Non-canonical autophagy in aging and age-related diseases
- 1Molecular Biology, Cell Biology and Biochemistry Department, Brown University, Providence, RI, United States
- 2Wheaton College, Biology Department, Norton, MA, United States
Autophagy, one of the arms of proteostasis, influences aging and age-related diseases. Recently, the discovery of additional roles of autophagy-related proteins in non-canonical degradation and secretion has revealed alternative fates of autophagic cargo. Some of these non-canonical pathways have been linked to neurodegenerative diseases and improving the understanding of this link is crucial for their potential targetability in aging and age-related diseases. This review discusses recent investigations of the involvement of non-canonical autophagy players and pathways in age-related diseases that are now beginning to be discovered. Unraveling these pathways and their relation to classical autophagy could unearth a fascinating new layer of proteostasis regulation during normal aging and in longevity.
1 Introduction
Autophagy, the process of sequestration of damaged macromolecules and organelles, culminates with cargo being degraded in lysosomes. Based on the specificity of cargo selection and the mechanism of cargo delivery to the lysosome, the process has been sorted into various forms of autophagy (reviewed by Kaushik and Cuervo (2012); Abdrakhmanov et al. (2020)). Macroautophagy requires the conjugation of members of the ATG8 family, ubiquitin-like proteins including LC3s and GABARAPs, to phosphatidylethanolamine (PE) (Ichimura et al., 2000). This enables double-membrane vesicles termed autophagosomes to recruit ATG8 proteins, which mediate loading and maturation of cargo (Johansen and Lamark, 2020). More recently, autophagy-independent functions of ATG8 proteins have been discovered (reviewed by Galluzzi and Green (2019); Nieto-Torres et al. (2021a)). Some of these functions involve unconventional conjugation of ATG8 proteins to phosphatidylserine (PS) in addition to that of PE and incorporation of ATG8-PE/PS into single-membrane vesicles, a process known as Conjugation of ATG8 to Single Membranes (CASM) (Durgan et al., 2021). Additionally, post translational modifications such as phosphorylation of LC3B/ATG8 on Thr50 regulates directionality of autophagosome movement toward the cell periphery in mammalian cells and neurons (Nieto-Torres et al., 2021b) which could potentially influence the fate of autophagosomes. Several recent studies have highlighted these additional roles of ATG8 proteins leading to alternative fates of their cargo in degradation and secretion, together referred to as non-canonical autophagy (NCA) (reviewed by Codogno et al., 2011; Nieto-Torres et al., 2021a).
2 Forms of non-canonical autophagy
Although autophagy has always been accepted as a degradative process, not all cargoes from NCA culminate with lysosomal degradation. Owing to the alternative fates of cargoes, NCA can be either degradative or secretory (Figure 1).
2.1 Degradative autophagy
2.1.1 LC3-associated phagocytosis
Combining the forces of phagocytosis with LC3 recruitment, LC3-associated phagocytosis (LAP) enhances the fusion of LC3-associated phagosomes (LAPosomes) with lysosomes to increase degradation and elimination of LAPosome-contained pathogens (reviewed Heckmann and Green, 2019; Herb et al., 2020). This is different than a branch of canonical selective autophagy, termed xenophagy, which targets cytosolic pathogens and other foreign material for degradation (reviewed by Sharma et al. (2018)). Although the fundamental LC3 conjugation machinery consisting of ATG7, ATG3, and a complex of ATG16L1, ATG5, and ATG12 are shared between xenophagy and LAP, the LAP pathway differs in the mechanism of induction, membrane PI(3)P generation, requirement for reactive oxygen species (ROS), conjugation of LC3 to single membranes, and regulation of associated genes (reviewed in Heckmann and Green (2019)).
Unlike canonical autophagy, LAP starts with phagocytosis that is initiated via receptors on the cell surface such as pattern recognition, IgG, and dead cell receptors (Sanjuan et al., 2007; Martinez et al., 2011). LAP and autophagy share the components of the PI3K complex, Beclin1, VPS15, and VPS34, for membrane PI(3)P generation, however, LAP PI3K complexes additionally require UVRAG and Rubicon which are necessary for downstream events such as LC3 recruitment (Martinez et al., 2015). Prior to LC3 conjugation, LAP requires NOX2-mediated ROS generation at the phagosome membrane which regulates phagosomal pH and signals that recruit the LC3 conjugation machinery ((Martinez et al., 2015); reviewed by Heckmann and Green (2019)). Phagosomes are then decorated with LC3 via CASM which requires the WD40 C-terminal domain of ATG16L1, which is a domain that is dispensable for ATG16L1’s role in canonical autophagy (Fletcher et al., 2018). Unlike in autophagy, LC3 lipidation occurs after cargo is selected and the phagosome is sealed (Sanjuan et al., 2007; Martinez et al., 2015), suggesting LC3’s role in cargo selection is unlikely, but rather LC3’s role in LAP is predominantly in phagosome-lysosome fusion (Martinez et al., 2015). Subsequent lysosome fusion results in degradation of engulfed pathogens making LAP an important process in immune regulation in aging as discussed later.
