LOX-1 Regulates P. gingivalis-Induced Monocyte Migration and Adhesion to Human Umbilical Vein Endothelial Cells
- 1Department of Periodontology, Peking University School and Hospital of Stomatology, Beijing, China
- 2First Clinical Division, Peking University School and Hospital of Stomatology, Beijing, China
Porphyromonas gingivalis (P. gingivalis) is one of the main periodontal bacteria. This pathogen was reported to enhance monocyte migration and adhesion to endothelial cells in atherosclerosis. The scavenger receptor lectin-like oxidized low-density lipoprotein receptor-1 (LOX-1) plays a pivotal role in atherogenesis. The aim of this study was to investigate whether LOX-1 modulates P. gingivalis-mediated monocyte migration and adhesion to endothelial cells and how it works. The results showed that the migration and adhesion of monocytic THP-1 cells to human umbilical vein endothelial cells (HUVECs) were significantly enhanced when HUVECs or THP-1 cells were challenged with P. gingivalis. Meanwhile, the expression level of LOX-1 in both HUVECs and THP-1 cells were also significantly increased by P. gingivalis stimulation. It is well known that ligand/receptor pairs monocyte chemoattractant protein-1 (MCP-1)/CC chemokine receptor 2 (CCR2), selectins/Integrins, and cell adhesion molecules (CAMs)/Integrins mediate monocyte migration and adhesion to endothelial cells. In this study, LOX-1 was demonstrated to be crucially involved in P. gingivalis-induced THP-1 cell migration and adhesion to HUVECs, by regulating expression of ligands MCP-1, intercellular adhesion molecule-1 (ICAM-1) and E-selectin in HUVECs and that of their receptors CCR2 and Integrin αMβ2 in THP-1 cells. The nuclear factor-kappa B (NF-κB) signaling pathway was proved to be involved in this process. In conclusion, LOX-1 plays a crucial role in P. gingivalis-induced monocyte migration and adhesion to endothelial cells. This result implies LOX-1 may act as a bridge in linking periodontitis to atherosclerosis.
Introduction
Atherosclerosis is the major etiology of cardiovascular disease, the leading cause of mortality worldwide (Mayer and Binder, 2019). The activation of endothelial cells, adhesion of monocytes, proliferation of smooth muscle cells, and formation of atherosclerotic plaques are the main pathological processes of atherosclerosis (Kaski, 2011; Theodorou and Boon, 2018). In atherogenesis, monocyte migration and adhesion to endothelial cells are crucial events. A tightly regulated multistep process involving ligand-receptor interactions is contributed to these events. Endothelial cells express various inflammatory molecules when they are activated by kinds of stimuli such as low-density lipoprotein, hyperlipidemia, low shear stress, and TNF-α (Mestas and Ley, 2008). These inflammatory molecules then interact with their receptors expressed in monocytes to mediate the recruitment, retention and firm adhesion of circulating monocytes. Among them, monocyte chemoattractant protein-1 (MCP-1) is a pivotal molecule which mobilizes monocytes by interacting with receptor C-C chemokine receptor type 2 (CCR2) (Bianconi et al., 2018). In addition, selectins and cell adhesion molecules (CAMs) interact with Integrins such as Integrin αMβ2, Integrin α4β1, and Integrin αLβ2, to regulate the subsequent rolling and firm adhesion of monocytes (Jaipersad et al., 2014). Therefore, the expression of MCP-1, selectins, CAMs and their receptors is extremely crucial for monocyte migration and adhesion to endothelial cells. After migrating and adhering to endothelial cells, monocytes would emigrate into the intima and become lipid-laden macrophages, which contribute to the formation of atherosclerotic plaques (Jaipersad et al., 2014).
Lectin-like oxidized LDL receptor-1 (LOX-1), a class E scavenger receptor belonging to the family of pattern recognition receptors (PRRs), was reported to play a critical role in atherosclerosis. It was originally identified in endothelial cells, and subsequently found to be expressed in other cells such as macrophages, smooth muscle cells (SMC), and platelets (Nvk et al., 2017). LOX-1 expression is at a low level under physiological conditions but can be upregulated by pathogens, oxidized low-density lipoprotein (ox-LDL), and proinflammatory stimuli such as the cytokines tumor necrosis factor α (TNF-α) and transfer growth factor β (TGF-β) (Navarra et al., 2010). Once activated, LOX-1 can initiate a cascade of intracellular signaling pathways, mainly involving MAPK, PI3K/Akt, JNK, PKC, NF-κB, PPAR-γ pathways, finally resulting in endothelial cell dysfunction, monocyte migration and adhesion, SMC growth and migration, and platelet aggregation, which are all key steps in atherogenesis (Zhu, 2005; Lu et al., 2011).
Periodontitis is a bacteria-initiated disease of the periodontium (Cardoso et al., 2018). This oral disease is the sixth most prevalent disease worldwide, affecting approximately 50% of the global population (West, 2019). It has been confirmed to be associated with an increased risk of atherosclerosis by multiple epidemiological and interventional studies. Porphyromonas gingivalis (P. gingivalis), which serves as a keystone pathogen in periodontitis, is implicated in various processes of atherosclerosis by direct invasion or systemic cytokine release (Amar and Engelke, 2015). Although P. gingivalis has been demonstrated to enhance monocyte migration and adhesion to endothelial cells (Hashizume et al., 2011; Zhou et al., 2011), the exact mechanisms are less well understood.
LOX-1 is reported to recognize bacteria such as S. aureus, Escherichia coli (Shimaoka et al., 2001) and Chlamydia pneumonia (Campbell et al., 2013). However, no studies have focused on the relationship between LOX-1 and periodontal pathogen P. gingivalis yet. Whether LOX-1 modulates the P. gingivalis-mediated monocyte migration and adhesion to endothelial cells also remains unexplored. Our study was designed to investigate whether and how LOX-1 modulates P. gingivalis-mediated monocyte migration and adhesion to endothelial cells.
Materials and Methods
Cell Culture
Human umbilical vein endothelial cells (HUVECs) and human monocytic THP-1 cells were purchased from ScienCell (California, United States). HUVECs were cultured in Endothelial Cell Medium (ECM; ScienCell), supplemented with 10% fetal calf serum (FBS; ScienCell) and 200 ng/ml endothelial cell growth supplements (ECGs; ScienCell) at 37°C with 5% CO2 in a humidified atmosphere. HUVECs from passages 3–6 were used for all experiments. THP-1 cells were maintained in RPMI 1640 medium (HyClone, Logan, UT, United States) containing 10% FBS (ScienCell) in a humidified atmosphere (37°C, 5% CO2).
Small Interfering RNA Transfection and Lentiviral Transduction
LOX-1-specific small interfering RNAs (siRNAs) including siLOX-1-1, siLOX-1-2, and siLOX-1-3 and non-targeting control siRNA (scrambled) were synthesized by Sangon Biotech (Shanghai, China). The sequences of siRNAs are listed in Table 1. The LOX-1-specific siRNAs and control siRNA (20 nM) were transfected into HUVECs and THP-1 cells using Lipofectamine3000® reagent (Invitrogen, Carlsbad, United States), according to the manufacturer’s instructions. After 48 h, Western blotting was applied to validate the effectiveness of RNA interference (RNAi).
Lentiviruses encoding the LOX-1 gene (LV-LOX-1) or containing a LOX-1-specific short hairpin RNA (LV-shLOX-1) as well as their respective controls (LV-Con1 and LV-Con2) were constructed by GeneChem (Shanghai, China). Their sequences are shown in Table 1. HUVECs and THP-1 cells were transduced with the lentiviral particles at a multiplicity of infection (MOI) of 10:1 in the presence of 10 ng/ml polybrene (Sigma-Aldrich, St Louis, MO, United States). The virus-containing medium was removed after 24 h and replaced with fresh medium. The transduction efficiency was assessed by Western blotting at 72–96 h after lentiviral transduction.
Bacterial Culture
Porphyromonas gingivalis strain W83 was a gift from Prof. Chenxiong Lai at Kaohsiung University. The P. gingivalis was grown for 4–6 days on brain heart infusion (BHI) blood agar plates (BD Biosciences, California, United States) which contained 5% defibrinated sheep blood, 5 mg/ml yeast extract, 5 μg/ml hemin, and 1 μg/ml vitamin K1 (Sigma-Aldrich) in an anaerobic system (10% H2, 85% N2, and 5% CO2) at 37°C. Bacterial colonies were then inoculated into BHI broth medium supplemented with 5 μg/ml hemin, and 1 μg/ml vitamin K1, and cultured for 24 h. The bacteria were then harvested by centrifugation (6000 rpm, 4°C, 10 min), washed with phosphate buffered salt solution (PBS, PH = 7.2), and resuspended in antibiotic-free cell medium. Bacterial resuspension was adjusted to an optical density (OD) of 0.5 at 600 nm, corresponding to a concentration of 108 CFU/ml.
Bacterial Challenge
Bacterial challenge assay was conducted as previously described (Wan et al., 2015). Briefly, the prepared bacterial resuspension was added to HUVECs monolayers or to THP-1 cells at a MOI of 100:1 for 2 h, after which the medium was replaced with fresh medium containing 0.5 mg/ml gentamicin and 0.1 mg/ml metronidazole (Zhongshan Golden Bridge, Beijing, China). Subsequently, the HUVECs and THP-1 cells were cultured for indicated times. The duration of P. gingivalis stimulation was the sum of these two periods. This treatment has been shown not to affect the viability of cells (Wan et al., 2015).
Inhibition of NF-κB Signaling Pathway
HUVECs and THP-1 cells were preincubated, respectively, with ammonium pyrrolidinedithiocarbamate (PDTC; Sigma-Aldrich), an inhibitor of NF-κB activation, at a concentration of 100 μM for 1 h before they were further challenged with P. gingivalis for 24 h. Likewise, LOX-1-overexpressing HUVECs and LOX-1-overexpressing THP-1 cells were treated with PDTC (100 μM) for 24 h before cells were harvested.
Migration Assay
The impact of P. gingivalis on migration of THP-1 cells toward HUVECs was determined using 24-well transwell systems (8-μm pore size; Corning, New York, United States).
On one hand, untreated HUVECs, HUVECs with or without LOX-1 knockdown (si-LOX-1 and scrambled, respectively), as well as LOX-1-overexpressing (LV-LOX-1) and control (LV-Con1) HUVECs (2 × 105 cells/well) were seeded, respectively, in the lower compartments containing ECM with 10% FBS to form confluent monolayers. Among them, the untreated HUVECs, and HUVECs with or without LOX-1-knockdown were challenged with P. gingivalis for 24 h or left untreated. At the same time, untreated THP-1 cells were labeled with calcein AM (Thermo Fisher, Waltham, MA, United States) or Hoechst 33342 (Sigma-Aldrich) for 30 min at 37°C, according to the manufacturer’s instructions, before being resuspended in RPMI 1640 medium (HyClone) with 10% FBS. Then, the labeled THP-1 cells were plated into the upper inserts (1 × 105 cells/well) and incubate with the primed HUVECs monolayers for 6 h at 37°C.
On the other hand, untreated HUVECs were seeded at a density of 2 × 105 cells per well in the lower compartments containing ECM with 10% FBS to form confluent monolayers. Untreated THP-1 cells, and THP-1 cells with or without LOX-1 deficiency (si-LOX-1 and scrambled, respectively) were challenged with P. gingivalis for 24 h or left untreated. These THP-1 cells as well as LOX-1-overexpressing (LV-LOX-1) and control (LV-Con1) THP-1 cells were labeled with calcein AM (Thermo Fisher) or Hoechst 33342 (Sigma-Aldrich), and resuspended in serum free RPMI 1640 medium, respectively. They were then added into the upper chambers at a concentration of 1 × 105 cells/well and incubated with the monolayers of HUVECs in the lower chambers for 6 h at 37°C.
