deconstruction, and reuse. For instance, in one implemented solution, steel connectors with plates and bolts have been designed along with the Re:Slab system due to their high strength and stiffness, standardization capabilities, and simple assembly by using readily available tools. Slab elements are arranged horizontally by in-plane slab-to-slab connections that transfer axial, bending, and shear loads between elements. In addition, if properly designed, in-plane connections might act as a transfer mechanism of lateral loads to the structure’s stiff core and enable a rigid diaphragm behaviour of the flooring system at each story. Figures 5A,B depict an in-plane slab-to-slab connection for two steel slab elements, where connection plates are fixed to standard steel beams by welding to guarantee force transfer between elements. For solutions employing different materials such as concrete or timber, a complete fixing between beams and connectors shall also be guaranteed. This latter might be achieved by embedding steel bars or bolts into concrete beams or by glueing them into timber ones, as shown in Figure 5C.
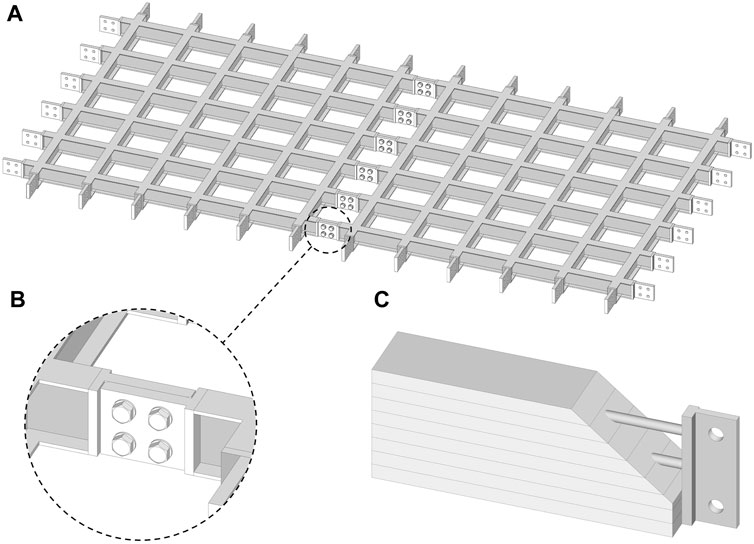
FIGURE 5. In-plane slab-to-slab connections: (A) in-plane arrangement of two steel slab elements, (B) detailing of in-plane connection, and (C) embedded connection for timber slab elements.
Slab elements can be stacked on top of each other by employing out-of-plane slab-to-slab connections in order to increase the structure’s depth and, therefore, its strength and stiffness. This is a convenient and reversible solution to strengthen high-demand zones or allow longer spans. Out-of-plane connections are designed to take the shear and axial loads generated in the slabs’ interface and, at the same time, not to interfere with vertical pipes and shafts being installed through slab openings. The perimeter faces of superimposed slabs are not required to coincide; therefore, the elements’ position can be optimized for each particular load scenario, as developed in (Muresan et al., 2018). The design and detailing of out-of-plane connections are strongly linked to the slab material; for instance, for steel I-beams, bolts and nuts can be installed through the beams’ flanges to fix two slabs and increase their structural depth. For monolithic materials such as timber, out-of-plane connections can be installed on the perimeter faces of the slab elements by employing steel bolts and plates, as shown in Figure 6.
4.4 Column elements and column-to-slab connectors
Column elements transfer vertical loads from the slab elements down to the foundation. Columns have a length equal to the floor height and a cross-section that fits within any slab opening or in between adjacent slab openings, as shown in Figure 7A; therefore, the placement of columns across the floor plan is flexible and adaptable to any engineering and architectural requirement. If requested by design, short column elements (spacers) can be employed to counter differences in height between slabs and columns if superimposed slabs are used at a given floor, as Figure 7B shows. Any structural material can be employed for column elements; nevertheless, preference should be given to lightweight and low-impact materials in order to ease the assembling process and reduce the environmental footprint of the structure. Column-to-slab connectors are placed at the end of columns to transfer the vertical loads from the upper slab. Bolts are employed to assemble the different elements of the joint in order to have a reversible and demountable connection; however, column-to-slab connectors can be embedded in the column if monolithic materials such as timber are employed, as shown in Figure 7C. It should be noted that the load-bearing system herein described is not intended to take lateral loads (such as wind or earthquake) and was designed as a gravity frame. However, slab elements must meet the in-plane stiffness requirements to properly transfer lateral loads to the stiff core or other bracing structures.