2.1.2 Endosomal microautophagy
Endosomal microautophagy (eMI) was discovered as a pathway distinct from macroautophagy that delivers cytosolic proteins to late endosomes or multivesicular bodies (MVBs) by a microautophagy-like process ((Sahu et al., 2011); reviewed by Schuck (2020)). eMI is a variant of general microautophagy that does not require the core autophagic machinery but instead depends on the Endosomal Sorting Complex Required for Transport (ESCRT). However, the recruitment of ESCRT is unlike during MVB synthesis (reviewed by Hurley (2008)). eMI is induced upon acute amino acid starvation resulting in rapid degradation independent of the nutrient sensor and classical autophagy regulator, MTOR (Mejlvang et al., 2018). This response was found to be immediate, setting in prior to macroautophagy, with substrates including LC3B, GABARAPL2, and autophagy receptors (Mejlvang et al., 2018). At fly synapses, protein turnover occurs by eMI facilitated by chaperone HSC70-4-dependent membrane deformation while the co-chaperone SGT inhibits microautophagy (Uytterhoeven et al., 2015). HSC70 recognizes synaptic proteins with KFERQ motifs and binds endosomes via membrane PS (Sahu et al., 2011). This recognition is distinct from KFERQ recognition during chaperone-mediated autophagy, which involves recognition and import of unfolded proteins into lysosomes (reviewed by Kaushik and Cuervo (2018)).
2.2 Secretory autophagy
2.2.1 LDELS
LDELS (LC3-dependent extracellular vesicle loading and secretion) is a form of “secretory autophagy” (SA) that requires the LC3 conjugation machinery for loading cargoes into vesicles which are ultimately released extracellularly. Proximity labeling and extracellular vesicles (EV) proteomics revealed several RNA binding proteins to be the main cargoes of this pathway that also impacts extracellular secretion of non-coding RNAs (ncRNA) (Eng et al., 2021) and small nucleolar RNAs (snoRNA) (Leidal et al., 2020). This highlights a previously unclear role of LC3 in loading cargo into secreted EVs. Yet, how this pathway crosstalks with classical degradative autophagy is still being elucidated. Inhibition of autophagosome maturation, autophagosome-lysosome fusion, or lysosomal acidification each upregulated SA dependent on several ATG proteins and the small GTPase Rab27a. Such EV- and particle-mediated SA facilitates release of autophagic cargo receptors, buffering against their accumulation when classical autophagy is inhibited (Solvik et al., 2022). This highlights an interesting alternative route for maintaining proteostasis by secretory autophagy when autophagosome maturation and lysosome function are impaired.
2.2.2 Unconventional secretion
Secreted proteins usually carry a leader peptide sequence which sorts them to the trans-Golgi network to vesicles destined for the plasma membrane (reviewed by Viotti (2016)). However, proteins with and without leader sequences have been found to bypass the Golgi apparatus to be secreted by pathways together known as unconventional protein secretion (UPS) [reviewed (Ponpuak et al., 2015; Balmer and Faso, 2021)]. Of these UPS pathways, unconventional secretion constitutes sequestration of leaderless proteins into autophagosomes and secretion either by direct binding of the autophagosome with the plasma membrane or by autophagosome fusion with a multivesicular body (MVB) to form an amphisome followed by its fusion with the plasma membrane (Dupont et al., 2011; Zhang et al., 2015). Such secretory autophagosome formation is thought to be facilitated by compartments of UPS (CUPS) in yeast and a yet uncharacterized equivalent in mammalian cells along with Golgi assembly stacking protein (GRASP), ESCRT proteins for MVB formation and sorting, and SNAREs for vesicular fusion (Duran et al., 2010; Manjithaya et al., 2010). Cargo selection, although yet unclear, is thought to involve Vps23, found at CUPS in yeast (Bruns et al., 2011). Cargoes of unconventional secretion include many cytosolic proteins such as IL-1β, IL-18, galectin, tubulin, organellar content, and aggregation-prone proteins (Schweers et al., 2007; Dupont et al., 2011; Ejlerskov et al., 2013; Nilsson et al., 2013; Pallet et al., 2013; Ohman et al., 2014) making UPS a protective pathway to prevent intracellular accumulation, but could also potentially be an important influencer of inflammation.
2.3 Recycling autophagy
2.3.1 LANDO
LC3-associated endocytosis (LANDO) begins with recognition of cargo by cell surface receptors like Toll-Like Receptors (TLR) and TREM2 followed by clathrin-mediated endosome internalization. The machinery for the formation of the PI3K complex and LC3 recruitment to the single membrane LANDOsome is similar to that of LAP (Heckmann et al., 2019; Heckmann et al., 2020), but unlike LAP, LANDO has multiple endpoints; LANDOsome fusion with the lysosome followed by ligand degradation and recycling of the cell surface receptors back to the plasma membrane ((Heckmann et al., 2019); reviewed in Pena-Martinez et al. (2022)). The protection offered by LANDO-mediated receptor recycling in microglia in neurodegeneration is discussed in the next section.
3 Non-canonical autophagy in aging and age-related diseases
Aging is the number one risk factor for many diseases, and with age, there is a general decrease in efficiency of degradative autophagy, both canonical and NCA (Finkbeiner, 2020; Krause et al., 2022) (Figure 1). Additionally, in what is likely a response to age-associated decreased degradation through the lysosome is the shift to SA (Krause et al., 2022); however, owing to the overlap of the initial steps of autophagosome formation, SA also decreases with age (Gonzalez et al., 2020). Understanding the mechanisms that differentially initiate and regulate NCA will help identify how defects in these pathways contribute to aging and disease.
