Transcriptome profiling of histone writers/erasers enzymes across spermatogenesis, mature sperm and pre-cleavage embryo: Implications in paternal epigenome transitions and inheritance mechanisms
- 1Centro de Estudios Biomédicos Básicos, Aplicados y Desarrollo (CEBBAD), Universidad Maimónides, Ciudad Autónoma de Buenos Aires, Buenos Aires, Argentina
- 2Instituto de Investigaciones Farmacológicas (Universidad de Buenos Aires–Consejo Nacional de Investigaciones Científicas y Técnicas), Ciudad Autónoma de Buenos Aires, Buenos Aires, Argentina
Accumulating evidence points out that sperm carry epigenetic instructions to embryo in the form of retained histones marks and RNA cargo that can transmit metabolic and behavioral traits to offspring. However, the mechanisms behind epigenetic inheritance of paternal environment are still poorly understood. Here, we curated male germ cells RNA-seq data and analyzed the expression profile of all known histone lysine writers and erasers enzymes across spermatogenesis, unraveling the developmental windows at which they are upregulated, and the specific activity related to canonical and non-canonical histone marks deposition and removal. We also characterized the epigenetic enzymes signature in the mature sperm RNA cargo, showing most of them positive translation at pre-cleavage zygote, suggesting that paternally-derived enzymes mRNA cooperate with maternal factors to embryo chromatin assembly. Our study shows several histone modifying enzymes not described yet in spermatogenesis and even more, important mechanistic aspects behind transgenerational epigenetics. Epigenetic enzymes not only can respond to environmental stressors, but could function as vectors of epigenetic information and participate in chromatin organization during maternal-to-zygote transition.
Introduction
In the last years, accumulating research has documented that male germ cell maturation presents windows of vulnerability for epigenetic reprogramming by environmental stressors that can affect fertility and even transmit developmental, metabolic and behavioral traits to offspring (Bale, 2015; Lee and Conine, 2022). This type of non-genetic “Lamarckian” transmission has been established for paternal lifestyle and different forms of chronic stress, drug abuse, dietary change, and social defeat, involving changes in non-coding RNAs cargo, DNA methylation, and histone post-translational modifications (PTMs) (Bale, 2015; Lee and Conine, 2022). However, most of the research in epigenetic inheritance has documented the role of non-coding RNAs and DNA methylation patterns, whereas the mechanisms by which the environment can trigger specific changes on histone PTMs are still largely unexplored.
Spermatogenesis is a finely regulated process where unipotent spermatogonia undergo meiosis and, subsequently, spermiogenesis, to become sperm cells. During the developmental stages of spermatogenesis, male germ cells experience dramatic chromatin reorganization, where approximately 90% (human) to 95% (mouse) of histones are evicted and replaced by protamines to compact the paternal genome (Rajender et al., 2011; Carrell, 2012). Importantly, a small fraction of histones in the sperm genome is retained in specific locations and carries several PTMs that play critical roles in epigenetic regulation of spermatogenesis and early embryonic development (Hammoud et al., 2009; Brykczynska et al., 2010; Yamaguchi et al., 2018; Chioccarelli et al., 2020; Lambrot et al., 2021; Okada, 2022). The epigenetic program of histone PTMs that controls spermatogenesis is regulated by enzymatic families known as writers and erasers of the so-called “histone code,” that modulate chromatin structure and transcription (Kouzarides, 2007). These epigenetic enzymes catalyze the deposition or removal of specific PTMs such as acetylation, methylation, phosphorylation, crotonylation, among others, at specific amino acid residues on the nucleosome’s core histones H2A, H2B, H3, and H4, and also on the linker H1/H5 (Chioccarelli et al., 2020). In male germ cells, histone PTMs not only control active vs. repressed chromatin states but also a range of processes including DNA replication and repair, chromosome maintenance and histone eviction (Luense et al., 2016). So far, the most studied histone PTMs related to the control of germ cells mitosis, meiosis and spermiogenesis are methylated and acetylated lysines (K) on H2A/B, H3 and H4. Histone K acetylation can be dynamically regulated by the opposing action of acetyltransferases (HATs) and deacetylases (HDACs). Acetylation of K residues neutralizes the positive charge on histones, allowing DNA-binding proteins better access to the DNA and resulting in activation of gene expression as well as histone eviction (Miller and Grant, 2013). Unlike acetylation, methylation does not affect histone charge but regulates recognition and interaction with chromatin-binding proteins that control the transcription or respond to DNA damage (Miller and Grant, 2013). Histone K methylation is finely regulated by methyltransferases (KMTs) and demethylases (KDMs) that control the mono-, di-, and/or tri-methylation of specific residues, and this can either activate or repress transcription depending on the residue position, the number of methylations and the presence of other methyl or acetyl groups in the vicinity (Mosammaparast and Shi, 2010; Miller and Grant, 2013).
The role of epigenetic enzymes comes to focus, as they are responsible for the histones’ PTMs writing and erasing balance. Moreover, histone-modifying enzymes have been shown to respond to the organism physiology and metabolism, several disease conditions, and environmental stressors (Bale, 2015; Nebbioso et al., 2018; Gonzalez et al., 2020). Even though epigenetic enzymes are the bridge linking the environment with epigenetic inheritance through retained histones PTMs, an in-depth analysis of their expression patterns throughout the spermatogenic process is still lacking. Moreover, these enzymes provide new targets for therapies for numerous diseases (Prachayasittikul et al., 2017) but the potential effect of these compounds on epigenetic inheritance is generally overlooked. To further elucidate the mechanisms driving the histone PTMs during spermatogenesis, a better understanding of the gene expression profiles of epigenetic modifying enzymes in male germ cells is required. Here, we curated transcriptomic data from spermatogonia to mature sperm populations and analyzed the expression profile and dynamic changes of all the known families of histone K acetylation and methylation writers and erasers. We provide a complete picture of the epigenetic enzymes across spermatogenesis in mice and the developmental windows when the transcription of these enzymes may be more susceptible to environmental disruption. Moreover, we analyzed pre-cleavage zygote translatome, confirming that mRNAs of several histone modifying enzymes carried by sperm are associated with ribosomes for protein synthesis in 1-cell embryo. Not only do we expand the knowledge on epigenetic enzymes with recognized roles in spermatogenesis, but provide evidence of many more whose roles in male germ cell development and zygote have not been described yet.
Methods
Data retrieval
FASTQ files were downloaded from NCBI GEO and ArrayExpress databases. Transcriptomic data of male germ cells was obtained from GSE162740, consisting of germ cells isolated from “Stra8-Tom” mice obtained from the cross of Stra8-cre mice with CAG-LSLtdTomato mice (Mayorek et al., 2022). The gradient expression of the Tomato transgene under Stra8 expression profile, combined with immunostaining for cKit to further discriminate the SGund and SGdiff spermatogonia, was used to FACS sort six populations of germ cells in triplicates, and sequence in an Illumina HiSeq 2,500 platform. Sperm cells data were obtained from: i) GSE81216, that sampled two total sperm and two sperm heads from C57BL/6J (JAX) mice using Ion Torrent PGM platform, ii) GSE88732, that sampled four mature sperm from adult C57BL/6J mice using Illumina HiSeq 4000 platform, and iii) E-MTAB-5834, that sampled four control mature sperm from adult C57BL/6J mice using Illumina HiSeq 2,500 platform. Data from 1 cell embryo, two total and two ribosome-bound RNA RPKMs, were obtained from GSE169632.
Data processing
FASTQ files from germ cells and sperm were processed with the following pipeline: quality control with FASTQC, trimming with fastp, mapping with STAR and GRCm39 (MM10 gencode.vM29.annotation), and counting with featureCounts. Processed data was manipulated using Rstudio (v1.2.1033) and Tidyverse packages Dplyr and ggplot2 (Wickham et al., 2019). The male germ cells count matrix was analyzed with DESeq2 package (Love et al., 2014), using the likelihood ratio test (LRT) for longitudinal data instead of the Wald test, and a formula that accounted for batch and cell group effects. Comparisons between consecutive cells groups were obtained by contrasts. Counts were converted to RPKM using mean gene length extracted from the gencode.vM29 file. Hierarchical clustering was performed with pheatmap package. Additionally, we performed a cross studies comparison with two other datasets that performed RNA-seq (GSE49622, Hammoud et al., 2014) and single-cell RNA-seq (GSE112393, Green et al., 2018) in adult mouse germ cells, and were able to cross validate 77% of the expression profile pressented here (see “Data cross validation” in Supplementary Material). The sperm RPKMs obtained for the three selected datasets were converted to percentile rank, and the sperm mean percentile rank calculated. Word cloud plot was performed with ggwordcloud package. The embryo translation efficiency (TE) was calculated as Ribo-seq RPKM/total RPKM for each gene, with RPKM >.5. All the plots shown in this study can be reproduced by downloading the counts tables, enzymes metadata table and R scripts available at https://github.com/Gonzalez-Lab/Gonzalez-2022-germ-cells.
Results
Epigenetic enzymes and histone K acetylation and methylation marks distribution during spermatogenic stages
Table 1 shows a curated list of writers and erasers families for histone K acetylation and methylation obtained from the Uniprot database, as proteins with confirmed catalytic activity towards histones K residues, and their most representative K targets reported in mammalian cells. Open chromatin is characterized by the presence of acetylated histones at several K residues such as H2AK5/9/13/15ac, H2BK5/12/15/20/23/24ac, H3K4/9/14/18/27/36/56/79/122ac, and H4K5/8/12/16/20/79/91ac. Many of these sites are also targets of mutually exclusive methylation. Figure 1A shows the canonical histone methylation sites that are found on H3K4/9/27/36/79 and H4K20, and characteristic of active or repressed chromatin. For instance, H3K4me1/2/3, H3K36me3, H3K9me1, H3K27me1, H3K79me2/3, and H4K20me1 are found in enhancers, promoters, and gene bodies of active genes, whereas H3K9me2/3, H3K27me2/3, and H4K20me3 are found in heterochromatin, telomeric regions and inactivated X chromosome (Figure 1A) (Black et al., 2012). In addition, there are multiple non-canonical histone methylation sites on H2AZK7, H3K23/56/63 and H4K5/12 with unknown functions, except for H3K56me3 that is involved in heterochromatin formation (Black et al., 2012). In Figure 1B we summarized the available information on the most important histone K acetylation and methylation marks distribution during spermatogenic stages, obtained from reported immunohistochemical and/or proteomic studies. We observed two main profiles on the marks distribution across spermatogenesis: H3/4ac, H3K4me2/3 and H3K9me2/3 increase with spermatogonia differentiation and early meiosis, decrease at late meiosis, and regain high levels at spermiogenesis, whereas H3K36me2/3, H3K27me2/3, H3K79me3 and H4K20me3 show sustained increased expression towards differentiation, but H4K20me is erased at RStid stage.