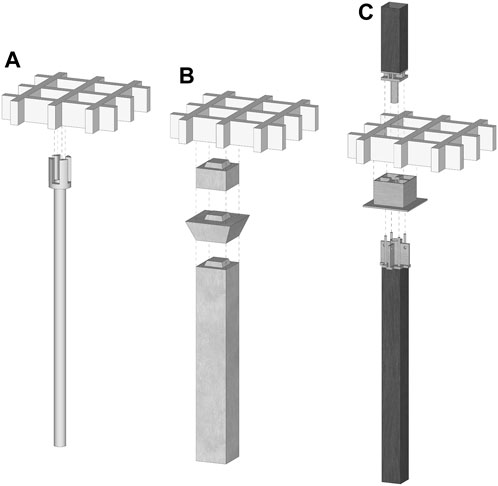
FIGURE 7. Different variations of column-to-slab connectors: (A) connector for adjacent slab openings, (B) connector for reinforced concrete columns, and (C) connector for timber columns.
4.5 Floor tiles and non-structural finishes
Floor tiles are installed over slab elements to provide a continuous surface on top of which other finishing layers can be laid out, as shown in Figure 8A. Floor tile dimensions are designed to fit into one or several slab openings at once, with joints of minimum thickness that guarantee complete sealing between elements. This allows tiles to meet the technical requirements and achieve non-structural functions such as compartmentalization (smoke barrier) or acoustic insulation. In order to ease the assembling process, lightweight materials should be preferred; however, tiles can also be devised to provide additional technical purposes such as fireproofing, thermal insulation, or thermal inertia. Tiles are fixed to slab elements and partition walls by employing reversible connections (bolts and nuts) so as to allow future unit replacements and effortless construction/deconstruction processes. Besides, as shown in Figure 8B, further flooring finishes and layers can be installed on top of floor tiles if required for technical or aesthetic purposes, and slab openings can be filled in with insulation materials to improve the non-structural performance of the system.
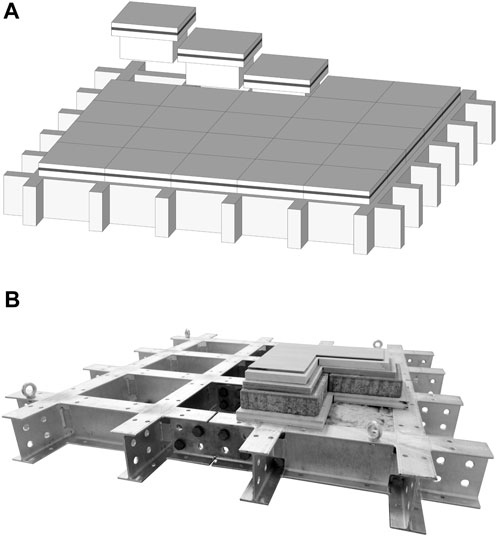
FIGURE 8. (A) Floor tiles installed over slab elements, and (B) full-scale steel prototype with non-structural finishing layers.
5 Discussion: opportunities and threats
The increasing interest in reuse among the construction community is explained by the emergence of a series of drivers of different natures in an industry well-known to be reticent to changes. As identified by Rakhshan et al. (Rakhshan et al., 2020), the main drivers encouraging reuse among policymakers and private stakeholders are economic, organizational, environmental, and social, with a growing trend towards multidisciplinary solutions that tackle technical and sustainability issues all at once. However, for reuse to reach an industrial scale and widespread adoption by practitioners and construction companies, two key aspects must be well understood by designers during early planning stages, namely construction feasibility and public engagement. This framework mimics the simplified marketing approach of retail companies when conceiving new products; first, the technological capability to develop the product should be demonstrated, and second, the market purchasing willingness should be guaranteed (or created if it does not exist).
Particular regard was paid to fitting the Re:Slab system within the aforementioned development scheme so as to maximize its market adoption and industry impact. Construction feasibility has been ensured by employing well-known materials and standard connections that do not require high-tech procedures or tools for assembling and disassembling. In this way, designing and deconstructing reusable buildings become easily accessible and less labour-intensive. Besides, deconstruction duration and transportation costs are cut down so as not to interfere with tight project schedules and budgets. Therefore, the proposed system can step smoothly into the real-estate industry without requiring major modifications to current construction procedures and equipment.