One of the defining hallmarks of aging is altered intercellular communication, with a prominent example being “inflammaging”, or the chronic inflammation that further amplifies the aging process (López-Otín et al., 2013). Growing evidence identifies inflammaging as the driver for NCA in aged microglia. SA has been shown to maintain proteostasis when autophagy is inhibited by blocking fusion with the lysosome in vitro (Solvik et al., 2022). However, the downstream effect of this is the release of cargo into the extracellular space, and, depending on what was targeted for degradation but is now in the extracellular space, can itself induce an immune response (Tan et al., 2022). Hyperactivation of macrophages will lead to increased phagocytosis of the discarded cargo, bringing it back into the cell to attempt to be cleared by LAP or LANDO. However, if the limitation is at the lysosome, the effort is futile and will lead to deposition of aggregated proteins both intracellularly and in the extracellular space. Thus, chronic inflammation seen with aging is a likely driver for aggregation-associated diseases, including many neurodegenerative diseases (Figure 2).
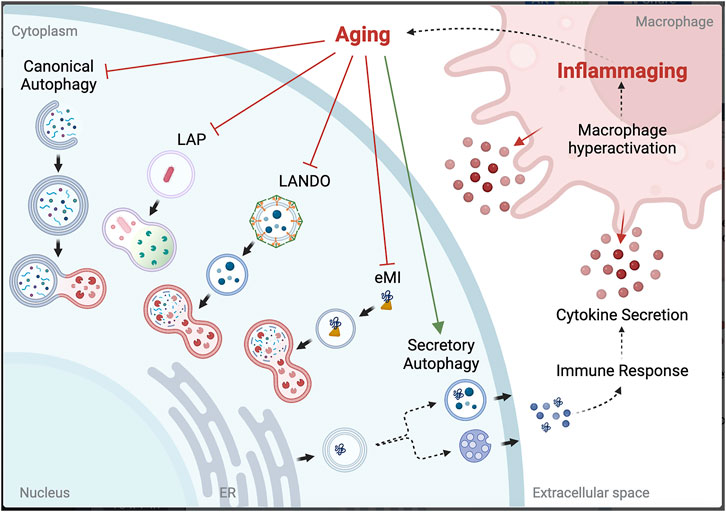
FIGURE 2. Potential link between autophagy dysfunction and inflammaging. Created with BioRender.com.
Cellular senescence is also a hallmark of aging that NCA may have a role in perpetuating. P53-regulated activation of the production and release of exosomes containing miRNA and protein cargoes from senescent cells could worsen senescence-associated secretory phenotypes (SASPs), ultimately resulting in LDELS-driven chronic inflammation (Xu and Tahara, 2013). When investigating NCA pathways in age-associated diseases and potential therapeutic targets, the roles of SA, LDELS, LAP, and LANDO must all be considered.
3.1 Alzheimer’s disease
A uniting characteristic of adult-onset neurodegenerative diseases is the abnormal deposition of misfolded aggregated proteins. The age-associated down-regulation of autophagy in the brain suggests autophagy dysfunction is a common mechanism in neurodegenerative disease (Lipinski et al., 2010; Friedman et al., 2012). Alzheimer’s Disease (AD) is caused by neuronal death associated with amyloid beta (Aβ) and tau tangle deposition in the brain. Exploration of canonical autophagy as a therapeutic target for AD has been extensively investigated (reviewed in Guo et al. (2018)), with the targeting of NCA just beginning to surface. Evidence to suggest targeting NCA as a therapeutic is supported by reports that several components that regulate the machinery for LANDO were found to be downregulated in mixed sex and age cohorts of human AD brains compared to matched controls (Heckmann et al., 2020). Experimentally, aged mice (two-years old) lacking the WD domain of ATG16L (specifically required for NCA) showed spontaneous deposits of endogenous Aβ, increased microglial inflammation, and neuronal death in their hippocampi (Heckmann et al., 2020). Further, loss of LANDO leads to a defect in returning the Aβ receptors to the cell surface (Heckmann et al., 2019), so LANDO protects against neuronal loss by improving Aβ clearance in mouse models of AD, owing to the efficient recycling of receptors for Aβ in microglia, including TREM2. This brings to question if inhibition of canonical autophagy to promote LANDO or LDELS would be an appropriate method to consider as an AD therapeutic (Limone et al., 2022).
Further evidence to target NCA for AD therapeutics focuses on LDELS and the role of EV secretion. With age, EV secretion decreases due to the disruption of the endosomal/lysosomal trafficking pathway involved in Aβ metabolism. In a non-human primate study, the contribution to age-associated intraneuronal accumulation of Aβ was partially due to Aβ build-up in EVs. Intraneuronal accumulation of Aβ precedes extracellular Aβ depositions, and the experimental downregulation of autophagosome formation enhanced EV secretion to ameliorate intracellular Aβ accumulation, although there was no success in clearing the extracellular Aβ. Understanding the spatiotemporal transition from intracellular to extracellular depositions may delineate the connection to the age-associated decrease of autophagy-related protein levels that precedes Aβ deposition (Koinuma et al., 2021).