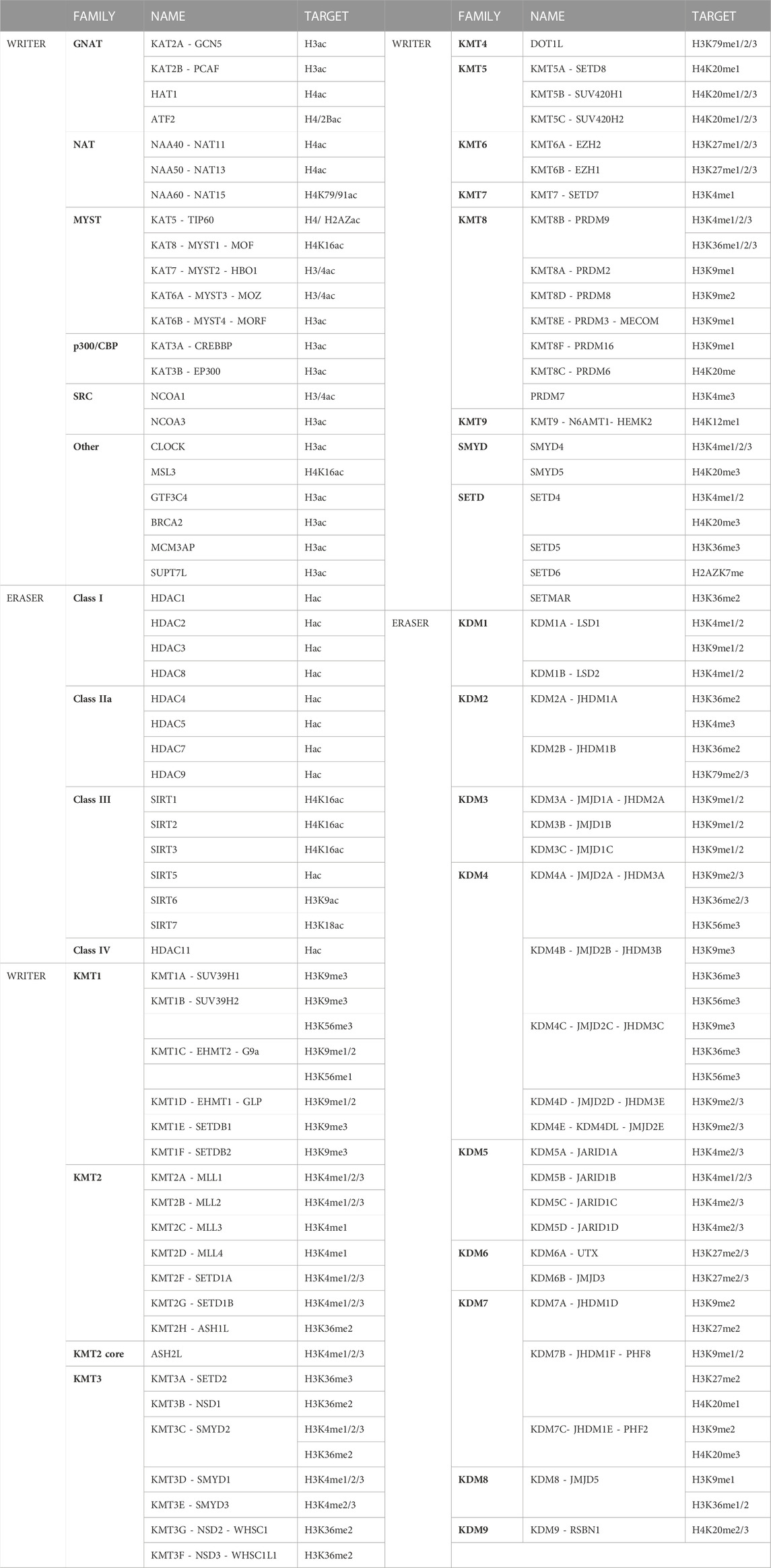
TABLE 1. Writers and erasers families for histone K acetylation and methylation and the targets described. The HATs can be divided into six major families: 1) the GNATs (GCN5-related N-acetyltransferases), 2) the NATs (N-terminal acetylases, which also possess a GNAT domain), 3) the MYST, 4) the p300/CBP, 5) the SRC steroid receptors coactivators, and 6) other HATs (Wapenaar and Dekker, 2016). HATs actions are counteracted by the HDACs, which include zinc-dependent class I, class IIa, and class IV HDACs, and NAD-dependent class III Sirtuins (Wapenaar and Dekker, 2016). On the other hand, the KMTs present two domains with annotated lysine methyltransferase activity: 1) SET domains, that catalyze all the canonical methylation sites except for H3K79, and 2) 7βS domains (DOT1L and N6AMT1) (Mosammaparast and Shi, 2010). The KDMs can be divided according to their catalytic activity in: 1) amine oxidases (KDM1), and 2) Jumonji C demethylases (KDM2/3/4/5/6/7/8) (Mosammaparast and Shi, 2010).
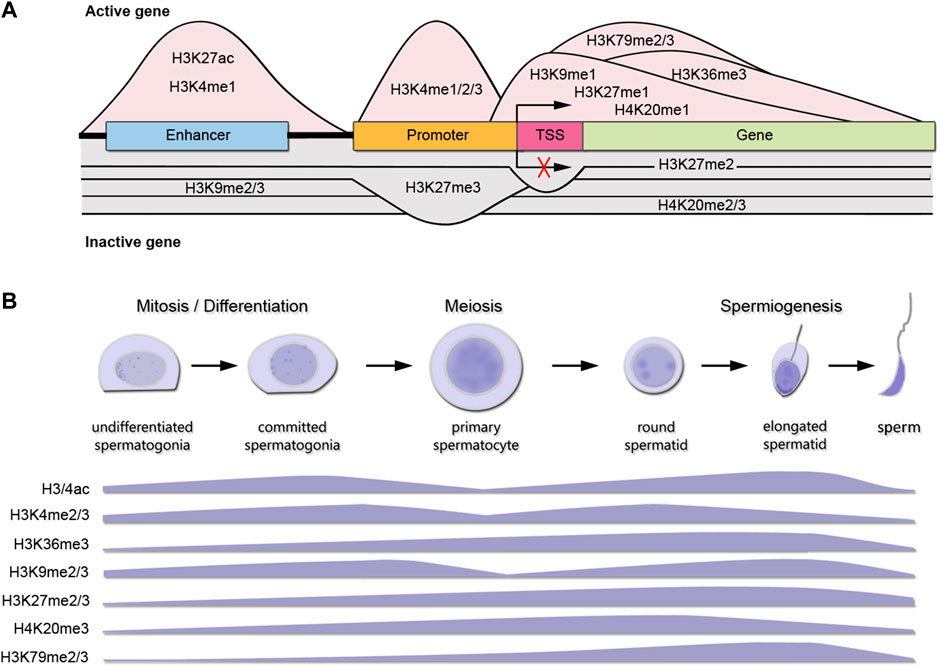
FIGURE 1. Canonical histone K marks distribution during spermatogenesis. (A) Histone K methylation PTMs distribution at DNA elements and functional roles in gene expression for an active gene (pink shading) or inactive gene (gray shading). TSS: transcription start site. Only K modifications with sufficient and consensual information about distribution across genes are shown. (B) Levels of main histone K PTMs involved in chromatin remodeling during spermatogenesis. The data presented is summarized from the following references: Song et al., 2011 (immunohistochemistry H3ac, H3K4me3, H3K27me3); Godmann et al., 2007 (immunohistochemistry and western blot H3K4me1/2/3); Zuo et al., 2018 (immunofluorescence H3K36me3); Luense et al., 2016 (Tandem mass spectrometry H3/4ac; H3K36me3, H3K9me2, H3K27me2/3, H4K20me3, H3K79me2); Wang et al., 2021 (immunofluorescence H3K20me3). SGund: spermatogonia Kit-, SGdiff: spermatogonia Kit+, PreL: pre-leptotene spermatocytes, LZ: leptotene/zygotene spermatocytes, PD: pachytene/diplotene spermatocytes, RStid: round spermatid.
Histone K acetylation and methylation enzymes expression during spermatogenesis
To analyze the expression profiles of writers and erasers families for histone K acetylation and methylation across spermatogenesis, we curated RNA-seq data available in purified male germ cells from adult mice and selected the dataset GSE162740 (Mayorek et al., 2022). This dataset contains the mRNA profiles of six populations of germ cells isolated in triplicates: undifferentiated Kit-negative (SGund) and differentiated Kit-positive spermatogonia (SGdiff) (spermatogonial phase), primary spermatocytes, including pre-leptotene (PreL), leptotene/zygotene (LZ) and pachytene/diplotene (PD) stages (meiosis), and round spermatids (RStid) (spermiogenesis phase). We downloaded the FASTQ files and mapped them to MM10 genome using STAR and featureCounts to obtain the count matrix. We corroborated the cell stages and purity of the dataset by analyzing spermatogenic and somatic cell marker genes profiles in each sample (Supplementary Figure S1; Supplementary Table S1). Figure 2 shows the hierarchical clustering of z-scores for the 102 epigenetic enzymes listed in Table 1. Column clustering shows two main profiles between the spermatogonial—early meiotic vs. late meiotic—spermiogenic phases. SGdiff and PreL stages are the most similar, whereas SGund and LZ show large clusters of enzymes upregulation. The late meiotic and spermiogenesis genes show two main clusters: genes specifically upregulated at PD and genes that increase at PD and remain high or even increase expression at RStid stage.
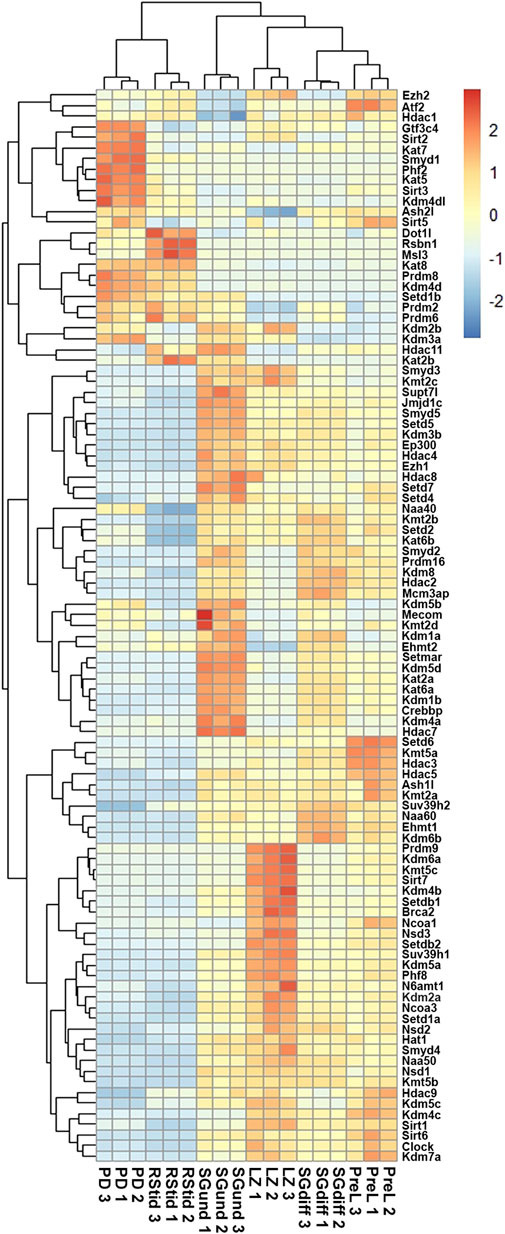
FIGURE 2. Unsupervised hierarchical clustering analysis of male germ cells transcriptome. Heatmap showing z-score values of histone K acetylation and methylation writer/eraser enzymes. SGund: spermatogonia Kit-, SGdiff: spermatogonia Kit+, PreL: pre-leptotene spermatocytes, LZ: leptotene/zygotene spermatocytes, PD: pachytene/diplotene spermatocytes, RStid: round spermatid.
Epigenetic enzymes mRNA regulation across spermatogenesis
To analyze upregulated mRNAs of epigenetic enzymes across male germ cells differentiation, we performed differential gene expression (DEG) analysis using the DESeq2 algorithm, and performed contrasts on consecutive stages of cell development: SGdiff vs. SGund, PreL vs. SGdiff, LZ vs. PreL, PD vs. LZ and RStid vs. PD (Figure 3A). For each developmental stage, we constructed heatmaps for the upregulated enzymes (padj<.05, log2FC > .5) during the spermatogonial (Figure 4), meiosis prophase I (Figure 5) and spermiogenesis (Figure 6) phases. Additionally, we also extracted the downregulated epigenetic enzymes mRNAs across male germ cells differentiation from the DEG analysis (padj<.05, log2FC < −.5, see Supplementary Figure S2). Figure 3B shows the enzymes without specific upregulation at any cell stage. These epigenetic enzymes showed a pattern of high expression at the spermatogonial—early meiotic phase that decreased with cell differentiation, forming three clusters: i) downregulated at LZ (Smyd2, Kdm8, Hdac2, Mcm3ap), ii) downregulated at PD (Kmt2a/b, Naa60, Kmt5b, Ash1l), and iii) downregulated at RStid (Setd2, Kat6b) (see Figure 3B; Supplementary Figure S2). To visualize the functional profile of the upregulated enzymes at each stage, we constructed buble charts indicating the target histone mark, the writer/eraser nature and mRNA abundance (RPKM).
