Wharton’s jelly mesenchymal stem cells: a concise review of their secretome and prospective clinical applications
- 1Human Genetics Unit, Department of Pathology, College of Medicine, Kuwait University, Jabriya, Kuwait
- 2Animal and Imaging Core Facilities, Dasman Diabetes Institute, Dasman, Kuwait
- 3Department of Immunology and Microbiology, Dasman Diabetes Institute, Dasman, Kuwait
- 4Department of Genetics and Bioinformatics, Dasman Diabetes Institute, Dasman, Kuwait
Accumulating evidence indicates that most primary Wharton’s jelly mesenchymal stem cells (WJ-MSCs) therapeutic potential is due to their paracrine activity, i.e., their ability to modulate their microenvironment by releasing bioactive molecules and factors collectively known as secretome. These bioactive molecules and factors can either be released directly into the surrounding microenvironment or can be embedded within the membrane-bound extracellular bioactive nano-sized (usually 30–150 nm) messenger particles or vesicles of endosomal origin with specific route of biogenesis, known as exosomes or carried by relatively larger particles (100 nm–1 μm) formed by outward blebbing of plasma membrane called microvesicles (MVs); exosomes and MVs are collectively known as extracellular vesicles (EVs). The bioactive molecules and factors found in secretome are of various types, including cytokines, chemokines, cytoskeletal proteins, integrins, growth factors, angiogenic mediators, hormones, metabolites, and regulatory nucleic acid molecules. As expected, the secretome performs different biological functions, such as immunomodulation, tissue replenishment, cellular homeostasis, besides possessing anti-inflammatory and anti-fibrotic effects. This review highlights the current advances in research on the WJ-MSCs’ secretome and its prospective clinical applications.
1 Introduction
In recent years, the biological and clinical interest in mesenchymal stem cells (MSCs) has grown remarkably due to their distinctive stemness characteristics. MSCs are multipotent non-hematopoietic cells that exhibit high degree of self-renewal, multi-lineage differentiation potential and immunomodulatory activity (Pittenger et al., 1999; Ali et al., 2015).
MSCs reside primarily in the bone marrow, where they were first characterized; nevertheless, they have a broad post-natal organ distribution (Friedenstein et al., 1970). MSCs have been isolated from different adult and fetal tissues (Uder et al., 2018). The adult tissues include adipose tissue, skeletal muscle, bone marrow, molar teeth/dental pulp, synovium/synovial fluid, skin, hematopoietic supportive stroma, and others (da Silva Meirelles et al., 2006). The fetal tissues include peripheral and umbilical cord blood, umbilical cord stroma or tissue, placenta, amniotic fluid, endometrium (da Silva Meirelles et al., 2006; Jiang et al., 2011). Although, MSCs share common characteristics including the expression of common cell surface markers (CD105, CD73 and CD90) and multipotency capacity to differentiate into osteoblasts, chondrocytes, or adipocytes (Carvalho et al., 2011; Ghaneialvar et al., 2018), they have different expression profiles and properties.
The unique properties of Wharton’s Jelly (WJ)-MSCs attracted the attention of scientific community as an alternative source of stem cells for regenerative medicine. Unlike embryonic stem cells, no ethical concerns are associated with WJ-MSCs clinical application. Remarkably, both cell types have comparable molecular signatures as depicted from genetic profiling studies (Hsieh et al., 2010). Worth mentioning, umbilical cord blood MSCs share similar characteristics to that of WJ-MSCs, however, they are less attractive for clinical application due to their low frequency, poor proliferation rate and culture limitations (Zeddou et al., 2010).
WJ-MSCs characteristics qualify them as a better alternative for clinical use since WJ-MSCs are isolated from the gelatinous layer of the umbilical cord tissue using a non-invasive and painless procedure. Moreover, the umbilical cord is deemed a medical waste eliminating ethical concerns for their use (Kim et al., 2013). Thus, the use of WJ-MSCs overcomes the clinical limitations associated with adult MSCs such as the invasive collection procedures and the availability of suitable cell donors (Ali et al., 2015). Because of the embryonic nature of WJ-MSCs, the expression of the pluripotency markers, NANOG, Oct 3/4 and Sox2, is higher than that of the adult MSCs (Nekanti et al., 2010; Higuchi et al., 2012), and also implies less exposure to environmental toxins and associated genetic modulation which, may in part, explain their superiority over the adult MSCs (Fong et al., 2011). In comparison to adult MSCs, WJ-MSCs have a higher proliferation rate, longevity, differentiation potential, immune-privilege, and lower immunogenicity properties (Kim et al., 2013). Together, these advantages enable the use of WJ-MSCs as therapeutic agents in regenerative medicine. Notably, several clinical trials have been established to investigate the safety and efficacy of treatment with allogeneic WJ-MSCs (Uder et al., 2018; Carlsson et al., 2023). Yet, there are critical issues including heterogenicity as depicted from single cell transcriptomic studies (Chen et al., 2023), lack of clinical longitudinal studies addressing the long-term safety and prospective adverse conditions such as potential tumorigenicity, profibrogenicity, which were reported using adult MSCs (Russo et al., 2006; Barkholt et al., 2013). Together, these complications may add some complexity to their clinical applications.
In general, it was initially believed that the therapeutic effects of transplanted MSCs were facilitated by the migration of the cells to sites of injury, where they integrated into the damaged tissue and differentiated into specialized cells. But only a small number of cells were detected to engraft and survive in the damaged host tissue. Therefore, it became evident that the transplanted MSCs do not necessarily need to come in proximity with the damaged tissue. A growing body of evidence supports that the therapeutic effects of MSCs occur largely through paracrine signaling of secretome (Fong et al., 2014), which is classified into soluble factors (growth factors, cytokines, chemokines, and enzymes) and extracellular vesicles (EVs) such as exosomes and microvesicles (MVs) that additionally contain lipids, proteins, RNA and DNA subtypes (Daneshmandi et al., 2020). Therefore, delineating the secretome components and properties may assist with improving the therapeutic potential of MSCs (Nooshabadi et al., 2018). In this review, we discuss the WJ-MSCs’ secretome components compared to the secretomes of other MSCs as well as the therapeutic applications of these cells and their secretome in different disease conditions.
2 WJ mesenchymal stem cell’s origin and isolation
During pregnancy, the umbilical cord forms a link between the mother and the fetus. From the outside, the umbilical cord is covered by a layer(s) of squamous-cubic epithelial cells, called umbilical epithelium (Can and Karahuseyinoglu, 2007; Wang et al., 2008). From the inside, the umbilical cord is composed of two arteries and one vein that are surrounded by a matrix of embryonic mucous connective tissue called WJ, which lies between the covering amniotic epithelium and the umbilical vessels (Figure 1) (Can and Karahuseyinoglu, 2007).
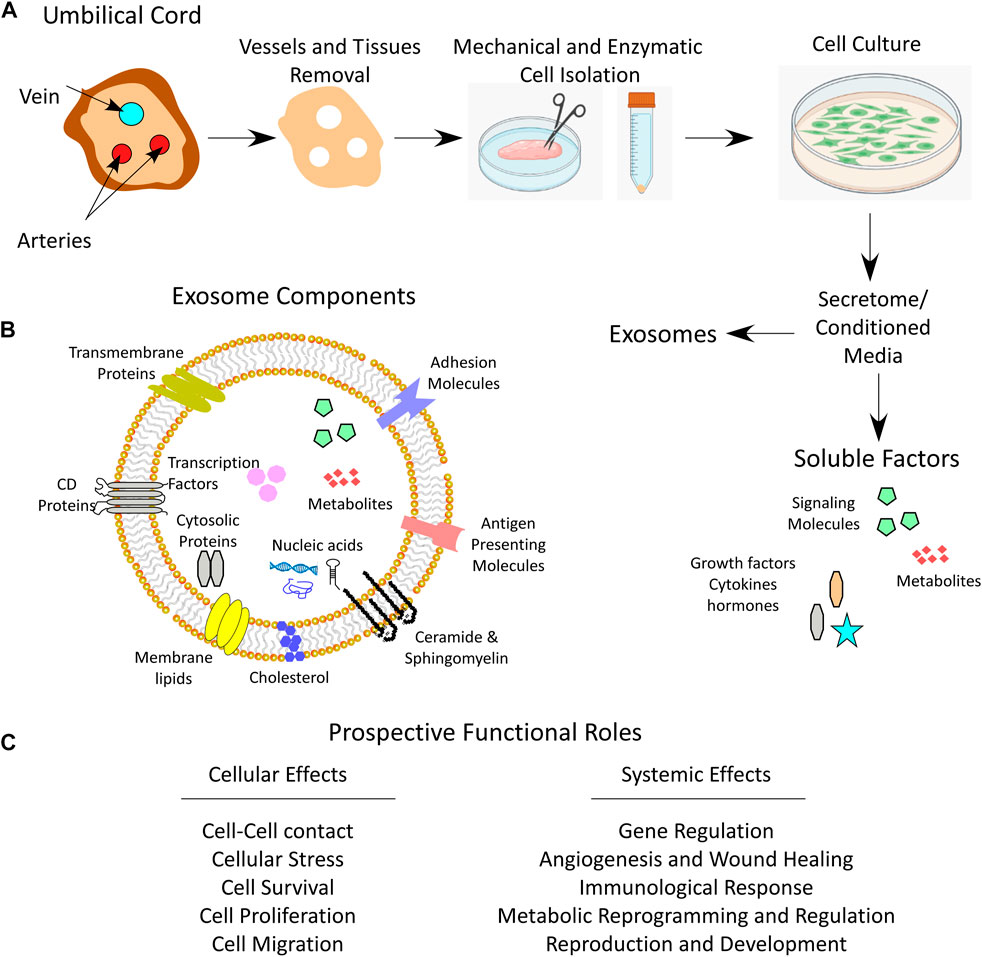
FIGURE 1. Umbilical cord WJ-MSCs and secretome. (A) Anatomical illustration of a cross section of umbilical cord depicting Wharton’s jelly, the process of WJ-MSCs mechanical and enzymatic isolation, culturing and secretome collection which contains both soluble and exosome fractions. (B) Schematic image for the exosome components. (C) Prospective functional roles of the secretome that influence cell function and system homeostasis.
WJ’s function is to protect the enclosed vessels from compression, torsion and bending to maintain the blood flow between the fetal and maternal circulations. The mucous connective tissue contains specialized fibroblast-like cells and some mast cells. These stromal cells are called myofibroblasts because they exhibit some ultrastructural features of both smooth muscle cells and fibroblasts (Karahuseyinoglu et al., 2007). WJ is the major source of MSCs from the umbilical cord due to the large number of MSCs that may reach up to 4,700,000 MSCs/cm of the umbilical cord (Subramanian et al., 2015). In addition, the cells isolated from WJ show specific characteristics of MSCs, such as pluripotency and self-renewal as well as the ability to adhere to plastic in culture, the expression of specific surface antigens, namely CD105, CD73 and CD90, as well as their ability to differentiate into osteoblasts, adipocytes and chondroblasts (Ali et al., 2015).
2.1 Origin of WJ-MSCs
Although the ontogeny of MSCs is well-documented in both human and rodent fetal and adult tissues, little is known about the origin of WJ-MSCs. However, it is widely accepted that WJ-MSCs and adult MSCs have common parental cells, since both have similar structure and shape, possess the same surface markers, and have similar plasticity and multipotency (Can and Karahuseyinoglu, 2007). At the human embryonic stage E26-E27 [E11-E12 in mice (Mendes et al., 2005)], mesenchymal progenitor/stem cells initially arise in unique structures within the intra-embryonic aorta-gonad-mesonephros (AGM) region, i.e. in the earliest hematopoietic-forming sites (Wang et al., 2008). Although the hemangioblast compartments provide a good niche for the maintenance and proliferation of the mesenchymal progenitor/stem cells, these cells are different from their neighboring hematopoietic or endothelial progenitor cells (Durand et al., 2006; Guillot et al., 2007). In addition, WJ-MSCs are capable of proliferation and differentiation independently from any support by neighboring cells, whereas the hematopoietic stem cells (HSCs) are dependent on stromal cells as feeder cells. (Oostendorp et al., 2002; Mendes et al., 2005). During embryogenesis, MSCs co-localize with hematopoietic stem and progenitor cells, and circulate from the AGM region to various tissues (Migliaccio et al., 1986; Takashina, 1987; Zanjani et al., 1993; Tavian et al., 1999). Campagnoli et al., (2001) and Guillot et al., (2007) recovered a large number of MSCs from human fetal blood, liver, and bone marrow in the first-trimester of pregnancy, which showed the expression of pluripotency markers, demonstrated rapid growth and increased telomere length. However, in the second- and third-trimesters, the detected frequency of MSCs was low in the circulation and hematopoietic tissues, but high in the bone marrow, suggesting that MSCs undergo a migration process and are eventually stored in the bone marrow (Campagnoli et al., 2001). During the migration of MSCs from the AGM region to the fetal liver and bone marrow, some cells get trapped, and thus colonize the gelatinous material of the WJ, forming WJ-MSCs (Mendes et al., 2005; Batsali et al., 2013).
2.2 Isolation and culture of stromal cells
Cells from the umbilical cord can be isolated using two different methods, the explant method, or the enzymatic digestion method (Figure 1). The explant method requires mechanical tissue mincing that is followed by placing the tissue at substrate/tissue interface, which results in cell outgrowth on a plastic surface. The enzymatic digestion method, on the other hand, involves an additional step of tissue enzymatic digestion before plating on tissue culture plates (Mushahary et al., 2018). To isolate WJ-MSCs by enzymatic method, a freshly removed 5–10 cm long umbilical cord needs to be immediately transported to the laboratory in a sterile and cooled transfer medium (e.g. Hanks’ balanced salt solution). Then, before further processing of the tissue, arteries and veins are aseptically removed. After that, the cord is mechanically chopped and can be digested using enzymes such as collagenase, hyaluronidase, caseinase, clostripain and tryptic activity (Can and Karahuseyinoglu, 2007). The tissue homogenate is then filtered through 70–100 µm pore sized sieves to remove unnecessary tissue debris and the cells are plated, displaying a fibroblast-like appearance over the first culture period until the first passage (Figure 1) (Can and Karahuseyinoglu, 2007; Karahuseyinoglu et al., 2007).