The fluorescence microscopy (Nikon, Tokyo, Japan) was applied to visualize and capture THP-1 cells entering into the lower chambers. Migrated THP-1 cells were counted from five random fields of view and quantified by Image J software (NIH, Bethesda, United States).
Adhesion Assay
Adhesion assay was performed in 24-well plates to measure the impact of P. gingivalis on adhesion of THP-1 cells to HUVECs.
On one hand, untreated HUVECs, LOX-1-knockdown (si-LOX-1) and control (scrambled) HUVECs, as well as LOX-1-overexpressing (LV-LOX-1) and control (LV-Con2) HUVECs (2 × 105 cells/well) were seeded, respectively, in plates containing ECM with 10% FBS to form confluent monolayers. The untreated HUVECs, LOX-1-knockdown and control HUVECs were challenged with P. gingivalis for 24 h or left untreated. At the same time, according to the manufacturer’s instructions, untreated THP-1 cells were labeled for 30 min with Hoechst 33342 (Sigma-Aldrich) at 37°C. They were then resuspended in ECM with 10% FBS and seeded at a density of 1 × 105 cells/well onto the primed HUVECs monolayers, respectively, followed by incubation for 2 h at 37°C.
On the other hand, the 24-well plates were seeded with untreated HUVECs at 2 × 105 cells per well to form confluent monolayers. Untreated THP-1 cells, LOX-1 deficient and control THP-1 cells were challenged with P. gingivalis for 24 h or left untreated. These THP-1 cells as well as LOX-1-overexpressing and control THP-1 cells were labeled with Hoechst 33342 (Sigma-Aldrich) and resuspended in ECM with 10% FBS, respectively. After that the prepared THP-1 cells were plated onto the HUVECs monolayers and incubated for 2 h at 37°C.
Non-adherent THP-1 cells were gently washed with PBS, and the adherent THP-1 cells were imaged with a fluorescence microscope (Nikon, Tokyo, Japan). The number of adherent THP-1 cells were counted in at least five randomly selected areas and quantified using Image J software (NIH).
RNA Extraction and Real-Time PCR Analysis
Total RNA was extracted from cultured cells using TRIzol reagent (Sigma-Aldrich). 1 μg aliquots of RNA from each sample were reverse transcribed into cDNA using the PrimeScript RT reagent Kit (TaKaRa, Kusatsu, Japan). SYBR® Premix Ex Taq (Takara) was used to perform Real-time PCR as described previously (Liu et al., 2018). The expression of target genes was quantified by the comparative 2–Δ Δ Ct method after normalization to glyceraldehyde-3-phosphate dehydrogenase (GAPDH) expression. The primers specific to LOX-1, MCP-1, ICAM-1, E-selectin, Integrin αM, Integrin β2, CCR2, and GADPH are listed in Table 2.
Western Blotting
Total cellular proteins were extracted from cultured cells using the protein extraction kit (Solarbio, Beijing, China). An equal amount of protein (40 μg) from each sample was subjected to SDS-PAGE and then transferred onto polyvinylidenedifluoride (PVDF) membranes (Thermo Fisher). After blocking with 5% skimmed milk at room temperature for 1 h, the membranes were incubated with primary antibodies at 4°C overnight followed by incubation with horseradish peroxidase (HRP)-conjugated anti-rabbit or anti-mouse secondary antibodies (1:10000, ZSGB-Bio, Beijing, China) for 1 h at room temperature. Finally, the protein bands were imaged with Chemidox XRS (Bio-Rad, Hercules, United States) and quantified with Quantity One software (Bio-Rad). The following primary antibodies were used: LOX-1, ICAM-1, E-selectin, Integrin αM, Integrin β2 (1:1000, Bioss, Beijing, China), CCR2 (1:200, Santa Cruze, Delaware Ave, United States), MCP-1, p65, p–p65 (1:1000, Abcam, Cambridge, United Kingdom) and GADPH (1:1000, Protein, Wuhan, China).
Statistical Analysis
The statistical analysis was performed using GraphPad Prism 7.0 software (GraphPad Software Inc, La Jolla, United States). Unpaired two-tailed Student’s t-test for two groups was used. Analysis of variance (ANOVA) followed by Dunnett’s multiple comparison test or Turkey’s multiple comparison test for multiple groups was carried out. Results are presented as the mean ± standard deviation (SD) from at least three independent experiments. ∗P < 0.05 was considered to be statistically significant. NS indicated no significance.
Results
Porphyromonas gingivalis Promotes the Migration and Adhesion of THP-1 Cells to HUVECs
Cell migration and adhesion assays were performed to detect the effects of P. gingivalis on monocyte migration and adhesion to endothelial cells. When HUVECs were challenged with P. gingivalis for 24 h, the number of THP-1 cells migrated to the HUVECs markedly increased approximately 4.8-fold (Figure 1A), and the number of THP-1 cells adhered to the HUVECs increased approximately 7-fold (Figure 1B). Similarly, treatment of THP-1 cells with P. gingivalis for 24 h also enhanced the migration and adhesion of the THP-1 cells to HUVECs (Figures 1C,D). The number of THP-1 cells that migrated and adhered to HUVECs increased approximately 3.4-fold (Figure 1C) and 8-fold (Figure 1D), respectively. These data demonstrate that the migration and adhesion of THP-1 cells to HUVECs are promoted by P. gingivalis.
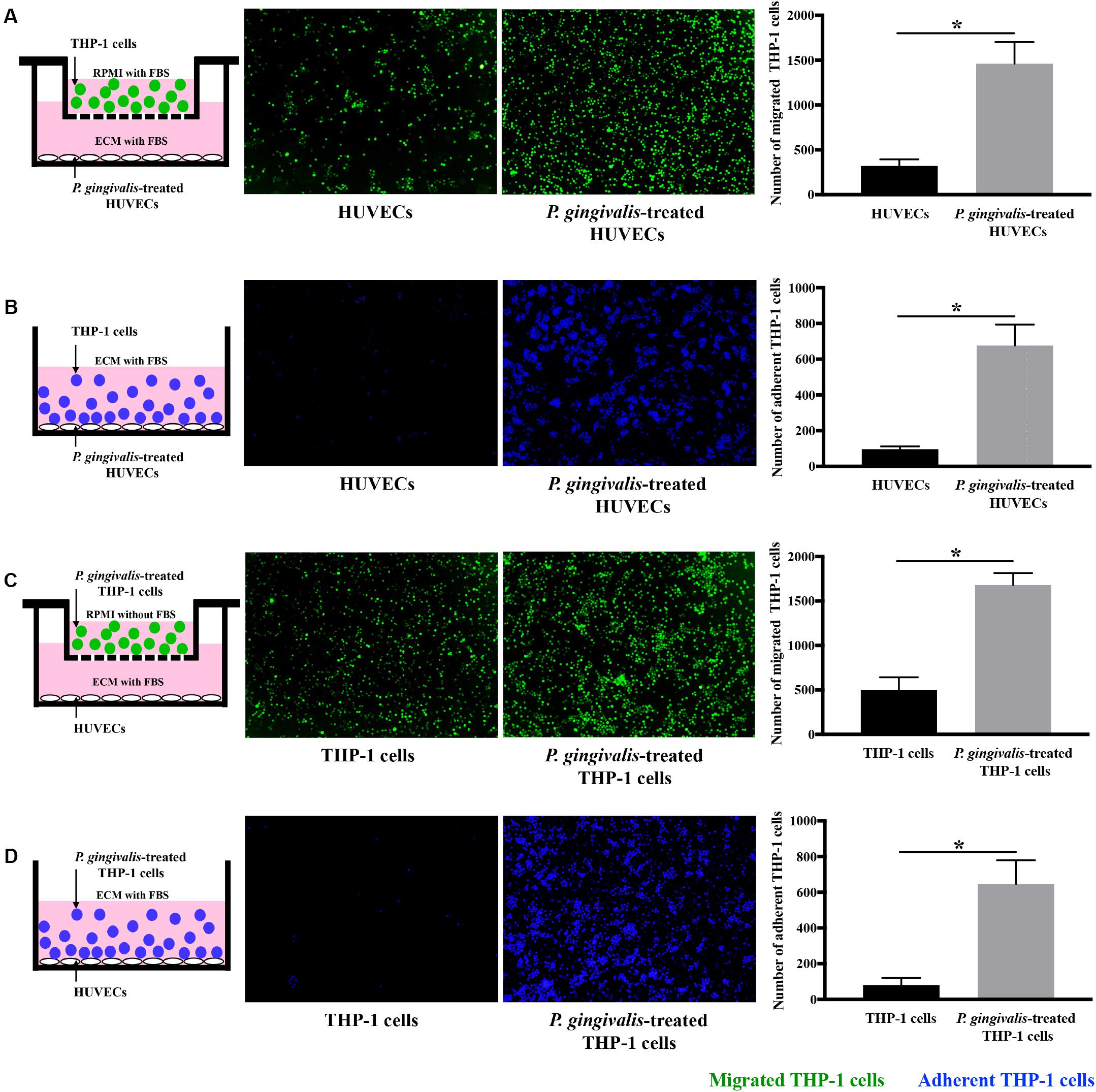
Figure 1. Porphyromonas gingivalis promotes THP-1 cell migration and adhesion to HUVECs. (A) HUVECs in the lower chambers of transwell systems remained unchallenged or were challenged with P. gingivalis for 24 h. Calcein AM-labeled THP-1 cells were loaded into the upper inserts. After incubation for 6 h, the migrated THP-1 cells with green fluorescence were visualized and counted. (B) HUVECs in 24-well plates were exposed to P. gingivalis for 24 h or untreated. Hoechst 33342-labeled THP-1 cells were added to the HUVECs and incubated for 2 h. After removing the non-adherent THP-1 cells, the adherent THP-1 cells with blue fluorescence were measured. (C) THP-1 cells were not treated or treated with P. gingivalis for 24 h, labeled with calcein AM, and then loaded into the upper chambers of transwell systems. HUVECs cultured in the lower compartments were cocultured with the THP-1 cells for 6 h. The migrated THP-1 cells were visualized and counted. (D) THP-1 cells were challenged with P. gingivalis for 24 h or untreated, labeled with Hoechst 33342, and then were added to HUVECs in 24-well plates and incubated for 2 h. The adherent THP-1 cells were imaged and quantified. The quantifications of migrated and adherent THP-1 cells are presented as the mean ± standard deviation (SD) of three independent experiments. All data were analyzed using unpaired two-tailed Student’s t-test. *P < 0.05 vs untreated control group.
Porphyromonas gingivalis Promotes the Expression of LOX-1 in Both HUVECs and THP-1 Cells
The exact mechanisms underlying the migration and adhesion of THP-1 cells to HUVECs enhanced by P. gingivalis are less well understood. The activation of LOX-1 in cardiovascular cell lineages is highly related with the progress of atherosclerosis including monocyte migration and adhesion (Yoshimoto et al., 2011). Whether LOX-1 was a key mediator regulating P. gingivalis-triggered migration and adhesion of THP-1 cells to HUVECs was questioned in this study. Therefore, the effect of P. gingivalis on the activation of LOX-1 in HUVECs and THP-1 cells was investigated firstly.