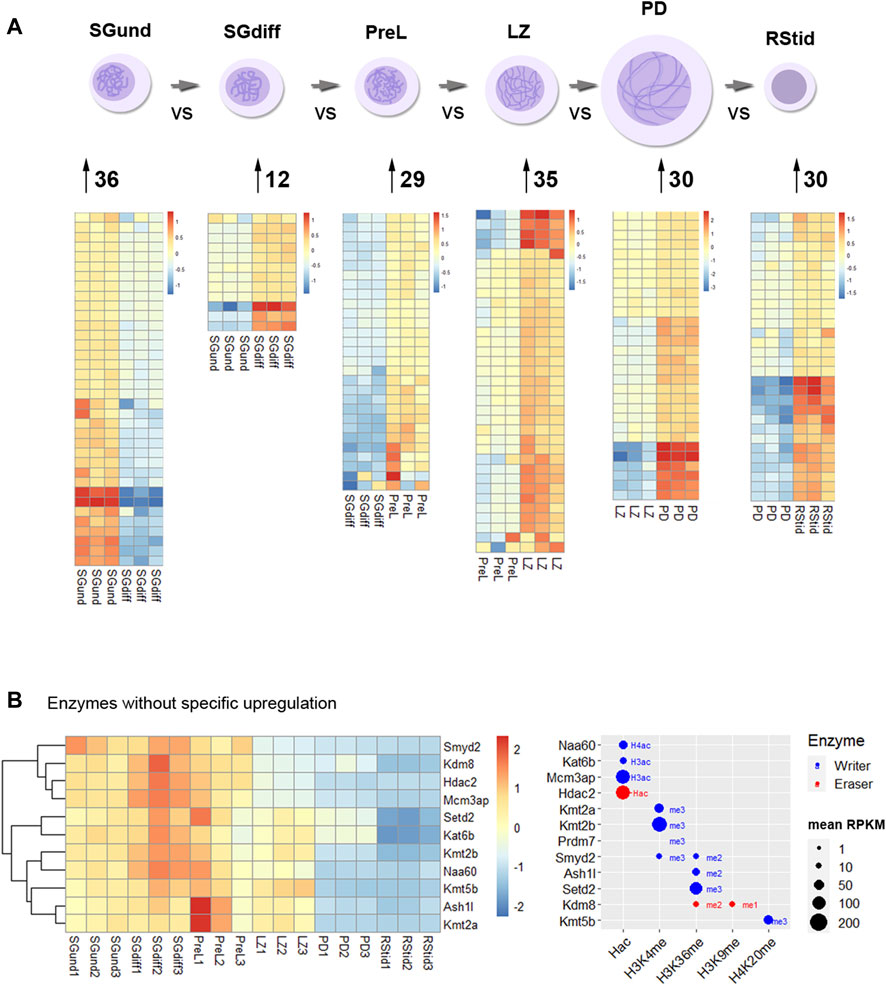
FIGURE 3. Histone K acetylation and methylation epigenetic enzymes expression during spermatogenesis. (A) Number of upregulated epigenetic enzymes and heatmap showing z-score values for each germ cell stage. Differential gene expression analysis was conducted with DESeq2 with LRT test and contrasts on consecutive populations across differentiation (SGdiff vs. SGund, PreL vs. SGdiff, LZ vs. PreL, PD vs. LZ and RStid vs. PD). Upregulated genes were selected with padj<.05 and log2FC > .5. (B) Left: Heatmap showing epigenetic enzymes without specific upregulation at any germ cell stage across spermatogenesis. Right: Bubble chart showing enzymes mean RPKM values from SGund to PD populations. Blue dots: writers, red dots: erasers. SGund: spermatogonia Kit-, SGdiff: spermatogonia Kit+, PreL: pre-leptotene spermatocytes, LZ: leptotene/zygotene spermatocytes, PD:pachytene/diplotene spermatocytes, RStid: round spermatid. The H2/3/4ac reference indicates the histone target reported for each lysine acetylation writer. Hac indicates that lysine acetylation eraser was reported to erase acetylation on all histones lysines.
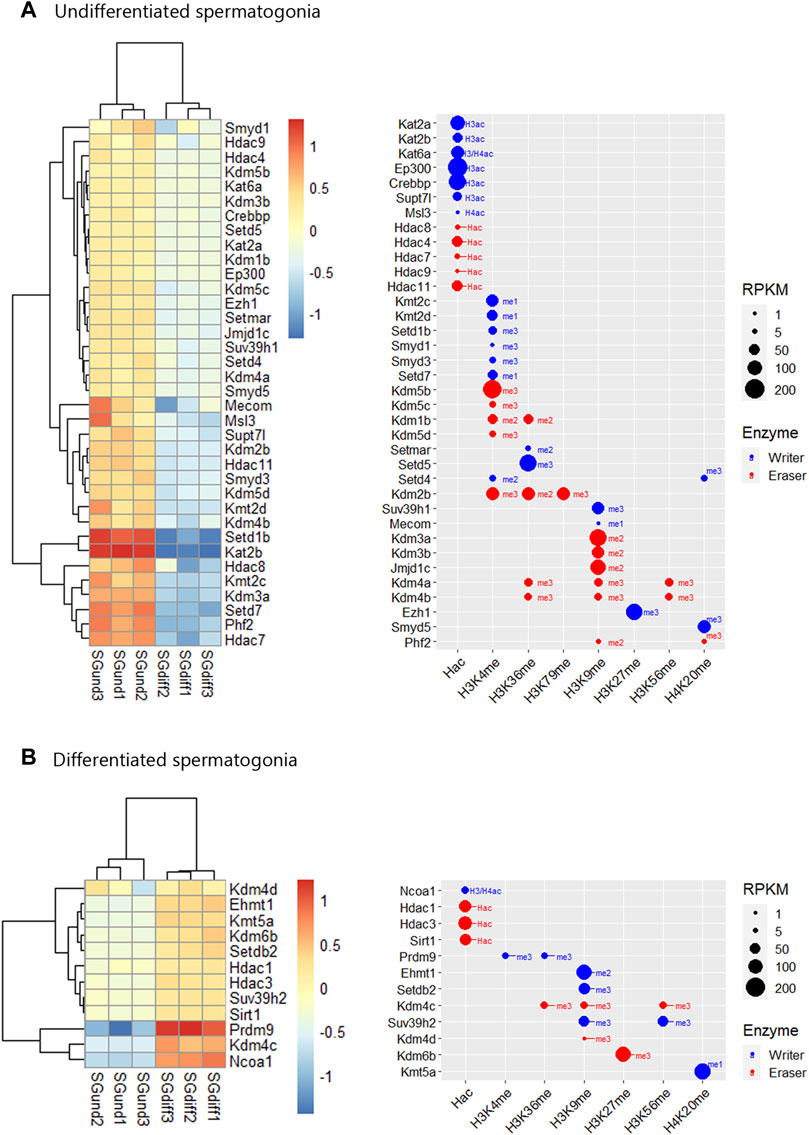
FIGURE 4. Epigenetic histone K writers and erasers upregulation at spermatogonial phase. The left panel indicates the heatmap showing z-score values and the right panel indicates the bubble chart showing enzymes RPKM values in SGund (A) and SGdiff (B) population. Blue dots: writers, red dots: erasers. SGund: spermatogonia Kit-, SGdiff: spermatogonia Kit+. The me1/2/3 reference for each dot indicates the highest methyl position reported for each lysine methylation enzyme. The H2/3/4ac reference indicates the histone target reported for each lysine acetylation writer. Hac indicates that lysine acetylation eraser was reported to erase acetylation on all histones lysines.
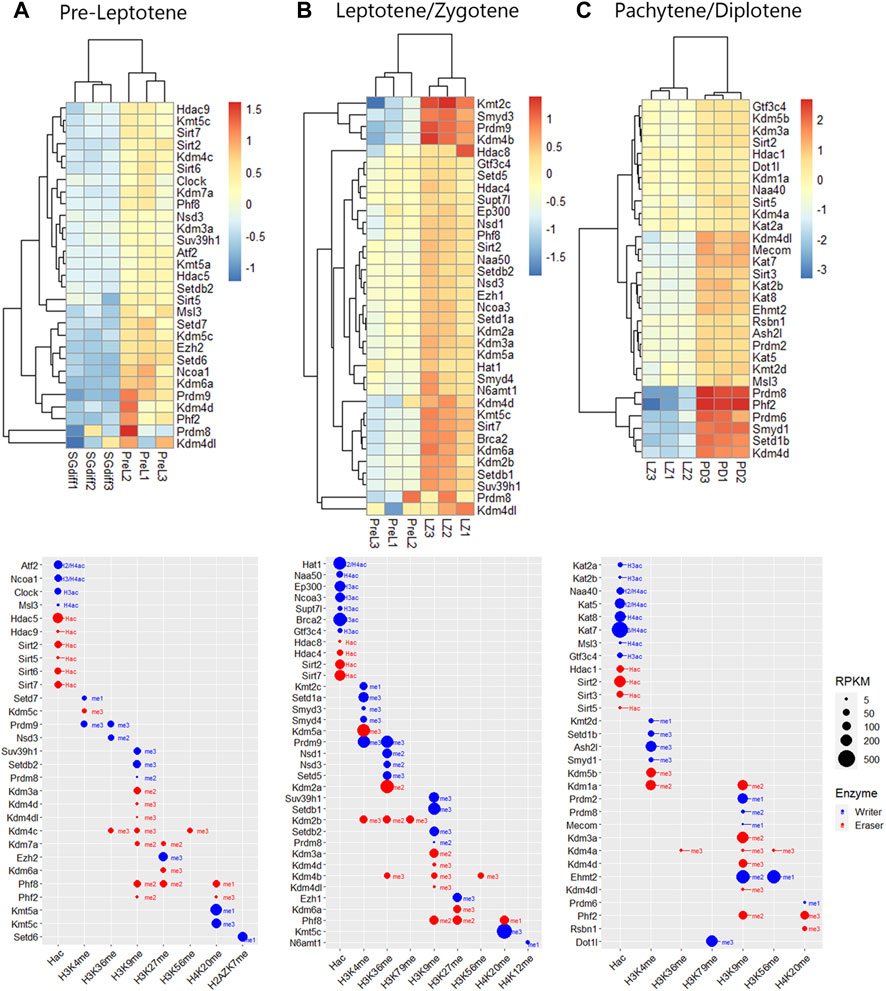
FIGURE 5. Epigenetic histone K writers and erasers upregulation at meiosis prophase I. The upper panels indicate the heatmap showing z-score values and the lower panels indicate the bubble chart showing enzymes RPKM values, in PreL (A), LZ (B) and PD (C) spermatocytes. Blue dots: writers, red dots: erasers. PreL: pre-leptotene spermatocytes, LZ: leptotene/zygotene spermatocytes, PD: pachytene/diplotene spermatocytes. The me1/2/3 reference for each dot indicates the highest methyl position reported for each lysine methylation enzyme. The H2/3/4ac reference indicates the histone target reported for each lysine acetylation writer. Hac indicates that lysine acetylation eraser was reported to erase acetylation on all histones lysines.
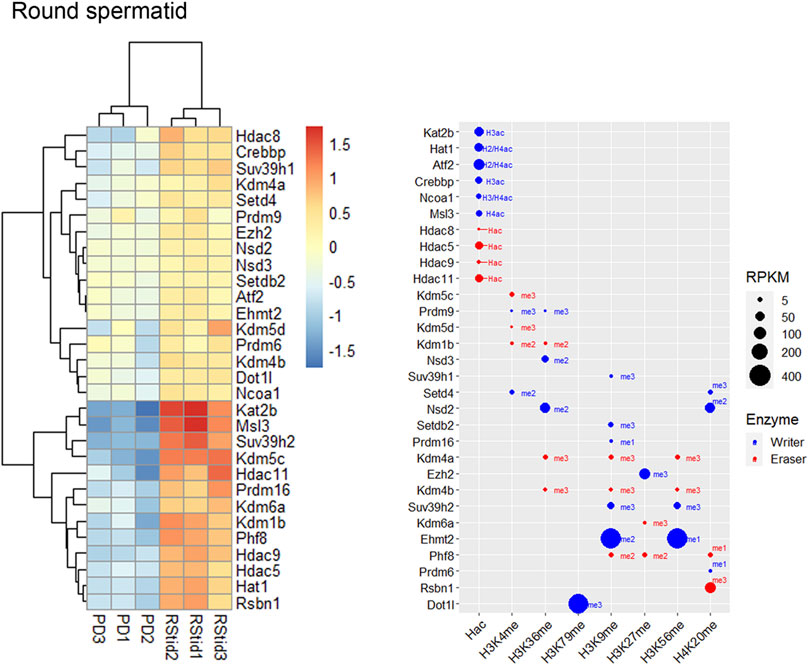
FIGURE 6. Epigenetic histone K writers and erasers upregulation at spermiogenesis. The left panel indicates the heatmap showing z-score values and the right panel indicates the bubble chart showing enzymes RPKM values in RStid. Blue dots: writers, red dots: erasers. PD: pachytene/diplotene spermatocytes, RStid: round spermatid. The me1/2/3 reference for each dot indicates the highest methyl position reported for each lysine methylation enzyme. The H2/3/4ac reference indicates the histone target reported for each lysine acetylation writer. Hac indicates that lysine acetylation eraser was reported to erase acetylation on all histones lysines.