As part of the conceptual development of the Re:Slab system, a reduced-scale model (Figure 9), full-scale prototypes in steel and GLT (Figure 8B; Figure 10), and a 5.2 × 4.0 m showroom (Figure 11) were built to validate the hypotheses described above. It was found that manufacturing processes do not require specialized know-how and can be carried out by conventional companies and assembly lines. Besides, construction and deconstruction proved to be effortless, without requiring trained workers nor special tools, favouring prefabrication and dry procedures. However, special attention must be paid to dimensional tolerances to counter additional component deformations due to manufacturing imperfections, thermal effects, and creep/shrinkage. Besides, the advantages of employing standardized elements were also highlighted, whose manufacturing can be fully automated and optimized to reduce waste, costs, and delivery time, increasing the competitiveness of the system while guaranteeing high-quality construction standards.
Regarding structural and architectural design, the Re:Slab system has been developed as an alternative solution that easily integrates into traditional design methods during early project phases. By employing standard interchangeable components and connections, the proposed system overcomes reuse barriers related to element uncertainty and tied stock (Gorgolewski, 2008; Gorgolewski et al., 2008), providing a more flexible and straightforward design and resulting in non-overdesigned structures, an issue discussed by previous researchers when traditional reuse methods are employed (Pongiglione and Calderini, 2014; Brütting et al., 2019). Besides, it enables compatibility with conventional lateral load-resisting systems (such as shear walls, moment frames, or diagonal bracing), can be designed along with any foundation solution, and allows integration with conventional structural systems to carry out floor plan expansions or reach high-rise buildings (hybrid construction). Finally, the envelope design remains open and might be enhanced by the system’s components if slab openings or floor tiles are adequately integrated into the building’s ecosystem. In this sense, it provides the means to improve the overall energy performance during the structure’s lifespan.
When it comes to public engagement and market purchasing willingness, the economic and environmental advantages of the Re:Slab system might play a significant role in increasing the market share of buildings with reusable components. From an economic standpoint, employing a reusable structural system increases the equity value of real estate investments since capital conservation is guaranteed at the end-of-life of the building. Therefore, value recapturing through reuse poses a relevant competitive advantage compared to traditional systems, being especially attractive to large-scale real estate developers whose construction cycles last decades. As the growing demand for natural resources is expected to increase construction materials’ costs in the upcoming years, building practices that incorporate circular economy principles become highly attractive to public and private investors.
On the other hand, the system’s capability to reduce construction waste and greenhouse gas emissions is strongly aligned with current government policies and organizational goals of private stakeholders worldwide; therefore, market opportunities are foreseeable at several levels in the short and medium terms. However, it should be noted that the environmental benefits of the Re:Slab concept might only be exploitable in the long term. A preliminary multi-scenario Life Cycle Analysis (LCA) was conducted as part of this research project, comparing a steel Re:Slab floor with a conventional, constant height, concrete slab that fulfils the same structural requirements (Küpfer et al., 2020). Results showed that if only one lifespan is considered, the Re:Slab’s emissions are higher than those of the concrete solution. This is mainly due to the high number of steel connections in the reusable solution, where steel casting processes play a major percentage in overall CO2 emissions. However, if multiple life spans are taken into account or if more standard steel connections are employed, the Re:Slab’s environmental impact per life cycle decreases and is significantly less than that of traditional systems. For instance, in a four-lifespan scenario, emissions caused by the concrete solution were about 80% higher than those of the reusable system. Besides, it should be noted that the emissions of the Re:Slab can be further lowered if different materials are used; for example, if the slab modules are manufactured employing GLT, the upfront embodied carbon of the system can be as low as one-third of those of an equivalent non-reusable concrete slab.
These results highlight the Re:Slab potential to reduce the carbon footprint of the construction industry. Nevertheless, previous investigations have argued that emissions due to disassembly and transportation of recovered components might have adverse effects on construction processes (Huuhka and Hakanen, 2015; Brambilla et al., 2019; Nußholz et al., 2019). This latter is mostly due to the increased greenhouse gases related to longer deconstruction times, heavy machinery use, and long-distance component hauling (>1,000 km) (Brambilla et al., 2019). Future research should investigate the relation between such implications and the emission savings due to reuse and less landfilling, recycling, and new component production.
On the other hand, previous investigations have argued that stringent legislation and policy are key factors required for the success of design for deconstruction and reuse (Akinade et al., 2017). This latter does not come as a surprise, mainly due to the significant role of governments in the current global sustainability agenda (Lu and Yuan, 2010; Oyedele et al., 2014; Ajayi et al., 2015). By setting goals for component reuse and providing supporting legislation and policies to drive such goals, local and national governments can enforce an extended adoption of sustainable customs among practitioners in the construction industry. A good example of such an approach is the recent guidelines set by the French government in 2020, mandating all government-funded structures to be built with at least 50% bio-sourced materials (Crook, 2020). However, indirect measures might also have a positive impact, such as tax reductions for construction projects with reused elements or increased landfilling costs. In this way, governments should take the lead in developing friendly environments for the growth of the component reuse market and a self-sustainable supply/demand chain.