Both HUVECs and THP-1 cells were treated with P. gingivalis (MOI = 100) for 2 h, respectively. Then the medium was replaced with fresh medium and the HUVECs and THP-1 cells continued to be cultured for 0–22 h. Afterward, the expression of LOX-1 in HUVECs and THP-1 cells was analyzed at the mRNA and protein levels. The results showed that LOX-1 expression in both HUVECs and THP-1 cells was low at baseline and increased after the infection of P. gingivalis (Figures 2A–D). The mRNA level of LOX-1 in both HUVECs (Figure 2A) and THP-1 cells (Figure 2B) rose to a peak at 8 h. Besides, the protein level of LOX-1 expressed in the HUVECs and THP-1 cells rose to a peak at 24 h (Figures 2C,D, respectively).
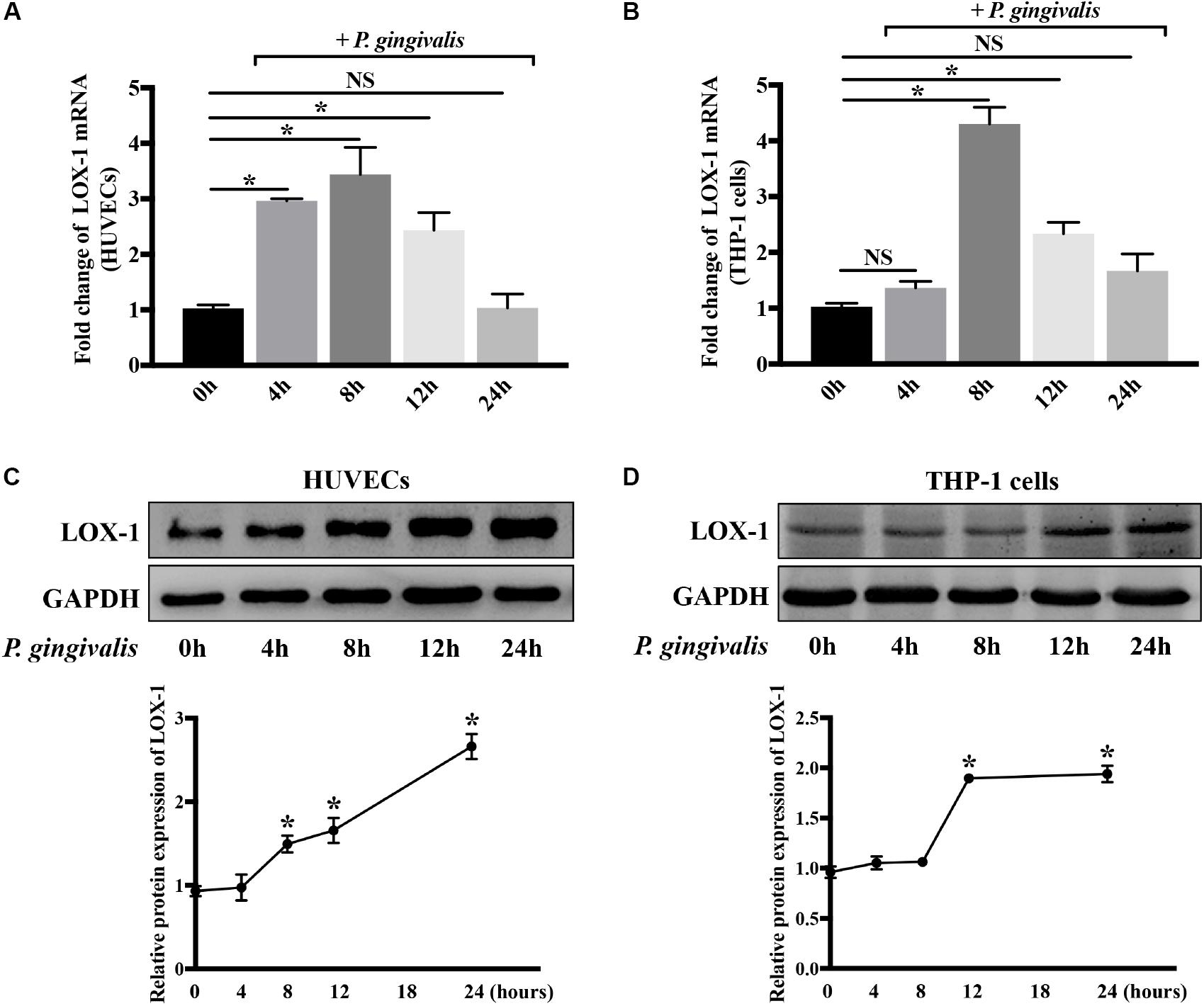
Figure 2. Porphyromonas gingivalis increases LOX-1 expression in both HUVECs and THP-1 cells. The mRNA and protein expression levels of LOX-1 in HUVECs (A,C) and THP-1 cells (B,D) were analyzed after a challenge with P. gingivalis at a MOI of 1:100 for 0–24 h. The results are presented as the mean ± SD of four independent experiments. Data were analyzed by one-way analysis of variance (one-way ANOVA) with Dunnett’s multiple comparison. *P < 0.05 vs 0 h.
LOX-1 Is Crucially Involved in P. gingivalis-Induced THP-1 Cell Migration and Adhesion to HUVECs
Subsequently, in order to make clear the role of LOX-1 in the P. gingivalis-induced THP-1 cell migration and adhesion to HUVECs, LOX-1 expression in HUVECs and THP-1 cells was knocked down by siRNA-mediated gene silencing. The siLOX-1-3 was ensured to be the most effective siRNA for causing a significant (≈76–81%) decrease in LOX-1 expression (Supplementary Figure S1) and was used in this study. Silencing of LOX-1 in HUVECs stimulated by P. gingivalis significantly reduced THP-1 cell migration and adhesion to the HUVECs (Figures 3A,B, respectively). Similarly, silencing of LOX-1 in THP-1 cells which were challenged with P. gingivalis also reduced migration (Figure 3C) and adhesion (Figure 3D) of the THP-1 cells to HUVECs.
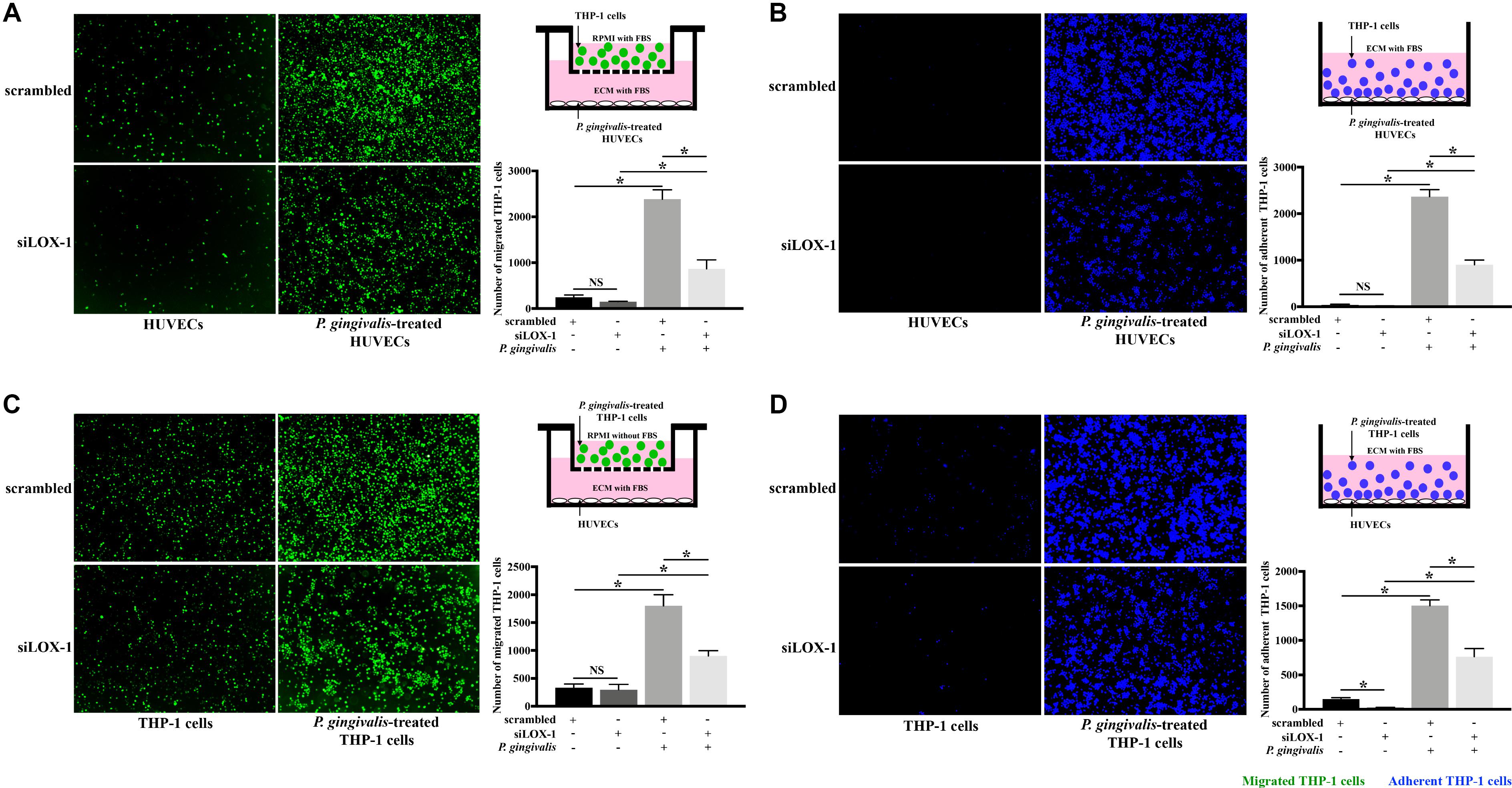
Figure 3. Knockdown of LOX-1 attenuates P. gingivalis-induced THP-1 cell migration and adhesion to HUVECs. LOX-1 downregulation by siRNA suppressed LOX-1 expression by about 76–81% in HUVECs and THP-1 cells. (A) HUVECs with or without LOX-1 knockdown in the lower compartments of transwell culture dishes remained untreated or were challenged with P. gingivalis for 24 h. Calcein AM-labeled THP-1 cells were added into the upper chambers. After 6 h incubation with the HUVECs, THP-1 cells migrating into the lower chambers were imaged and counted. (B) HUVECs with or without LOX-1 knockdown in 24-well plates were untreated or treated with P. gingivalis for 24 h. They were then cocultured with THP-1 cells (labeled with Hoechst 33342) for 2 h, respectively. The adherent THP-1 cells were measured. (C) THP-1 cells with or without LOX-1 knockdown were untreated or challenged with P. gingivalis for 24 h before being stained with calcein AM. They were then loaded into the upper chambers of transwell culture dishes separately and incubated with HUVECs cultured in the lower chambers for 6 h. The number of migrated THP-1 cells were counted. (D) THP-1 cells with or without LOX-1 knockdown were untreated or treated with P. gingivalis for 24 h, and labeled with Hoechst 33342. They were then added, respectively, to HUVECs cultured in 24-well plates and incubated for 2 h. The number of THP-1 cells adhered to the HUVECs was measured under fluorescence microscopy picture. Images are representative of three independent experiments, and the number of migrated or adherent THP-1 cells is presented as the mean ± SD. Data were analyzed by one-way ANOVA with Turkey’s multiple comparison test. *P < 0.05. vs scrambled, siLOX-1, or scrambled + P. gingivalis group.