2.3 Proliferation and senescence
For cell-based therapy, using adult MSCs involves some challenges, including also their failure to proliferate infinitely. They have a limited number of population doublings before they become senescent, that is a state of cell division arrest which eventually limits their immunomodulatory and differentiation capacities and thus, their clinical application is impeded (Fan et al., 2011; Turinetto et al., 2016). Due to their embryonic origin, WJ-MSCs show a delayed progression to senescence, compared to other MSCs (Batsali et al., 2017; Liau et al., 2020). Liau et al., (2020) observed no significant differences in WJ-MSCs’ proliferation, cell cycle, phenotype, and stemness marker expression after serial cell passaging. However, the expression of senescence-related gene, p21, and oncogene, c-Myc, was significantly upregulated at late passages (>20 cell passages). Furthermore, at low (<10) cell passages, WJ-MSCs adopt small fine-spindle shape which then transforms into flat, long, and broader cell morphology at later passages associated with low proliferation rate (Panwar et al., 2021). The late passage cells are non-tumorigenic, show slow cellular aging and do not exhibit chromosomal abnormalities. However, further passages demonstrate shorter telomere length (Panwar et al., 2021). Due to their embryonic nature, WJ-MSCs have low senescence rate relative to adult MSCs. Therefore, earlier passages of WJ-MSCs are good candidates for therapeutic use.
3 Principle of cell-fee based therapy
Several studies have demonstrated promising results for the treatment of different diseases using MSC-based therapy (Connick et al., 2012; Karantalis et al., 2014; Rushkevich et al., 2015; Vega et al., 2015; Fernández et al., 2018). Although the exact mechanism of action of MSCs remains unclear, various studies show that it is the secreted factors and EVs, collectively called the secretome, that cause the improvement rather than cellular differentiation at the site of injury or tumor per se (Gomes et al., 2018). The term secretome was originally defined by Tjalsma et al., (2000) as “both the components of machineries for protein secretion and the native secreted proteins.” However, currently the secretome is defined as “the factors that are secreted by a cell, tissue, or organ to the extracellular space at a specific time and under defined conditions” (Hathout, 2007; Agrawal et al., 2010). As mentioned above, the secretome is composed of soluble factors (growth factors, cytokines, chemokines, interleukins, prostaglandins, angiogenic mediators, hormones) and EVs including exosomes and MVs that harbor the vital molecules including lipids, proteins (cell adhesion molecules, extracellular matrix proteins, receptors, enzymes, metabolites, transcription factors), RNA and DNA subtypes inside or on their surfaces (Baraniak and McDevitt, 2010; Vizoso et al., 2017; Witwer and Théry, 2019; Daneshmandi et al., 2020; Xunian and Kalluri, 2020; Al Madhoun et al., 2021).
The use of cells’ secretome as a whole or only the EVs for treatment of diseases is termed as cell-free based therapy. Its benefits include the overcoming of ethical issues associated with cellular transplantation and preventing survival or complications resulting from incorrect differentiation of the cells in the host tissue, while maintaining the therapeutic potential (Chronopoulos and Kalluri, 2020; Kalluri and LeBleu, 2020).
4 Extracellular vesicles (EVs), their origin, subtypes, and composition
EVs are lipid bound vesicles harboring proteins, lipids and nucleic acids (Zaborowski et al., 2015; Bebelman et al., 2018) that are secreted into the extracellular space (Yáñez-Mó et al., 2015; Zaborowski et al., 2015; Théry et al., 2018). They play a role in intercellular communication and have the potential to alter the function of the recipient cell (White et al., 2006; Harding et al., 2013; Zaborowski et al., 2015). There are three principal subtypes of EVs including microvesicles (MVs), exosomes, and apoptotic bodies, which are distinguished based on their biogenesis and release pathways, their size, content, and function (Borges et al., 2013; Yáñez-Mó et al., 2015; Zaborowski et al., 2015). Despite the fact that their protein profiles vary based on their formation pathways, there are no specific distinguishing protein markers identified as yet. Exosomes are vesicles (30–150 nm in diameter) that are enclosed within a single outer membrane, originate from the endosome, and are secreted by all types of cells (Yáñez-Mó et al., 2015; Bebelman et al., 2018). Exosomes play a role in intercellular communication, cell maintenance, and tumor progression. They may also induce immune responses by acting as antigen-presenting vesicles (Bobrie et al., 2011; Chaput and Théry, 2011; Doyle and Wang, 2019). Microvesicles (MVs), also known as ectosomes, microparticles or shedding MVs, on the other hand, are vesicles (100 nm to 1 µm in diameter) (Borges et al., 2013; Yáñez-Mó et al., 2015; Zaborowski et al., 2015) that form by direct outward budding or pinching of the cell’s plasma membrane. It is believed that their formation requires cytoskeleton components (actin and microtubules), molecular motors (kinesins and myosins), and fusion machinery (SNAREs and tethering factors) (Cai et al., 2007). Due to their outward blebbing from the plasma membrane, in contrast to exosomes, MVs are abundant in cytosolic and plasma membrane associated proteins (Doyle and Wang, 2019), such as cytoskeletal proteins, integrins, heat shock proteins (HSPs), and tetraspanins (Willms et al., 2018). Annexin A1 which belongs to the family of Ca2+-dependent phospholipid-binding membrane proteins has been identified as a specific marker of MVs (Jeppesen et al., 2019). In mammals, MVs can be released by almost all cell types such as blood cells (platelets, leukocytes, and erythrocytes) (Wolf, 1967), endothelial cells (Elsner et al., 2023), and vascular smooth muscle cells (Boulanger et al., 2006). Apart from differences in their size and biogenesis, MVs and exosomes express different surface molecules used as biomarkers for their identification (Wu et al., 2013). Prototypic exosome markers include tetraspannins (CD9, CD63 and CD81) and ESCRT proteins (Alix and TSG101) (Hessvik and Llorente, 2018; Mathieu et al., 2021) while MVs are well studied in tumor cells and their markers frequently include CD40, ARF6, selectins, and flotillin-2 (Sedgwick and D'Souza-Schorey, 2018). MVs, like exosomes, are involved in intercellular communication. Apoptotic bodies (50 nm up to 5,000 nm in diameter) are released by dying cells due to separation of the plasma membrane from the cytoskeleton (Wickman et al., 2012). Unlike both exosomes and MVs, apoptotic bodies may contain intact organelles, chromatin, and small amounts of glycosylated proteins (Thery et al., 2001).
5 Wharton’s jelly MSCs secretome
5.1 Comparison of WJ-MSC’s secretome to that derived from other MSCs
The ability of the secretome to mediate various biological functions prompted exploratory studies on its use in cell-free therapies. The secretome of MSCs displays heterogeneous profiles depending on factors such as host age, source of MSCs, and the cell culture/differentiation media used (Paliwal et al., 2018). Investigating the differences in MSCs’ secretome and elucidating the mechanisms of action of their components may potentially facilitate effective and cell-free use of the secretome for treating different diseases (Kupcova Skalnikova, 2013; Driscoll and Patel, 2019; Kandoi et al., 2019; Wang L-T. et al., 2021; Munoz-Perez et al., 2021; Sandonà et al., 2021; Muzes and Sipos, 2022; Ghasemi et al., 2023).
Moreover, recent advances in analytical techniques have allowed the mapping of MSCs’ secretome and identifying the therapeutic factors applicable in regenerative medicine. The proteomic methods used for characterizing the secretome of MSCs are based on approaches involving immunological, shotgun and proteomic assays (Lavoie and Rosu-Myles, 2013). Immunological assays, including enzyme-linked immunosorbent assay (ELISA), Luminex antibody bead-based array, microarray, Western blotting, and cytokine antibody array, are highly specific, sensitive, and reproducible. While, the shotgun-based proteomics, two-dimensional gel electrophoresis, liquid chromatography with tandem mass spectrometry, stable isotope labeling by amino acids in cell culture (SILAC), matrix-assisted laser desorption/ionization time of flight (MALDI-TOF), MS/MS and quadrupole time-of-flight mass spectrometry (QTOF-MS), enable the identification of unknown and uniquely secreted proteins (Kandoi et al., 2019).
Significant differences in the secretomes’ profiles of MSCs from different sources have been documented (Shin et al., 2021). Moreover, Kim et al. observed a donor-to-donor variation in the secretome profiles of WJ-MSCs, even under identical culture conditions and passage number (Kim et al., 2019). Therefore, it is important to analyze the composition and functions of the secretome of different MSCs as it may affect their therapeutic potential. Hitherto, the best characterized secretome are those of bone marrow derived MSCs and adipose stem/stromal cells (Kupcova Skalnikova, 2013). Only recently, a comparative analysis of human WJ-MSC secretome has revealed the presence of a large number of proteins (Shin et al., 2021). For example, a study showed that alpha-2-macroglobulin (α2M) was the most highly expressed protein, after serum albumin (Bakhtyar et al., 2018). The secretome of these cells was also found to be enriched with cytokines/chemokines and growth factors, including interleukin (IL) 1-alpha (IL-1α), IL-1β, IL-6, IL-8, and granulocyte-macrophage colony-stimulating factor (GM-CSF), which was shown to have both pro- and anti-tumorigenic effects (Mirabdollahi et al., 2019). Other secreted factors include IL-2, IL-7, IL-12, IL-15, monocyte chemoattractant protein-1 (MCP-1), macrophage inflammatory protein-1beta (MIP-1β), regulated upon activation, normal T cell expressed and presumably secreted (RANTES), and platelet-derived growth factor (PDGF)-AA (Mirabdollahi et al., 2019). These factors are involved in cellular proliferation and differentiation, tissue remodeling, and regulating inductive events in patterning and morphogenesis; while chemoattractants such as MCP1, MIP-1β, RANTES, hepatocyte growth factor (HGF), fibroblast growth factor-2 (FGF-2), and PDGF-AA, facilitate mobilizing of immune cells in the process (Yoo et al., 2009; Prasanna et al., 2010; Konala et al., 2020). A recent study that compared expression profiles of WJ-MSCs and bone marrow derived MSCs reported significant differences between both (Barrett et al., 2019). They found that 436 genes were significantly and differentially expressed in WJ-MSCs (Barrett et al., 2019). These genes play a role in different processes, such as immunomodulation, angiogenesis, wound healing, apoptosis, antitumor activity, and chemotaxis (Barrett et al., 2019). The authors are suggesting that these differences may explain the advantages of using WJ-MSCs over BM-MSCs in clinical applications (Barrett et al., 2019). A myriad of biomolecules and factors detected in the secretome of different MSCs is summarized in Table 1.
5.2 Therapeutic potential and applications of WJ-MSCs and their secretome
As mentioned above, it is thought that MSCs facilitate the tissue and organ repair by their multipotent potential that enables them to replace the damaged cells (Mahmood et al., 2003; Murphy et al., 2003). However, it was later suggested that in response to tissue injury, MSCs home to the damaged site and stimulate repair by producing trophic factors such as growth factors, cytokines, and antioxidants (Chen et al., 2008; Karp and Leng Teo, 2009). Some of these factors impart MSCs their immunomodulatory potential (English et al., 2010). In general, the biological characteristics of MSCs that form the basis of their clinical applications include: (a) their ability to home to sites of inflammation following tissue injury when injected intravenously (Rustad and Gurtner, 2012); (b) the secretion of multiple bioactive molecules capable of stimulating recovery of injured cells and inhibiting inflammation (Ranganath et al., 2012), (c) modulating the immune functions (Lo Iacono et al., 2018), (d) differentiation into various cell types (Wang S. et al., 2012), and (e) as a tool for gene therapy (Kamal and Kassem, 2020).
Because the secretome of WJ-MSCs plays roles in cellular homeostasis, anti-inflammation, tissue replenishment, immunomodulation, and other functions (Tang et al., 2021), the therapeutic potentials of WJ-MSCs and their secretome have been explored for several disease conditions as briefly reviewed in the following sections.
5.3 Immunomodulatory properties
The clinical utility of WJ-MSCs is tantamount, due basically to their low immunogenicity (Liu et al., 2012; Kim et al., 2013; Varaa et al., 2019). WJ-MSCs were found to express low-to-moderate levels of MHC class I (HLA-ABC) molecules (Prasanna et al., 2010; Liu et al., 2012; de Girolamo et al., 2013) and lack the expression of MHC class II (HLA-DR) and co-stimulatory antigens such as CD40, CD80 and CD86 that lead to T- and B-cell mediated responses (Pappa and Anagnou, 2009; Prasanna et al., 2010). Their immunosuppressive potential also relates to their ability to produce large quantities of immunosuppressant cytokines, such as TGF-β, IL-10, and VEGF (Weiss et al., 2008).
Interestingly, MSCs, including WJ-MSCs, may interact with and modulate the activation and function of all key immune effector cells including T or B cells (Le Blanc et al., 2004; Aggarwal and Pittenger, 2005; Uccelli et al., 2006; Carreras-Planella et al., 2019), monocyte or macrophages (Cutler et al., 2010; Dymowska et al., 2021; Lu et al., 2021), dendritic cells (DCs) (Tipnis et al., 2010; Gao et al., 2017; Vieira Paladino et al., 2019), neutrophils (Khan et al., 2015; Ahn et al., 2020; Taghavi-Farahabadi et al., 2021), mast cells (Brown et al., 2011; Cho et al., 2022), and natural killer cells (Casado et al., 2013; Najar et al., 2018; Abbasi et al., 2022). Although the mechanism of immunomodulatory activity remains to be elucidated, it is thought that both cell-to-cell contact and soluble factors are the key players in WJ-MSCs mediated immunosuppression (Shi et al., 2010; Ma et al., 2014). Of note, WJ-MSCs and their secretome possess the immunomodulatory properties (Mrahleh et al., 2021; Muzes and Sipos, 2022), in addition to exerting anti-inflammatory effects (Munoz-Perez et al., 2021). In this regard, immune-modulatory effects of WJ-MSCs secretome were related to the presence of several secreted factors, including IL-2, IL-6, IL-12, IL-15, CXCL8 (IL-8), CCL2 (MCP-1), CCL3/4 (MIP-1), CCL5 (RANTES), and prostaglandin-E2 (PGE2) (Yoo et al., 2009). Taghavi-Farahabadi et al., (2021) demonstrated that WJ-MSCs’ secretome improved the function and expanded the lifespan of neutrophils, which might have therapeutic applications for treating neutropenia or chronic granulomatous disease. These positive effects of exosomes were ascribed to miRNAs and mRNAs, as well as several secreted factors present in exosomes, including tumor necrosis factor α (TNFα), G-CSF, interferon (IFN)-γ, IFN-α, IL-8, and IL-6 (Taghavi-Farahabadi et al., 2021). Moreover, WJ-MSCs can modify T cell receptor-mediated T cell activation via EVs enriched with programmed death-ligand 1 (PD-L1) which reduces T cell activation in acute graft versus host disease (Li et al., 2021). WJ-MSCs’ exosomes also proved to be beneficial for treating lymphedema by increasing the expression of lymphangiogenic factors including angiopoietin-2 (Ang2), prospero-homeobox protein 1 (Prox1), and phospho-Akt (Ting et al., 2021). Moreover, based on their immunomodulatory effects, whether through cell-to-cell contact or soluble factors, WJ-MSCs and their secretome have been used to successfully treat morbid conditions, such as graft versus host disease (Newell et al., 2014; Soder et al., 2020; Pochon et al., 2022), diabetes (Katuchova et al., 2015; Moreira et al., 2017; Gao et al., 2018; Qi et al., 2019), and cancer (Hendijani et al., 2015).