Along the same lines, the lack of technical regulations that support reuse poses a barrier ahead of the required approvals for deconstruction projects, leading regulatory bodies to have a prohibitive approach towards reuse (Rameezdeen et al., 2016). Besides, the scarcity of standard methods to evaluate and certify the residual mechanical properties of deconstructed components (Dunant et al., 2017; Huang et al., 2018) further cut down the reuse rate in the construction industry, mainly due to the uncertainty in the elements’ quality. Tingley et al. (Densley Tingley et al., 2017) highlight that this latter has a negative effect on the building cost since specific testing procedures might be required for certain projects, and higher insurance policies are quoted due to the higher perceived risk when reused components are employed. However, standardization and automatization of control tests and certification procedures for reused elements can play an essential role in lowering the cost associated with quality control while ensuring the same level of reliability of brand-new components. Future investigations should focus on the issues above, aiming at laying down the grounds for an expanded and sustainable adoption of deconstruction and reuse within the construction industry.
6 Conclusion
This paper presents the conceptual development of a new load-bearing system called Re:Slab, which was devised for deconstruction and reuse over several lifespans. By employing reversible connections and a set of standard modules, the Re:Slab system presents an alternative solution for new buildings designed for assembly and disassembly, aiming at maximizing the open-ended reuse potential of building components in future unanticipated configurations. The modular nature of the proposed system provides practitioners with a versatile design framework regarding floor geometries, spans, load conditions, and element distribution, allowing a broad spectrum of feasible implementations in terms of architecture and engineering. Furthermore, the system has been conceived to have high prefabrication levels in order to expedite its market entry and use among construction companies and private stakeholders. It is expected that a widespread application of the solution proposed in this investigation contributes to reducing the resource consumption and waste production of the construction industry and decreasing its overall environmental footprint. The main highlights of the Re:Slab system are:
• The system is reversible and can be easily disassembled without damage to its components.
• The system can be transformed into diverse floor plans and layouts since the placement of columns is freed from usual structural and geometric constraints, and the thickness of slab, i.e., its ‘static height’, is reconfigurable.
• The system is adaptable and allows parallel assembly and disassembly, i.e., slab modules and tiles can be installed, removed, or replaced in any sequence without disturbing adjacent components.
• The system allows wide integration with non-structural components and subsystems.
• No high-tech procedures and tools are required for the assembly and disassembly of the system, and only common equipment is necessary.
• Handling, storage, and transportation of deconstructed components are facilitated due to compact dimensions.
• The construction process is dry and produces no waste on-site.
• The system uses standardized elements that can be prefabricated in an automated manner with lower costs and optimal resource consumption for a reduced delivery timeframe.
Future research is still required to develop standard procedures intended to verify and certify the residual properties of deconstructed elements, to benchmark economic costs, and to better understand the environmental implications of the longer deconstruction and transportation times related to disassembly and reuse. Besides, further development of policies and regulations that support reuse is still needed to pave the way for an extended adoption of component reuse in the short term.
Data availability statement
The original contributions presented in the study are included in the article/supplementary material, further inquiries can be directed to the corresponding author.
Author contributions
XE: Writing–original draft, Writing–review and editing. AM: Conceptualization, Investigation, Methodology, Writing–review and editing. JB: Conceptualization, Investigation, Methodology, Writing–review and editing. DR: Conceptualization, Investigation, Methodology, Writing–review and editing. CF: Conceptualization, Investigation, Methodology, Writing–review and editing.
Funding
The author(s) declare financial support was received for the research, authorship, and/or publication of this article. This project has been partially funded by the Smart Living Lab, Fribourg (https://smartlivinglab.ch), which also provided technical support and infrastructure for prototyping. The patenting of the concept is done with the support of the Technology Transfer Office at EPFL. The fabrication of steel prototypes sponsored by Stephan SA, Givisiez Switzerland.
Acknowledgments
The authors thank Loran Albertoni for his contribution in developing the structural designs for several Re:Slab variations.
Conflict of interest
The authors declare that the research was conducted in the absence of any commercial or financial relationships that could be construed as a potential conflict of interest.
Publisher’s note
All claims expressed in this article are solely those of the authors and do not necessarily represent those of their affiliated organizations, or those of the publisher, the editors and the reviewers. Any product that may be evaluated in this article, or claim that may be made by its manufacturer, is not guaranteed or endorsed by the publisher.