Expression profile of histone K acetylation and methylation enzymes during spermatogenesis phases
During the spermatogonial phase, the SGund population is characterized by a mitotic activity to maintain testicular homeostasis through self-renewal. Some of these SGund initiate extensive chromatin reorganization and their epigenetic landscape shifts dramatically to differentiate to committed SGdiff (Shirakawa et al., 2013). SGund showed increased KDM3/4 H3K9me erasers (Figure 4A), and SGdiff increased KMT1 H3K9/K56me and KMT5 H4K20me writers expression (Figure 4B). At this phase, H3K27me regulation showed high expression of the polycomb H3K27me writer subunit Ezh1 at the SGund (Figure 4A), and the transition to SGdiff upregulated the H3K27me eraser Kdm6b (Figure 4B). We found enzymes with high expression at both SGund and SGdiff, including important methylation writers such as Kmt2a/b (Mll1/2), Setd2, Kmt5b (Suv420h1), Ehmt2 and Kdm1a (see Figures 2, 3B). The SGund also showed high levels of several writers and erasers for H3K4me and H3K36me, including COMPASS subunits Kmt2c/d (Mll3/4) and Setd1b, the H3K36me3 writer Setd5, and erasers Kdm1b and polycomb PRC1 subunit Kdm2b (Figure 4A). Several HATs showed increased levels at SGund, including Kat2a/b, Ep300, Msl3 and Supt7l (Figure 4A). The SGund also showed high expression of class I Hdac8, class II Hdac4/7/9 and class IV Hdac11 (Figure 4A). The transition to SGdiff specifically upregulated H3K4me and H3K36me Prdm9 and Nsd2 writers, together with increased Ncoa1, class I Hdac1/3 and class III Sirt1 (Figure 4B). Other HATs like Kat6a/b, Naa60 and Mcm3ap were elevated at both spermatogonial populations (see Figure 3B).
Meiotic entry occurs concomitantly with the pre-meiotic S phase and is followed by the meiotic prophase that initiates with PreL stage. Here, we found some enzymes that continue to increase expression from SGdiff to LZ stage, including Prdm9, Setdb2 and Kmt5a/c, and enzymes that seem to be specific to each meiotic stage, like Setd6 at PreL stage that deposits H2AZK7me1 mark and N6amt1 at LZ stage, that deposits H4K12me mark (Figure 5). Histone acetylation writers increased from PreL to PD stage, with upregulated expression of almost all members of GNATs, NATs, SRC, MYST, and p300/CBP families, and other HATs like Supt7l, Msl3, Clock, Gtf3c4 and Brca2, showing most of them high RPKM values (Figure 5). During meiotic prophase I, several class I, II and III HDACs were increased, including Hdac1/8, Hdac4/5/9 and Sirt2/3/5/6/7. Additionally, note that the major enzymatic regulation of active H3K4me2 and H3K36me marks occurs during chromosomal synapsis at LZ stage, allowing the crossing over of homologous chromosomes at hotspots during PD stage (Figure 5). Moreover, H3K36me writers Nsd1/2/3, Setd5 and Prdm9 peak expression from PreL to LZ stage, while PD stage did not show peak expression of neither H3K36me writers nor erasers (Figure 5).
Also, prophase I stages showed increased expression of KDM5 family and Kdm1a, consistent with the decreased H3K4me2/3 global expression reported at this stage (see Figure 1B). We also detected increased expression and high RPKM values of H3K27me writers and erasers at PreL and LZ stages, including polycomb writers Ezh1/2, and Kdm6a, which form the COMPASS complex with Kmt2b/c/d and Ashl2 H3K4me writers increased at these stages (Figure 5). Meiotic prophase I also showed upregulation of KDM7 family, Kdm7a and Phf2/8, that mediate gene activation programs by removal of several histone repressive marks (Figure 5). In addition, there is considerable enzymatic regulation on H3K9me mark, evidenced in the number and peak expression of H3K9me writers and erasers during all stages of prophase I, including KDM3/4 families that would mediate the decreased global H3K9me3 levels detected at this stage (see Figure 1B).
Spermiogenesis is the final phase of spermatogenesis, where the haploid RStid engage in chromatin remodeling programs involving specific histone variants incorporation, H3/4 hyperacetylation and subsequent replacement of 90%–99% histones by protamines (PRMs) to compact the nucleus. Figure 6 shows that the RStid stage presents expression of histone K acetylation writers and erasers that continued to increase expression from PD stage, including Kdm4a, Msl3, Ehmt2, Rsbn1 and Dot1l, and others that seemed to reactivate expression from previous spermatogenic and early meiotic stages. We found specifically upregulation of several HATs at RStid (Figure 6), and also Kat8 that maintained high levels from PD stage (see Figure 2) consistent with the histone hyperacetylation that takes place at this stage (see Figure 1B). The RStid stage showed high RPKM values for the H3K79me writer Dotil, the H3K27me writer Ezh2 and the H3K9me2 writer Ehmt2 (Figure 6), consistent with the high global expression of these marks at spermiogenesis stage (see Figure 1B). Additionally, RStid population showed peak expression of Rsbn1, a known specific eraser involved in decreasing H4K20me levels at spermiogenesis (Figure 6) (Li et al., 2021).
Epigenetic enzymes detected at mature sperm and one cell embryo translatome
Recent findings indicate that the sperm delivers RNAs to the oocyte at fertilization which stay stable until the activation of the embryonic genome, having putative roles at zygote formation (Smith and Spadafora, 2005; Boerke et al., 2007). Zygote’s early molecular processes involve differential epigenetic reprogramming of the parental genomes, including controlled degradation/translation of inherited RNAs (McLay and Clarke, 2003; Schulz and Harrison, 2019). Paternal RNAs were found enriched in genes associated with the ontologies of embryonic cleavage and blastocyst formation, and their removal greatly diminished embryo viability (Guo et al., 2017). Hence, the epigenetic enzymes mRNA present in the sperm RNA pool could not only be remnants of the spermatogenic process, but play a role in embryo epigenetic reprogramming. To characterize the epigenetic enzymes mRNA present in the mature sperm, we analyzed data from three datasets that performed long non-coding and mRNA RNAseq: GSE81216 (total and head sperm, Schuster et al., 2016), GSE88732 (Zhang et al., 2017), and E-MTAB-5834 (Gapp et al., 2020). For each study, we obtained the mean RPKM for each gene and converted it to percent rank to transform values into a 0-1 scale, where 0 means no detection and one is the highest detected gene in each study (see Supplementary Table S2). Then, we calculated the mean percent rank for the three studies. We found positive mRNA levels for all epigenetic enzymes in the three sperm datasets (see Supplementary Table S2), and a positive correlation between sperm and RStid mean percent ranks (Figure 7A), which favors the idea that the sperm enzymes mRNA comes from the spermiogenesis phase. Figure 7B shows a word cloud plot, where word size indicates the sperm enzymes abundance (mean percent rank). We also evaluated the epigenetic enzymes mRNA levels in meiosis II (MII) oocytes and pre-cleavage zygotes RNA-seq data (GSE169632, Zhang et al., 2022), finding positive expression for almost all of them, as it was found in sperm (see Supplementary Table S4). In addition, with the aim to elucidate if the epigenetic enzymes detected in mature sperm may participate in embryo chromatin assembly, we analyzed recently reported data that profiled the mRNA translation landscape in mouse pre-implantation embryos by Ribo-seq (Zhang et al., 2022). We analyzed the translation efficiency (TE), obtained as mean RPKM detected at ribosomes/mean RPKM detected at whole RNAseq (RPKM>.5) (see Supplementary Table S3). Figure 7C shows that several writers and erasers of all histone acetylation and methylation marks are detected in the translatome of the 1-cell embryo, where many of these mRNAs are highly detected in the sperm RNA pool (Figure 7B, enzymes in red). Although we cannot discriminate the maternal or paternal origin of the mRNAs being translated, the results obtained in the one cell embryo traslatome favors the idea that some enzymes of paternal origin could be translated and participate in the male pronucleus chromatin assembly, as it was reported for other paternal factors (Sone et al., 2005; Yao et al., 2010). To further test for paternal contribution of epigenetic enzymes to the zygotic mRNA pool, we searched for genes showing increased mean RPKM at whole RNAseq in one cell embryo compared to MII-oocytes (Fold >1), and detected 14 candidate enzymes (Table 2).
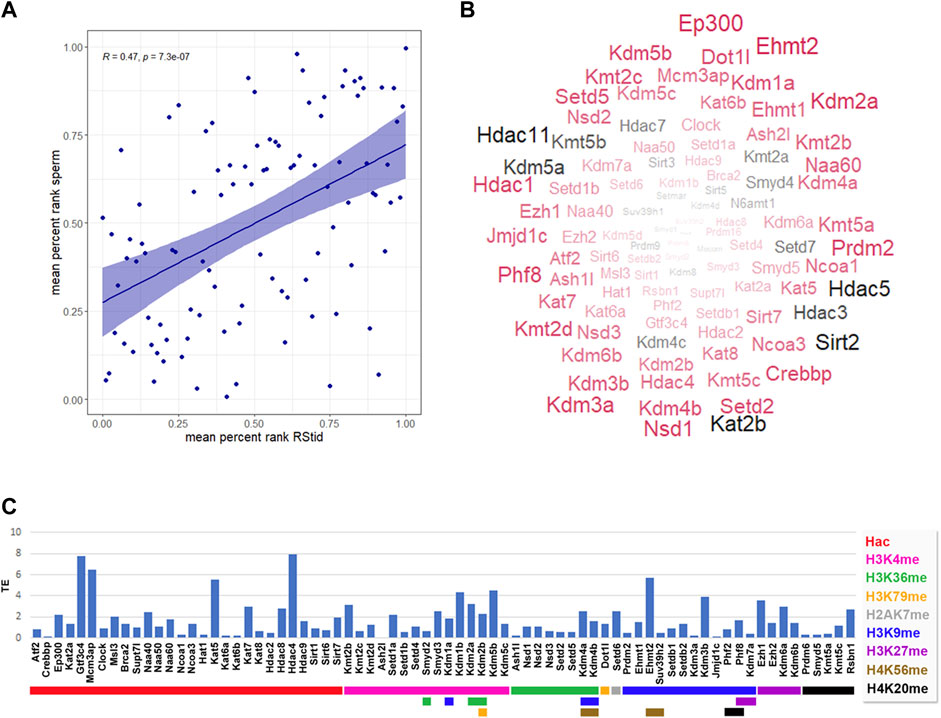
FIGURE 7. Epigenetic histone K writers and erasers detected at mature sperm RNA cargo and 1-cell embryo translatome. (A) Pearson correlation between epigenetic enzymes mean percent rank detected at RStid and sperm. (B) Mature sperm word cloud for epigenetic enzymes indicating the enzyme abundance (word size = mean percent rank) and translation efficiency (TE) detected at one cell embryo (red: TE > 0, black: TE = 0). (C) Epigenetic enzymes TE in mouse pre-implantation embryos (TE = RNA RPKM at ribosome/total RNA RPKM, RPKM>.5). Red arrows indicate epigenetic enzymes that showed increased total RNA values in the one-cell embryo compared to the meiosis II (MII oocyte), indicative of possible paternal contribution to the zygotic mRNA pool.