3.2 Parkinson’s disease
Parkinson’s Disease (PD) is characterized by neuronal death associated with α-synuclein deposits in the brain. The age-associated loss of autophagy in neurons does not drastically affect the total amount of soluble α-synuclein, suggesting the proteasome is the preferred degradative pathway for α-synuclein, and autophagy would only be activated to clear the aggregated α-synuclein (Ebrahimi-Fakhari et al., 2011). This suggests that autophagy of α-synuclein in neurons does not greatly contribute to the degradation of the protein until it becomes pathological (α-synuclein structure changes or aggregation) and overloads the system (Choi et al., 2022).
The investigation of the specific role of NCA in PD is very undeveloped, although there have been clues to the involvement of SA historically. For instance, it has been demonstrated that α-synuclein is secreted from neurons in PD models (Kim et al., 2013), and that this secretion is a driver of the disease because of the impact it has on neighboring cells. This secretion may be a response to decreased degradative autophagy (Cuervo et al., 2004) by switching to SA to maintain proteostasis in neurons, similar to the mitochondrial SA in cardiomyocytes shown by Huang et al. (2018). Disruption of the canonical autophagic pathway seems to drive SA, indicated by TPPP-p25α’s α-synuclein aggregation properties that also prevents maturation of autophagosomes into autolysosomes by limiting mobility (Ejlerskov et al., 2013). This begs the question if canonical autophagy and SA coexist or if SA is meant as a last resort protective response to the loss of the lysosomal degradation pathway.
3.3 Infection and immunity
Responding to infections and inducing an immune response is heavily supported by efficient killing and clearance of pathogens and directing proinflammatory responses by LAP and LANDO in systemic macrophages and dendritic cells in the brain (Heckmann et al., 2017). LC3 recruitment to phagosomes enhances antigen presentation by MHC class II molecules, and the failure of fungal antigen presentation by MHC class II molecules was seen in both mouse and human macrophages when LAP was inhibited (Ma et al., 2012; Romao et al., 2013; Jülg et al., 2020). This could explain why the decreased efficiency of LAP seen with age could make the elderly more susceptible to infectious diseases (Inomata et al., 2020).
Control of the inflammatory response after an infection is as important as modulating an immune response, and NCA has a suggested role in this control. Mitochondria are found to be cleared independently of lysosomal degradation in HeLa cells harboring knockouts of the ATG8 conjugation machinery (ATG7, ATG5, and ATG3). The SA pathway clears mitochondria via their extracellular release by a process defined as Autophagic Secretion of Mitochondria along with concurrent increased pro-inflammatory cytokine release from recipient cells (Tan et al., 2022). This study highlights the role of ATG8 lipidation in suppressing inflammatory responses by preventing inflammation-inducing SA of mitochondria.
3.4 Cancer
Age is an associated risk factor for many cancers as well, and autophagy in established tumor cells and the supporting cells in the tumor microenvironment is often hyperactivated to support the increased metabolic demand (Sousa et al., 2016; Kimmelman and White, 2017). Therefore, it is not surprising that we are beginning to discover that tumor and associated cells have also adapted the use of the NCA pathways to support tumor growth and progression. For instance, LAP in macrophages that clears dead cancer cells actually helps to suppress an inflammatory response against tumor cells, leading to immune tolerance of these mutant cells (Asare et al., 2020).
However, inhibition of canonical autophagy as a therapeutic must consider the downstream effects of a broad inhibition. As mentioned above, inhibition of autophagy tends to drive NCA. This is particularly detrimental when activating SA, since increased SA is associated with increased cancer proliferation (Gonzalez et al., 2020). While much more work must go into this investigation, in theory, inhibiting canonical autophagy would push the cancer cell to increase exosome release of dangerous ncRNA that perpetuates tumor progression through the uptake of oncogenic exosomes by neighboring cells (Eng et al., 2021). Similar effects could be seen when expelled unhealthy mitochondria are taken up by recipient cells (Tan et al., 2022). With evidence that suppression of LAP has anti-tumor effects (Cunha et al., 2018) and the risk of driving oncogenesis via SA, NCA may become a more attractive cancer therapeutic target than canonical autophagy.
4 Conclusion and future prospects
The role of NCA in aging and age-related diseases is still under intense investigation. To name a few, preliminary studies have defined roles for LANDO and SA in neurodegenerative diseases, LAP, LANDO, and SA in infection and immune responses, and LAP and SA in cancer, but many questions remain to be answered. It is still not clear how cargo is recruited for NCA, whether NCA and canonical autophagy coexist, if differential signals direct the decision to complete canonical versus NCA, and whether the cell has a preference for either type. Alternatively, NCA may only be initiated when canonical autophagy cannot meet cellular requirements, and thus becomes the dominant response for cargo clearance. Furthermore, the molecular pathways and vesicular trafficking in SA are not fully described, but canonical autophagy machinery is required for the initiation. So, if the same machinery is needed, but there are different outcomes, what determines if degradation occurs in the lysosome or if SA is induced? Moreover, with so many pathways to deliver cargo to the lysosomes for degradation, does everything come down to functional lysosomes? This seems to be the case, since the switch from degradation to SA does not solve the overall problem in neurodegenerative diseases, but instead seems to exacerbate the pathology by inducing a vicious cycle that propagates inflammation (Solvik et al., 2022; Tan et al., 2022). Finally, the most important question is how we can harness NCA to use for prevention, prognosis, or therapeutics for aging and age-associated diseases.