5.4 Tissue repair and injury prevention
5.4.1 Tissue repair
Tissue repair is defined as a compensatory regeneration and restoration of tissue architecture and function following a surgical, mechanical, or chemical-induced injury (Krafts, 2010). Tissue repair is a dynamic complex process that involves the coordinated action of many different cells and molecules. The mechanism of tissue repair includes the activation of immune response, angiogenesis, innervation, epithelialization, and scar formation, best reviewed in (Deng et al., 2022) and (Eming et al., 2014). Notably, the administration of WJ and other sources MSCs’ secretome improves the tissue repair due to its ability to modulate the process of inflammation by inducing anti-inflammatory responses (Chen et al., 2015; Hervás-Salcedo et al., 2021; Miyano et al., 2022; Wang et al., 2022), including also the M2 macrophage polarization (Nakajima et al., 2012; Zhou et al., 2016; Luz-Crawford et al., 2017; Wang J. et al., 2021). Furthermore, WJ-MSCs’ secretome was found to mediate angiogenesis, neuroprotection and neurogenesis (Hsieh et al., 2013).
5.4.2 Wound healing and repair
Despite the efforts focused on wound care and new therapeutic approaches for acute and chronic wound management, wound healing is still a challenging clinical problem. The process of wound healing involves an interplay between several cell populations, the extracellular matrix and the action of soluble mediators including growth factors and cytokines. The process may be studied by dividing it into four phases, (i) coagulation and hemostasis, (ii) inflammation, (iii) proliferation, and (iv) wound remodeling with scar tissue formation, best reviewed in (Velnar et al., 2009).
The pioneering work by Bakhtyar et al., (2018) identified that the exosomes isolated from WJ-MSCs promote skin wound healing by increasing fibroblasts viability, migration, and the expression of myofibroblast marker alpha smooth muscle actin (αSMA) and enhanced skin wound healing in the punch biopsy wound model in mice. Proteomic analysis of exosomes revealed that the alpha-2-macroglobulin (α2M) protein played a key role in promoting wound healing (Bakhtyar et al., 2018). Similarly, another group of researchers also found that the exosomes of WJ-MSCs were instrumental in enhancing skin wound healing and the underlying mechanism involved attenuation of cell death by suppressing nuclear translocation of apoptosis-inducing factor (AIF) which is a mitochondrial oxidoreductase that contributes to cell death and participates in the respiratory chain assembly (Zhao et al., 2020). Kim et al., (2019) reported that the pro-angiogenic activities of WJ-MSCs were related to their secretome containing angiogenin, MCP-1, IL-8, and VEGF.
5.4.3 Neuroprotection
Perinatal brain injury (PBI) is one of the main causes of perinatal morbidity and mortality (Volpe, 1995). PBI is mainly caused by cerebral ischemia, cerebral hemorrhage, or ascending intrauterine infection because of accidental trauma or genetic disorders. PBI has an enormous impact on the effected family and society which requires co-operation between physicians, neurologists, physio-, speech-, and psychotherapists, as well as other specialists. (Jensen et al., 2003). More effective neuroprotective strategies are being developed. One of these strategies involves the use of WJ-MSCs’ exosomes, such as to alleviate the pathogenesis of PBI which is associated with the death of neurons and pre-oligodendrocytes and by reducing microglia-mediated neuroinflammation (Thomi et al., 2019a). Thomi et al., (2019a) demonstrated that exosomes of WJ-MSCs exhibited the anti-inflammatory potential, both in vitro and in vivo, by targeting microglia cells which reduced the expression of pro-inflammatory cytokines through interference with the toll-like receptor 4 (TLR4)/CD14 pathway. The same group of researchers also reported that intranasal administration of WJ-MSCs’ exosomes could protect white and gray matter in PBI by improving neuron cell viability, development, and the recovery of learning ability in animal models of PBI (Thomi et al., 2019b).
Neuroprotective potential of WJ-MSCs’ secretome was also demonstrated in Alzheimer’s disease. Alzheimer’s disease is a progressive brain disease that negatively affects the performance of daily activities in older individuals. This progressive cognitive decline is associated with the accumulation of amyloid-beta (Aβ) and tau proteins (Selkoe and Hardy, 2016). The accumulation of Aβ, produced by sequential cleavage of amyloid precursor protein (APP) by beta-secretase and gamma-secretase, results in the formation of Aβ oligomers that are toxic to neurons (Haass and Selkoe, 2007). In contrast, tau protein results from alternative splicing of the microtubule associated protein tau (MAPT) gene, forming soluble protein isoforms (Goedert et al., 1989). Several functional interactions between these two proteins result in neural circuit damage and cognitive decline in Alzheimer’s disease. Unfortunately, no treatment that cures this disease is available yet. However, one of the recent treatment approaches is to explore the neuroprotective potentials of MSCs’ exosomes (Zhang et al., 2021; Kandimalla et al., 2023). EVs from WJ-MSCs were shown to protect against Alzheimer’s disease by preventing the damage caused by amyloid beta oligomers in hippocampal neurons (Bodart-Santos et al., 2019). WJ-MSCs’ exosomes also improved spatial memory in Alzheimer’s disease models of olfactory bulbectomized mice (Zhdanova et al., 2021). WJ-MSCs-conditioned media was reported to improve Schwann cell viability and proliferation (Guo et al., 2015). Similarly, hepatocyte growth factor (HGF) and brain-derived neurotrophic factor (BDNF) secreted by WJ-MSCs were found to have neuroprotective effects on the damaged neurons (Mukai et al., 2018).
5.4.4 Anti-fibrotic potential of WJ-MSCs and their secretome
Fibrosis is defined as an overgrowth, hardening, and/or scarring of different tissues due to the formation and deposition of excess extracellular matrix components including collagen and fibronectin, leading to formation of scar tissue (Schuppan et al., 2001; Wynn, 2008; Wynn and Ramalingam, 2012). The resulting replacement of the normal tissue by fibrous tissue disrupts the structure and function of the tissue (Tomasek et al., 2002; Friedman, 2004; Wynn, 2007), causing an impaired function of the organ affected which may lead to life-threatening complications. Fibrotic diseases can affect different organs and tissues including the lung (pulmonary fibrosis), liver (liver cirrhosis), heart (cardiac fibrosis), kidney (renal fibrosis) and skin (systemic sclerosis) (Wernig et al., 2017).
Although it is believed that fibrosis is the end result of chronic inflammation (Wynn, 2008), accumulating evidence suggests that the mechanisms inducing fibrogenesis are different from those regulating inflammation (Wynn, 2008). Fibrosis is a complex and multifactorial process that may be triggered by different factors (Wynn, 2008). However, in all fibrotic diseases, fibrotic tissue remodeling begins by the activation of ECM-producing myofibroblasts (Gabbiani, 2003; Wynn and Ramalingam, 2012) that leads to the production of surplus quantities of extracellular matrix proteins comprising of 43 types of collagen subunits, 36 proteoglycans and about 200 types of complicated glycoproteins (Hynes and Naba, 2012). These myofibroblasts can develop from different sources including the resident mesenchymal cells, epithelial/endothelial-mesenchymal (EMT/EndMT) transition (Kalluri and Neilson, 2003; Quan et al., 2006; Willis et al., 2006; Zeisberg et al., 2007) or from fibrocytes that are derived from bone-marrow stem cells (Bucala et al., 1994). Autocrine factors secreted by myofibroblasts as well as different paracrine signals from lymphocytes and macrophages can activate myofibroblasts (Wynn, 2008; Van Linthout et al., 2014). In addition, the pathogen-associated molecular patterns (PAMPs) may also play a role in myofibroblast activation (Otte et al., 2003; Coelho et al., 2005; Meneghin and Hogaboam, 2007).
Currently, the treatment options for fibrotic diseases are limited (Rosenbloom et al., 2017) and mainly focus on symptom management and target the inflammatory response (Zhao et al., 2022). The multifactorial etiology and redundancy of pathways involved make it hard to find a single drug that will be successful in stopping or modifying fibrotic disease progression. Therefore, anti-fibrotic therapy development requires targeting the molecular pathways that lead to fibrosis including inhibiting the activation or proliferation of fibroblasts, promotion of excessive ECM degradation, or modulating the immune response (McVicker and Bennett, 2017). In this regard, research studies concerned with the development of anti-fibrotic therapies are reporting encouraging results (Zhao et al., 2022), even in cell-based therapies (Cheng et al., 2022).
The fact that WJ-MSCs possess immunomodulatory and anti-fibrotic properties attracted attention to their therapeutic potential. The anti-fibrotic potential of WJ-MSCs is multifactorial and may involve a combination of direct and indirect effects on the cellular and molecular mechanisms involved in fibrosis (Lin et al., 2010). The direct effects may include inhibition of fibroblast activation and proliferation, and the reduction of collagen synthesis and deposition in the extracellular matrix (Lin et al., 2010). WJ-MSCs’ secretome contains factors that can directly modulate these processes, including transforming growth factor β (TGF-β) inhibitors, matrix metalloproteases (MMPs) that degrade excess extracellular matrix and anti-inflammatory cytokines such as IL-10 that can reduce inflammation and tissue damage (Muzes and Sipos, 2022). The indirect effects of WJ-MSCs that contribute to their anti-fibrotic potential may involve their ability to modulate the immune response, stimulate tissue regeneration and repair and enhance angiogenesis (Ahangar et al., 2020). Tissue repair and regeneration is mediated by the ability of WJ-MSCs to differentiate into various cell types, such as fibroblasts, epithelial cells, and endothelial cells (Ali et al., 2015). Moreover, WJ-MSCs ability to enhance angiogenesis, which in turn improves tissue perfusion and oxygenation, stimulates healing and may play a role in the anti-fibrotic characteristics of these cells (Hsieh et al., 2013).
In contrast, WJ-MSCs may exert indirect effects on fibrosis by their ability to modulate the immune response, promote tissue regeneration, and enhance angiogenesis (Planat-Benard et al., 2021). The immunomodulatory activity of WJ-MSCs is mediated by their secretome which contains factors, such as TGF-β and IL-10, that regulate the activity and proliferation of immune cells eventually reducing pro-inflammatory cytokines production and inhibiting the immune response that leads to fibrosis (Planat-Benard et al., 2021). It was demonstrated that WJ-MSCs exhibit increased expression of immunosuppressive proteins, such as leukocyte antigen G6 (HLA-G6) that plays a vital role in avoiding immune-based responses against the embryo, indoleamine-2,3-dioxygenase (IDO), and PGE2 (Weiss et al., 2008). Preliminary results of clinical studies using WJ-MSCs’ secretome, on the other hand, have demonstrated promising anti-fibrotic potential in patients with liver cirrhosis (Ding et al., 2022), pulmonary fibrosis (Liu et al., 2020), and renal fibrosis (Di Vizio et al., 2012).
5.4.4.1 Liver fibrosis
Liver fibrosis is a wound healing response to chronic injuries which if not treated can progress to liver cirrhosis (Suk and Kim, 2015; Liedtke et al., 2022). Although, numerous drugs were proven to have anti-fibrotic activity both in vitro and in animal models, none of them was effective for clinical use. Therefore, until now, the only effective therapy for end-stage liver disease remains the liver transplantation. Recently, research of liver disease treatment using MSCs is gaining attention, especially that studies have demonstrated the ability of human WJ-MSCs to differentiate into hepatocyte-like cells in vitro (Campard et al., 2008; Zhang et al., 2009; An et al., 2014).
Recent studies provide promising evidence for the use of WJ-MSCs in the treatment of liver fibrosis (Kao et al., 2020; Afarin et al., 2022). The suggested mechanisms of the therapeutic potential of WJ-MSCs regarding liver fibrosis include the paracrine effects, trans-differentiation into hepatocyte-like cells, and immunomodulatory functions (Liu et al., 2015).
The effect of WJ-MSCs on liver fibrosis has been assessed by several investigators (Tsai et al., 2009; Lin et al., 2010; Kao et al., 2020; Afarin et al., 2022). In rats, Tsai et al., (2009) have shown that injection of WJ-MSCs significantly reduced the liver fibrosis by decreasing collagen deposition, serum levels of glutamic oxaloacetic transaminase, glutamic pyruvate transaminase, and TGF-β1 and increasing mesenchymal-epithelial transition factor-phosphorylated type and hepatocyte growth factor.
Lin et al., (2010), on the other hand, investigated the use of WJ-MSCs in treatment of liver fibrosis using chemically induced liver fibrosis model. In this model, liver fibrosis was induced in rats via intraperitoneal injection of thioacetamide. WJ-MSCs were transplanted into liver-damaged rats via the portal vein and the effects were monitored by serum biochemistry and histopathology assessment and the authors found that WJ-MSCs transplantation significantly recovered serum prothrombin time and serum albumin was also improved (Lin et al., 2010). Collagen accumulation decreased after 14 days of transplantation and immunohistochemical analysis revealed that the transplanted WJ-MSCs produced albumin, HGF, and metalloproteinase (MMP), suggesting that WJ-MSCs might alleviate liver collagen and could be used in liver fibrosis therapy (Lin et al., 2010). Another study by Hammam et al., (2016) investigated the antifibrotic potential of combining either early or late WJ-MSCs treatment combined with praziquantel on both acute and chronic stages of Schistosoma mansoni-induced liver fibrosis in mice. Following transplantation, WJ-MSCs exhibited differentiation into functioning liver-like cells, which was proven by their expression of human hepatocyte-specific markers (Hammam et al., 2016). Regression of liver fibrosis was also evidenced by histopathological, morphometric, and gelatin zymographic results, in addition to the reduction of three vital contributors to liver fibrosis in the model studied including alpha smooth muscle actin, collagen-I, and interleukin-13 (Hammam et al., 2016). Praziquantel enhanced the benefits observed in the WJ-MSCs treated groups (Hammam et al., 2016). However, Rengasamy et al., (2017) indicated that CCl4-induced liver fibrosis was alleviated more effectively using human BM-MSCs than by WJ-MSCs in rat models. This could be explained by differential expression patterns of matrix metalloproteases and angiogenic factors produced by bone marrow and Wharton’s jelly derived MSCs.