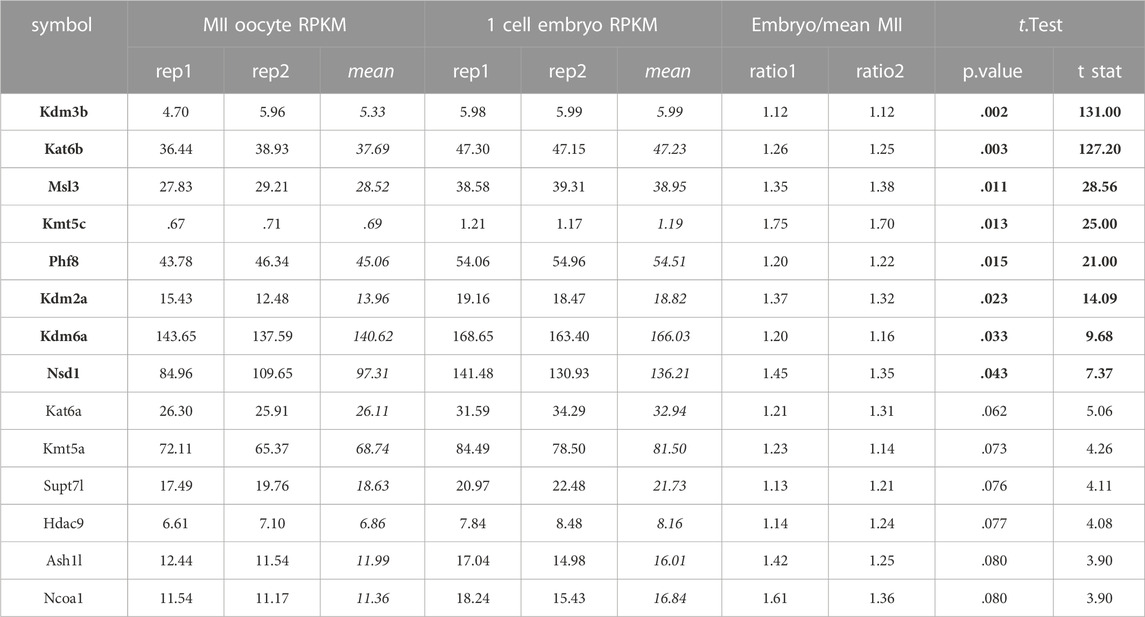
TABLE 2. Candidate epigenetic enzymes for paternal contribution. RPKM values obtained for MII oocytes and one cell embryos enzymes from GSE169632 (Zhang et al., 2022). Embryo RPKMs were converted to ratio over mean MII oocytes RPKM. One sample t-test, values in black font p < .05, values in regular font p < .1.
Discussion
Accumulating evidence has put on focus the existence of windows of vulnerability during spermatogenesis, at which environmental stressors can induce epigenetic reprogramming and the transmission of developmental, metabolic, and behavioral traits to offspring. To further elucidate the mechanisms driving histone PTMs changes during the spermatogenic process, we analyzed the epigenetic enzyme expression programs across germ cell differentiation to mature sperm. Here, we expand the knowledge on enzymes with recognized roles in spermatogenesis and provide evidence of many more whose role in male germ cells development has not been described yet. We show that cell transition across spermatogenesis is characterized by the upregulation of specific histone K methylation and acetylation writers and erasers driving the epigenome changes necessary for spermatogonia differentiation, meiosis entry and spermiogenesis. Moreover, most of these epigenetic enzymes were detected in the mature sperm mRNA pool. Histone modifying enzymes were also detected in the early zygote translatome, suggesting a possible paternal contribution of epigenetic enzymes to the zygote. Our study shows important mechanistic aspects behind transgenerational epigenetics, where epigenetic enzymes not only can respond to environmental stressors and alter the germ cell epigenome but could function as vectors of epigenetic information transmission themselves, by participating at the maternal-to-zygote transition.
The spermatogonia stage seems to be vulnerable for stress-induced epigenetic reprogramming since they reside outside the blood-testis barrier (González et al., 2020). Furthermore, current data support a dynamic stem cell model in which the fate of SGund population is context-dependent and plastic, and several epigenetic mechanisms are implicated in the regulation of spermatogonia maintenance and cell fate (Mäkelä and Hobbs, 2019). Here, we found that the transition to the SGdiff population is characterized by increased expression of writers of activation marks H3K4me and H3K36me and repression marks H3K9me and H4K20me. At the spermatogonial phase, we found high expression of H3K4me epigenetic enzymes, including Kmt2a/b/c writers and Kdm1a/b erasers. In this context, at spermatogonial stage occurs the deposition of monovalent and bivalent H3K4me marking at promoters by Kmt2b (Mll2), preparing germ cells for gene activation at late spermatogenesis and embryonic development (Tomizawa et al., 2018). Accumulating evidence also points to H3K4me2 in the maintenance of transcriptional states during cell development and, its removal by erasers expressed at spermatogonia such as KDM1A (LSD1) is a key step for epigenetic reprogramming and cell fate (Martinez-Gamero et al., 2021). KDM1A was found to mediate spermatogonia commitment and differentiation, as testicular deletion of Kdm1a leads to progressive germ cell loss, downregulation of self-renewal factors and abnormal accumulation of meiotic spermatocytes (Lambrot et al., 2015; Myrick et al., 2017). Interestingly, overexpression of Kdm1a altered the H3K4me2/3 levels and the RNA profile of sperm and embryos transgenerationally (Siklenka et al., 2015; Lismer et al., 2020). Hence, the spermatogonial phase seems to be specially sensitive to alterations in H3K4me balance linked to epigenetic inheritance. SGdiff showed a specific increase of Prdm9, responsible for H3K4me3 and H3K36me3 that mediate the double-strand breaks (DSBs) formation at meiotic hot spots (Getun et al., 2017; Sun et al., 2020). In line with this, it has been shown that meiosis entry of SGdiff is driven by increased levels of H3K36me3 (Godmann et al., 2007; Song et al., 2011; Zuo et al., 2018). Here, we found Setd2 expression until PD stage, and Setd5 specifically upregulated at SGund, suggesting their involvement in the specific H3K36me3 landscape of spermatogonia differentiation. The SGund population also expressed H3K4me/H3K36me eraser Kdm2b, a subunit of non-canonical PRC1.1 complex that was found to protect the polycomb-silenced promoters against ectopic de novo H3K36me methylation (Blackledge and Klose, 2021). Furthermore, KDM2B was recently found to erase H3K79me2/3 and to induce transcriptional repression via SIRT1 chromatin silencing (Kang et al., 2018).
It is established that SGund typically lacks heterochromatin, which forms as they differentiate to the spermatogonia population committed to meiosis (Chiarini-Garcia and Russell, 2002). The SGund showed increased expression of H3K9me erasers Kdm3a/b and Jmjd1c (Kdm3c), that were found to counteract the enzymatic activity of EHMT2 (Tachibana et al., 2007), which is highly expressed in the spermatogonia population. Methylation of H3K9 by EHMT2 blocks gene expression of the stem cell factors OCT4 and NANOG, therefore, demethylation of H3K9 by KDM3 family enzymes may be a key step in the maintenance of self-renewal of spermatogonial stem cells (Chioccarelli et al., 2020; Kuroki et al., 2020). In agreement, we found increased Ehmt1, Suv39h2 and Setdb2 expression at SGdiff stage, consistent with H3K9me2/3 deposition during spermatogonia differentiation. In particular, EHMT1 was found in a complex that binds E2F- and Myc-responsive genes to repress the mitotic program (Ogawa et al., 2002). Moreover, SGdiff population showed decreased Ezh1 and increased Kdm6b compared to SGund, suggesting that spermatogonia differentiation involves H3K27me removal. We also observed that the transition to the SGdiff population is characterized by specific HDACs upregulation. In this sense, it was shown that class IIa HDACs enzymatic activity depends on their recruitment into a complex containing class I HDAC3 and retinoid acid (RA) receptor (RAR), suggesting that these HDACs could mediate chromatin silencing induced by RA, essential for the transition of SGund into SGdiff (Parra, 2015). Moreover, histone acetylation writer Kat2b expressed in SGund functions as a co-activator for RAR and can interact with NCOA1 to promote transcription (Spencer et al., 1997), which is upregulated from SGdiff to LZ stage. SGund also showed peak expression of Kat2a, and its germ-cell-specific knockout results in abnormal chromatin dynamics, leading to increased sperm histone retention and severe reproductive phenotype (Luense et al., 2019). Finally, SGdiff population showed increased Sirt1, described as a key regulator of spermatogonia differentiation since SIRT1-deficient mice show a delay of pre-meiotic differentiation, aberrant expression of spermatogenic genes, abnormal spermatozoa with elevated DNA damage, and reduced fertility (Coussens et al., 2008; Bell et al., 2014). Therefore, the action of certain epigenetic enzymes in pre-meiotic stages seems to enable the post-meiotic transcriptome and chromatin remodeling processes.
Initiation of male meiosis is one of the most important events that coincide with spermatocyte differentiation, and meiotic prophase is accompanied by several alterations of epigenetic and gene expression programs for post-meiotic spermiogenesis (Maezawa et al., 2018). Histone acetylation writers increase during prophase I progression, and H3K9/18/23ac and H4K5/8/12/16/91ac marks have been associated with open chromatin and hot spot cores (Getun et al., 2017; Chioccarelli et al., 2020). Accordingly, MYST enzymes were previously found strongly related to meiosis control, increasing their expression from early pachytene through diplotene stages (Getun et al., 2017). Interestingly, p300/CBP family and KAT2B were found to regulate histone eviction by acetylation of transition nuclear protein (TNP) 2, affecting DNA condensation properties and interaction with histone chaperones (Pradeepa et al., 2009). Among other acetylation writers upregulated during prophase I, MSL3 binds to H3K36me3 marked sites and deposits H4K16ac, controlling meiosis entry and STRA8 (stimulated by retinoic acid 8) functions (McCarthy et al., 2022). On the other hand, we detected several SIRT enzymes throughout prophase I. Deacetylation of H3K9 by SIRT6 modulates telomeric chromatin function (Michishita et al., 2008) while SIRT7 is highly selective toward H3K18ac, and might play an upstream role in DNA repair and telomere maintenance (Wu et al., 2018). We also detected peak expression of Hdac1 and Kdm1a (Lsd1) at the PD stage, that were recently shown to interact with BEND2 and participate in DSB repair, synapsis and transcriptional repression (Ma et al., 2022). HDAC1 was also found to form a complex with DNA methyltransferase DNMT3L to regulate X chromosome compaction (Deplus et al., 2002; Zamudio et al., 2011).