References
ABRELPE (2018). Panorama dos resíduos sólidos no Brasil 2017. São Paulo, Brazil: Associação Brasileira de Empresas de Limpeza Pública e Resíduos Especiais.
Addis, B. (2012). Building with reclaimed components and materials: a design handbook for reuse and recycling. Taylor and Francis GroupRoutledge.
Ajayi, S. O., Oyedele, L. O., Bilal, M., Akinade, O. O., Alaka, H. A., Owolabi, H. A., et al. (2015). Waste effectiveness of the construction industry: understanding the impediments and requisites for improvements. Resour. Conserv. Recycl 102, 101–112. doi:10.1016/j.resconrec.2015.06.001
Akinade, O. O., Oyedele, L. O., Ajayi, S. O., Bilal, M., Alaka, H. A., Owolabi, H. A., et al. (2017). Design for Deconstruction (DfD): critical success factors for diverting end-of-life waste from landfills. Waste Manag. 60, 3–13. doi:10.1016/j.wasman.2016.08.017
Aninthaneni, P. K., and Dhakal, R. P (2017). Demountable precast concrete frame–building system for seismic regions: conceptual development. Journal of Architectural Engineering 23 (4), 4017024. doi:10.1061/(ASCE)AE.1943-5568.0000275
Benachio, G. L. F., Freitas, M. D. C., and Tavares, S. F. (2020). Circular economy in the construction industry: a systematic literature review. J. Clean. Prod. 260, 121046. doi:10.1016/j.jclepro.2020.121046
Bertram, N., Fuchs, S., Mischke, J., Palter, R., Strube, G., and Woetzel, J. (2019). Modular construction: from projects to products report published by the consulting company. McKinsey and Company.
Brambilla, G., Lavagna, M., Vasdravellis, G., and Castiglioni, C. A. (2019). Environmental benefits arising from demountable steel-concrete composite floor systems in buildings. Resour. Conserv. Recycl 141, 133–142. doi:10.1016/j.resconrec.2018.10.014
Braungart, M., McDonough, W., and Bollinger, A. (2007). Cradle-to-cradle design: creating healthy emissions – a strategy for eco-effective product and system design. J. Clean. Prod. 15 (13), 1337–1348. doi:10.1016/j.jclepro.2006.08.003
Brütting, J., Desruelle, J., Senatore, G., and Fivet, C. (2019). Design of truss structures through reuse. Structures 18, 128–137. doi:10.1016/j.istruc.2018.11.006
Brütting, J., Senatore, G., Schevenels, M., and Fivet, C. (2020). Optimum design of frame structures from a stock of reclaimed elements. Front. Built Environ. 6, 57. doi:10.3389/fbuil.2020.00057
Cai, G., Xiong, F., Xu, Y., Si Larbi, A., Lu, Y., and Yoshizawa, M. (2019). A demountable connection for low-rise precast concrete structures with DfD for construction sustainability-A preliminary test under cyclic loads. Sustainability 11 (13), 3696. doi:10.3390/su11133696
Chen, C., Habert, G., Bouzidi, Y., and Jullien, A. (2010). Environmental impact of cement production: detail of the different processes and cement plant variability evaluation. J. Clean. Prod. 18 (5), 478–485. doi:10.1016/j.jclepro.2009.12.014
Cheng, E. W. L., Chiang, Y. H., and Tang, B. S. (2006). Exploring the economic impact of construction pollution by disaggregating the construction sector of the input–output table. Build. Environ. 41 (12), 1940–1951. doi:10.1016/j.buildenv.2005.06.020
Chourasia, A., Singhal, S., and Manivannan, (2023). Prefabricated volumetric modular construction: a review on current systems, challenges, and future prospects. Pract. Periodical Struct. Des. Constr. 28 (1), 3122009. Available from: https://ascelibrary.org/doi/abs/10.1061/PPSCFX.SCENG-1185. doi:10.1061/PPSCFX.SCENG-1185
Crook, L. (2020). French public buildings to be built with 50 per cent wood. Available from: https://www.dezeen.com/2020/02/12/france-public-buildings-sustainability-law-50-per-cent-wood/ (Accessed June 24, 2021).
Defra, U. K. (2019). Statistics on waste – february 2019 update. York UK: Department for Environment Food and Rural Affairs.