To further verify LOX-1 was able to regulate the P. gingivalis-induced THP-1 cells migration and adhesion to HUVECs, LOX-1 was overexpressed in HUVECs and THP-1 cells by adenovirus-mediated gene transduction. The effective overexpression of LOX-1 level (increased about 1.7 times) was illustrated as shown in Supplementary Figure S2. The migration (Figures 4A,C) and adhesion (Figures 4B,D) of THP-1 cells to HUVECs were significantly increased by the overexpression of LOX-1 in either HUVECs or THP-1 cells.
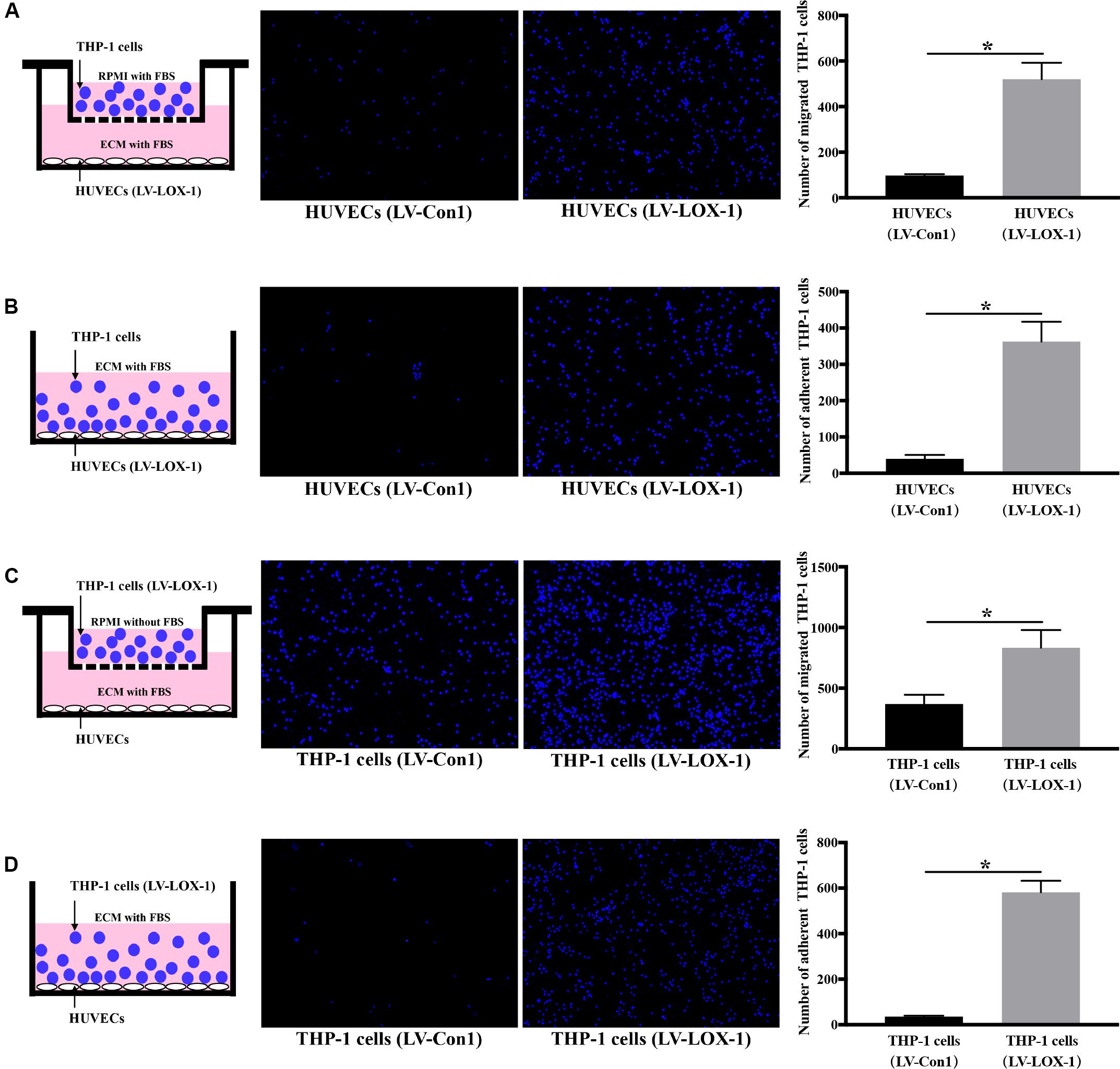
Figure 4. LOX-1 overexpression promotes migration and adhesion of THP-1 cells to HUVECs. LOX-1 in HUVECs and THP-1 cells was overexpressed by about 1.7-fold. (A) LOX-1-overexpressing and control HUVECs were cultured in the lower chambers of transwell systems, respectively. THP-1 cells stained with Hoechst 33342 were loaded into the upper chambers and incubated with the HUVECs for 6 h. Migrated THP-1 cells were visualized and counted. (B) LOX-1-overexpressing and control HUVECs grown in 24-well plates were cocultured with the Hoechst 33342-labeled THP-1 cells for 2 h, respectively. The non-adherent THP-1 cells were washed, while the adherent THP-1 cells were imaged and quantified. (C) LOX-1-overexpressing and control THP-1 cells were labeled with Hoechst 33342 and loaded into the upper inserts of transwell culture dishes separately. HUVECs cultured in the lower chambers were incubated with the THP-1 cells for 6 h. The migrated THP-1 cells were imaged and counted. (D) LOX-1-overexpressing THP-1 cells and the control THP-1 cells were labeled with Hoechst 33342, and then added separately to HUVECs cultured in 24-well plates and incubated for 2 h. Adherent THP-1 cells were counted. The number of migrated or adherent THP-1 cells is shown as the mean ± SD (n = 4). Data were obtained by unpaired two-tailed Student’s t-test. *P < 0.05. vs LV-Con1 group.
These results above show that LOX-1 participates in P. gingivalis-mediated THP-1 cell migration and adhesion to HUVECs.
LOX-1 Regulates P. gingivalis-Induced Expression of MCP-1, ICAM-1, and E-Selectin in HUVECs and That of CCR2 and Integrin αMβ2 in THP-1 Cells
The increased expression of ligand/receptor pairs MCP-1/CCR2, ICAM-1/Integrin αMβ2, and E-selectin/Integrin αMβ2 have been known to mediate monocyte migration and adhesion to endothelial cells (Jaipersad et al., 2014; França et al., 2017). Therefore, we detected the influence of LOX-1 on P. gingivalis-induced expression of MCP-1, ICAM-1, and E-selectin in HUVECs and that of CCR2 and Integrin αMβ2 in THP-1 cells.
Silencing of LOX-1 in HUVECs and THP-1 cells was achieved by lentiviral shRNA transduction. A transduction efficiency of 70–80% was obtained (Supplementary Figures S2A,B). HUVECs and THP-1 cells with or without LOX-1 knockdown (LV-shLOX-1 and LV-Con2, respectively) were challenged with P. gingivalis for 24 h or untreated before cells were harvested. The results showed that in HUVECs, the mRNA (Figures 5A–C) and protein (Figure 5G) expression levels of ligands MCP-1, ICAM-1, and E-selectin, especially ICAM-1 and E-selectin were upregulated strongly by P. gingivalis. However, LOX-1 silencing dramatically repressed the upregulation (Figures 5A–C,G). Likewise, in THP-1 cells, stimulation with P. gingivalis also resulted in an increase in the expression of receptors CCR2, Integrin αM and Integrin β2, and the increase was substantially reduced by knockdown of LOX-1 (Figures 5D–F,H).
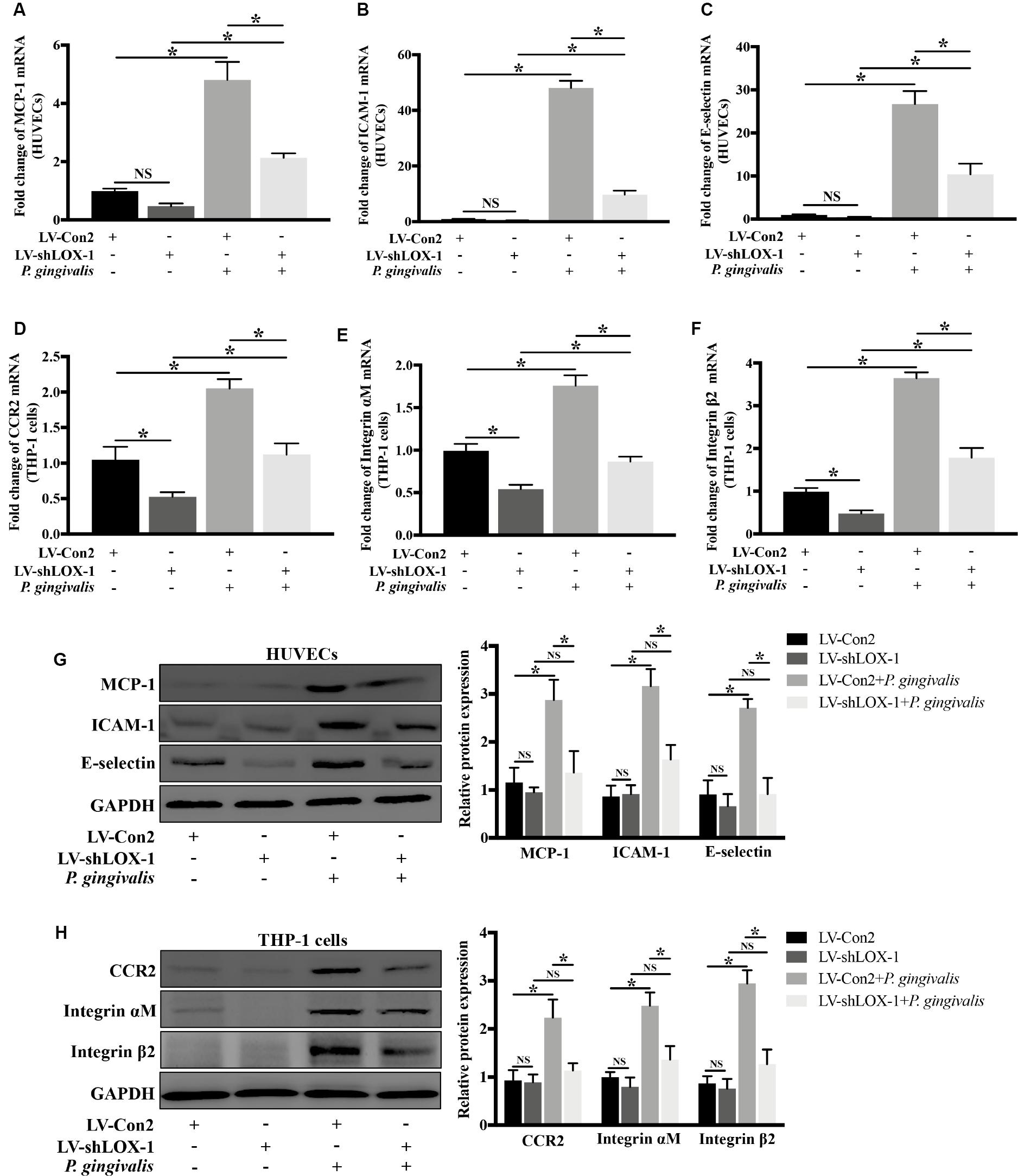
Figure 5. Knockdown of LOX-1 reduces the P. gingivalis-induced upregulation of ligands MCP-1, ICAM-1, and E-selectin in HUVECs and that of receptors CCR2 and Integrin αMβ2 in THP-1 cells. Adenovirus-mediated LOX-1 knockdown was achieved in HUVECs and THP-1 cells (knockdown efficiency was about 76%). HUVECs with or without LOX-1 knockdown (LV-shLOX-1 and LV-Con2, respectively) were challenged with P. gingivalis for 24 h or untreated. The expression of MCP-1, ICAM-1, and E-selectin was examined by Real-time PCR (A–C) and Western blotting (G). THP-1 cells with or without LOX-1 knockdown (LV-shLOX-1 and LV-Con2, respectively) were not treated or treated with P. gingivalis for 24 h. The mRNA (D–F) and protein (H) expression levels of CCR2 and Integrin αMβ2 were also analyzed by Real-time PCR and Western blotting. Results are representative of three independent experiments with similar results ± SD. Data were analyzed using one-way ANOVA with Turkey’s multiple comparison test. *P < 0.05. vs LV-Con2, LV-shLOX-1, or LV-Con2 + P. gingivalis group.