Author contributions
AK and JM wrote and edited the manuscript together. AK designed and created the figures.
Conflict of interest
The authors declare that the research was conducted in the absence of any commercial or financial relationships that could be construed as a potential conflict of interest.
Publisher’s note
All claims expressed in this article are solely those of the authors and do not necessarily represent those of their affiliated organizations, or those of the publisher, the editors and the reviewers. Any product that may be evaluated in this article, or claim that may be made by its manufacturer, is not guaranteed or endorsed by the publisher.
References
Abdrakhmanov, A., Gogvadze, V., and Zhivotovsky, B. (2020). To eat or to die: Deciphering selective forms of autophagy. Trends Biochem. Sci. 45, 347–364. doi:10.1016/j.tibs.2019.11.006
Asare, P. F., Roscioli, E., Hurtado, P. R., Tran, H. B., Mah, C. Y., and Hodge, S. (2020). LC3-Associated phagocytosis (LAP): A potentially influential mediator of efferocytosis-related tumor progression and aggressiveness. Front. Oncol. 10, 1298. doi:10.3389/fonc.2020.01298
Balmer, E. A., and Faso, C. (2021). The road less traveled? Unconventional protein secretion at parasite-host interfaces. Front. Cell Dev. Biol. 9, 662711. doi:10.3389/fcell.2021.662711
Bruns, C., Mccaffery, J. M., Curwin, A. J., Duran, J. M., and Malhotra, V. (2011). Biogenesis of a novel compartment for autophagosome-mediated unconventional protein secretion. J. Cell Biol. 195, 979–992. doi:10.1083/jcb.201106098
Choi, I., Heaton, G. R., Lee, Y.-K., and Yue, Z. (2022). Regulation of α-synuclein homeostasis and inflammasome activation by microglial autophagy. Sci. Adv. 8, eabn1298. doi:10.1126/sciadv.abn1298
Codogno, P., Mehrpour, M., and Proikas-Cezanne, T. (2011). Canonical and non-canonical autophagy: Variations on a common theme of self-eating? Nat. Rev. Mol. Cell Biol. 13, 7–12. doi:10.1038/nrm3249
Cuervo, A. M., Stefanis, L., Fredenburg, R., Lansbury, P. T., and Sulzer, D. (2004). Impaired degradation of mutant alpha-synuclein by chaperone-mediated autophagy. Science 305, 1292–1295. doi:10.1126/science.1101738
Cunha, L. D., Yang, M., Carter, R., Guy, C., Harris, L., Crawford, J. C., et al. (2018). LC3-Associated phagocytosis in myeloid cells promotes tumor immune tolerance. Cell 175, 429–441.e16. doi:10.1016/j.cell.2018.08.061
Dupont, N., Jiang, S., Pilli, M., Ornatowski, W., Bhattacharya, D., and Deretic, V. (2011). Autophagy-based unconventional secretory pathway for extracellular delivery of IL-1β. EMBO J. 30, 4701–4711. doi:10.1038/emboj.2011.398
Duran, J. M., Anjard, C., Stefan, C., Loomis, W. F., and Malhotra, V. (2010). Unconventional secretion of Acb1 is mediated by autophagosomes. J. Cell Biol. 188, 527–536. doi:10.1083/jcb.200911154
Durgan, J., Lystad, A. H., Sloan, K., Carlsson, S. R., Wilson, M. I., Marcassa, E., et al. (2021). Non-canonical autophagy drives alternative ATG8 conjugation to phosphatidylserine. Mol. Cell 81, 2031–2040.e8. doi:10.1016/j.molcel.2021.03.020
Ebrahimi-Fakhari, D., Cantuti-Castelvetri, I., Fan, Z., Rockenstein, E., Masliah, E., Hyman, B. T., et al. (2011). Distinct roles in vivo for the ubiquitin-proteasome system and the autophagy-lysosomal pathway in the degradation of α-synuclein. J. Neurosci. 31, 14508–14520. doi:10.1523/JNEUROSCI.1560-11.2011
Ejlerskov, P., Rasmussen, I., Nielsen, T. T., Bergstrom, A. L., Tohyama, Y., Jensen, P. H., et al. (2013). Tubulin polymerization-promoting protein (TPPP/p25α) promotes unconventional secretion of α-synuclein through exophagy by impairing autophagosome-lysosome fusion. J. Biol. Chem. 288, 17313–17335. doi:10.1074/jbc.M112.401174
Eng, G. W. L., Zheng, Y., Yap, D. W. T., Teo, A. Y. T., and Cheong, J. K. (2021). Autophagy and ncRNAs: Dangerous liaisons in the crosstalk between the tumor and its microenvironment. Cancers 14, 20. doi:10.3390/cancers14010020
Finkbeiner, S. (2020). The autophagy lysosomal pathway and neurodegeneration. Cold Spring Harb. Perspect. Biol. 12, a033993. doi:10.1101/cshperspect.a033993