It was also shown that paracrine activity of MSCs plays a role in tissue damage repair through exosomes (Salehipour Bavarsad et al., 2022). However, the types and concentrations of inflammatory mediators, such as TGF-β1 in the MSCs’ microenvironment may affect their function and therapeutic potential. In this concern, Salehipour Bavarsad et al., (2022) investigated whether WJ-MSCs pretreated with different concentrations of TGF-β1 change the anti-fibrotic properties of their exosomes on activated hepatic stellate cells. Their results demonstrated that exosomes isolated from untreated WJ-MSCs reduced TGFβ-smad2/3 signaling and expression of fibrotic markers. These effects were even more intense upon using exosomes derived from 0.1 ng/ml TGFβ-pretreated WJ-MSCs, suggesting that these pre-treated WJ-MSCs might significantly benefit the liver fibrosis patients (Salehipour Bavarsad et al., 2022).
5.4.4.2 Pulmonary fibrosis
Pulmonary fibrosis is a chronic, progressive lung disease that is characterized by progressive lung scarring, eventually leading to respiratory failure and death (Martinez et al., 2017). There are currently only two anti-fibrotic agents, namely nintedanib (ofev) and pirfenidone (Esbriet) (Marijic et al., 2021), that are FDA-approved for treating idiopathic pulmonary fibrosis which is the most common form of pulmonary fibrosis and slow down the disease progression and scarring in the lungs, but also have multiple side effects and do not cure the disease (Marijic et al., 2021). Therefore, research is attracted to investigating the utility of anti-fibrotic characteristics of MSCs for the treatment of pulmonary fibrosis. The applicability of WJ-MSCs as an anti-fibrotic agent in lungs has been demonstrated in the following studies. For example, Periera-Simon et al., (2021) compared the therapeutic potential of different sources of MSCs including WJ-MSCs in the aging mouse model of bleomycin (BLM)-induced lung fibrosis. Their results showed that all sources of MSCs, except chorionic membrane cells (CSC), decreased the Ashcroft score [a pulmonary fibrosis evaluation procedure based on histological sample analysis (Hübner et al., 2008)] and hydroxyproline levels [collagen metabolism evaluation test (Qiu et al., 2014)] on day 10 after infusion into the BLM-treated mice. The observed phenotype was mainly due to a reduction in the gene expression of αv-integrin- and TNF-α, protein markers for fibrosis and inflammation, respectively; thus,, suggesting that WJ-MSCs could promote the repair of fibrotic lung tissue (Periera-Simon et al., 2021). Another study reported that WJ-MSCs repress inflammation, reduce myofibroblast action, and enhance MMP-9 and TLR-4 receptor expression, leading to alleviation of fibrosis (Chu et al., 2019). Moreover, in a small pilot study of patients with pulmonary fibrosis, WJ-MSCs infusion led to improved lung function and reduced fibrosis as assessed by imaging studies (Yang et al., 2021; Saleh et al., 2022a).
The therapeutic potential of WJ-MSCs was also tested for the treatment of coronavirus pandemic 2019 (COVID-19) (Saleh et al., 2021; Saleh et al., 2022b), caused by SARS-CoV-2 which is known to induce a severe cytokine storm in the lungs that causes edema, defective respiration, acute respiratory distress syndrome, acute heart damage, and secondary infections (Huang C. et al., 2020), and eventually death (Huang P. et al., 2020). Owing to their’ immunomodulatory property, WJ-MSCs were suggested to attenuate COVID-19 cytokine storms by suppressing T-lymphocytes (Aggarwal and Pittenger, 2005). WJ-MSCs play an important role in modulating immune system by secreting large amounts of anti-inflammatory cytokines such as IL-10, TGF-β, IL-6, and VEGF (Zhang et al., 2020; Saleh et al., 2021). The immunomodulatory secretome of MSCs is stimulated by the pathogen-related molecules including LPS and/or dsRNA of viruses that activate the TLR receptors on MSCs (Waterman et al., 2010; Li et al., 2012). MSCs secrete paracrine factors such as keratinocyte growth factor (KGF), Ang-1, PGE2, IL-10, and other trophic cytokines which eventually enhance the alveolar fluid clearance, regulate epithelial and endothelial permeability of the lung, promote endothelial repair, and reduce inflammation (Maron-Gutierrez et al., 2014). In critically severe-type COVID-9 patients, Zhang et al., (2020) demonstrated that WJ-MSCs intravenous injections improves pulmonary function, lung inflammation, and patients’ recovery within 7 days with no obvious adverse conditions. Similarly, a phase I clinical trial demonstrated the therapeutic potential of WJ-MSCs in COVID-19 patients. In this trial, patients received WJ-MSCs intravenous injections three times three days apart, which was sufficient to improve the immune system function as demonstrated by an increase in lymphocytes percentage, absolute lymphocyte count, and CD4 and CD8 T cell ratios (Saleh et al., 2021). Moreover, a 1-year follow up of these patients demonstrated that WJ-MSCs treatment did not cause any serious complications or tumor development (Saleh et al., 2022b). Currently, there are several ongoing clinical studies that may improve the understanding about the therapeutic potential of WJ-MSCs’ and their secretome in the therapy of COVID-19 (Harrell et al., 2020). Together, these results indicate that WJ-MSCs inhibit overactivation of the immune system caused by COVID-19 and promote endogenous repair by improving the microenvironment.
5.4.4.3 Renal fibrosis
Hu et al., (2020) have shown that seeding the human WJ-MSCs into the decellularized kidney scaffold ameliorates the renal fibrosis through decreasing EMT by the TGF-β/SMAD signaling pathway following subtotal nephrectomy in rats. WJ-MSCs exhibited anti-fibrotic effects in unilateral ischemia-reperfusion injury rat model of renal fibrosis through the mechanism involving delayed epithelial-to-mesenchymal transition and reduced renal fibrosis (Du et al., 2013). Thus, WJ-MSCs hold a promising potential for the treatment of fibrotic diseases. However, more research is required to better understand their mechanisms of action, optimal dosing and delivery strategies, as well as long-term safety and efficacy concerns in clinical settings.
5.4.5 WJ-MSCs in treatment of diabetes mellitus
Diabetes mellitus is a group of metabolic diseases characterized by hyperglycemia due to deficiency in insulin secretion, insulin action, or both (Udler et al., 2017). The current cell therapy approach, i.e. islet transplantation, is challenging due to the limited donor availability, immune rejections and adverse effects of immunosuppressants (Bhonde et al., 2014). Therefore, utilizing MSCs’ secretome could be an effective intervention. In diabetes treatments, the mechanism of action of MSCs could be related to their ability to reside in pancreas and/or promoting repair by producing trophic factors including the growth factors, anti-inflammatory cytokines, and anti-oxidants (Chen et al., 2008; Karp and Leng Teo, 2009), all of which may exert anti-diabetic effects by modulating the immune system and by enhancing insulin sensitivity (English et al., 2010; Xie et al., 2016; Yin et al., 2018).
In type 2 diabetes (T2D) rodent model, WJ-MSCs injected intravenously through the tail vein were detected in several tissues including the lung, liver, spleen and pancreas, implying that the homing of WJ-MSCs was associated with recruitment to sites of tissue damage (Yin et al., 2018). Relative to UCB-MSCs and BM-MSCs, WJ-MSCs demonstrated a superior potential to differentiate into glucose stimulated insulin secreting (GSIS) cells and for better hyperglycemia control in type 1 diabetes (T1D) animal models (Chao et al., 2008; Wu et al., 2009; Wang et al., 2014; El-Demerdash et al., 2015). Interestingly, pancreatic islets co-cultured with umbilical cord blood (UCB)-MSCs induced a notable improvement in GSIS index and provided glycemic control post-transplantation in T1D mice model, supporting the notation that MSCs’ secretome enhanced the islet survival and function (Park et al., 2010; Keshtkar et al., 2020). In humans, a recent meta-analysis study assessing the therapeutic efficacy of WJ-MSCs and UCB-MSCs revealed a superior efficiency of the former cells for treating both types of diabetes mellitus (Kassem and Kamal, 2020). WJ-MSCs improved the glycemic control, β-cell function, decreased incidence of diabetic complications, and ameliorated the need for insulin injection in some of the patients (Kassem and Kamal, 2020). Furthermore, T1D patients treated with undifferentiated WJ-MSCs experienced a controlled postprandial plasma glucose levels and significant improvements in C-peptide and HbA1c levels during a 21-month follow-up period (Hu J. et al., 2013). In T2D patients, WJ-MSCs transplantation via intravenous and intrapancreatic endovascular injections retuned glycemic control and improved beta cell function by mechanisms that inhibited systemic inflammation and/or improved immunological regulation (Liu et al., 2014). Although, WJ-MSCs were used in these human clinical trials, yet most of the observed phenotypes were mainly due to the effects of their secretome (Fong et al., 2014).
6 WJ-MSCs and their microRNAs cargo
The human genome contains 1% microRNA (miRNA) coding genes, and around 30% of the protein coding genes are regulated by miRNAs (Gao et al., 2011). miRNAs are single-stranded, short (21–25 nucleotides), non-protein-coding RNAs that inhibit gene expression at post-transcriptional level by binding at the 3′ untranslated region of the target messenger RNA (mRNA) (Greco and Rameshwar, 2007; Lakshmipathy and Hart, 2008; Wagner et al., 2008; Aranda et al., 2009; Chen et al., 2010; Chen and Chen, 2011; Raut and Khanna, 2016). In most (80%) cases, this leads to the degradation of the mRNA or inhibition of protein translation (Sato et al., 2011; Dong et al., 2013; Raut and Khanna, 2016). In addition to containing proteins, WJ-MSCs’ exosomes harbor the coding (mRNAs) and non-coding (miRNAs) RNAs. In general, miRNA content of exosomes play a vital role in the biological function of exosomes and the source cells (Zhang et al., 2006; Gartel and Kandel, 2008; Gangaraju and Lin, 2009; Singh et al., 2013), by acting as signalosomes that can reprogram the cellular functions (Thomi et al., 2019a).
In general, miRNAs in stem cells have different-functions and play a significant role in determining fate of the cell. Stem cells exhibit the expression of specific miRNAs that are particularly associated with their distinct stages of differentiation (Greco and Rameshwar, 2007; Lakshmipathy et al., 2007; Bork et al., 2011; Tomé et al., 2011; Raut and Khanna, 2016). These characteristic expression signatures regulate the pluripotency and differentiation factors and can be used to characterize and monitor cell populations (Raut and Khanna, 2016). For example, an integrated analysis of miRNA and mRNA expression profiles of WJ-MSCs revealed 41 upregulated genes that represented the functions of WJ-MSCs (Chen et al., 2012). The key genes identified were KAL1 and PAPPA which are involved in maintaining the stemness of these cells, and regulate tissue development, cellular differentiation, and osteogenic protein signaling pathways (Bribián et al., 2006; Chapman et al., 2008). Moreover, the role of miRNA in determining a cell’s fate was confirmed by studying miRNA expression patterns during trans-differentiation of WJ-MSCs to hepatocyte-like cells (Raut and Khanna, 2016). The trans-differentiation of WJ-MSCs was initiated by treatment with histone deacetylase inhibitor and valproic acid and miRNA analysis revealed a significant upregulation of miRNAs involved in hepatic differentiation, including miR-23b cluster, miR-30a-5p, miR-26a-5p, miR-148a-3p, miR-192-5p, and miR-122-5p (Raut and Khanna, 2016). The targets of the upregulated miRNAs included pathways that block hepatic differentiation including transforming growth factor beta (TGFβ) and notch signaling pathways and those that inhibit the expression of transcription factors required to maintain the mesenchymal status (Li et al., 2011). Therefore, inhibition of these targets promoted the hepatic differentiation.
The miRNA expression patterns of WJ-MSCs EVs revealed miRNAs that were specific to WJ-MSCs EVs, along with those found in other types of stem cells (Zhou et al., 2018). They identified eight miRNAs that are common to WJ- and other MSCs-derived EVs, including miR-199a-3p, miR-24-3p, miR-29a-3p, miR-23a-3p, miR-638, miR-125b-5p, miR-630, and miR-21-5p (Zhou et al., 2018). The identified WJ-MSC-EV-specific miRNAs comprised of 25 miRNAs, including miR-144-3p and miR-142-3p for which biological activities have been documented (Zhou et al., 2018). miR-144-3p targets SMAD4, leading to negative regulation of osteogenic differentiation and proliferation of murine stem cells (Huang et al., 2016), while miR-142-3p promotes myeloid differentiation in hematopoietic stem cells, osteoblast differentiation in human fetal mesenchymal precursor cells, and erythroid differentiation in human embryonic stem cells (Wang XS. et al., 2012; Hu W. et al., 2013).
The therapeutic landscape of WJ-MSCs EVs, mediated by their cargo miRNAs, has shown neuroprotective and neuro-regenerative potential during hypoxic-ischemic injury (Joerger-Messerli et al., 2018). This protective and regenerative potential has been shown to be mediated by the let-7-5p miRNA family (let-7a and let-7e) that regulates caspase 3 activity (Joerger-Messerli et al., 2018). The potential of WJ-MSCs EVs to facilitate tissue repair was demonstrated by their ability to promote angiogenesis via the activation of the endogenous vascular endothelial growth factors (VEGF)-A expression (Chinnici et al., 2021). The EVs contained five miRNAs: miR-146a-5p, miR-27b-3p, miR-137, miR-125a-5p and miR-126-3p, which were upregulated and targeted the VEGF-A gene that is associated with angiogenesis (Chinnici et al., 2021). They also contained 15 miRNAs, including let-7b-5p, let-7e-5p, 21-5p, 99a-5p, 100-5p, 125b-5p, 127-3p, 145-5p, 193b-3p, 199a-3p, 214-3p, 221-3p, 222-3p, 320a, and 484, that were highly expressed in these EVs and exclusively targeted the thrombospondin 1 (THBS1) gene which is associated with the regulation of tissue repair (Chinnici et al., 2021). Moreover, WJ-MSCs-derived EVs were also found to promote the migration and proliferation of bone marrow-derived MSCs, chondrocytes, and M2 polarization of macrophages, eventually leading to osteochondral regeneration (Jiang et al., 2021). This effect was found to be promoted by 5 miRNAs (miR-92b, miR-29b, miR-374a, miR148a, and miR23a) in the EVs (Jiang et al., 2021).