To verify the effect of LOX-1 on the expression of ligands MCP-1, ICAM-1, and E-selectin in HUVECs and that of receptors CCR2 and Integrin αMβ2 in THP-1 cells, LOX-1 was overexpressed in HUVECs and THP-1 cells (increased by about 1.7-fold). It was found that the mRNA (Figure 6A) and the protein (Figure 6C) expression levels of MCP-1, ICAM-1 and E-selectin in HUVECs were elevated by LOX-1 overexpression. Meanwhile, LOX-1 overexpression also caused a increase in the mRNA (Figure 6B) and protein (Figure 6D) presentation of CCR2 and Integrin αMβ2 in THP-1 cells.
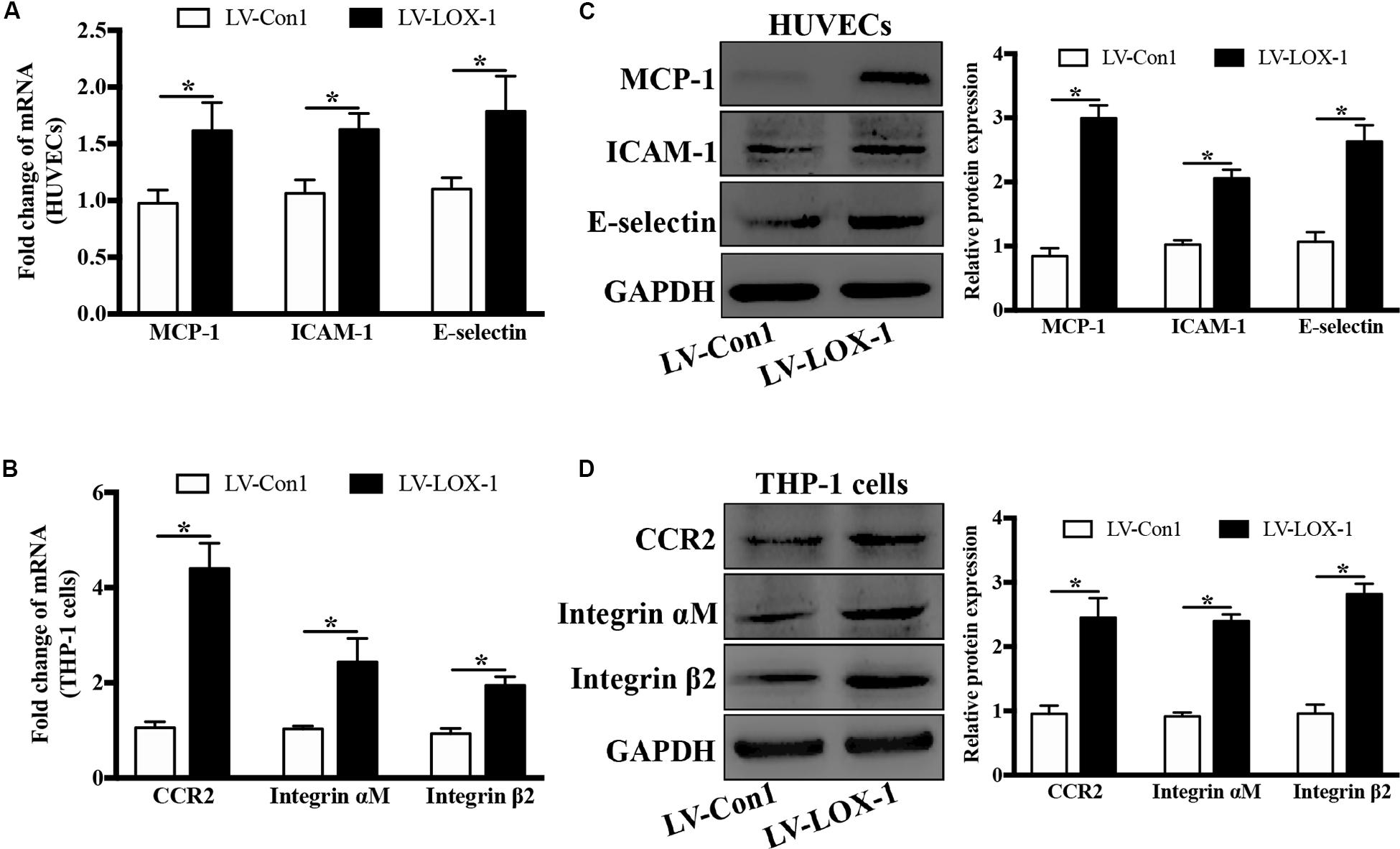
Figure 6. LOX-1 overexpression increases expression of ligands MCP-1, ICAM-1, and E-selectin in HUVECs and that of receptors CCR2 and Integrin αMβ2 in THP-1 cells. LOX-1 expression in HUVECs and THP-1 cells was enhanced by about 1.7-fold by lentiviral transduction. The mRNA (A) and protein (C) expression levels of MCP-1, ICAM-1, and E-selectin in LOX-1-overexpressing and control (LV-LOX-1 and LV-Con1, respectively) HUVECs were detected by Real-time PCR and Western blotting, respectively. The same methods were also adopted to examine the mRNA (B) and protein (D) expression levels of CCR2 and Integrin αMβ2 in LOX-1-overexpressing (LV-LOX-1) and control (LV-Con1) THP-1 cells. The results are representative of three independent experiments and are presented as the mean ± SD. Data were analyzed using unpaired two-tailed Student’s t-test. *P < 0.05. vs LV-Con1 group.
These results demonstrate that LOX-1 plays a positive role in P. gingivalis-induced expression of MCP-1, ICAM-1 and E-selectin in HUVECs and that of CCR2 and Integrin αMβ2 in THP-1 cells.
Induction of LOX-1 by P. gingivalis Is Dependent on NF-κB Activation
NF-κB as a crucial transcription factor, is involved in response to various stimuli such as bacterial infections and inflammatory factors. In order to evaluate whether NF-κB signaling pathway mediates the activation of LOX-1 in HUVECs and THP-1 cells induced by P. gingivalis, HUVECs and THP-1 cells were preincubated with PDTC (100 μM) for 1 h to inhibit the activation of NF-κB, then followed by P. gingivalis stimulation for another 24 h before cells were harvested. The results of Real-time PCR showed that the mRNA level of LOX-1 in both HUVECs and THP-1 cells increased significantly after P. gingivalis stimulation, but the increase was inhibited by PDTC pretreatment (Figures 7A,B). Western blotting revealed that P. gingivalis stimulation led to an increased expression of p–p65, which was markedly suppressed by PDTC preincubation (Figures 7C,D). Moreover, the increased protein expression of LOX-1 in both HUVECs and THP-1 cells by P. gingivalis stimulation was also inhibited substantially by PDTC (Figures 7C,D). These results demonstrate that the NF-κB signal pathway mediates the LOX-1 expression in HUVECs and THP-1 cells induced by P. gingivalis.
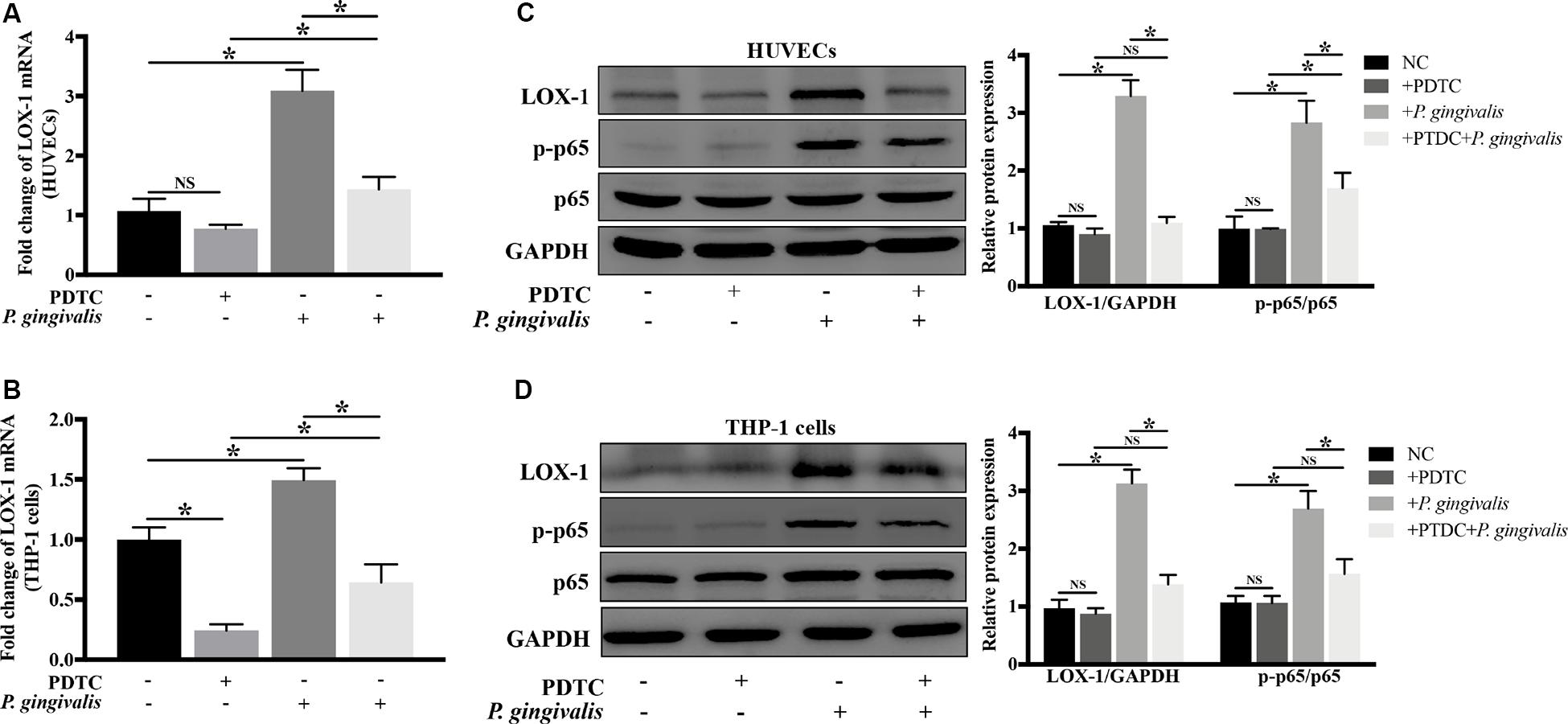
Figure 7. Blockage of NF-κB signaling abrogates P. gingivalis-induced LOX-1 production in HUVECs and THP-1 cells. HUVECs and THP-1 cells were pretreated with PDTC (100 μM, an inhibitor of NF-κB activation) or not treated before they were challenged with P. gingivalis or left unchallenged. The inhibitory effect of PDTC on NF-κB activation in HUVECs (C) and THP-1 cells (D) was evaluated by Western blotting. Meanwhile, the effect of PDTC on P. gingivalis-upregulated LOX-1 expression in HUVECs (A,C) and THP-1 cells (B,D) was examined. Data represents average ± SD (n = 4). Statistical differences were determined using one-way ANOVA followed by Turkey’s multiple comparison test. *P < 0.05. vs normal control cells (NC), + PDTC, or + P. gingivalis group.