During prophase I, recombination hotspots are mainly marked by H3K4me3 catalyzed by KMT2 family and PRDM9 methyltransferases (Sollier et al., 2004; Buard et al., 2009). Moreover, the levels of H3K4me3 and H3K36me3 are highly correlated at hotspots, but mutually exclusive elsewhere, and PRDM9 is capable of placing both marks on the same nucleosomes in vivo (Powers et al., 2016). It was shown that Prdm9 knockout changes the distribution of DSBs across the genome inducing defective synapses and male infertility (Paigen and Petkov, 2018; Bhattacharyya et al., 2019). We also detected peak expression of H3K79me writer Dot1L at PD stage, consistent with previous reports showing increased levels of DOT1L, and H3K79me2/3 from pachytene onwards (Ontoso et al., 2014). The heterochromatic centromeric regions and the sex body are enriched of H3K79me3, while H3K79me2 is present all over the chromatin, but is largely excluded from the sex body despite the accumulation of DOT1L (Ontoso et al., 2014). Repressive histone methylation marks H3K27me3, H3K9me3 and H4K20me3 are present in chromatin regions with reduced recombination or involved in heterochromatin formation. In vitro and in vivo models disrupting the action of H3K9me writers Suv39h1, Setdb1/2 and Ehmt2 detected during prophase I, showed an abnormal distribution of H3K9me3 at pericentromeric heterochromatin, compromised meiotic silencing of unsynapsed chromatin (MSUC), misregulation of meiotic and somatic genes, anomalous synapsis and misssegregation of chromosomes and apoptosis at pachytene stage (Peters et al., 2001; Tachibana et al., 2007; Takada et al., 2011). Interestingly, low protein diet in the father was linked to altered levels of H3K9me2 through EHMT2, that changed tRNAs in sperm and transmitted metabolic phenotypes to the offspring (Yoshida et al., 2020). Also, SETDB1 haploinsufficiency in mice was shown to induce changes in DNA methylation in transposable elements, and to influence coat color in the offspring, further linking epigenetic inheritance with altered epigenetic enzymes levels during spermatogenesis (Daxinger et al., 2016). A recent work by Barral et al., 2022 reported dual regions in mouse embryonic stem cells that rely on the SETDB1 and NSD proteins to generate H3K9me3 and H3K36me3, respectively. They found that SETDB1 removal induces loss of both marks in dual regions, gains signatures of active enhancers, and comes into contact with upregulated genes, providing a mechanistic insight by which genes are controlled by heterochromatin (Barral et al., 2022). Moreover, NSD1-mediated H3K36me2 was found to prevent H3K27me3 deposition by PRC2, modulating PRC2-mediated H3K27me domains demarcation (Blackledge and Klose, 2021). In line with this, we detected the expression of H3K27me writers Ezh1/2 at PreL and LZ stages that were found involved in mammalian X chromosome inactivation (Schuettengruber et al., 2007). Furthermore, Kdm6a is part of the COMPASS complex with Kmt2b/c/d and Ashl2 that activate genes in response to RA by H3K4me deposition and H3K27me removal (Lavery et al., 2020) and moreover, alterations in Kdm6a expression induce defects that persisted transgenerationally (Siklenka et al., 2015; Lesch et al., 2019). Additionally, the loss of H3K27me3 from bivalent signature in the promoter of endonuclease SPO11 induces chromatin activation that favors meiotic entry (Hammoud et al., 2014). We also detected high expression of H2AZK7me1 writer Setd6 at PreL stage, which in ESC was found with H3K27me3 close to differentiation marker genes and removed upon RA signal (Binda et al., 2013), suggesting that SETD6 may have a role at early male meiosis. Finally, we observed expression of the H4K12me writer N6amt1 at LZ stage, a recently described epigenetic mark found at promoters of genes encoding cell cycle regulators (Metzger et al., 2019).
Chromatin organization dramatically changes during mid-to late-spermiogenesis, due to histone eviction and replacing by PRMs to facilitate the condensation and packaging of the paternal genome. In mice, 1%–10% of histones are retained in sperm chromatin and form a heterogeneous mixture of nucleo-histones and nucleo-protamines (Luense et al., 2016). At the spermiogenesis phase, histone acetylation is essential in destabilization and remodeling of nucleosomes. Recently, it has been reported the specific expression of Kat2b and Kat8 at RStid stage, suggesting that these acetyltransferases are responsible for histone hyperacetylation prior to the histone-to-protamine transition (Li et al., 2021). In line with this, we observed that the RStid population showed high transcriptional levels of H4K16ac writers Msl3 and Kat8, and also p300/CBP and Kat2b that keep their expression from spermatocyte stage, due to their crucial role to regulate histone eviction (Pradeepa et al., 2009). Moreover, KAT2B was found in spermatids and involved in H3K9ac, an epigenetic mark detected at unmethylated active genes/enhancers (Hammoud et al., 2014) that could influence gene expression directly after fertilization (Steilmann et al., 2011). Additionally, Hat1 expression was found related to the incorporation of H4K5/12ac and H3.3 variant at DSBs sites and the promotion of DNA repair (Yang et al., 2013). H4K5/8/12 marks have been also observed just before eviction of histones during spermiogenesis (Meistrich et al., 1992; Hecht et al., 2009) and moreover, H4K8/12ac was detected prior to full decondensation of the sperm nucleus, suggesting that these marks are transmitted to the zygote (van der Heijden et al., 2006).
Multiple histone methylation have been also identified in spermatids, pointing to a balance of “opened” and “closed” chromatin regions during the histone-to-protamine transition (Wang et al., 2019). Here, we detected upregulated expression of H3K79me writer Dotl1, H3K9me writer Ehtm2 and H3K27me writer Ezh2 at RStid stage. It was found that DOTL1 is enriched in post-meiotic stages of mouse germ cells and precedes the histone-to-protamine transition (Dottermusch-Heidel et al., 2014). Moreover, Ezh2 and Ehmt2 methyltransferases were detected at spermatid stage, suggesting that spermatids have a potential preference for epigenetic transcriptional repression and heterochromatin formation (Li et al., 2021). During spermiogenesis, there is a reactivation of transcription from MSUC and MSCI sites that is enabled by the deposition of histone crotonylation (Kcr). It was proposed that CDYL, an HKcr eraser, prevents post-meiotic chromatin reactivation by binding to H3K9me3 and H3K27me2/3 marked sites and facilitating H3K9me2 deposition by EHMT2 (Mulligan et al., 2008). Moreover, there is specific H3K27me deposition to establish bivalency at developmental genes (Maezawa et al., 2018), and proteomic studies found substantial H3K27me3/H3K36me2 double marking in RStid and sperm (Luense et al., 2016).
It is established that mature sperm transport a cargo of miRNAs, tsRNAs, lncRNAs, circRNAs, and protein-coding mRNAs, that carry an epigenetic blueprint involved in early embryo development (Guo et al., 2017). Some of these RNAs remain from the last stages of elongated spermatids, and others are acquired along the passage through the epididymis, as sperm absorb epididymosomes released by somatic cells (Trigg et al., 2019). Here, we found that the mature sperm transport a signature of epigenetic enzymes mRNAs similar to the one detected at spermiogenesis phase, and most of these enzymes show positive translation at one cell embryo. After fertilization, the zygote genome is transcriptionally silent, and cellular processes are carried on with inherited RNAs and proteins until the onset of zygotic genome activation (ZGA) around the two to four cells stage (Schulz and Harrison, 2019). Also, at 1-cell stage there is significant removal of some maternal RNAs and specific translation of others, including RNAs specifically delivered by the sperm like the egg-activating factor PLC-zeta (Sone et al., 2005; Yao et al., 2010). The parental pronuclei have asymmetric reprogramming capacities and the reprogramming factors reside predominantly in the male pronucleus. In this context, previous work suggested that KMT5C deposits H4K20me3 to allow the timely and coordinated progression of replication after fertilization (Eid et al., 2016). Also, it was found that EHMT2 activates soon after fertilization and deposit H3K9me2 patterns in the paternal genome (Ma et al., 2015). Here, we detected high sperm values and embryo translation of several enzymes involved in histone acetylation and methylation, including the H4K20me2/3 writer Kmt5a/c, the H3K9me2 writers Ehmt1/2, and the H3K9me3 writers Suv39h2 and Setdb1/2, with established roles in chromatin structure organization. Moreover, some of these enzymes were found increased in the embryo compared to the MII oocyte, as indicative of paternal contribution to their pool levels. Hence, sperm’s epigenetic enzymes contribution to the zygote’s mRNA pool could cooperate to the translation of the machinery necessary for the extensive chromatin remodeling that takes place to allow a new developmental program to start.
Conclusion
In summary, the analysis performed here shows important windows during spermatogenesis, where interference with epigenetic enzymes gene expression may have phenotypic consequences in the offspring, even when they are not inherited. Moreover, we show that epigenetic enzymes mRNA could become functional in pre-cleavage zygotes and contribute to early chromatin organization with deep implications in future embryo development. The epigenetic enzyme’s paternal contribution could be another mechanism for epigenetic inheritance that deserves further consideration.
Data availability statement
The datasets presented in this study can be found in online repositories. The names of the repository/repositories and accession number (s) can be found below: https://github.com/Gonzalez-Lab/Gonzalez-2022-germ-cells, 1.
Author contributions
BG and CG conceived the project, analyzed the data and wrote the manuscript. GB, MS, and CP contributed to data mining and analysis. AV contributed to the critical reading and editing of the manuscript. All authors read and approved the submitted version.
Funding
This work was supported by Agencia Nacional de Promoción Científica y Tecnológica (PICT 2019-00171, BG) and Fundación Científica Felipe Fiorellino (AV and CG).
Conflict of interest
The authors declare that the research was conducted in the absence of any commercial or financial relationships that could be construed as a potential conflict of interest.
Publisher’s note
All claims expressed in this article are solely those of the authors and do not necessarily represent those of their affiliated organizations, or those of the publisher, the editors and the reviewers. Any product that may be evaluated in this article, or claim that may be made by its manufacturer, is not guaranteed or endorsed by the publisher.
Supplementary material
The Supplementary Material for this article can be found online at: https://www.frontiersin.org/articles/10.3389/fcell.2023.1086573/full#supplementary-material.