Fletcher, K., Ulferts, R., Jacquin, E., Veith, T., Gammoh, N., Arasteh, J. M., et al. (2018). The WD40 domain of ATG16L1 is required for its non-canonical role in lipidation of LC3 at single membranes. EMBO J. 37, e97840. doi:10.15252/embj.201797840
Friedman, L. G., Lachenmayer, M. L., Wang, J., He, L., Poulose, S. M., Komatsu, M., et al. (2012). Disrupted autophagy leads to dopaminergic axon and dendrite degeneration and promotes presynaptic accumulation of α-synuclein and LRRK2 in the brain. J. Neurosci. 32, 7585–7593. doi:10.1523/JNEUROSCI.5809-11.2012
Galluzzi, L., and Green, D. R. (2019). Autophagy-independent functions of the autophagy machinery. Cell 177, 1682–1699. doi:10.1016/j.cell.2019.05.026
Gonzalez, C. D., Resnik, R., and Vaccaro, M. I. (2020). Secretory autophagy and its relevance in metabolic and degenerative disease. Front. Endocrinol. 11, 266. doi:10.3389/fendo.2020.00266
Guo, F., Liu, X., Cai, H., and Le, W. (2018). Autophagy in neurodegenerative diseases: Pathogenesis and therapy. Brain Pathol. 28, 3–13. doi:10.1111/bpa.12545
Heckmann, B. L., Boada-Romero, E., Cunha, L. D., Magne, J., and Green, D. R. (2017). LC3-Associated phagocytosis and inflammation. J. Mol. Biol. 429, 3561–3576. doi:10.1016/j.jmb.2017.08.012
Heckmann, B. L., and Green, D. R. (2019). LC3-associated phagocytosis at a glance. J. Cell Sci. 132, jcs222984. doi:10.1242/jcs.222984
Heckmann, B. L., Teubner, B. J. W., Boada-Romero, E., Tummers, B., Guy, C., Fitzgerald, P., et al. (2020). Noncanonical function of an autophagy protein prevents spontaneous Alzheimer's disease. Sci. Adv. 6, eabb9036. doi:10.1126/sciadv.abb9036
Heckmann, B. L., Teubner, B. J. W., Tummers, B., Boada-Romero, E., Harris, L., Yang, M., et al. (2019). LC3-Associated endocytosis facilitates beta-amyloid clearance and mitigates neurodegeneration in murine alzheimer's disease. Cell 178, 536–551.e14. doi:10.1016/j.cell.2019.05.056
Herb, M., Gluschko, A., and Schramm, M. (2020). LC3-associated phagocytosis - the highway to hell for phagocytosed microbes. Semin. Cell Dev. Biol. 101, 68–76. doi:10.1016/j.semcdb.2019.04.016
Huang, C.-Y., Kuo, W.-W., Ho, T.-J., Chiang, S.-F., Pai, P.-Y., Lin, J.-Y., et al. (2018). Rab9-dependent autophagy is required for the IGF-IIR triggering mitophagy to eliminate damaged mitochondria. J. Cell. Physiology 233, 7080–7091. doi:10.1002/jcp.26346
Hurley, J. H. (2008). ESCRT complexes and the biogenesis of multivesicular bodies. Curr. Opin. Cell Biol. 20, 4–11. doi:10.1016/j.ceb.2007.12.002
Ichimura, Y., Kirisako, T., Takao, T., Satomi, Y., Shimonishi, Y., Ishihara, N., et al. (2000). A ubiquitin-like system mediates protein lipidation. Nature 408, 488–492. doi:10.1038/35044114
Inomata, M., Xu, S., Chandra, P., Meydani, S. N., Takemura, G., Philips, J. A., et al. (2020). Macrophage LC3-associated phagocytosis is an immune defense against Streptococcus pneumoniae that diminishes with host aging. Proc. Natl. Acad. Sci. U. S. A. 117, 33561–33569. doi:10.1073/pnas.2015368117
Johansen, T., and Lamark, T. (2020). Selective autophagy: ATG8 family proteins, LIR motifs and cargo receptors. J. Mol. Biol. 432, 80–103. doi:10.1016/j.jmb.2019.07.016
Jülg, J., Strohm, L., and Behrends, C. (2020). Canonical and non-canonical autophagy pathways in microglia. Mol. Cell. Biol. 41, e00389-20. doi:10.1128/MCB.00389-20
Kaushik, S., and Cuervo, A. M. (2012). Chaperone-mediated autophagy: A unique way to enter the lysosome world. Trends Cell Biol. 22, 407–417. doi:10.1016/j.tcb.2012.05.006
Kaushik, S., and Cuervo, A. M. (2018). The coming of age of chaperone-mediated autophagy. Nat. Rev. Mol. Cell Biol. 19, 365–381. doi:10.1038/s41580-018-0001-6
Kim, C., Ho, D. H., Suk, J. E., You, S., Michael, S., Kang, J., et al. (2013). Neuron-released oligomeric α-synuclein is an endogenous agonist of TLR2 for paracrine activation of microglia. Nat. Commun. 4, 1562. doi:10.1038/ncomms2534
Kimmelman, A. C., and White, E. (2017). Autophagy and tumor metabolism. Cell Metab. 25, 1037–1043. doi:10.1016/j.cmet.2017.04.004