LOX-1 Modulates the Production of MCP-1, ICAM-1, and E-Selectin in HUVECs and That of CCR2 and Integrin αMβ2 in THP-1 Cells via NF-κB Activation
Next, the regulatory role of NF-κB signaling pathway in LOX-1-dependent induction of MCP-1, ICAM-1 and E-selectin in HUVECs and that of CCR2 and Integrin αMβ2 in THP-1 cells was investigated. As shown in Figure 8A, there was a higher level of p–p65 expression in LOX-1-overexpressing HUVECs and LOX-1-overexpressing THP-1 cells than that in their control groups, which indicated the activation of NF-κB signaling pathway by LOX-1. Therefore, PDTC (100 μM, 24 h) was used to inhibit the NF-κB activation, and the p–p65 expression in both LOX-1-overexpressing HUVECs and LOX-1-overexpressing THP-1 cells was considerably decreased by PDTC treatment (Figure 8B). In addition, the mRNA expression of MCP-1, ICAM-1 and E-selectin in LOX-1-overexpressing HUVECs was significantly attenuated by PDTC (Figure 8C). This attenuation was verified at the protein level (Figure 8E). The mRNA (Figure 8D) and protein (Figure 8F) expression of CCR2 and Integrin αMβ2 in LOX-1-overexpressing THP-1 cells was decreased as well by treatment with PDTC. These results reveal that NF-κB signaling is involved in LOX-1-induced expression of MCP-1, ICAM-1 and E-selectin in HUVECs and that of CCR2 and Integrin αMβ2 in THP-1 cells.
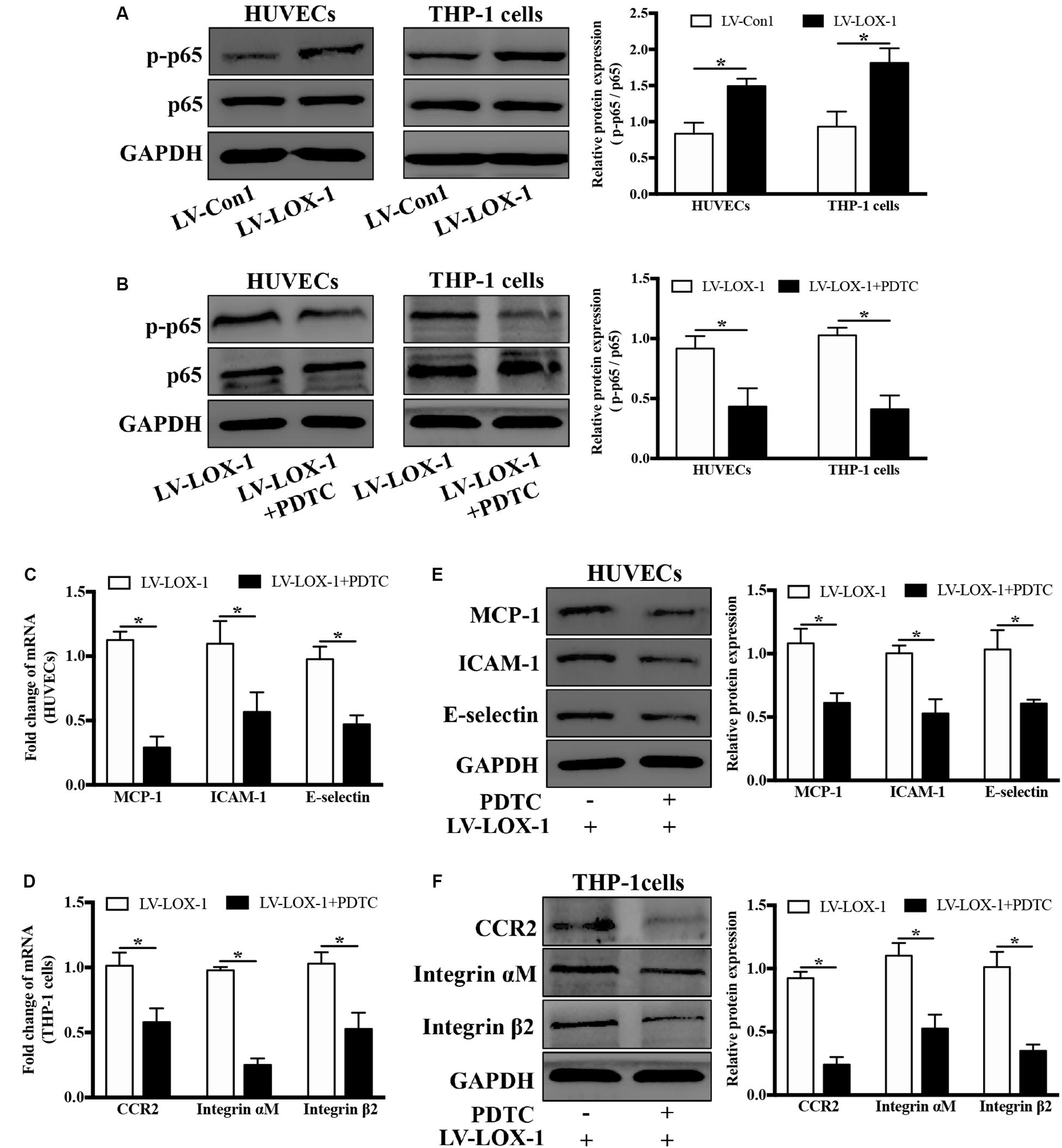
Figure 8. Blockage of NF-κB signaling attenuates LOX-1-upregulated expression of MCP-1, ICAM-1, and E-selectin in HUVECs and that of CCR2 and Integrin αMβ2 in THP-1 cells. (A) The expression levels of p–p65 and p65 in LOX-1-overexpressing HUVECs, LOX-1-overexpressing THP-1 cells, and their corresponding control groups were detected by Western blotting. (B) PDTC (100 μM) was applied to block the activation of NF-κB in LOX-1-overexpressing HUVECs and LOX-1-overexpressing THP-1 cells. After inhibition of NF-κB signaling, the expression change of MCP-1, ICAM-1, and E-selectin in LOX-1-overexpressing HUVECs (C,E) and that of CCR2 and Integrin αMβ2 in LOX-1-overexpressing THP-1 cells (D,F) were evaluated. Data are mean ± SD (n = 4), which were analyzed by unpaired two-tailed Student’s t-test. *P < 0.05. vs LV-Con1 or LV-LOX-1 group.
Discussion
Monocyte migration and adhesion to endothelial cells are crucial steps in the early stages of atherosclerosis development, in which ligand-receptor pairs such as MCP-1/CCR2, ICAM-1/Integrin αMβ2, and E-selectin/Integrin αMβ2 are crucially involved. The significant finding of this study is that LOX-1 is crucially involved in P. gingivalis-induced monocyte migration and adhesion to endothelial cells, by regulating the expression of not only the chemokine MCP-1, and adhesion molecules ICAM-1, and E-selectin in HUVECs but also their corresponding receptors CCR2 and Integrin αMβ2 in THP-1 cells.
It was demonstrated for the first time that LOX-1 in both HUVECs (Figures 2A,C) and THP-1 cells (Figures 2B,D) could be activated by the periodontal pathogen P. gingivalis. Previous studies have reported that LOX-1 recognized some pathogens such as S. aureus, E. coli (Shimaoka et al., 2001), C. pneumoniae (Campbell et al., 2013) and Aspergillus (He et al., 2016). However, no studies have focused on the response of LOX-1 to P. gingivalis. P. gingivalis is a keystone periodontal pathogen. A close relationship between P. gingivalis and cardiovascular disease has been suggested. This pathogen could translocate from periodontal pockets to the cardiovascular system (Spahr et al., 2006). P. gingivalis was even found in human atheroma (Fiehn et al., 2005; Mahendra et al., 2015) and cells from atherosclerotic lesions (Njoroge et al., 1997; Olsen and Progulske-Fox, 2015). LOX-1 is widely known to play a vital role in atherosclerosis (Singh and Gautam, 2019). This receptor has been proved to contribute to the formation of atherosclerotic lesions in the initiation of atherosclerosis. Furthermore, the persistent accumulation of the LOX-1 is also involved in atherosclerotic plaque stability and myocardial infarction, which implies the long-term effects of LOX-1 in atherosclerosis (Chen et al., 2002). In the present study, periodontal pathogen P. gingivalis was found to increase the expression level of LOX-1 in both HUVECs (Figures 2A,C) and THP-1 cells (Figures 2B,D). The upregulation of LOX-1 expression by P. gingivalis supports the important role of LOX-1 in P. gingivalis-mediated pathological processes in atherosclerosis and reinforces the speculation that LOX-1 is a potential molecule linking periodontitis with atherosclerosis.
Previous studies have suggested that LOX-1 was absent in monocytes but induced in differentiated macrophages (Yoshida et al., 1998; Noriaki et al., 2006). However, our results were not in line with those results. In our study, the expression of LOX-1 in monocytic THP-1 cells was low, but it was increased significantly by P. gingivalis stimulation (Figures 2B,D). More recently, Tanimoto (Tanimoto et al., 2001) and Yamagata (Yamagata et al., 2014) revealed that LOX-1 expression in unstimulated monocytes was low, which was consistent with our results. These two research teams also detected upregulated LOX-1 expression in monocytes which were stimulated with histamine and TNF-α, respectively (Tanimoto et al., 2001; Yamagata et al., 2014). Their results were similar to our results that LOX-1 in THP-1 cells was inducible by some external stimuli. In addition, the production of LOX-1 has been demonstrated to be remarkably high in monocytes from patients with acute coronary syndrome, compared with those from healthy controls (Wang et al., 2008). Hence, it is concluded that LOX-1 is actually expressed at a low level in normal monocytes but it is highly expressed in activated monocytes.