In triple negative breast cancer (TNBC), WJ-MSCs-derived EVs were used to modify the cellular behavior and communication of TNBC cells and the non-cancer cells involved in tumorigenesis and metastasis (Chang et al., 2022). This effect was mediated by the internalization of WJ-MSCs EVs by the cells which resulted in the inhibition of tumor progression and metastasis (Chang et al., 2022). The transformation of the phenotypic characteristics is suggested to be mediated by the transfer of miRNA-125b from the WJ-MSCs EVs, which targets hypoxia-inducible factor 1-alpha (HIF1-α) and other genes related to proliferation, epithelial-mesenchymal transition, and angiogenesis (Chang et al., 2022). Therefore, analyzing the patterns of miRNA expression in WJ-MSCs EVs and characterizing their target genes and pathways can provide an insight into the therapeutic potential of WJ-MSCs. Similarly, as a novel method for the treatment of glioblastoma multiforme, WJ-MSCs’ exosomes were used to deliver miR-124, which reduced the expression of CDK6 and enhanced chemosensitivity to temozolomide, along with decreasing the migration of glioblastoma multiforme cells (Sharif et al., 2018).
Recent studies identified that a large amount of endogenous non-coding RNAs (ncRNAs) exist in MSCs which have critical regulatory effects on cell homeostasis and interaction with microenvironment, best reviewed in (Pant et al., 2021). Using an elegant transwell co-culture system, Sun et al., (2018) showed that circular RNA molecules were upregulated and secreted by WJ-MSCs in response to damaged endometrial stromal cells which improved the survival and repair of damaged endometrial cells. The authors reported a significant elevation in the expression levels of circRNA-8881-21, circRNA-0020492, circRNA-0026141, circRNA-4049-38, circRNA-0015825, circRNA-5028-15, and circRNA-0111659; as well as their host genes ASPM, MKI67, TROAP, WDR62, KIF14, and MYBL2, which were closely related to cellular proliferation, differentiation, and survival (Sun et al., 2018). Later, using the same experimental approach, the same research group reported the mechanistic role of circ6401-RNA, derived from WJ-MSCs secretome, in repairing the damaged endometrium by targeting miR26-b-1-5p, and hence upregulating the level of RAP1B which is a crucial angiogenic protein involved in the VEGF signaling pathway (Shi et al., 2020). Exosomes derived from WJ-MSCs, on the other hand, promoted the repair of myocardial infarction in rodent models and prevented ischemic cardiomyocytes apoptosis via the action of circ-0001273 RNA (Li et al., 2020).
7 Conclusion and future perspectives
Wharton’s jelly tissue in humans is an attractive source for MSCs. The isolated WJ-MSCs are of a naïve embryonic cell origin with a robust proliferation rate, reputable self-renewal rate, and multi-lineage differentiation potentials. In comparison with adult MSCs, WJ-MSCs are superior in respect of their minimal exposure to the environmental factors and genetic alteration, and by exhibiting better stemness characteristics. Therefore, the use of WJ-MSCs for clinical applications is ethically acceptable with minimal risk associated with the formation of teratoma (Zeddou et al., 2010; Al Madhoun et al., 2020).
WJ-MSCs’ secretome is enriched in bioactive molecules with the capacity to sustain cellular and tissue homeostasis. Owing to these dynamic characteristics, interventions using the WJ-MSCs’ secretome have been successful in treating inflammation, skin wounds, tumors, neurodegenerative disorders, tissue fibrosis, and diabetes. Therefore, it is noteworthy that WJ-MSCs’ secretome has tremendous potential, allowing for its allogeneic therapeutic applications. However, more detailed secretome profiling studies, especially those including proteomics and metabolomics, are required to gain a more in-depth understanding of its components and the underlying molecular mechanisms regulating their expression and secretion.
Noteworthy to mention that MSCs and WJ-MSCs cell-free therapeutic approach is a booming research field with tremendous potentials for novel clinical applications. There are several research publications and this review article may have covered only a limited number of the published research, yet we have overall reviewed, discussed and updated emerging research in the field to highlight importance and point to future directions.
Human WJ-MSCs and their secretome are a promising therapeutic modality for different diseases. Currently, in vitro and in vivo investigations have demonstrated the potential clinical benefits of these cells, in particular using the cell-free secretome for various clinical applications and avoiding the ethical concerns associated with cell transplantation. Although human clinical trials at phase I/II, for some diseases, are in progress, there remains a growing need for the longitudinal studies addressing the long-term efficacy of secretome-based cell-free therapy.
Indeed, WJ-MSCs and their secretome applications should follow the good manufacturing practice (GMP) guidelines for isolation, storage, quality assurance, and administration, all the while ensuring the safety and efficacy of its clinical applications. Future studies should focus on the cellular mechanisms and signaling pathways that could be exploited to enhance quality and benefits of secretome for a wide variety of biomedical applications. Importantly, an international society or organization should be on board to implement the safe practice of cell-free therapy.
Author contributions
HD, SS, and AA wrote the manuscript. RA, DH, and FA-M revised and approved the manuscript. All authors contributed to the article and approved the submitted version.
Funding
This work was funded by Kuwait Foundation for the Advancement of Sciences (KFAS) under project number RA CB-2021-007.
Acknowledgments
We would like to thank Lubaina Koti for providing writing and editing assistance. The authors also thank the Dasman Diabetes Institute for providing financial support.
Conflict of interest
The authors declare that the research was conducted in the absence of any commercial or financial relationships that could be construed as a potential conflict of interest.
Publisher’s note
All claims expressed in this article are solely those of the authors and do not necessarily represent those of their affiliated organizations, or those of the publisher, the editors and the reviewers. Any product that may be evaluated in this article, or claim that may be made by its manufacturer, is not guaranteed or endorsed by the publisher.
References
Abbasi, B., Shamsasenjan, K., Ahmadi, M., Beheshti, S. A., and Saleh, M. (2022). Mesenchymal stem cells and natural killer cells interaction mechanisms and potential clinical applications. Stem Cell Res. Ther. 13 (1), 97. doi:10.1186/s13287-022-02777-4
Afarin, R., Behdarvand, T., Shakerian, E., Salehipour Bavarsad, S., and Rashidi, M. (2022). Exosomes of Whartons' jelly mesenchymal stem cell reduce the NOX genes in TGF-β-induced hepatic fibrosis. Iran. J. basic Med. Sci. 25 (12), 1498–1503. doi:10.22038/IJBMS.2022.66802.14649
Aggarwal, S., and Pittenger, M. F. (2005). Human mesenchymal stem cells modulate allogeneic immune cell responses. Blood 105 (4), 1815–1822. doi:10.1182/blood-2004-04-1559
Agrawal, G. K., Jwa, N. S., Lebrun, M. H., Job, D., and Rakwal, R. (2010). Plant secretome: Unlocking secrets of the secreted proteins. Proteomics 10 (4), 799–827. doi:10.1002/pmic.200900514
Ahangar, P., Mills, S. J., and Cowin, A. J. (2020). Mesenchymal stem cell secretome as an emerging cell-free alternative for improving wound repair. Int. J. Mol. Sci. 21 (19), 7038. doi:10.3390/ijms21197038
Ahn, S. Y., Maeng, Y-S., Kim, Y. R., Choe, Y. H., Hwang, H. S., and Hyun, Y-M. (2020). In vivo monitoring of dynamic interaction between neutrophil and human umbilical cord blood-derived mesenchymal stem cell in mouse liver during sepsis. Stem Cell Res. Ther. 11 (1), 44. doi:10.1186/s13287-020-1559-4
Al Madhoun, A., Marafie, S. K., Haddad, D., Melhem, M., Abu-Farha, M., Ali, H., et al. (2020). Comparative proteomic analysis Identifies EphA2 as a specific cell surface marker for Wharton's jelly-derived mesenchymal stem cells. Int. J. Mol. Sci. 21 (17), 6437. doi:10.3390/ijms21176437
Al Madhoun, A., Sindhu, S., Haddad, D., Atari, M., Ahmad, R., and Al-Mulla, F. (2021). Dental pulp stem cells derived from adult human third molar Tooth: A Brief review. Front. Cell Dev. Biol. 9, 717624. doi:10.3389/fcell.2021.717624
Ali, H., Al-Yatama, M. K., Abu-Farha, M., Behbehani, K., and Al Madhoun, A. (2015). Multi-lineage differentiation of human umbilical cord Wharton's Jelly Mesenchymal Stromal Cells mediates changes in the expression profile of stemness markers. PLoS One 10 (4), e0122465. doi:10.1371/journal.pone.0122465
An, S. Y., Han, J., Lim, H. J., Park, S. Y., Kim, J. H., Do, B. R., et al. (2014). Valproic acid promotes differentiation of hepatocyte-like cells from whole human umbilical cord-derived mesenchymal stem cells. Tissue & cell 46 (2), 127–135. doi:10.1016/j.tice.2013.12.006
An, Y. H., Kim, D. H., Lee, E. J., Lee, D., Park, M. J., Ko, J., et al. (2021). High-efficient production of adipose-derived stem cell (ADSC) secretome through maturation process and its non-scarring wound healing applications. Front. Bioeng. Biotechnol. 9, 681501. doi:10.3389/fbioe.2021.681501
Aranda, P., Agirre, X., Ballestar, E., Andreu, E. J., Román-Gómez, J., Prieto, I., et al. (2009). Epigenetic signatures associated with different levels of differentiation potential in human stem cells. PLoS One 4 (11), e7809. doi:10.1371/journal.pone.0007809
Baberg, F., Geyh, S., Waldera-Lupa, D., Stefanski, A., Zilkens, C., Haas, R., et al. (2019). Secretome analysis of human bone marrow derived mesenchymal stromal cells. Biochimica Biophysica Acta (BBA) - Proteins Proteomics 1867 (4), 434–441. doi:10.1016/j.bbapap.2019.01.013
Bakhtyar, N., Jeschke, M. G., Herer, E., Sheikholeslam, M., and Amini-Nik, S. (2018). Exosomes from acellular Wharton's jelly of the human umbilical cord promotes skin wound healing. Stem Cell Res. Ther. 9 (1), 193. doi:10.1186/s13287-018-0921-2
Baraniak, P. R., and McDevitt, T. C. (2010). Stem cell paracrine actions and tissue regeneration. Regen. Med. 5 (1), 121–143. doi:10.2217/rme.09.74
Barkholt, L., Flory, E., Jekerle, V., Lucas-Samuel, S., Ahnert, P., Bisset, L., et al. (2013). Risk of tumorigenicity in mesenchymal stromal cell-based therapies--bridging scientific observations and regulatory viewpoints. Cytotherapy 15 (7), 753–759. doi:10.1016/j.jcyt.2013.03.005
Barrett, A. N., Fong, C. Y., Subramanian, A., Liu, W., Feng, Y., Choolani, M., et al. (2019). Human Wharton's jelly mesenchymal stem cells show unique gene expression compared with bone marrow mesenchymal stem cells using single-cell RNA-Sequencing. Stem Cells Dev. 28 (3), 196–211. doi:10.1089/scd.2018.0132
Batsali, A. K., Kastrinaki, M. C., Papadaki, H. A., and Pontikoglou, C. (2013). Mesenchymal stem cells derived from Wharton's jelly of the umbilical cord: Biological properties and emerging clinical applications. Curr. Stem Cell Res. Ther. 8 (2), 144–155. doi:10.2174/1574888x11308020005
Batsali, A. K., Pontikoglou, C., Koutroulakis, D., Pavlaki, K. I., Damianaki, A., Mavroudi, I., et al. (2017). Differential expression of cell cycle and WNT pathway-related genes accounts for differences in the growth and differentiation potential of Wharton's jelly and bone marrow-derived mesenchymal stem cells. Stem Cell Res. Ther. 8 (1), 102. doi:10.1186/s13287-017-0555-9