Koinuma, S., Shimozawa, N., Yasutomi, Y., and Kimura, N. (2021). Aging induces abnormal accumulation of Aβ in extracellular vesicle and/or intraluminal membrane vesicle-rich fractions in nonhuman primate brain. Neurobiol. Aging 106, 268–281. doi:10.1016/j.neurobiolaging.2021.06.022
Krause, G. J., Diaz, A., Jafari, M., Khawaja, R. R., Agullo-Pascual, E., Santiago-Fernández, O., et al. (2022). Reduced endosomal microautophagy activity in aging associates with enhanced exocyst-mediated protein secretion. Aging Cell 21, e13713. doi:10.1111/acel.13713
Leidal, A. M., Huang, H. H., Marsh, T., Solvik, T., Zhang, D., Ye, J., et al. (2020). The LC3-conjugation machinery specifies the loading of RNA-binding proteins into extracellular vesicles. Nat. Cell Biol. 22, 187–199. doi:10.1038/s41556-019-0450-y
Limone, A., Veneruso, I., Izzo, A., Renna, M., Bonavita, R., Piscitelli, S., et al. (2022). Activation of non-canonical autophagic pathway through inhibition of non-integrin laminin receptor in neuronal cells. Cells, 11, 466. doi:10.3390/cells11030466
Lipinski, M. M., Hoffman, G., Ng, A., Zhou, W., Py, B. F., Hsu, E., et al. (2010). A genome-wide siRNA screen reveals multiple mTORC1 independent signaling pathways regulating autophagy under normal nutritional conditions. Dev. Cell 18, 1041–1052. doi:10.1016/j.devcel.2010.05.005
López-Otín, C., Blasco, M. A., Partridge, L., Serrano, M., and Kroemer, G. (2013). The hallmarks of aging. Cell 153, 1194–1217. doi:10.1016/j.cell.2013.05.039
Ma, J., Becker, C., Lowell, C. A., and Underhill, D. M. (2012). Dectin-1-triggered recruitment of light chain 3 protein to phagosomes facilitates major histocompatibility complex class II presentation of fungal-derived antigens. J. Biol. Chem. 287, 34149–34156. doi:10.1074/jbc.M112.382812
Manjithaya, R., Anjard, C., Loomis, W. F., and Subramani, S. (2010). Unconventional secretion of Pichia pastoris Acb1 is dependent on GRASP protein, peroxisomal functions, and autophagosome formation. J. Cell Biol. 188, 537–546. doi:10.1083/jcb.200911149
Martinez, J., Almendinger, J., Oberst, A., Ness, R., Dillon, C. P., Fitzgerald, P., et al. (2011). Microtubule-associated protein 1 light chain 3 alpha (LC3)-associated phagocytosis is required for the efficient clearance of dead cells. Proc. Natl. Acad. Sci. U. S. A. 108, 17396–17401. doi:10.1073/pnas.1113421108
Martinez, J., Malireddi, R. K., Lu, Q., Cunha, L. D., Pelletier, S., Gingras, S., et al. (2015). Molecular characterization of LC3-associated phagocytosis reveals distinct roles for Rubicon, NOX2 and autophagy proteins. Nat. Cell Biol. 17, 893–906. doi:10.1038/ncb3192
Mejlvang, J., Olsvik, H., Svenning, S., Bruun, J. A., Abudu, Y. P., Larsen, K. B., et al. (2018). Starvation induces rapid degradation of selective autophagy receptors by endosomal microautophagy. J. Cell Biol. 217, 3640–3655. doi:10.1083/jcb.201711002
Nieto-Torres, J. L., Leidal, A. M., Debnath, J., and Hansen, M. (2021a). Beyond autophagy: The expanding roles of ATG8 proteins. Trends Biochem. Sci. 46, 673–686. doi:10.1016/j.tibs.2021.01.004
Nieto-Torres, J. L., Shanahan, S. L., Chassefeyre, R., Chaiamarit, T., Zaretski, S., Landeras-Bueno, S., et al. (2021b). LC3B phosphorylation regulates FYCO1 binding and directional transport of autophagosomes. Curr. Biol. 31, 3440–3449.e7. doi:10.1016/j.cub.2021.05.052
Nilsson, P., Loganathan, K., Sekiguchi, M., Matsuba, Y., Hui, K., Tsubuki, S., et al. (2013). Aβ secretion and plaque formation depend on autophagy. Cell Rep. 5, 61–69. doi:10.1016/j.celrep.2013.08.042
Ohman, T., Teirila, L., Lahesmaa-Korpinen, A. M., Cypryk, W., Veckman, V., Saijo, S., et al. (2014). Dectin-1 pathway activates robust autophagy-dependent unconventional protein secretion in human macrophages. J. Immunol. 192, 5952–5962. doi:10.4049/jimmunol.1303213
Pallet, N., Sirois, I., Bell, C., Hanafi, L. A., Hamelin, K., Dieude, M., et al. (2013). A comprehensive characterization of membrane vesicles released by autophagic human endothelial cells. Proteomics 13, 1108–1120. doi:10.1002/pmic.201200531
Pena-Martinez, C., Rickman, A. D., and Heckmann, B. L. (2022). Beyond autophagy: LC3-associated phagocytosis and endocytosis. Sci. Adv. 8, eabn1702. doi:10.1126/sciadv.abn1702