Surprisingly, P. gingivalis triggered a similar kinetics of LOX-1 expression in HUVECs and THP-1 cells, in our study. Although LOX-1 in THP-1 cells seemed to exhibit a more limited response to P. gingivalis than that in HUVECs, the mRNA and protein levels of LOX-1 expression in both cell lines peaked at 8 h and 24 h, respectively, under the same stimulation of P. gingivalis (Figures 2A–D). To our knowledge, for the first time the kinetics of LOX-1 expression in HUVECs and THP-1 cells is compared. Endothelial cells and monocytes are thought to coordinately regulate some specific immune processes such as monocyte migration and adhesion (Chanput et al., 2014), in which LOX-1 plays a vital role. The similar kinetics of LOX-1 expression in HUVECs and THP-1 cells is possibly the premise and foundation for synchronous cooperation between these two cell lines, finally guiding THP-1 cell migration and adhesion to HUVECs. Moreover, it was of interest to note that LOX-1 induction by P. gingivalis in both HUVECs and THP-1 cells was regulated by NF-κB pathway (Figures 7A–D). NF-κB activation is considered to be a central initiating cellular event in response to a wide range of pathogens (Rahman and McFadden, 2011). As the full spectrum of NF-κB-dependent genes are being gradually systematically explored, the sequential expression pattern of NF-κB-downstream genes is revealed (Tian et al., 2005; O’Dea and Hoffmann, 2010). LOX-1 expression represented in a form of quantitative accumulation and rose to a peak at 8 h or even later after P. gingivalis stimulation (Figures 2A–D). Thus LOX-1 can be classed into the late response group of NF-κB-downstream genes (Tian et al., 2005). Given that the kinetics of NF-κB activation is a central regulator of its effect on downstream genes (Sen and Smale, 2009; O’Dea and Hoffmann, 2010), it is suggested that HUVECs and THP-1 cells share a similar activation pattern of NF-κB for the similar kinetics of LOX-1 expression. This possibility requires further research. In conclusion, the similar kinetics of LOX-1 expression via NF-κB activation in HUVECs and THP-1 cells may be the possible foundation for their synchronous cooperation, which determines the migration and adhesion of THP-1 cells to HUVECs. This postulation inspires us to explore the effect of LOX-1 on monocyte-endothelial cell communication in a new dimension.
Moreover, it is worth noting that LOX-1 was involved in the regulation of not only ligands MCP-1, ICAM-1 and E-selectin expression in HUVECs but also corresponding receptors CCR2 and Integrin αMβ2 expression in THP-1 cells (Figures 6A–D). The binding of chemokines and adhesion molecules in HUVECs to their counterreceptors in monocytes are importantly implicated in monocyte migration and adhesion to HUVECs (Miller and Blystone, 2015). Monocyte chemoattractant protein-1 (MCP-1) is one of the most important regulators, whose properties of monocyte activation and recruitment are primarily mediated through the activation of CCR2 (França et al., 2017). Monocyte adhesion to endothelial cells fosters atherosclerotic lesion initiation. In this process, the interaction of E-selectin/Integrin αMβ2 mediates monocyte rolling on endothelial cells and monocyte adhesion to endothelial cells, and the interaction of ICAM-1/Integrin αMβ2 mainly determines the firm adhesion of monocytes (Jaipersad et al., 2014). The activation of various migration-related and adhesion-related molecules in our study emphasizes the importance of LOX-1 in monocyte migration and adhesion to endothelial cells. Besides, the dual regulatory role of LOX-1 in activating ligands in HUVECs and corresponding receptors in THP-1 cells, compared with the activation of ligands or receptors alone, would enhance the affinity between these two cell lines, further facilitating monocyte migration and adhesion to endothelial cells. More interestingly, P. gingivalis as an effective activator for LOX-1, was also found to upregulate the expression of the chemokine MCP-1, adhesion molecules ICAM-1 and E-selectin in HUVECs and that of their receptors CCR2 and Integrin αMβ2 in THP-1 cells, an effect was substantially reduced following LOX-1 knockdown (Figures 5A–D). Thus, these evidences prominently emphasize the importance of LOX-1 in P. gingivalis-induced monocyte migration and adhesion of monocytes to endothelial cells, by modulating migration-related and adhesion-related molecules.
NF-κB is generally known to be associated with inflammatory diseases, such as periodontitis, atherosclerosis and type 2 diabetes (Baker et al., 2011; Hajishengallis and Sahingur, 2014). More importantly, NF-κB has a central role in inflammatory network involving complicated cross talks and feedbacks (Tieri et al., 2012; Hu et al., 2017). Our results revealed that induction of LOX-1 by P. gingivalis was dependent on NF-κB activation (Figures 7A–D). The promoter region of the LOX-1 gene was reported to contain a binding site for NF-κB (Kelly et al., 2008). This important discovery supports our result that NF-κB signaling pathway plays an important role in LOX-1 activation. The ligand-receptor pairs MCP-1/CCR2, ICAM-1/Integrin αMβ2, and E-selectin/Integrin αMβ2 are important molecules for monocyte migration and adhesion to endothelial cells (Jaipersad et al., 2014; França et al., 2017). We found that NF-κB signaling pathway also mediate LOX-1-dependent expression of MCP-1, ICAM-1 and E-selectin in HUVECs, and that of their receptors CCR2 and Integrin αMβ2 in THP-1 cells (Figures 8A–F). This result indicated that NF-κB is an important signaling pathway for LOX-1-downstream genes. Based on these results, it is so interesting to note that NF-κB-dependent LOX-1 regulates downstream molecules through NF-κB activation as well. We suggest that the central role of NF-κB/LOX-1 positive feedback loop is the main reason. Specifically, P. gingivalis-activated NF-κB signaling result in the induction of LOX-1, which subsequently induces NF-κB activation, thereby creating a positive feedback loop. The loop can further serve as an “inflammation amplifier”, whose role is to further enhance the presentation of downstream migration-related and adhesion-related molecules. In this interaction mode, NF-κB acts as a key node regulating the expression of LOX-1 and LOX-1-dependent downstream molecules. Similar interaction patterns are found in other researches, in which molecules achieving self-regulation through NF-κB activation can regulate other NF-κB-dependent responses at the same time (Hosokawa et al., 2013; Ogura et al., 2013; Zhu et al., 2019). Collectively, our results highlight the central role of NF-κB/LOX-1 positive feedback loop in the expression of ligands MCP-1, ICAM-1, and E-selectin in HUVECs, and that of receptors CCR2 and Integrin αMβ2 in THP-1 cells.
Altogether, the results of this study demonstrate an essential involvement of LOX-1 in P. gingivalis-triggered monocyte migration and adhesion to endothelial cells by regulating the presentation of the chemokine MCP-1, adhesion molecules ICAM-1 and E-selectin in HUVECs and that of their receptors CCR2 and Integrin αMβ2 in THP-1 cells. These evidences in vitro deepen our understanding of the molecular mechanism involved in P. gingivalis-induced monocyte migration and adhesion to endothelial cells. In addition, a potential role for LOX-1 in the link between periodontitis and atherosclerosis is preliminarily verified.
Data Availability Statement
All datasets generated for this study are included in the article/Supplementary Material.
Author Contributions
QL designed and performed the research, collected and analyzed the data, and wrote the manuscript. JL and WL performed the research, collected the data, and edited the manuscript. YC, JZ, YX, and XL performed the research, collected the data, and provided analysis tools. XO designed the research, supported financial support for the research, and edited the manuscript. All authors read and approved the manuscript.
Funding
This study was supported by the National Natural Science Foundation of China (Nos. 81870772 and 81570986).
Conflict of Interest
The authors declare that the research was conducted in the absence of any commercial or financial relationships that could be construed as a potential conflict of interest.
Acknowledgments
We gratefully acknowledge Prof. Chenxiong Lai for providing the P. gingivalis strain W83.
Supplementary Material
The Supplementary Material for this article can be found online at: https://www.frontiersin.org/articles/10.3389/fcell.2020.00596/full#supplementary-material
FIGURE S1 | Efficiency of LOX-1 knockdown in HUVECs and THP-1 cells. LOX-1 knockdown in HUVECs (A) and THP-1 cells (B) was achieved with a small interfering RNA (siLOX-1). Their transfection efficiency was determined by Western blotting. GAPDH was used for normalization. The results are presented as the mean ± SD of three independent experiments. The statistical significance was measured by one-way ANOVA and Dunnett’s multiple comparison tests. ∗P < 0.05. vs scrambled group.
FIGURE S2 | Generation of LOX-1-overexpressing and LOX-1-knockdown cell lines. Stable overexpression or knockdown of LOX-1 (LV-LOX-1 and LV-shLOX-1, respectively) in HUVECs (A) and THP-1 cells (B) was achieved by lentiviral infection. Their efficiencies were determined by Western blotting. Data shown are the mean ± SD (n = 3). Statistical differences were determined by unpaired two-tailed Student’s t-test. ∗P < 0.05. vs LV-Con1 or LV-Con2 group.
Abbreviations
CCR2, C–C chemokine receptor 2; HUVECs, human umbilical vein endothelial cells; ICAM-1, intercellular adhesion molecule-1; LOX-1, lectin-like oxidized low-density lipoprotein receptor-1; LV-Con1, control of LOX-1 overexpression; LV-LOX-1, LOX-1 overexpression; LV-shLOX-1, control of LOX-1 knockdown; LV-shLOX-1, LOX-1 knockdown; MCP-1, monocyte chemoattractant protein-1; MOI, multiplicity of infection; NF- κ B, nuclear factor-kappa B; ox-LDL, oxidized low-density lipoprotein; P. gingivalis, Porphyromonas gingivalis; PDTC, ammonium pyrrolidinedithiocarbamate; PRR, pattern recognition receptor; PVDF, polyvinylidenedifluoride; siRNA, small interfering RNA; SMC, smooth muscle cells; TGF- β, transfer growth factor β; TNF- α, Tumor necrosis factor α.