Bebelman, M. P., Smit, M. J., Pegtel, D. M., and Baglio, S. R. (2018). Biogenesis and function of extracellular vesicles in cancer. Pharmacol. Ther. 188, 1–11. doi:10.1016/j.pharmthera.2018.02.013
Bhonde, R. R., Sheshadri, P., Sharma, S., and Kumar, A. (2014). Making surrogate β-cells from mesenchymal stromal cells: Perspectives and future endeavors. Int. J. Biochem. Cell Biol. 46, 90–102. doi:10.1016/j.biocel.2013.11.006
Bobrie, A., Colombo, M., Raposo, G., and Théry, C. (2011). Exosome secretion: Molecular mechanisms and roles in immune responses. Traffic 12 (12), 1659–1668. doi:10.1111/j.1600-0854.2011.01225.x
Bodart-Santos, V., de Carvalho, L. R. P., de Godoy, M. A., Batista, A. F., Saraiva, L. M., Lima, L. G., et al. (2019). Extracellular vesicles derived from human Wharton's jelly mesenchymal stem cells protect hippocampal neurons from oxidative stress and synapse damage induced by amyloid-β oligomers. Stem Cell Res. Ther. 10 (1), 332. doi:10.1186/s13287-019-1432-5
Borges, F. T., Reis, L. A., and Schor, N. (2013). Extracellular vesicles: Structure, function, and potential clinical uses in renal diseases. Braz J. Med. Biol. Res. 46 (10), 824–830. doi:10.1590/1414-431X20132964
Bork, S., Horn, P., Castoldi, M., Hellwig, I., Ho, A. D., and Wagner, W. (2011). Adipogenic differentiation of human mesenchymal stromal cells is down-regulated by microRNA-369-5p and up-regulated by microRNA-371. J. Cell Physiol. 226 (9), 2226–2234. doi:10.1002/jcp.22557
Boulanger, C. M., Amabile, N., and Tedgui, A. (2006). Circulating microparticles: A potential prognostic marker for atherosclerotic vascular disease. Hypertension 48 (2), 180–186. doi:10.1161/01.HYP.0000231507.00962.b5
Bribián, A., Barallobre, M. J., Soussi-Yanicostas, N., and de Castro, F. (2006). Anosmin-1 modulates the FGF-2-dependent migration of oligodendrocyte precursors in the developing optic nerve. Mol. Cell Neurosci. 33 (1), 2–14. doi:10.1016/j.mcn.2006.05.009
Brown, J. M., Nemeth, K., Kushnir-Sukhov, N. M., Metcalfe, D. D., and Mezey, E. (2011). Bone marrow stromal cells inhibit mast cell function via a COX2-dependent mechanism. Clin. Exp. Allergy 41 (4), 526–534. doi:10.1111/j.1365-2222.2010.03685.x
Bucala, R., Spiegel, L. A., Chesney, J., Hogan, M., and Cerami, A. (1994). Circulating fibrocytes define a new leukocyte subpopulation that mediates tissue repair. Mol. Med. 1 (1), 71–81. doi:10.1007/bf03403533
Cai, H., Reinisch, K., and Ferro-Novick, S. (2007). Coats, tethers, Rabs, and SNAREs work together to mediate the intracellular destination of a transport vesicle. Dev. Cell 12 (5), 671–682. doi:10.1016/j.devcel.2007.04.005
Campagnoli, C., Roberts, I. A., Kumar, S., Bennett, P. R., Bellantuono, I., and Fisk, N. M. (2001). Identification of mesenchymal stem/progenitor cells in human first-trimester fetal blood, liver, and bone marrow. Blood 98 (8), 2396–2402. doi:10.1182/blood.v98.8.2396
Campard, D., Lysy, P. A., Najimi, M., and Sokal, E. M. (2008). Native umbilical cord matrix stem cells express hepatic markers and differentiate into hepatocyte-like cells. Gastroenterology 134 (3), 833–848. doi:10.1053/j.gastro.2007.12.024
Can, A., and Karahuseyinoglu, S. (2007). Concise review: Human umbilical cord stroma with regard to the source of fetus-derived stem cells. Stem Cells 25 (11), 2886–2895. doi:10.1634/stemcells.2007-0417
Carlsson, P. O., Espes, D., Sisay, S., Davies, L. C., Smith, C. I. E., and Svahn, M. G. (2023). Umbilical cord-derived mesenchymal stromal cells preserve endogenous insulin production in type 1 diabetes: A phase I/II randomised double-blind placebo-controlled trial. Diabetologia. doi:10.1007/s00125-023-05934-3
Carreras-Planella, L., Monguio-Tortajada, M., Borras, F. E., and Franquesa, M. (2019). Immunomodulatory effect of MSC on B cells is independent of secreted extracellular vesicles. Front. Immunol. 10, 1288. doi:10.3389/fimmu.2019.01288
Carvalho, M. M., Teixeira, F. G., Reis, R. L., Sousa, N., and Salgado, A. J. (2011). Mesenchymal stem cells in the umbilical cord: Phenotypic characterization, secretome and applications in central nervous system regenerative medicine. Curr. Stem Cell Res. Ther. 6 (3), 221–228. doi:10.2174/157488811796575332
Casado, J. G., Tarazona, R., and Sanchez-Margallo, F. M. (2013). NK and MSCs crosstalk: The sense of immunomodulation and their sensitivity. Stem Cell Rev. Rep. 9 (2), 184–189. doi:10.1007/s12015-013-9430-y
Chang, P. Y., Zhang, B. Y., Cui, S., Qu, C., Shao, L. H., Xu, T. K., et al. (2017). MSC-derived cytokines repair radiation-induced intra-villi microvascular injury. Oncotarget 8 (50), 87821–87836. doi:10.18632/oncotarget.21236
Chang, Y-H., Vuong, K., Ngo, N-H., Yamashita, T., Ye, X., Futamura, Y., et al. (2022). Extracellular vesicles derived from Wharton’s Jelly mesenchymal stem cells inhibit the tumor environment via the miR-125b/HIF1α signaling pathway. Sci. Rep. 12, 13550. doi:10.1038/s41598-022-17767-y
Chao, K. C., Chao, K. F., Fu, Y. S., and Liu, S. H. (2008). Islet-like clusters derived from mesenchymal stem cells in Wharton's Jelly of the human umbilical cord for transplantation to control type 1 diabetes. PLoS One 3 (1), e1451. doi:10.1371/journal.pone.0001451
Chapman, E. J., Kelly, G., and Knowles, M. A. (2008). Genes involved in differentiation, stem cell renewal, and tumorigenesis are modulated in telomerase-immortalized human urothelial cells. Mol. Cancer Res. 6 (7), 1154–1168. doi:10.1158/1541-7786.MCR-07-2168
Chaput, N., and Théry, C. (2011). Exosomes: Immune properties and potential clinical implementations. Semin. Immunopathol. 33 (5), 419–440. doi:10.1007/s00281-010-0233-9
Chen, G., Park, C. K., Xie, R. G., and Ji, R. R. (2015). Intrathecal bone marrow stromal cells inhibit neuropathic pain via TGF-beta secretion. J. Clin. Invest 125 (8), 3226–3240. doi:10.1172/JCI80883
Chen, H. C., Lee, Y. S., Sieber, M., Lu, H. T., Wei, P. C., Wang, C. N., et al. (2012). MicroRNA and messenger RNA analyses of mesenchymal stem cells derived from teeth and the Wharton jelly of umbilical cord. Stem Cells Dev. 21 (6), 911–922. doi:10.1089/scd.2011.0186
Chen, L., Tredget, E. E., Wu, P. Y., and Wu, Y. (2008). Paracrine factors of mesenchymal stem cells recruit macrophages and endothelial lineage cells and enhance wound healing. PLoS One 3 (4), e1886. doi:10.1371/journal.pone.0001886
Chen, P., Tang, S., Li, M., Wang, D., Chen, C., Qiu, Y., et al. (2023). Single-cell and spatial transcriptomics Decodes Wharton's jelly-derived mesenchymal stem cells Heterogeneity and a subpopulation with wound repair signatures. Adv. Sci. 10 (4), 2204786. doi:10.1002/advs.202204786
Chen, S. J., and Chen, H. C. (2011). Analysis of targets and functions coregulated by microRNAs. Methods Mol. Biol. 676, 225–241. doi:10.1007/978-1-60761-863-8_16
Chen, T. S., Lai, R. C., Lee, M. M., Choo, A. B., Lee, C. N., and Lim, S. K. (2010). Mesenchymal stem cell secretes microparticles enriched in pre-microRNAs. Nucleic Acids Res. 38 (1), 215–224. doi:10.1093/nar/gkp857
Chen, Y. C., Fu, Y. S., Tsai, S. W., Wu, P. K., Chen, C. M., Chen, W. M., et al. (2022). IL-1b in the secretomes of MSCs seeded on human decellularized allogeneic bone promotes angiogenesis. Int. J. Mol. Sci. 23 (23), 15301. doi:10.3390/ijms232315301
Cheng, W., Zeng, Y., and Wang, D. (2022). Stem cell-based therapy for pulmonary fibrosis. Stem Cell Res. Ther. 13 (1), 492. doi:10.1186/s13287-022-03181-8
Chinnici, C. M., Iannolo, G., Cittadini, E., Carreca, A. P., Nascari, D., Timoneri, F., et al. (2021). Extracellular vesicle-derived microRNAs of human Wharton's jelly mesenchymal stromal cells may activate endogenous VEGF-A to promote angiogenesis. Int. J. Mol. Sci. 22 (4), 2045. doi:10.3390/ijms22042045
Cho, K. A., Cha, J. E., Kim, J., Kim, Y. H., Ryu, K. H., and Woo, S. Y. (2022). Mesenchymal stem cell-derived exosomes attenuate TLR7-mediated mast cell activation. Tissue Eng. Regen. Med. 19 (1), 117–129. doi:10.1007/s13770-021-00395-4
Chronopoulos, A., and Kalluri, R. (2020). Emerging role of bacterial extracellular vesicles in cancer. Oncogene 39 (46), 6951–6960. doi:10.1038/s41388-020-01509-3
Chu, K. A., Wang, S. Y., Yeh, C. C., Fu, T. W., Fu, Y. Y., Ko, T. L., et al. (2019). Reversal of bleomycin-induced rat pulmonary fibrosis by a xenograft of human umbilical mesenchymal stem cells from Wharton's jelly. Theranostics 9 (22), 6646–6664. doi:10.7150/thno.33741
Coelho, A. L., Hogaboam, C. M., and Kunkel, S. L. (2005). Chemokines provide the sustained inflammatory bridge between innate and acquired immunity. Cytokine & growth factor Rev. 16 (6), 553–560. doi:10.1016/j.cytogfr.2005.03.004
Connick, P., Kolappan, M., Crawley, C., Webber, D. J., Patani, R., Michell, A. W., et al. (2012). Autologous mesenchymal stem cells for the treatment of secondary progressive multiple sclerosis: An open-label phase 2a proof-of-concept study. Lancet Neurology 11 (2), 150–156. doi:10.1016/S1474-4422(11)70305-2
Cutler, A. J., Limbani, V., Girdlestone, J., and Navarrete, C. V. (2010). Umbilical cord-derived mesenchymal stromal cells modulate monocyte function to suppress T cell proliferation. J. Immunol. 185 (11), 6617–6623. doi:10.4049/jimmunol.1002239
da Silva Meirelles, L., Chagastelles, P. C., and Nardi, N. B. (2006). Mesenchymal stem cells reside in virtually all post-natal organs and tissues. J. Cell Sci. 119 (11), 2204–2213. doi:10.1242/jcs.02932
Daneshmandi, L., Shah, S., Jafari, T., Bhattacharjee, M., Momah, D., Saveh-Shemshaki, N., et al. (2020). Emergence of the stem cell secretome in regenerative Engineering. Trends Biotechnol. 38 (12), 1373–1384. doi:10.1016/j.tibtech.2020.04.013
de Girolamo, L., Lucarelli, E., Alessandri, G., Avanzini, M. A., Bernardo, M. E., Biagi, E., et al. (2013). Mesenchymal stem/stromal cells: A new ''cells as drugs'' paradigm. Efficacy and critical aspects in cell therapy. Curr. Pharm. Des. 19 (13), 2459–2473. doi:10.2174/1381612811319130015
Deng, X., Gould, M., and Ali, M. A. (2022). A review of current advancements for wound healing: Biomaterial applications and medical devices. J. Biomed. Mater Res. B Appl. Biomater. 110 (11), 2542–2573. doi:10.1002/jbm.b.35086
Di Vizio, D., Morello, M., Dudley, A. C., Schow, P. W., Adam, R. M., Morley, S., et al. (2012). Large oncosomes in human prostate cancer tissues and in the circulation of mice with metastatic disease. Am. J. Pathol. 181 (5), 1573–1584. doi:10.1016/j.ajpath.2012.07.030
Ding, Y., Botchway, B. O. A., Zhang, Y., Jin, T., and Liu, X. (2022). The combination of autologous mesenchymal stem cell-derived exosomes and neurotrophic factors as an intervention for amyotrophic lateral sclerosis. Ann. Anat. 242, 151921. doi:10.1016/j.aanat.2022.151921
Dong, H., Lei, J., Ding, L., Wen, Y., Ju, H., and Zhang, X. (2013). MicroRNA: Function, detection, and bioanalysis. Chem. Rev. 113 (8), 6207–6233. doi:10.1021/cr300362f
Doyle, L. M., and Wang, M. Z. (2019). Overview of extracellular vesicles, their origin, composition, Purpose, and methods for exosome isolation and analysis. Cells 8 (7), 727. doi:10.3390/cells8070727
Driscoll, J., and Patel, T. (2019). The mesenchymal stem cell secretome as an acellular regenerative therapy for liver disease. J. Gastroenterology 54 (9), 763–773. doi:10.1007/s00535-019-01599-1
Du, T., Zou, X., Cheng, J., Wu, S., Zhong, L., Ju, G., et al. (2013). Human Wharton's jelly-derived mesenchymal stromal cells reduce renal fibrosis through induction of native and foreign hepatocyte growth factor synthesis in injured tubular epithelial cells. Stem Cell Res. Ther. 4 (3), 59. doi:10.1186/scrt215
Durand, C., Robin, C., and Dzierzak, E. (2006). Mesenchymal lineage potentials of aorta-gonad-mesonephros stromal clones. Haematologica 91 (9), 1172–1179.