Ponpuak, M., Mandell, M. A., Kimura, T., Chauhan, S., Cleyrat, C., and Deretic, V. (2015). Secretory autophagy. Curr. Opin. Cell Biol. 35, 106–116. doi:10.1016/j.ceb.2015.04.016
Romao, S., Gasser, N., Becker, A. C., Guhl, B., Bajagic, M., Vanoaica, D., et al. (2013). Autophagy proteins stabilize pathogen-containing phagosomes for prolonged MHC II antigen processing. J. Cell Biol. 203, 757–766. doi:10.1083/jcb.201308173
Sahu, R., Kaushik, S., Clement, C. C., Cannizzo, E. S., Scharf, B., Follenzi, A., et al. (2011). Microautophagy of cytosolic proteins by late endosomes. Dev. Cell 20, 131–139. doi:10.1016/j.devcel.2010.12.003
Sanjuan, M. A., Dillon, C. P., Tait, S. W., Moshiach, S., Dorsey, F., Connell, S., et al. (2007). Toll-like receptor signalling in macrophages links the autophagy pathway to phagocytosis. Nature 450, 1253–1257. doi:10.1038/nature06421
Schuck, S. (2020). Microautophagy - distinct molecular mechanisms handle cargoes of many sizes. J. Cell Sci. 133, jcs246322. doi:10.1242/jcs.246322
Schweers, R. L., Zhang, J., Randall, M. S., Loyd, M. R., Li, W., Dorsey, F. C., et al. (2007). NIX is required for programmed mitochondrial clearance during reticulocyte maturation. Proc. Natl. Acad. Sci. U. S. A. 104, 19500–19505. doi:10.1073/pnas.0708818104
Sharma, V., Verma, S., Seranova, E., Sarkar, S., and Kumar, D. (2018). Selective autophagy and xenophagy in infection and disease. Front. Cell Dev. Biol. 6, 147. doi:10.3389/fcell.2018.00147
Solvik, T. A., Nguyen, T. A., Tony Lin, Y. H., Marsh, T., Huang, E. J., Wiita, A. P., et al. (2022). Secretory autophagy maintains proteostasis upon lysosome inhibition. J. Cell Biol. 221, e202110151. doi:10.1083/jcb.202110151
Sousa, C. M., Biancur, D. E., Wang, X., Halbrook, C. J., Sherman, M. H., Zhang, L., et al. (2016). Pancreatic stellate cells support tumour metabolism through autophagic alanine secretion. Nature 536, 479–483. doi:10.1038/nature19084
Tan, H. W. S., Lu, G., Dong, H., Cho, Y.-L., Natalia, A., Wang, L., et al. (2022). A degradative to secretory autophagy switch mediates mitochondria clearance in the absence of the mATG8-conjugation machinery. Nat. Commun. 13, 3720. doi:10.1038/s41467-022-31213-7
Uytterhoeven, V., Lauwers, E., Maes, I., Miskiewicz, K., Melo, M. N., Swerts, J., et al. (2015). Hsc70-4 deforms membranes to promote synaptic protein turnover by endosomal microautophagy. Neuron 88, 735–748. doi:10.1016/j.neuron.2015.10.012
Viotti, C. (2016). ER to golgi-dependent protein secretion: The conventional pathway. Methods Mol. Biol. 1459, 3–29. doi:10.1007/978-1-4939-3804-9_1
Xu, D., and Tahara, H. (2013). The role of exosomes and microRNAs in senescence and aging. Adv. Drug Deliv. Rev. 65, 368–375. doi:10.1016/j.addr.2012.07.010
Keywords: non-canonical autophagy, aging, neurodegenerative diseasaes, secretory autophagy, LAP (LC3 associated phagocytosis), LANDO
Citation: Kumar AV and Mills J (2023) Non-canonical autophagy in aging and age-related diseases. Front. Cell Dev. Biol. 11:1137870. doi: 10.3389/fcell.2023.1137870
Received: 04 January 2023; Accepted: 13 February 2023;
Published: 23 February 2023.
Edited by:
Fu-Hui Xiao, Kunming Institute of Zoology, Chinese Academy of Sciences (CAS), ChinaReviewed by:
James Harper, Sam Houston State University, United StatesGhita Ghislat, Imperial College London, United Kingdom
Copyright © 2023 Kumar and Mills. This is an open-access article distributed under the terms of the Creative Commons Attribution License (CC BY). The use, distribution or reproduction in other forums is permitted, provided the original author(s) and the copyright owner(s) are credited and that the original publication in this journal is cited, in accordance with accepted academic practice. No use, distribution or reproduction is permitted which does not comply with these terms.
*Correspondence: Anita V. Kumar, anitavk17@gmail.com; Joslyn Mills, Mills_Joslyn@wheatoncollege.edu
†These authors have contributed equally to this work and share first authorship
‡Present Address: Anita V. Kumar, Core Biology, JoVE, Navi Mumbai, India