References
Amar, S., and Engelke, M. (2015). Periodontal innate immune mechanisms relevant to atherosclerosis. Mol. Oral. Microbiol. 30, 171–185. doi: 10.1111/omi.12087
Baker, R. G., Hayden, M. S., and Ghosh, S. (2011). NF-κB, inflammation, and metabolic disease. Cell. Metabol. 13, 11–22. doi: 10.1016/j.cmet.2010.12.008
Bianconi, V., Sahebkar, A., Atkin, S. L., and Pirro, M. (2018). The regulation and importance of monocyte chemoattractant protein-1. Curr. Opin. Hematol. 25, 44–51. doi: 10.1097/MOH.0000000000000389
Campbell, L. A., Lee, A. W., Rosenfeld, M. E., and Kuo, C. C. (2013). Chlamydia pneumoniae induces expression of pro-atherogenic factors through activation of the lectin-like oxidized LDL receptor-1. Pathog. Dis. 69, 1–6. doi: 10.1111/2049-632X.12058
Cardoso, E. M., Reis, C., and Manzanares-Céspedes, M. C. (2018). Chronic periodontitis, inflammatory cytokines, and interrelationship with other chronic diseases. Postgrad. Med. 130, 98–104. doi: 10.1080/00325481.2018.1396876
Chanput, W., Mes, J. J., and Wichers, H. J. (2014). THP-1 cell line: an in vitro cell model for immune modulation approach. Int. Immunopharmacol. 23, 37–45. doi: 10.1016/j.intimp.2014.08.002
Chen, M., Masaki, T., and Sawamura, T. (2002). LOX-1, the receptor for oxidized low-density lipoprotein identified from endothelial cells: implications in endothelial dysfunction and atherosclerosis. Pharmacol. Ther. 95, 89–100. doi: 10.1016/s0163-7258(02)00236-x
Fiehn, N. E., Larsen, T., Christiansen, N., Holmstrup, P., and Schroeder, T. V. (2005). Identification of periodontal pathogens in atherosclerotic vessels. J. Periodontol. 76, 731–736. doi: 10.1902/jop.2005.76.5.731
França, C. N., Izar, M. C. O., Hortêncio, M. N. S., Amaral, J. B. D., Ferreira, C. E. S., Tuleta, I. D., et al. (2017). Monocyte subtypes and the CCR2 chemokine receptor in cardiovascular disease. Clin. Sci. 131, 1215–1224. doi: 10.1042/CS20170009
Hajishengallis, G., and Sahingur, S. E. (2014). Novel inflammatory pathways in periodontitis. Adv. Dent. Res. 26, 23–29. doi: 10.1177/0022034514526240
Hashizume, T., Kurita-Ochiai, T., and Yamamoto, M. (2011). Porphyromonas gingivalis stimulates monocyte adhesion to human umbilical vein endothelial cells. FEMS Immunol. Med. Microbiol. 62, 57–65. doi: 10.1111/j.1574-695X.2011.00786.x
He, K., Yue, L. H., Zhao, G. Q., Li, C., Lin, J., Jiang, N., et al. (2016). The role of LOX-1 on innate immunity against Aspergillus keratitis in mice. Int. J. Ophthalmol. 9, 1245–1250. doi: 10.18240/ijo.2016.09.01
Hosokawa, S., Haraguchi, G., Sasaki, A., Arai, H., Muto, S., Itai, A., et al. (2013). Pathophysiological roles of nuclear factor kappaB (NF-κB) in pulmonary arterial hypertension: effects of synthetic selective NF-κB inhibitor IMD 0354. Cardiovas. Res. 99, 35–43. doi: 10.1093/cvr/cvt105
Hu, G., Xiao, F., Li, Y., Li, Y., and Vongsangnak, W. (2017). Protein-protein interface and disease: perspective from biomolecular networks. Adv. Biochem. Eng. Biot. 160, 57–74. doi: 10.1007/10_2016_40
Jaipersad, A. S., Lip, G. Y. H., Silverman, S., and Shantsila, E. (2014). The role of monocytes in angiogenesis and atherosclerosis. J. Am. Coll. Cardiol. 63, 1–11. doi: 10.1016/j.jacc.2013.09.019
Kaski, J. (2011). Inflammation, infection and acute coronary plaque events. Eur. Heart. J. Suppl. 3, I10–I15. doi: 10.1016/s1520-765x(01)90101-4
Kelly, K. J., Wu, P., Patterson, C. E., Temm, C., and Dominguez, J. H. (2008). LOX-1 and inflammation: a new mechanism for renal injury in obesity and diabetes. AM. J. Physiol. Renal. 294, F1136–F1145. doi: 10.1152/ajprenal.00396.2007
Liu, W., Liu, J., Wang, W., Wang, Y., and Ouyang, X. (2018). NLRP6 induces pyroptosis by activation of caspase-1 in gingival fibroblasts. J. Dent. Res. 97, 1391–1398. doi: 10.1177/0022034518775036
Lu, J., Mitra, S., Wang, X., Khaidakov, M., and Mehta, J. L. (2011). Oxidative stress and lectin-like Ox-LDL-receptor LOX-1 in atherogenesis and tumorigenesis. Antioxid. Redox. Sign. 15, 2301–2333. doi: 10.1089/ars.2010.3792
Mahendra, J., Mahendra, L., Nagarajan, A., and Mathew, K. (2015). Prevalence of eight putative periodontal pathogens in atherosclerotic plaque of coronary artery disease patients and comparing them with noncardiac subjects: a case-control study. Indian J. Dent. Res. 26, 189–195. doi: 10.4103/0970-9290.159164
Mayer, F. J., and Binder, C. J. (2019). “Atherosclerosis,” in Fundamentals of Vascular Biology, ed. M. Geiger (Cham, CH: Springer), 195–233.
Mestas, J., and Ley, K. (2008). Monocyte-endothelial cell interactions in the development of atherosclerosis. Trends. Cardiovasc. Med. 18, 228–232. doi: 10.1016/j.tcm.2008.11.004
Miller, M. R., and Blystone, S. D. (2015). Human macrophages utilize the podosome formin FMNL1 for adhesion and migration. Cellbiology 4, 1–11. doi: 10.4236/cellbio.2015.41001
Navarra, T., Del Turco, S., Berti, S., and Basta, G. (2010). The lectin-like oxidized low-density lipoprotein receptor-1 and its soluble form: cardiovascular implications. J. Atheroscler. Thromb. 17, 317–331. doi: 10.5551/jat.3228
Njoroge, T. G. R. J., Genco, R. J., Sojar, H. T., Hamada, N., and Genco, C. A. (1997). A role for fimbriae in Porphyromonas gingivalis invasion of oral epithelial cells. Infect. Immun. 65, 1980–1984. doi: 10.1007/BF02113615
Noriaki, K., Hisataka, M., Hiroharu, K., and Manabu, M. (2006). Inducible expression of LOX-1, a novel receptor for oxidized LDL, in macrophages and vascular smooth muscle cells. Ann. N.Y. Acad. Sci. 902, 323–327. doi: 10.1161/01.RES.83.3.322
Nvk, P., Karathanasis, S. K., Ding, Z., Arulandu, A., Varughese, K. I., and Mehta, J. L. (2017). LOX-1 in atherosclerosis and myocardial ischemia: biology, genetics, and modulation. J. AM. Coll. Cardiol. 69, 2759–2768. doi: 10.1016/j.jacc.2017.04.010
O’Dea, E., and Hoffmann, A. (2010). The regulatory logic of the NF-kappaB signaling system. Cold Spring Harbor. Perspect. Biol. 2:a000216. doi: 10.1101/cshperspect.a000216
Ogura, H., Arima, Y., Kamimura, D., and Murakami, M. (2013). The gateway theory: how regional neural activation creates a gateway for immune cells via an inflammation amplifier. Biomed. J. 36, 269–273. doi: 10.4103/2319-4170.113187
Olsen, I., and Progulske-Fox, A. (2015). Invasion of Porphyromonas gingivalis strains into vascular cells and tissue. J. Oral Microbiol. 7:28788. doi: 10.3402/jom.v7.28788
Rahman, M. M., and McFadden, G. (2011). Modulation of NF-κB signalling by microbial pathogens. Nat. Rev. Microbiol. 9, 291–306. doi: 10.1038/nrmicro2539
Sen, R., and Smale, S. T. (2009). Selectivity of the NF-κB response. Cold Spring Harb. Perspect. Biol. 2:a000257. doi: 10.1101/cshperspect.a000257
Shimaoka, T., Kume, N., Minami, M., Hayashida, K., Sawamura, T., Kita, T., et al. (2001). LOX-1 supports adhesion of gram-positive and gram-negative bacteria. J. Immunol. 166, 5108–5114. doi: 10.4049/jimmunol.166.8.5108
Singh, S., and Gautam, A. S. (2019). Upregulated LOX-1 receptor: key player of the pathogenesis of atherosclerosis. Curr. Atheroscler. Rep. 21:38. doi: 10.1007/s11883-019-0801-y
Spahr, A., Klein, E., Khuseyinova, N., Boeckh, C., Muche, R., Kunze, M., et al. (2006). Periodontal infections and coronary heart disease: role of periodontal bacteria and importance of total pathogen burden in the coronary event and periodontal disease (CORODONT) study. Arch. Intern. Med. 166, 554–559. doi: 10.1001/archinte.166.5.554
Tanimoto, A., Murata, Y., Nomaguchi, M., Kimura, S., and Sasaguri, Y. (2001). Histamine increases the expression of LOX-1 via 2 receptor in human monocytic THP-1 cells. FEBS Lett. 508, 345–349. doi: 10.1016/S0014-5793(01)03073-3
Theodorou, K., and Boon, R. A. (2018). Endothelial cell metabolism in atherosclerosis. Front. Cell. Dev. Biol. 6:82. doi: 10.3389/fcell.2018.00082
Tian, B., Nowak, D. E., and Brasier, A. R. (2005). A TNF-induced gene expression program under oscillatory NF-κB control. BMC Genom. 6:137. doi: 10.1186/1471-2164-6-137
Tieri, P., Termanini, A., Bellavista, E., Salvioli, S., Capri, M., and Franceschi, C. (2012). Charting the NF-κB pathway interactome map. PLoS One 7:e32678. doi: 10.1371/journal.pone.0032678
Wan, M., Liu, J. R., Wu, D., Chi, X. P., and Ouyang, X. Y. (2015). E-selectin expression induced by Porphyromonas gingivalis in human endothelial cells via nucleotide-binding oligomerization domain-like receptors and Toll-like receptors. Mol. Oral Microbiol. 30, 399–410. doi: 10.1111/omi.12102
Wang, J. G., Liang, Z. S., Kan, Y., Huang, Z. J., and Li, J. L. (2008). Effect of IL-10 on LOX-1 expression of peripheral blood monocytes in patients with acute coronary syndrome. J. Cent. S. U. 33, 169–173.
Yamagata, K., Tusruta, C., Ohtuski, A., and Tagami, M. (2014). Docosahexaenoic acid decreases TNF-α-induced lectin-like oxidized low-density lipoprotein receptor-1 expression in THP-1 cells. Prostag. Leukotr. Ess. 90, 125–132. doi: 10.1016/j.plefa.2013.12.011
Yoshida, H., Kondratenko, N., Green, S., Steinberg, D., and Quehenberger, O. (1998). Identification of the lectin-like receptor for oxidized low-density lipoprotein in human macrophages and its potential role as a scavenger receptor. Biochem. J. 334, 9–13. doi: 10.1042/bj3340009
Yoshimoto, R., Fujita, Y., Kakino, A., Iwamoto, S., Takaya, T., and Sawamura, T. (2011). The discovery of LOX-1, its ligands and clinical significance. Cardiovasc. Drugs. Ther. 25, 379–391. doi: 10.1007/s1055701163246
Zhou, J., Zhang, J., and Chao, J. (2011). Porphyromonas gingivalis promotes monocyte migration by activating MMP-9. J. Periodontal. Res. 47, 236–242. doi: 10.1111/j.1600-0765.2011.01427.x
Zhu, H. (2005). Ox-LDL plays dual effect in modulating expression of inflammatory molecles through LOX-1 pathway in human umbilical vein endothelial cells. Front. Biosci. 10, 2585–2594. doi: 10.2741/1722
Keywords: Porphyromonas gingivalis, LOX-1, THP-1 cells, HUVECs, migration, adhesion
Citation: Li Q, Liu J, Liu W, Chu Y, Zhong J, Xie Y, Lou X and Ouyang X (2020) LOX-1 Regulates P. gingivalis-Induced Monocyte Migration and Adhesion to Human Umbilical Vein Endothelial Cells. Front. Cell Dev. Biol. 8:596. doi: 10.3389/fcell.2020.00596
Received: 06 February 2020; Accepted: 18 June 2020;
Published: 14 July 2020.
Edited by:
Claudia Tanja Mierke, Leipzig University, GermanyReviewed by:
Nagaraj Balasubramanian, Indian Institute of Science Education and Research, IndiaOleh Andrukhov, University Dental Clinic Vienna, Austria
Jin Chung, Pusan National University, South Korea
Yaping Pan, China Medical University, China
Copyright © 2020 Li, Liu, Liu, Chu, Zhong, Xie, Lou and Ouyang. This is an open-access article distributed under the terms of the Creative Commons Attribution License (CC BY). The use, distribution or reproduction in other forums is permitted, provided the original author(s) and the copyright owner(s) are credited and that the original publication in this journal is cited, in accordance with accepted academic practice. No use, distribution or reproduction is permitted which does not comply with these terms.
*Correspondence: Xiangying Ouyang, kqouyangxy@bjmu.edu.cn