Dymowska, M., Aksamit, A., Zielniok, K., Kniotek, M., Kaleta, B., Roszczyk, A., et al. (2021). Interaction between macrophages and human mesenchymal stromal cells derived from bone marrow and Wharton's jelly-A comparative study. Pharmaceutics 13 (11), 1822. doi:10.3390/pharmaceutics13111822
El-Demerdash, R. F., Hammad, L. N., Kamal, M. M., and El Mesallamy, H. O. (2015). A comparison of Wharton's jelly and cord blood as a source of mesenchymal stem cells for diabetes cell therapy. Regen. Med. 10 (7), 841–855. doi:10.2217/rme.15.49
Elsner, C., Ergün, S., and Wagner, N. (2023). Biogenesis and release of endothelial extracellular vesicles: Morphological aspects. Ann. Anat. 245, 152006. doi:10.1016/j.aanat.2022.152006
Eming, S. A., Martin, P., and Tomic-Canic, M. (2014). Wound repair and regeneration: Mechanisms, signaling, and translation. Sci. Transl. Med. 6, 265sr6. doi:10.1126/scitranslmed.3009337
English, K., French, A., and Wood, K. J. (2010). Mesenchymal stromal cells: Facilitators of successful transplantation? Cell Stem Cell 7 (4), 431–442. doi:10.1016/j.stem.2010.09.009
Fan, Y. Y., Kohno, M., Hitomi, H., Kitada, K., Fujisawa, Y., Yatabe, J., et al. (2011). Aldosterone/Mineralocorticoid receptor stimulation induces cellular senescence in the kidney. Endocrinology 152 (2), 680–688. doi:10.1210/en.2010-0829
Fernández, O., Izquierdo, G., Fernández, V., Leyva, L., Reyes, V., Guerrero, M., et al. (2018). Adipose-derived mesenchymal stem cells (AdMSC) for the treatment of secondary-progressive multiple sclerosis: A triple blinded, placebo controlled, randomized phase I/II safety and feasibility study. PLOS ONE 13 (5), e0195891. doi:10.1371/journal.pone.0195891
Fong, C. Y., Chak, L. L., Biswas, A., Tan, J. H., Gauthaman, K., Chan, W. K., et al. (2011). Human Wharton's jelly stem cells have unique transcriptome profiles compared to human embryonic stem cells and other mesenchymal stem cells. Stem Cell Rev. Rep. 7 (1), 1–16. doi:10.1007/s12015-010-9166-x
Fong, C. Y., Tam, K., Cheyyatraivendran, S., Gan, S. U., Gauthaman, K., Armugam, A., et al. (2014). Human Wharton's jelly stem cells and its conditioned medium enhance healing of excisional and diabetic wounds. J. Cell Biochem. 115 (2), 290–302. doi:10.1002/jcb.24661
Friedenstein, A. J., Chailakhjan, R. K., and Lalykina, K. S. (1970). The development of fibroblast colonies in monolayer cultures of Guinea-pig bone marrow and spleen cells. Cell Tissue Kinet. 3 (4), 393–403. doi:10.1111/j.1365-2184.1970.tb00347.x
Friedman, S. L. (2004). Mechanisms of disease: Mechanisms of hepatic fibrosis and therapeutic implications. Nat. Clin. Pract. Gastroenterol. Hepatol. 1 (2), 98–105. doi:10.1038/ncpgasthep0055
Gabbiani, G. (2003). The myofibroblast in wound healing and fibrocontractive diseases. J. Pathol. 200 (4), 500–503. doi:10.1002/path.1427
Gangaraju, V. K., and Lin, H. (2009). MicroRNAs: Key regulators of stem cells. Nat. Rev. Mol. Cell Biol. 10 (2), 116–125. doi:10.1038/nrm2621
Gao, J., Yang, T., Han, J., Yan, K., Qiu, X., Zhou, Y., et al. (2011). MicroRNA expression during osteogenic differentiation of human multipotent mesenchymal stromal cells from bone marrow. J. Cell Biochem. 112 (7), 1844–1856. doi:10.1002/jcb.23106
Gao, L. R., Zhang, N. K., Zhang, Y., Chen, Y., Wang, L., Zhu, Y., et al. (2018). Overexpression of apelin in Wharton' jelly mesenchymal stem cell reverses insulin resistance and promotes pancreatic beta cell proliferation in type 2 diabetic rats. Stem Cell Res. Ther. 9 (1), 339. doi:10.1186/s13287-018-1084-x
Gao, W-X., Sun, Y-Q., Shi, J., Li, C-L., Fang, S-B., Wang, D., et al. (2017). Effects of mesenchymal stem cells from human induced pluripotent stem cells on differentiation, maturation, and function of dendritic cells. Stem Cell Res. Ther. 8 (1), 48. doi:10.1186/s13287-017-0499-0
Gartel, A. L., and Kandel, E. S. (2008). miRNAs: Little known mediators of oncogenesis. Semin. Cancer Biol. 18 (2), 103–110. doi:10.1016/j.semcancer.2008.01.008
Ghaneialvar, H., Soltani, L., Rahmani, H. R., Lotfi, A. S., and Soleimani, M. (2018). Characterization and Classification of mesenchymal stem cells in several species using surface markers for cell therapy Purposes. Indian J. Clin. Biochem. 33 (1), 46–52. doi:10.1007/s12291-017-0641-x
Ghasemi, M., Roshandel, E., Mohammadian, M., Farhadihosseinabadi, B., Akbarzadehlaleh, P., and Shamsasenjan, K. (2023). Mesenchymal stromal cell-derived secretome-based therapy for neurodegenerative diseases: Overview of clinical trials. Stem Cell Res. Ther. 14 (1), 122. doi:10.1186/s13287-023-03264-0
Goedert, M., Spillantini, M. G., Jakes, R., Rutherford, D., and Crowther, R. A. (1989). Multiple isoforms of human microtubule-associated protein tau: Sequences and localization in neurofibrillary tangles of Alzheimer's disease. Neuron 3 (4), 519–526. doi:10.1016/0896-6273(89)90210-9
Gomes, E. D., Mendes, S. S., Assunção-Silva, R. C., Teixeira, F. G., Pires, A. O., Anjo, S. I., et al. (2018). Co-transplantation of adipose tissue-derived stromal cells and olfactory Ensheathing cells for spinal cord injury repair. Stem Cells 36 (5), 696–708. doi:10.1002/stem.2785
Greco, S. J., and Rameshwar, P. (2007). MicroRNAs regulate synthesis of the neurotransmitter substance P in human mesenchymal stem cell-derived neuronal cells. Proc. Natl. Acad. Sci. U. S. A. 104 (39), 15484–15489. doi:10.1073/pnas.0703037104
Guillot, P. V., Gotherstrom, C., Chan, J., Kurata, H., and Fisk, N. M. (2007). Human first-trimester fetal MSC express pluripotency markers and grow faster and have longer telomeres than adult MSC. Stem Cells 25 (3), 646–654. doi:10.1634/stemcells.2006-0208
Guo, Z. Y., Sun, X., Xu, X. L., Zhao, Q., Peng, J., and Wang, Y. (2015). Human umbilical cord mesenchymal stem cells promote peripheral nerve repair via paracrine mechanisms. Neural Regen. Res. 10 (4), 651–658. doi:10.4103/1673-5374.155442
Haass, C., and Selkoe, D. J. (2007). Soluble protein oligomers in neurodegeneration: Lessons from the Alzheimer's amyloid beta-peptide. Nat. Rev. Mol. Cell Biol. 8 (2), 101–112. doi:10.1038/nrm2101
Hammam, O. A., Elkhafif, N., Attia, Y. M., Mansour, M. T., Elmazar, M. M., Abdelsalam, R. M., et al. (2016). Wharton’s jelly-derived mesenchymal stem cells combined with praziquantel as a potential therapy for Schistosoma mansoni-induced liver fibrosis. Sci. Rep. 6 (1), 21005. doi:10.1038/srep21005
Harding, C. V., Heuser, J. E., and Stahl, P. D. (2013). Exosomes: Looking back three decades and into the future. J. Cell Biol. 200 (4), 367–371. doi:10.1083/jcb.201212113
Harrell, C. R., Jovicic, B. P., Djonov, V., and Volarevic, V. (2020). Therapeutic potential of mesenchymal stem cells and their secretome in the treatment of SARS-CoV-2-induced acute respiratory distress syndrome. Anal. Cell Pathol. (Amst) 2020, 1939768. doi:10.1155/2020/1939768
Hathout, Y. (2007). Approaches to the study of the cell secretome. Expert Rev. Proteomics 4 (2), 239–248. doi:10.1586/14789450.4.2.239
Hendijani, F., Javanmard, S. H., Rafiee, L., and Sadeghi-Aliabadi, H. (2015). Effect of human Wharton's jelly mesenchymal stem cell secretome on proliferation, apoptosis and drug resistance of lung cancer cells. Res. Pharm. Sci. 10 (2), 134–142.
Hervás-Salcedo, R., Fernández-García, M., Hernando-Rodríguez, M., Quintana-Bustamante, O., Segovia, J-C., Alvarez-Silva, M., et al. (2021). Enhanced anti-inflammatory effects of mesenchymal stromal cells mediated by the transient ectopic expression of CXCR4 and IL10. Stem Cell Res. Ther. 12 (1), 124. doi:10.1186/s13287-021-02193-0
Hessvik, N. P., and Llorente, A. (2018). Current knowledge on exosome biogenesis and release. Cell Mol. Life Sci. 75 (2), 193–208. doi:10.1007/s00018-017-2595-9
Higuchi, O., Okabe, M., Yoshida, T., Fathy, M., Saito, S., Miyawaki, T., et al. (2012). Stemness of human Wharton's jelly mesenchymal cells is maintained by floating cultivation. Cell Reprogr. 14 (5), 448–455. doi:10.1089/cell.2012.0020
Hsieh, J. Y., Fu, Y. S., Chang, S. J., Tsuang, Y. H., and Wang, H. W. (2010). Functional Module analysis Reveals differential osteogenic and stemness potentials in human mesenchymal stem cells from bone marrow and Wharton's jelly of umbilical cord. Stem Cells Dev. 19 (12), 1895–1910. doi:10.1089/scd.2009.0485
Hsieh, J. Y., Wang, H. W., Chang, S. J., Liao, K. H., Lee, I. H., Lin, W. S., et al. (2013). Mesenchymal stem cells from human umbilical cord express preferentially secreted factors related to neuroprotection, neurogenesis, and angiogenesis. PLoS One 8 (8), e72604. doi:10.1371/journal.pone.0072604
Hu, D., Zhang, D., Liu, B., Liu, Y., Zhou, Y., Yu, Y., et al. (2020). Human ucMSCs seeded in a decellularized kidney scaffold attenuate renal fibrosis by reducing epithelial-mesenchymal transition via the TGF-β/Smad signaling pathway. Pediatr. Res. 88 (2), 192–201. doi:10.1038/s41390-019-0736-6
Hu, J., Yu, X., Wang, Z., Wang, F., Wang, L., Gao, H., et al. (2013a). Long term effects of the implantation of Wharton's jelly-derived mesenchymal stem cells from the umbilical cord for newly-onset type 1 diabetes mellitus. Endocr. J. 60 (3), 347–357. doi:10.1507/endocrj.ej12-0343
Hu, W., Ye, Y., Zhang, W., Wang, J., Chen, A., and Guo, F. (2013b). miR-142-3p promotes osteoblast differentiation by modulating Wnt signaling. Mol. Med. Rep. 7 (2), 689–693. doi:10.3892/mmr.2012.1207
Huang, C., Geng, J., Wei, X., Zhang, R., and Jiang, S. (2016). MiR-144-3p regulates osteogenic differentiation and proliferation of murine mesenchymal stem cells by specifically targeting Smad4. FEBS Lett. 590 (6), 795–807. doi:10.1002/1873-3468.12112
Huang, C., Wang, Y., Li, X., Ren, L., Zhao, J., Hu, Y., et al. (2020a). Clinical features of patients infected with 2019 novel coronavirus in Wuhan, China. Lancet 395 (10223), 497–506. doi:10.1016/S0140-6736(20)30183-5
Huang, P., Liu, T., Huang, L., Liu, H., Lei, M., Xu, W., et al. (2020b). Use of chest CT in combination with negative RT-PCR assay for the 2019 novel coronavirus but high clinical Suspicion. Radiology 295 (1), 22–23. doi:10.1148/radiol.2020200330
Hübner, R. H., Gitter, W., El Mokhtari, N. E., Mathiak, M., Both, M., Bolte, H., et al. (2008). Standardized quantification of pulmonary fibrosis in histological samples. Biotechniques 44 (4), 507-511–514-507. doi:10.2144/000112729
Hynes, R. O., and Naba, A. (2012). Overview of the matrisome--an inventory of extracellular matrix constituents and functions. Cold Spring Harb. Perspect. Biol. 4 (1), a004903. doi:10.1101/cshperspect.a004903
Jensen, A., Garnier, Y., Middelanis, J., and Berger, R. (2003). Perinatal brain damage--from pathophysiology to prevention. Eur. J. Obstet. Gynecol. Reprod. Biol. 110 (1), S70–S79. doi:10.1016/s0301-2115(03)00175-1
Jeppesen, D. K., Fenix, A. M., Franklin, J. L., Higginbotham, J. N., Zhang, Q., Zimmerman, L. J., et al. (2019). Reassessment of exosome composition. Cell 177 (2), 428–445. doi:10.1016/j.cell.2019.02.029
Jiang, R., Han, Z., Zhuo, G., Qu, X., Li, X., Wang, X., et al. (2011). Transplantation of placenta-derived mesenchymal stem cells in type 2 diabetes: A pilot study. Front. Med. 5 (1), 94–100. doi:10.1007/s11684-011-0116-z
Jiang, S., Tian, G., Yang, Z., Gao, X., Wang, F., Li, J., et al. (2021). Enhancement of acellular cartilage matrix scaffold by Wharton's jelly mesenchymal stem cell-derived exosomes to promote osteochondral regeneration. Bioact. Mater 6 (9), 2711–2728. doi:10.1016/j.bioactmat.2021.01.031
Joerger-Messerli, M. S., Oppliger, B., Spinelli, M., Thomi, G., di Salvo, I., Schneider, P., et al. (2018). Extracellular vesicles derived from Wharton's jelly mesenchymal stem cells prevent and Resolve programmed cell death mediated by perinatal hypoxia-ischemia in neuronal cells. Cell Transpl. 27 (1), 168–180. doi:10.1177/0963689717738256
Kalluri, R., and LeBleu, V. S. (2020). The biology, function, and biomedical applications of exosomes. Science 367 (6478), eaau6977. doi:10.1126/science.aau6977
Kalluri, R., and Neilson, E. G. (2003). Epithelial-mesenchymal transition and its implications for fibrosis. J. Clin. Invest 112 (12), 1776–1784. doi:10.1172/JCI20530
Kamal, M. M., and Kassem, D. H. (2020). Therapeutic potential of Wharton's jelly mesenchymal stem cells for diabetes: Achievements and challenges. Front. Cell Dev. Biol. 8, 16. doi:10.3389/fcell.2020.00016
Kandimalla, R., Saeed, M., Tyagi, N., Gupta, R. C., and Aqil, F. (2023). Exosome-based approaches in the management of Alzheimer's disease. Neurosci. Biobehav Rev. 144, 104974. doi:10.1016/j.neubiorev.2022.104974
Kao, Y. H., Lin, Y. C., Lee, P. H., Lin, C. W., Chen, P. H., Tai, T. S., et al. (2020). Infusion of human mesenchymal stem cells improves regenerative niche in thioacetamide-injured mouse liver. Tissue Eng. Regen. Med. 17 (5), 671–682. doi:10.1007/s13770-020-00274-4
Karahuseyinoglu, S., Cinar, O., Kilic, E., Kara, F., Akay, G. G., Demiralp, D. O., et al. (2007). Biology of stem cells in human umbilical cord stroma: In situ and in vitro surveys. Stem Cells 25 (2), 319–331. doi:10.1634/stemcells.2006-0286