Densley Tingley, D., Cooper, S., and Cullen, J. (2017). Understanding and overcoming the barriers to structural steel reuse, a UK perspective. J. Clean. Prod. 148, 642–652. doi:10.1016/j.jclepro.2017.02.006
Donovan, I., Schnitzler, J., Lee, K. J., Wongsittikan, P., Liu, Y., and Mueller, C. T. (2023). PixelFrame: a reconfigurable, precast, post-tensioned concrete structural system for a circular building economy. J. Phys. Conf. Ser. 2600 (19), 192007. doi:10.1088/1742-6596/2600/19/192007
Dunant, C. F., Drewniok, M. P., Sansom, M., Corbey, S., Allwood, J. M., and Cullen, J. M. (2017). Real and perceived barriers to steel reuse across the UK construction value chain. Resour. Conserv. Recycl 126, 118–131. doi:10.1016/j.resconrec.2017.07.036
Durmisevic, E. (2006). Transformable building structures: design for dissassembly as a way to introduce sustainable engineering to building design and construction. . Delft: Ph.D. Dissertation, Faculty of Architecture, Delft University of Technology.
Durmisevic, E. (2019a). Circular economy in construction - design strategies for reversible buildings. Delf, Netherlands: University of Twente.
Durmisevic, E. (2019b). Explorations for reversible buildings. Delf, Netherlands: University of Twente.
Elhacham, E., Ben-Uri, L., Grozovski, J., Bar-On, Y. M., and Milo, R. (2020). Global human-made mass exceeds all living biomass. Nature 588 (7838), 442–444. doi:10.1038/s41586-020-3010-5
Ellen MacArthur Foundation (2013). Towards the circular economy: economic and business rationale for an accelerated transition. United Kingdom: Cowes.
Ellen MacArthur Foundation (2015). Towards a circular economy: business rationale for an accelerated transition. United Kingdom: Cowes.
Ellen MacArthur Foundation (2016). Circularity in the built environment: case studies. United Kingdom: Cowes.
Erman, E. (2002). Demountable timber joints for timber construction systems. Archit. Sci. Rev. 45 (2), 133–143. doi:10.1080/00038628.2002.9697501
Estrella, X., Guindos, P., Almazán, J., and Malek, S. (2020). Efficient nonlinear modeling of strong wood frame shear walls for mid-rise buildings. Eng. Struct. 215, 110670–110715. doi:10.1016/j.engstruct.2020.110670
Estrella, X., Guindos, P., Almazán, J. L., Malek, S., Santa María, H., Montaño, J., et al. (2021b). Seismic performance factors for timber buildings with woodframe shear walls. Eng. Struct. 248, 113185. doi:10.1016/j.engstruct.2021.113185
Estrella, X., Malek, S., Almazán, J. L., Guindos, P., and Santa María, H. (2021a). Experimental study of the effects of continuous rod hold-down anchorages on the cyclic response of wood frame shear walls. Eng. Struct. 230, 111641. doi:10.1016/j.engstruct.2020.111641
European Commission (2018). Development and implementation of initiatives fostering investment and innovation in construction and demolition waste recycling infrastructure. Brussels, Belgium: European Commission.
Fivet, C. (2019). “Design of load-bearing systems for open-ended downstream reuse,” in IOP Conference Series: Earth and Environmental Science (IOP Publishing), 12031.
Fivet, C., and Brütting, J. (2020). Nothing is lost, nothing is created, everything is reused: structural design for a circular economy. Struct. Eng. 98 (1), 74–81. doi:10.56330/lxah1188
Gann, D. M. (1996). Construction as a manufacturing process? Similarities and differences between industrialized housing and car production in Japan. Constr. Manag. Econ. 14 (5), 437–450. doi:10.1080/014461996373304
Generalova, E. M., Generalov, V. P., and Kuznetsova, A. A. (2016). Modular buildings in modern construction. Procedia Eng. 153, 167–172. doi:10.1016/j.proeng.2016.08.098
Gorgolewski, M. (2008). Designing with reused building components: some challenges. Build. Res. Inf. 36 (2), 175–188. doi:10.1080/09613210701559499
Gorgolewski, M., Straka, V., Edmonds, J., and Sergio-Dzoutzidis, C. (2008). Designing buildings using reclaimed steel components. J. Green Build. 3 (3), 97–107. doi:10.3992/jgb.3.3.97
Grooten, M., and Almond, R. E. A. (2018). WWF. Living planet report - 2018: aiming higher (Gland, Switzerland: WWF).
Habraken, N. J. (2003). “Open building as a condition for industrial construction,” in 20th International Symposium on Automation and Robotics in Construction, Eindhoven, the Netherlands, 37–42.