References
Bale, T. L. (2015). Epigenetic and transgenerational reprogramming of brain development. Nat. Rev. Neurosci. 16 (6), 332–344. doi:10.1038/nrn3818
Barral, A., Pozo, G., Ducrot, L., Papadopoulos, G. L., Sauzet, S., Oldfield, A. J., et al. (2022). SETDB1/NSD-dependent H3K9me3/H3K36me3 dual heterochromatin maintains gene expression profiles by bookmarking poised enhancers. Mol. Cell 82 (4), 816–832.e12. e12. doi:10.1016/j.molcel.2021.12.037
Bell, E. L., Nagamori, I., Williams, E. O., Del Rosario, A. M., Bryson, B. D., Watson, N., et al. (2014). SirT1 is required in the male germ cell for differentiation and fecundity in mice. Dev. Camb. Engl. 141 (18), 3495–3504. doi:10.1242/dev.110627
Bhattacharyya, T., Walker, M., Powers, N. R., Brunton, C., Fine, A. D., Petkov, P. M., et al. (2019). Prdm9 and meiotic cohesin proteins cooperatively promote DNA double-strand break formation in mammalian spermatocytes. Curr. Biol. CB 29 (6), 1002–1018. e7. doi:10.1016/j.cub.2019.02.007
Binda, O., Sevilla, A., LeRoy, G., Lemischka, I. R., Garcia, B. A., and Richard, S. (2013). SETD6 monomethylates H2AZ on lysine 7 and is required for the maintenance of embryonic stem cell self-renewal. Epigenetics 8 (2), 177–183. doi:10.4161/epi.23416
Black, J. C., Van Rechem, C., and Whetstine, J. R. (2012). Histone lysine methylation dynamics: Establishment, regulation, and biological impact. Mol. Cell 48 (4), 491–507. doi:10.1016/j.molcel.2012.11.006
Blackledge, N. P., and Klose, R. J. (2021). The molecular principles of gene regulation by Polycomb repressive complexes. Nat. Rev. Mol. Cell Biol. 22 (12), 815–833. doi:10.1038/s41580-021-00398-y
Boerke, A., Dieleman, S. J., and Gadella, B. M. (2007). A possible role for sperm RNA in early embryo development. Theriogenology 68 (1). doi:10.1016/j.theriogenology.2007.05.058
Brykczynska, U., Hisano, M., Erkek, S., Ramos, L., Oakeley, E. J., Roloff, T. C., et al. (2010). Repressive and active histone methylation mark distinct promoters in human and mouse spermatozoa. Nat. Struct. Mol. Biol. 17 (6), 679–687. doi:10.1038/nsmb.1821
Buard, J., Barthès, P., Grey, C., and de Massy, B. (2009). Distinct histone modifications define initiation and repair of meiotic recombination in the mouse. EMBO J. 28 (17), 2616–2624. doi:10.1038/emboj.2009.207
Carrell, D. T. (2012). Epigenetics of the male gamete. Fertil. Steril. 97 (2), 267–274. doi:10.1016/j.fertnstert.2011.12.036
Chiarini-Garcia, H., and Russell, L. D. (2002). Characterization of mouse spermatogonia by transmission electron microscopy. Reprod. Camb. Engl. 123 (4), 567–577. doi:10.1530/rep.0.1230567
Chioccarelli, T., Pierantoni, R., Manfrevola, F., Porreca, V., Fasano, S., Chianese, R., et al. (2020). Histone post-translational modifications and CircRNAs in mouse and human spermatozoa: Potential epigenetic marks to assess human sperm quality. J. Clin. Med. 9 (3), 640. doi:10.3390/jcm9030640
Coussens, M., Maresh, J. G., Yanagimachi, R., Maeda, G., and Allsopp, R. (2008). Sirt1 deficiency attenuates spermatogenesis and germ cell function. PloS one 3 (2), e1571. doi:10.1371/journal.pone.0001571
Daxinger, L., Oey, H., Isbel, L., Whitelaw, N. C., Youngson, N. A., Spurling, A., et al. (2016). Hypomethylation of ERVs in the sperm of mice haploinsufficient for the histone methyltransferase Setdb1 correlates with a paternal effect on phenotype. Sci. Rep. 6, 25004. doi:10.1038/srep25004
Deplus, R., Brenner, C., Burgers, W. A., Putmans, P., Kouzarides, T., de Launoit, Y., et al. (2002). Dnmt3L is a transcriptional repressor that recruits histone deacetylase. Nucleic acids Res. 30 (17), 3831–3838. doi:10.1093/nar/gkf509
Dottermusch-Heidel, C., Klaus, E. S., Gonzalez, N. H., Bhushan, S., Meinhardt, A., Bergmann, M., et al. (2014). H3K79 methylation directly precedes the histone-to-protamine transition in mammalian spermatids and is sensitive to bacterial infections. Andrology 2 (5), 655–665. doi:10.1111/j.2047-2927.2014.00248.x
Eid, A., Rodriguez-Terrones, D., Burton, A., and Torres-Padilla, M. E. (2016). SUV4-20 activity in the preimplantation mouse embryo controls timely replication. Genes & Dev. 30 (22), 2513–2526. doi:10.1101/gad.288969.116
Gapp, K., van Steenwyk, G., Germain, P. L., Matsushima, W., Rudolph, K., Manuella, F., et al. (2020). Alterations in sperm long RNA contribute to the epigenetic inheritance of the effects of postnatal trauma. Mol. psychiatry 25 (9), 2162–2174. doi:10.1038/s41380-018-0271-6
Getun, I. V., Wu, Z., Fallahi, M., Ouizem, S., Liu, Q., Li, W., et al. (2017). Functional roles of acetylated histone marks at mouse meiotic recombination hot spots. Mol. Cell. Biol. 37 (3), 009422–e1015. doi:10.1128/MCB.00942-15
Godmann, M., Auger, V., Ferraroni-Aguiar, V., Di Sauro, A., Sette, C., Behr, R., et al. (2007). Dynamic regulation of histone H3 methylation at lysine 4 in mammalian spermatogenesis. Biol. reproduction 77 (5), 754–764. doi:10.1095/biolreprod.107.062265
González, B., Gancedo, S. N., Garazatua, S., Roldán, E., Vitullo, A. D., and González, C. R. (2020). Dopamine receptor D1 contributes to cocaine epigenetic reprogramming of histone modifications in male germ cells. Front. Cell Dev. Biol. 8, 216. doi:10.3389/fcell.2020.00216
Guo, L., Chao, S. B., Xiao, L., Wang, Z. B., Meng, T. G., Li, Y. Y., et al. (2017). Sperm-carried RNAs play critical roles in mouse embryonic development. Oncotarget 8 (40), 67394–67405. doi:10.18632/oncotarget.18672
Hammoud, S. S., Low, D. H., Yi, C., Carrell, D. T., Guccione, E., and Cairns, B. R. (2014). Chromatin and transcription transitions of mammalian adult germline stem cells and spermatogenesis. Cell stem Cell 15 (2), 239–253. doi:10.1016/j.stem.2014.04.006
Hammoud, S. S., Nix, D. A., Zhang, H., Purwar, J., Carrell, D. T., and Cairns, B. R. (2009). Distinctive chromatin in human sperm packages genes for embryo development. Nature 460 (7254), 473–478. doi:10.1038/nature08162
Hecht, N., Behr, R., Hild, A., Bergmann, M., Weidner, W., and Steger, K. (2009). The common marmoset (Callithrix jacchus) as a model for histone and protamine expression during human spermatogenesis. Hum. Reprod. Oxf. Engl. 24 (3), 536–545. doi:10.1093/humrep/den390
Kang, J. Y., Kim, J. Y., Kim, K. B., Park, J. W., Cho, H., Hahm, J. Y., et al. (2018). KDM2B is a histone H3K79 demethylase and induces transcriptional repression via sirtuin-1-mediated chromatin silencing. FASEB J. official Publ. Fed. Am. Soc. Exp. Biol. 32 (10), 5737–5750. doi:10.1096/fj.201800242R
Kouzarides, T. (2007). Chromatin modifications and their function. Cell 128 (4), 693–705. doi:10.1016/j.cell.2007.02.005
Kuroki, S., Maeda, R., Yano, M., Kitano, S., Miyachi, H., Fukuda, M., et al. (2020). H3K9 demethylases JMJD1A and JMJD1B control prospermatogonia to spermatogonia transition in mouse germline. Stem Cell Rep. 15 (2), 424–438. doi:10.1016/j.stemcr.2020.06.013
Lambrot, R., Chan, D., Shao, X., Aarabi, M., Kwan, T., Bourque, G., et al. (2021). Whole-genome sequencing of H3K4me3 and DNA methylation in human sperm reveals regions of overlap linked to fertility and development. Cell Rep. 36 (3), 109418. doi:10.1016/j.celrep.2021.109418
Lambrot, R., Lafleur, C., and Kimmins, S. (2015). The histone demethylase KDM1A is essential for the maintenance and differentiation of spermatogonial stem cells and progenitors. FASEB J. official Publ. Fed. Am. Soc. Exp. Biol. 29 (11), 4402–4416. doi:10.1096/fj.14-267328
Lavery, W. J., Barski, A., Wiley, S., Schorry, E. K., and Lindsley, A. W. (2020). KMT2C/D COMPASS complex-associated diseases [KCDCOM-ADs]: An emerging class of congenital regulopathies. Clin. epigenetics 12 (1), 10. doi:10.1186/s13148-019-0802-2
Lee, G. S., and Conine, C. C. (2022). The transmission of intergenerational epigenetic information by sperm microRNAs. Epigenomes 6 (2), 12. doi:10.3390/epigenomes6020012
Lesch, B. J., Tothova, Z., Morgan, E. A., Liao, Z., Bronson, R. T., Ebert, B. L., et al. (2019). Intergenerational epigenetic inheritance of cancer susceptibility in mammals. eLife 8, e39380. doi:10.7554/eLife.39380
Li, Y., Mi, P., Chen, X., Wu, J., Qin, W., Shen, Y., et al. (2021). Dynamic profiles and transcriptional preferences of histone modifications during spermiogenesis. Endocrinology 162 (1), bqaa210. doi:10.1210/endocr/bqaa210
Lismer, A., Siklenka, K., Lafleur, C., Dumeaux, V., and Kimmins, S. (2020). Sperm histone H3 lysine 4 trimethylation is altered in a genetic mouse model of transgenerational epigenetic inheritance. Nucleic acids Res. 48 (20), 11380–11393. doi:10.1093/nar/gkaa712
Love, M. I., Huber, W., and Anders, S. (2014). Moderated estimation of fold change and dispersion for RNA-seq data with DESeq2. Genome Biol. 15 (12), 550. doi:10.1186/s13059-014-0550-8
Luense, L. J., Donahue, G., Lin-Shiao, E., Rangel, R., Weller, A. H., Bartolomei, M. S., et al. (2019). Gcn5-Mediated histone acetylation governs nucleosome dynamics in spermiogenesis. Dev. Cell 51 (6), 745–758. e6. doi:10.1016/j.devcel.2019.10.024
Luense, L. J., Wang, X., Schon, S. B., Weller, A. H., Lin Shiao, E., Bryant, J. M., et al. (2016). Comprehensive analysis of histone post-translational modifications in mouse and human male germ cells. Epigenetics chromatin 9, 24. doi:10.1186/s13072-016-0072-6
Ma, L., Xie, D., Luo, M., Lin, X., Nie, H., Chen, J., et al. (2022). Identification and characterization of BEND2 as a key regulator of meiosis during mouse spermatogenesis. Sci. Adv. 8 (21), eabn1606. doi:10.1126/sciadv.abn1606
Ma, X. S., Chao, S. B., Huang, X. J., Lin, F., Qin, L., Wang, X. G., et al. (2015). The dynamics and regulatory mechanism of pronuclear H3k9me2 asymmetry in mouse zygotes. Sci. Rep. 5, 17924. doi:10.1038/srep17924
Maezawa, S., Hasegawa, K., Yukawa, M., Kubo, N., Sakashita, A., Alavattam, K. G., et al. (2018). Polycomb protein SCML2 facilitates H3K27me3 to establish bivalent domains in the male germline. Proc. Natl. Acad. Sci. U. S. A. 115 (19), 4957–4962. doi:10.1073/pnas.1804512115
Mäkelä, J. A., and Hobbs, R. M. (2019). Molecular regulation of spermatogonial stem cell renewal and differentiation. Reprod. Camb. Engl. 158 (5), R169–R187. doi:10.1530/REP-18-0476
Martinez-Gamero, C., Malla, S., and Aguilo, F. (2021). LSD1: Expanding functions in stem cells and differentiation. Cells 10 (11), 3252. doi:10.3390/cells10113252
Mayorek, N., Schlossberg, M., Mansour, Y., Pillar, N., Stein, I., Mushasha, F., et al. (2022). 2-hydroxyglutarate controls centromere and heterochromatin conformation and function in the male germline. bioRxiv 29, 493890. doi:10.1101/2022.05.29.493890
McCarthy, A., Sarkar, K., Martin, E. T., Upadhyay, M., Jang, S., Williams, N. D., et al. (2022). Msl3 promotes germline stem cell differentiation in female Drosophila. Dev. Camb. Engl. 149 (1), dev199625. doi:10.1242/dev.199625
McLay, D., and Clark, H. J. (2003). Remodelling the paternal chromatin at fertilization in mammals. Reproduction 125 (5), 625–633. doi:10.1530/rep.0.1250625
Meistrich, M. L., Trostle-Weige, P. K., Lin, R., Bhatnagar, Y. M., and Allis, C. D. (1992). Highly acetylated H4 is associated with histone displacement in rat spermatids. Mol. reproduction Dev. 31 (3), 170–181. doi:10.1002/mrd.1080310303
Metzger, E., Wang, S., Urban, S., Willmann, D., Schmidt, A., Offermann, A., et al. (2019). KMT9 monomethylates histone H4 lysine 12 and controls proliferation of prostate cancer cells. Nat. Struct. Mol. Biol. 26 (5), 361–371. doi:10.1038/s41594-019-0219-9