Hairstans, R., Smith, R., and Wilson, P. (2018). “The merits of varying forms of mass timber products for offsite and modular construction,” in Modular and offsite construction (MOC) summit proceedings (FL, USA: Hollywood).
Herczeg, M., McKinnon, D., Milios, L., Bakas, I., Klaassens, E., Svatikova, K., et al. (2014). Resource efficiency in the building sector. Rotterdam Netherlands: ECORYS Nederland BV.
Huang, B., Wang, X., Kua, H., Geng, Y., Bleischwitz, R., and Ren, J. (2018). Construction and demolition waste management in China through the 3R principle. Resour. Conserv. Recycl 129, 36–44. doi:10.1016/j.resconrec.2017.09.029
Huuhka, S., and Hakanen, J. (2015). Potential and barriers for reusing load-bearing building components in Finland. Int. J. Hous. Sci. Its Appl. 39 (4), 215–224.
John Habraken, N. (2008). Design for flexibility. Build. Res. Inf. 36 (3), 290–296. doi:10.1080/09613210801995882
Kalyun, M., and Wodajo, T. (2012). Application of a design method for manufacture and assembly, flexible assembly methods and their evaluation for the construction of bridges. Goteborg, Sweden: Master’s Thesis, Chalmers University of Technology.
Karimah, A., and Paramita, K. D. (2020). Investigating the domestic layers adaptation during pandemic. Interiority 3 (2), 185–200. doi:10.7454/in.v3i2.101
Kendall, S. H., and Teicher, J. (2010). Residential open building. Taylor and Francis Group: Routledge.
Kibert, C. J. (2016). Sustainable construction: green building design and delivery. New Jersey, USA: John Wiley and Sons.
Kinzig, A. P., Ehrlich, P. R., Alston, L. J., Arrow, K., Barrett, S., Buchman, T. G., et al. (2013). Social norms and global environmental challenges: the complex interaction of behaviors, values, and policy. Bioscience 63 (3), 164–175. doi:10.1525/bio.2013.63.3.5
Kozma, A., Odenbreit, C., Braun, M. V., Veljkovic, M., and Nijgh, M. P. (2019). Push-out tests on demountable shear connectors of steel-concrete composite structures. Structures 21, 45–54. doi:10.1016/j.istruc.2019.05.011
Küpfer, C., Lambec, B., Louvet, A., Reboul, A., and Gutierrez-Navarro, D. (2020). Comparative life-cycle assessment of “(Reu)Slab.” course ENV510 report. Lausanne, Switzerland: École Polytechnique Fédérale de Lausanne.
Lawson, M., Ogden, R., and Goodier, C. I. (2014). Design in modular construction, 476. Boca Raton, FL: CRC Press.
Lawson, R. M., Ogden, R. G., and Bergin, R. (2012). Application of modular construction in high-rise buildings. J. Archit. Eng. 18 (2), 148–154. doi:10.1061/(asce)ae.1943-5568.0000057
Liberman, B. (2014). Modular building structures. Patent published by the US Patent and Trademark Office. US8844242B2.
Liu, G., Xu, K., Zhang, X., and Zhang, G. (2014). Factors influencing the service lifespan of buildings: an improved hedonic model. Habitat Int. 43, 274–282. doi:10.1016/j.habitatint.2014.04.009
López Ruiz, L. A., Roca Ramón, X., and Gassó Domingo, S. (2020). The circular economy in the construction and demolition waste sector – a review and an integrative model approach. J. Clean. Prod. 248, 119238. doi:10.1016/j.jclepro.2019.119238
Lu, N., and Liska, R. W. (2008). Designers’ and general contractors’ perceptions of offsite construction techniques in the United State construction industry. Int. J. Constr. Educ. Res. 4 (3), 177–188. doi:10.1080/15578770802494565
Lu, W., and Yuan, H. (2010). Exploring critical success factors for waste management in construction projects of China. Resour. Conserv. Recycl 55 (2), 201–208. doi:10.1016/j.resconrec.2010.09.010
Moore, D., Cranston, G., Reed, A., and Galli, A. (2012). Projecting future human demand on the Earth’s regenerative capacity. Ecol. Indic. 16, 3–10. doi:10.1016/j.ecolind.2011.03.013
Muresan, A., Brütting, J., Cañada, J., Redaelli, D., and Fivet, C. (2018). “Design space of modular slab systems with discrete stiffness distribution and irregular column layout,” in Proceedings of the IASS Symposium 2018, Boston, USA.
Muresan, A., Brütting, J., Radaelli, D., and Fivet, C. (2020). “Sustainability through reuse: a reconfigurable structural system for residential and office buildings,” in BEYOND 2020 – World Sustainable Built Environment Conference.
Nußholz, J. L. K., Nygaard Rasmussen, F., and Milios, L. (2019). Circular building materials: carbon saving potential and the role of business model innovation and public policy. Resour. Conserv. Recycl 141, 308–316. doi:10.1016/j.resconrec.2018.10.036
O’Neill, D. W., Fanning, A. L., Lamb, W. F., and Steinberger, J. K. (2018). A good life for all within planetary boundaries. Nat. Sustain 1 (2), 88–95. doi:10.1038/s41893-018-0021-4
Orellana, P., Santa María, H., Almazán, J. L., and Estrella, X. (2021). Cyclic behavior of wood-frame shear walls with vertical load and bending moment for mid-rise timber buildings. Eng. Struct. 240, 112298. doi:10.1016/j.engstruct.2021.112298
Oyedele, L. O., Ajayi, S. O., and Kadiri, K. O. (2014). Use of recycled products in UK construction industry: an empirical investigation into critical impediments and strategies for improvement. Resour. Conserv. Recycl 93, 23–31. doi:10.1016/j.resconrec.2014.09.011
Pongiglione, M., and Calderini, C. (2014). Material savings through structural steel reuse: a case study in Genoa. Resour. Conserv. Recycl 86, 87–92. doi:10.1016/j.resconrec.2014.02.011
Rakhshan, K., Morel, J. C., Alaka, H., and Charef, R. (2020). Components reuse in the building sector – a systematic review. Waste Manag. Res. 38 (4), 347–370. doi:10.1177/0734242x20910463
Rameezdeen, R., Chileshe, N., Hosseini, M. R., and Lehmann, S. (2016). A qualitative examination of major barriers in implementation of reverse logistics within the South Australian construction sector. Int. J. Constr. Manag. 16 (3), 185–196. doi:10.1080/15623599.2015.1110275
Remøy, H., and van der Voordt, T. (2014). Adaptive reuse of office buildings into housing: opportunities and risks. Build. Res. Inf. 42 (3), 381–390. doi:10.1080/09613218.2014.865922
Segall, S. (2018). Relocatable habitat unit. US. Patent published by the US Patent and Trademark Office. US10036157B2.
Steffen, W., Broadgate, W., Deutsch, L., Gaffney, O., and Ludwig, C. (2015). The trajectory of the anthropocene: the great acceleration. Anthropocene Rev. 2 (1), 81–98. doi:10.1177/2053019614564785
Swiss Society of Engineers and Architects (2003). Sia 261: actions on structures. Switzerland: Zürich.
United Nations (2017). Towards a zero emission, efficient, and resilient buildings and construction sector,” in Global status report 2017”. United Nations Environment and International Energy Agency.
United Nations (2017). World population prospects: the 2017 revision, key findings and advance tables. New York, USA: Department of Economic and Social Affairs.
Uy, B., Patel, V., Li, D., and Aslani, F. (2017). Behaviour and design of connections for demountable steel and composite structures. Structures 9, 1–12. doi:10.1016/j.istruc.2016.06.005
Wilson, J., and Wilson, T. (2017). Modular housing units, reusable support structure, and utility connector. US: United States Patent and Trademark Office. US9617748B2.
WST (2012). Sustainable steel: at the core of a green econom. Brussels, Belgium: World Steel Association.
Keywords: component reuse, design for disassembly & reuse, adaptability, circular economy, sustainable buildings, construction and demolition waste, material recovery, structural design
Citation: Estrella X, Muresan A, Brütting J, Redaelli D and Fivet C (2024) RE:SLAB—a load bearing system for open-ended component reuse in building structures. Front. Built Environ. 10:1355445. doi: 10.3389/fbuil.2024.1355445
Received: 13 December 2023; Accepted: 31 January 2024;
Published: 09 February 2024.
Edited by:
Aysu Kuru, The University of Sydney, AustraliaReviewed by:
Bernardino D’Amico, Edinburgh Napier University, United KingdomMohammad Afrazi, Tarbiat Modares University, Iran
Copyright © 2024 Estrella, Muresan, Brütting, Redaelli and Fivet. This is an open-access article distributed under the terms of the Creative Commons Attribution License (CC BY). The use, distribution or reproduction in other forums is permitted, provided the original author(s) and the copyright owner(s) are credited and that the original publication in this journal is cited, in accordance with accepted academic practice. No use, distribution or reproduction is permitted which does not comply with these terms.
*Correspondence: Xavier Estrella, edisson.estrellaarcos@epfl.ch