Michishita, E., McCord, R. A., Berber, E., Kioi, M., Padilla-Nash, H., Damian, M., et al. (2008). SIRT6 is a histone H3 lysine 9 deacetylase that modulates telomeric chromatin. Nature 452 (7186), 492–496. doi:10.1038/nature06736
Miller, J. L., and Grant, P. A. (2013). The role of DNA methylation and histone modifications in transcriptional regulation in humans. Sub-cellular Biochem. 61, 289–317. doi:10.1007/978-94-007-4525-4_13
Mosammaparast, N., and Shi, Y. (2010). Reversal of histone methylation: Biochemical and molecular mechanisms of histone demethylases. Annu. Rev. Biochem. 79, 155–179. doi:10.1146/annurev.biochem.78.070907.103946
Mulligan, P., Westbrook, T. F., Ottinger, M., Pavlova, N., Chang, B., Macia, E., et al. (2008). CDYL bridges REST and histone methyltransferases for gene repression and suppression of cellular transformation. Mol. Cell 32 (5), 718–726. doi:10.1016/j.molcel.2008.10.025
Myrick, D. A., Christopher, M. A., Scott, A. M., Simon, A. K., Donlin-Asp, P. G., Kelly, W. G., et al. (2017). KDM1A/LSD1 regulates the differentiation and maintenance of spermatogonia in mice. PloS one 12 (5), e0177473. doi:10.1371/journal.pone.0177473
Nebbioso, A., Tambaro, F. P., Dell'Aversana, C., and Altucci, L. (2018). Cancer epigenetics: Moving forward. PLoS Genet. 14 (6), e1007362. doi:10.1371/journal.pgen.1007362
Ogawa, H., Ishiguro, K., Gaubatz, S., Livingston, D. M., and Nakatani, Y. (2002). A complex with chromatin modifiers that occupies E2F- and Myc-responsive genes in G0 cells. Sci. (New York, N.Y.) 296 (5570), 1132–1136. doi:10.1126/science.1069861
Okada, Y. (2022). Sperm chromatin condensation: Epigenetic mechanisms to compact the genome and spatiotemporal regulation from inside and outside the nucleus. Genes & Genet. Syst. 97 (1), 41–53. doi:10.1266/ggs.21-00065
Ontoso, D., Kauppi, L., Keeney, S., and San-Segundo, P. A. (2014). Dynamics of DOT1L localization and H3K79 methylation during meiotic prophase I in mouse spermatocytes. Chromosoma 123 (1-2), 147–164. doi:10.1007/s00412-013-0438-5
Paigen, K., and Petkov, P. M. (2018). PRDM9 and its role in genetic recombination. Trends Genet. TIG 34 (4), 291–300. doi:10.1016/j.tig.2017.12.017
Parra, M. (2015). Class IIa HDACs - new insights into their functions in physiology and pathology. FEBS J. 282 (9), 1736–1744. doi:10.1111/febs.13061
Peters, A. H., O'Carroll, D., Scherthan, H., Mechtler, K., Sauer, S., Schöfer, C., et al. (2001). Loss of the Suv39h histone methyltransferases impairs mammalian heterochromatin and genome stability. Cell 107 (3), 323–337. doi:10.1016/s0092-8674(01)00542-6
Powers, N. R., Parvanov, E. D., Baker, C. L., Walker, M., Petkov, P. M., and Paigen, K. (2016). The meiotic recombination activator PRDM9 trimethylates both H3K36 and H3K4 at recombination hotspots in vivo. PLoS Genet. 12 (6), e1006146. doi:10.1371/journal.pgen.1006146
Prachayasittikul, V., Prathipati, P., Pratiwi, R., Phanus-Umporn, C., Malik, A. A., Schaduangrat, N., et al. (2017). Exploring the epigenetic drug discovery landscape. Expert Opin. drug Discov. 12 (4), 345–362. doi:10.1080/17460441.2017.1295954
Pradeepa, M. M., Nikhil, G., Hari Kishore, A., Bharath, G. N., Kundu, T. K., and Rao, M. R. (2009). Acetylation of transition protein 2 (TP2) by KAT3B (p300) alters its DNA condensation property and interaction with putative histone chaperone NPM3. J. Biol. Chem. 284 (43), 29956–29967. doi:10.1074/jbc.M109.052043
Rajender, S., Avery, K., and Agarwal, A. (2011). Epigenetics, spermatogenesis and male infertility. Mutat. Res. 727 (3), 62–71. doi:10.1016/j.mrrev.2011.04.002
Schuettengruber, B., Chourrout, D., Vervoort, M., Leblanc, B., and Cavalli, G. (2007). Genome regulation by polycomb and trithorax proteins. Cell 128 (4), 735–745. doi:10.1016/j.cell.2007.02.009
Schulz, K. N., and Harrison, M. M. (2019). Mechanisms regulating zygotic genome activation. Nat. Rev. Genet. 20 (4), 221–234. doi:10.1038/s41576-018-0087-x
Schuster, A., Tang, C., Xie, Y., Ortogero, N., Yuan, S., and Yan, W. (2016). SpermBase: A database for sperm-borne RNA contents. Biol. reproduction 95 (5), 99. doi:10.1095/biolreprod.116.142190
Shirakawa, T., Yaman-Deveci, R., Tomizawa, S., Kamizato, Y., Nakajima, K., Sone, H., et al. (2013). An epigenetic switch is crucial for spermatogonia to exit the undifferentiated state toward a Kit-positive identity. Dev. Camb. Engl. 140 (17), 3565–3576. doi:10.1242/dev.094045
Siklenka, K., Erkek, S., Godmann, M., Lambrot, R., McGraw, S., Lafleur, C., et al. (2015). Disruption of histone methylation in developing sperm impairs offspring health transgenerationally. Sci. (New York, N.Y.) 350 (6261), aab2006. doi:10.1126/science.aab2006
Smith, K., and Spadafora, C. (2005). Sperm-mediated gene transfer: applications and implications. Bioessays. 27 (5), 551–562. doi:10.1002/bies.20211
Sollier, J., Lin, W., Soustelle, C., Suhre, K., Nicolas, A., Géli, V., et al. (2004). Set1 is required for meiotic S-phase onset, double-strand break formation and middle gene expression. EMBO J. 23 (9), 1957–1967. doi:10.1038/sj.emboj.7600204
Sone, Y., Ito, M., Shirakawa, H., Shikano, T., Takeuchi, H., Kinoshita, K., et al. (2005). Nuclear translocation of phospholipase C-zeta, an egg-activating factor, during early embryonic development. Biochem. biophysical Res. Commun. 330 (3), 690–694. doi:10.1016/j.bbrc.2005.03.032
Song, N., Liu, J., An, S., Nishino, T., Hishikawa, Y., and Koji, T. (2011). Immunohistochemical analysis of histone H3 modifications in germ cells during mouse spermatogenesis. Acta Histochem. Cytochem. 44 (4), 183–190. doi:10.1267/ahc.11027
Spencer, T. E., Jenster, G., Burcin, M. M., Allis, C. D., Zhou, J., Mizzen, C. A., et al. (1997). Steroid receptor coactivator-1 is a histone acetyltransferase. Nature 389 (6647), 194–198. doi:10.1038/38304
Steilmann, C., Paradowska, A., Bartkuhn, M., Vieweg, M., Schuppe, H. C., Bergmann, M., et al. (2011). Presence of histone H3 acetylated at lysine 9 in male germ cells and its distribution pattern in the genome of human spermatozoa. Reproduction, Fertil. Dev. 23 (8), 997–1011. doi:10.1071/RD10197
Sun, Z., Zhang, Y., Jia, J., Fang, Y., Tang, Y., Wu, H., et al. (2020). H3K36me3, message from chromatin to DNA damage repair. Cell & Biosci. 10, 9. doi:10.1186/s13578-020-0374-z
Tachibana, M., Nozaki, M., Takeda, N., and Shinkai, Y. (2007). Functional dynamics of H3K9 methylation during meiotic prophase progression. EMBO J. 26 (14), 3346–3359. doi:10.1038/sj.emboj.7601767
Takada, Y., Naruse, C., Costa, Y., Shirakawa, T., Tachibana, M., Sharif, J., et al. (2011). HP1γ links histone methylation marks to meiotic synapsis in mice. Dev. Camb. Engl. 138 (19), 4207–4217. doi:10.1242/dev.064444
Tomizawa, S. I., Kobayashi, Y., Shirakawa, T., Watanabe, K., Mizoguchi, K., Hoshi, I., et al. (2018). Kmt2b conveys monovalent and bivalent H3K4me3 in mouse spermatogonial stem cells at germline and embryonic promoters. Dev. Camb. Engl. 145 (23), dev169102. doi:10.1242/dev.169102
Trigg, N. A., Eamens, A. L., and Nixon, B. (2019). The contribution of epididymosomes to the sperm small RNA profile. Reprod. Camb. Engl. 157 (6), R209–R223. doi:10.1530/REP-18-0480
van der Heijden, G. W., Derijck, A. A., Ramos, L., Giele, M., van der Vlag, J., and de Boer, P. (2006). Transmission of modified nucleosomes from the mouse male germline to the zygote and subsequent remodeling of paternal chromatin. Dev. Biol. 298 (2), 458–469. doi:10.1016/j.ydbio.2006.06.051
Wang, T., Gao, H., Li, W., and Liu, C. (2019). Essential role of histone replacement and modifications in male fertility. Front. Genet. 10, 962. doi:10.3389/fgene.2019.00962
Wang, Y., Iwamori, T., Kaneko, T., Iida, H., and Iwamori, N. (2021). Comparative distributions of RSBN1 and methylated histone H4 Lysine 20 in the mouse spermatogenesis. PloS one 16 (6), e0253897. doi:10.1371/journal.pone.0253897
Wapenaar, H., and Dekker, F. J. (2016). Histone acetyltransferases: Challenges in targeting bi-substrate enzymes. Clin. epigenetics 8, 59. doi:10.1186/s13148-016-0225-2
Wickham, H., Averick, M., Bryan, J., Chang, W., D'Agostino, L., Francois, R., et al. (2019). Welcome to the tidyverse. J. Open Source Softw. 4 (43), 1686. doi:10.21105/joss.01686
Wu, D., Li, Y., Zhu, K. S., Wang, H., and Zhu, W. G. (2018). Advances in cellular characterization of the sirtuin isoform, SIRT7. Front. Endocrinol. 9, 652. doi:10.3389/fendo.2018.00652
Yamaguchi, K., Hada, M., Fukuda, Y., Inoue, E., Makino, Y., Katou, Y., et al. (2018). Re-Evaluating the localization of sperm-retained histones revealed the modification-dependent accumulation in specific genome regions. Cell Rep. 23 (13), 3920–3932. doi:10.1016/j.celrep.2018.05.094
Yang, X., Li, L., Liang, J., Shi, L., Yang, J., Yi, X., et al. (2013). Histone acetyltransferase 1 promotes homologous recombination in DNA repair by facilitating histone turnover. J. Biol. Chem. 288 (25), 18271–18282. doi:10.1074/jbc.M113.473199
Yao, C. J., Xu, W. J., Gong, X. L., Zhou, Y., Yan, Z. Q., Zhu, Z. J., et al. (2010). The role of Dby mRNA in early development of male mouse zygotes. Asian J. Androl. 12 (4), 567–577. doi:10.1038/aja.2010.28
Yoshida, K., Maekawa, T., Ly, N. H., Fujita, S. I., Muratani, M., Ando, M., et al. (2020). ATF7-Dependent epigenetic changes are required for the intergenerational effect of a paternal low-protein diet. Mol. Cell 78 (3), 445–458. e6. doi:10.1016/j.molcel.2020.02.028
Zamudio, N. M., Scott, H. S., Wolski, K., Lo, C. Y., Law, C., Leong, D., et al. (2011). DNMT3L is a regulator of X chromosome compaction and post-meiotic gene transcription. PloS one 6 (3), e18276. doi:10.1371/journal.pone.0018276
Zhang, C., Wang, M., Li, Y., and Zhang, Y. (2022). Profiling and functional characterization of maternal mRNA translation during mouse maternal-to-zygotic transition. Sci. Adv. 8 (5), eabj3967. doi:10.1126/sciadv.abj3967
Zhang, X., Gao, F., Fu, J., Zhang, P., Wang, Y., and Zeng, X. (2017). Systematic identification and characterization of long non-coding RNAs in mouse mature sperm. PloS one 12 (3), e0173402. doi:10.1371/journal.pone.0173402
Keywords: spermatogenesis, epigenetic enzymes, histone post-traslational modifications, sperm, zygote
Citation: Barbero G, de Sousa Serro MG, Perez Lujan C, Vitullo AD, González CR and González B (2023) Transcriptome profiling of histone writers/erasers enzymes across spermatogenesis, mature sperm and pre-cleavage embryo: Implications in paternal epigenome transitions and inheritance mechanisms. Front. Cell Dev. Biol. 11:1086573. doi: 10.3389/fcell.2023.1086573
Received: 01 November 2022; Accepted: 04 January 2023;
Published: 27 January 2023.
Edited by:
Mengcheng Luo, Wuhan University, ChinaReviewed by:
Jianqiang Bao, University of Science and Technology of China, ChinaJulie Cocquet, INSERM U1016 Institut Cochin, France
Copyright © 2023 Barbero, de Sousa Serro, Perez Lujan, Vitullo, González and González. This is an open-access article distributed under the terms of the Creative Commons Attribution License (CC BY). The use, distribution or reproduction in other forums is permitted, provided the original author(s) and the copyright owner(s) are credited and that the original publication in this journal is cited, in accordance with accepted academic practice. No use, distribution or reproduction is permitted which does not comply with these terms.
*Correspondence: Betina González, bgonzalez@ffyb.uba.ar
†These authors share last authorship