Karantalis, V., DiFede, D. L., Gerstenblith, G., Pham, S., Symes, J., Zambrano, J. P., et al. (2014). Autologous mesenchymal stem cells produce concordant improvements in regional function, tissue perfusion, and fibrotic burden when administered to patients undergoing coronary artery bypass grafting: The Prospective Randomized Study of Mesenchymal Stem Cell Therapy in Patients Undergoing Cardiac Surgery (PROMETHEUS) trial. Circulation Res. 114 (8), 1302–1310. doi:10.1161/CIRCRESAHA.114.303180
Karp, J. M., and Leng Teo, G. S. (2009). Mesenchymal stem cell homing: The devil is in the details. Cell Stem Cell 4 (3), 206–216. doi:10.1016/j.stem.2009.02.001
Kassem, D. H., and Kamal, M. M. (2020). Therapeutic efficacy of umbilical cord-derived stem cells for diabetes mellitus: A meta-analysis study. Stem Cell Res. Ther. 11 (1), 484. doi:10.1186/s13287-020-01996-x
Katagiri, W., Kawai, T., Osugi, M., Sugimura-Wakayama, Y., Sakaguchi, K., Kojima, T., et al. (2017). Angiogenesis in newly regenerated bone by secretomes of human mesenchymal stem cells. Maxillofac. Plast. Reconstr. Surg. 39 (1), 8. doi:10.1186/s40902-017-0106-4
Katagiri, W., Osugi, M., Kawai, T., and Hibi, H. (2016). First-in-human study and clinical case reports of the alveolar bone regeneration with the secretome from human mesenchymal stem cells. Head. Face Med. 12, 5. doi:10.1186/s13005-016-0101-5
Katuchova, J., Harvanova, D., Spakova, T., Kalanin, R., Farkas, D., Durny, P., et al. (2015). Mesenchymal stem cells in the treatment of type 1 diabetes mellitus. Endocr. Pathol. 26 (2), 95–103. doi:10.1007/s12022-015-9362-y
Keshtkar, S., Kaviani, M., Sarvestani, F. S., Ghahremani, M. H., Aghdaei, M. H., Al-Abdullah, I. H., et al. (2020). Exosomes derived from human mesenchymal stem cells preserve mouse islet survival and insulin secretion function. EXCLI J. 19, 1064–1080. doi:10.17179/excli2020-2451
Khan, I., Zhang, L., Mohammed, M., Archer, F. E., Abukharmah, J., Yuan, Z., et al. (2015). Effects of Wharton's jelly-derived mesenchymal stem cells on neonatal neutrophils. J. Inflamm. Res. 8, 1–8. doi:10.2147/JIR.S71987
Kim, D. W., Staples, M., Shinozuka, K., Pantcheva, P., Kang, S. D., and Borlongan, C. V. (2013). Wharton's jelly-derived mesenchymal stem cells: Phenotypic characterization and optimizing their therapeutic potential for clinical applications. Int. J. Mol. Sci. 14 (6), 11692–11712. doi:10.3390/ijms140611692
Kim, H. K., Lee, S. G., Lee, S. W., Oh, B. J., Kim, J. H., Kim, J. A., et al. (2019). A Subset of paracrine factors as efficient biomarkers for Predicting vascular regenerative efficacy of mesenchymal stromal/stem cells. Stem Cells 37 (1), 77–88. doi:10.1002/stem.2920
Konala, V. B. R., Bhonde, R., and Pal, R. (2020). Secretome studies of mesenchymal stromal cells (MSCs) isolated from three tissue sources reveal subtle differences in potency. Vitro Cell Dev. Biol. Anim. 56 (9), 689–700. doi:10.1007/s11626-020-00501-1
Krafts, K. P. (2010). Tissue repair: The hidden drama. Organogenesis 6 (4), 225–233. doi:10.4161/org.6.4.12555
Kupcova Skalnikova, H. (2013). Proteomic techniques for characterisation of mesenchymal stem cell secretome. Biochimie 95 (12), 2196–2211. doi:10.1016/j.biochi.2013.07.015
Lakshmipathy, U., and Hart, R. P. (2008). Concise review: MicroRNA expression in multipotent mesenchymal stromal cells. Stem Cells 26 (2), 356–363. doi:10.1634/stemcells.2007-0625
Lakshmipathy, U., Love, B., Goff, L. A., Jörnsten, R., Graichen, R., Hart, R. P., et al. (2007). MicroRNA expression pattern of undifferentiated and differentiated human embryonic stem cells. Stem Cells Dev. 16 (6), 1003–1016. doi:10.1089/scd.2007.0026
Lavoie, J. R., and Rosu-Myles, M. (2013). Uncovering the secretes of mesenchymal stem cells. Biochimie 95 (12), 2212–2221. doi:10.1016/j.biochi.2013.06.017
Le Blanc, K., Rasmusson, I., Götherström, C., Seidel, C., Sundberg, B., Sundin, M., et al. (2004). Mesenchymal stem cells inhibit the expression of CD25 (interleukin-2 receptor) and CD38 on phytohaemagglutinin-activated lymphocytes. Scand. J. Immunol. 60 (3), 307–315. doi:10.1111/j.0300-9475.2004.01483.x
Li, B., Zheng, Y. W., Sano, Y., and Taniguchi, H. (2011). Evidence for mesenchymal-epithelial transition associated with mouse hepatic stem cell differentiation. PLoS One 6 (2), e17092. doi:10.1371/journal.pone.0017092
Li, C. X., Song, J., Li, X., Zhang, T., and Li, Z. M. (2020). Circular RNA 0001273 in exosomes derived from human umbilical cord mesenchymal stem cells (UMSCs) in myocardial infarction. Eur. Rev. Med. Pharmacol. Sci. 24 (19), 10086–10095. doi:10.26355/eurrev_202010_23228
Li, M., Soder, R., Abhyankar, S., Abdelhakim, H., Braun, M. W., Trinidad, C. V., et al. (2021). WJMSC-derived small extracellular vesicle enhance T cell suppression through PD-L1. J. Extracell. Vesicles 10 (4), e12067. doi:10.1002/jev2.12067
Li, W., Ren, G., Huang, Y., Su, J., Han, Y., Li, J., et al. (2012). Mesenchymal stem cells: A double-edged sword in regulating immune responses. Cell Death Differ. 19 (9), 1505–1513. doi:10.1038/cdd.2012.26
Liau, L. L., Ruszymah, B. H. I., Ng, M. H., and Law, J. X. (2020). Characteristics and clinical applications of Wharton's jelly-derived mesenchymal stromal cells. Curr. Res. Transl. Med. 68 (1), 5–16. doi:10.1016/j.retram.2019.09.001
Liedtke, C., Nevzorova, Y. A., Luedde, T., Zimmermann, H., Kroy, D., Strnad, P., et al. (2022). Liver fibrosis—from mechanisms of injury to modulation of disease. Front. Med. 8, 814496. doi:10.3389/fmed.2021.814496
Lin, S. Z., Chang, Y. J., Liu, J. W., Chang, L. F., Sun, L. Y., Li, Y. S., et al. (2010). Transplantation of human Wharton's Jelly-derived stem cells alleviates chemically induced liver fibrosis in rats. Cell Transpl. 19 (11), 1451–1463. doi:10.3727/096368910X514198
Liu, G., David, B. T., Trawczynski, M., and Fessler, R. G. (2020). Advances in pluripotent stem cells: History, mechanisms, Technologies, and applications. Stem Cell Rev. Rep. 16 (1), 3–32. doi:10.1007/s12015-019-09935-x
Liu, M., Wang, J., Liu, M., Hu, X., and Xu, J. (2015). Study of immunomodulatory function of exosomes derived from human umbilical cord mesenchymal stem cells. Zhonghua Yi Xue Za Zhi 95 (32), 2630–2633.
Liu, S., Yuan, M., Hou, K., Zhang, L., Zheng, X., Zhao, B., et al. (2012). Immune characterization of mesenchymal stem cells in human umbilical cord Wharton’s jelly and derived cartilage cells. Cell. Immunol. 278 (1), 35–44. doi:10.1016/j.cellimm.2012.06.010
Liu, X., Zheng, P., Wang, X., Dai, G., Cheng, H., Zhang, Z., et al. (2014). A preliminary evaluation of efficacy and safety of Wharton's jelly mesenchymal stem cell transplantation in patients with type 2 diabetes mellitus. Stem Cell Res. Ther. 5 (2), 57. doi:10.1186/scrt446
Lo Iacono, M., Russo, E., Anzalone, R., Baiamonte, E., Alberti, G., Gerbino, A., et al. (2018). Wharton's jelly mesenchymal stromal cells support the expansion of cord blood-derived CD34(+) cells Mimicking a hematopoietic niche in a direct cell-cell contact culture system. Cell Transpl. 27 (1), 117–129. doi:10.1177/0963689717737089
Lu, D., Xu, Y., Liu, Q., and Zhang, Q. (2021). Mesenchymal stem cell-macrophage crosstalk and maintenance of inflammatory microenvironment homeostasis. Front. Cell Dev. Biol. 9, 681171. doi:10.3389/fcell.2021.681171
Luz-Crawford, P., Jorgensen, C., and Djouad, F. (2017). Mesenchymal stem cells direct the immunological fate of macrophages. Results problems cell Differ. 62, 61–72. doi:10.1007/978-3-319-54090-0_4
Ma, S., Xie, N., Li, W., Yuan, B., Shi, Y., and Wang, Y. (2014). Immunobiology of mesenchymal stem cells. Cell Death Differ. 21 (2), 216–225. doi:10.1038/cdd.2013.158
Mahmood, A., Lu, D., Lu, M., and Chopp, M. (2003). Treatment of traumatic brain injury in adult rats with intravenous administration of human bone marrow stromal cells. Neurosurgery 53 (3), 697–702. discussion 702-693. doi:10.1227/01.neu.0000079333.61863.aa
Marijic, P., Schwarzkopf, L., Schwettmann, L., Ruhnke, T., Trudzinski, F., and Kreuter, M. (2021). Pirfenidone vs. nintedanib in patients with idiopathic pulmonary fibrosis: A retrospective cohort study. Respir. Res. 22 (1), 268. doi:10.1186/s12931-021-01857-y
Maron-Gutierrez, T., Laffey, J. G., Pelosi, P., and Rocco, P. R. (2014). Cell-based therapies for the acute respiratory distress syndrome. Curr. Opin. Crit. Care 20 (1), 122–131. doi:10.1097/MCC.0000000000000061
Martinez, F. J., Collard, H. R., Pardo, A., Raghu, G., Richeldi, L., Selman, M., et al. (2017). Idiopathic pulmonary fibrosis. Nat. Rev. Dis. Prim. 3 (1), 17074. doi:10.1038/nrdp.2017.74
Mathieu, M., Névo, N., Jouve, M., Valenzuela, J. I., Maurin, M., Verweij, F. J., et al. (2021). Specificities of exosome versus small ectosome secretion revealed by live intracellular tracking of CD63 and CD9. Nat. Commun. 12 (1), 4389. doi:10.1038/s41467-021-24384-2
McVicker, B. L., and Bennett, R. G. (2017). Novel anti-fibrotic therapies. Front. Pharmacol. 8, 318. doi:10.3389/fphar.2017.00318
Mendes, S. C., Robin, C., and Dzierzak, E. (2005). Mesenchymal progenitor cells localize within hematopoietic sites throughout ontogeny. Development 132 (5), 1127–1136. doi:10.1242/dev.01615
Meneghin, A., and Hogaboam, C. M. (2007). Infectious disease, the innate immune response, and fibrosis. J. Clin. Invest 117 (3), 530–538. doi:10.1172/JCI30595
Migliaccio, G., Migliaccio, A. R., Petti, S., Mavilio, F., Russo, G., Lazzaro, D., et al. (1986). Human embryonic hemopoiesis. Kinetics of progenitors and precursors underlying the yolk sac----liver transition. J. Clin. Invest 78 (1), 51–60. doi:10.1172/JCI112572
Mirabdollahi, M., Haghjooy Javanmard, S., and Sadeghi-Aliabadi, H. (2019). In vitro assessment of cytokine expression profile of MCF-7 cells in response to hWJ-MSCs secretome. Adv. Pharm. Bull. 9 (4), 649–654. doi:10.15171/apb.2019.075
Miyano, K., Ikehata, M., Ohshima, K., Yoshida, Y., Nose, Y., Yoshihara, S-i., et al. (2022). Intravenous administration of human mesenchymal stem cells derived from adipose tissue and umbilical cord improves neuropathic pain via suppression of neuronal damage and anti-inflammatory actions in rats. PLOS ONE 17 (2), e0262892. doi:10.1371/journal.pone.0262892
Moreira, A., Kahlenberg, S., and Hornsby, P. (2017). Therapeutic potential of mesenchymal stem cells for diabetes. J. Mol. Endocrinol. 59 (3), R109–R120. doi:10.1530/JME-17-0117
Mrahleh, M. A-, Matar, S., Jafar, H., Wehaibi, S., Aslam, N., and Awidi, A. (2021). Human Wharton’s jelly-derived mesenchymal stromal cells primed by tumor necrosis factor-α and interferon-γ modulate the innate and Adaptive immune cells of type 1 diabetic patients. Front. Immunol. 12, 732549. doi:10.3389/fimmu.2021.732549
Mukai, T., Tojo, A., and Nagamura-Inoue, T. (2018). Umbilical cord-derived mesenchymal stromal cells contribute to neuroprotection in neonatal Cortical neurons damaged by Oxygen-glucose Deprivation. Front. Neurol. 9, 466. doi:10.3389/fneur.2018.00466
Munoz-Perez, E., Gonzalez-Pujana, A., Igartua, M., Santos-Vizcaino, E., and Hernandez, R. M. (2021). Mesenchymal stromal cell secretome for the treatment of immune-mediated inflammatory diseases: Latest Trends in isolation, content Optimization and delivery Avenues. Pharmaceutics 13 (11), 1802. doi:10.3390/pharmaceutics13111802
Murphy, J. M., Fink, D. J., Hunziker, E. B., and Barry, F. P. (2003). Stem cell therapy in a caprine model of osteoarthritis. Arthritis Rheum. 48 (12), 3464–3474. doi:10.1002/art.11365
Mushahary, D., Spittler, A., Kasper, C., Weber, V., and Charwat, V. (2018). Isolation, cultivation, and characterization of human mesenchymal stem cells. Cytom. A 93 (1), 19–31. doi:10.1002/cyto.a.23242
Mussano, F., Genova, T., Corsalini, M., Schierano, G., Pettini, F., Di Venere, D., et al. (2017). Cytokine, chemokine, and growth factor profile characterization of undifferentiated and Osteoinduced human adipose-derived stem cells. Stem Cells Int. 2017, 6202783. doi:10.1155/2017/6202783
Muzes, G., and Sipos, F. (2022). Mesenchymal stem cell-derived secretome: A potential therapeutic option for Autoimmune and immune-mediated inflammatory diseases. Cells 11 (15), 2300. doi:10.3390/cells11152300
Najar, M., Fayyad-Kazan, M., Meuleman, N., Bron, D., Fayyad-Kazan, H., and Lagneaux, L. (2018). Immunological impact of Wharton's Jelly mesenchymal stromal cells and natural killer cell co-culture. Mol. Cell Biochem. 447 (1-2), 111–124. doi:10.1007/s11010-018-3297-9
Nakajima, H., Uchida, K., Guerrero, A. R., Watanabe, S., Sugita, D., Takeura, N., et al. (2012). Transplantation of mesenchymal stem cells promotes an alternative pathway of macrophage activation and functional recovery after spinal cord injury. J. neurotrauma 29 (8), 1614–1625. doi:10.1089/neu.2011.2109
Nekanti, U., Rao, V. B., Bahirvani, A. G., Jan, M., Totey, S., and Ta, M. (2010). Long-term expansion and pluripotent marker array analysis of Wharton's jelly-derived mesenchymal stem cells. Stem Cells Dev. 19 (1), 117–130. doi:10.1089/scd.2009.0177
Newell, L. F., Deans, R. J., and Maziarz, R. T. (2014). Adult adherent stromal cells in the management of graft-versus-host disease. Expert Opin. Biol. Ther. 14 (2), 231–246. doi:10.1517/14712598.2014.866648
Nooshabadi, V. T., Mardpour, S., Yousefi-Ahmadipour, A., Allahverdi, A., Izadpanah, M., Daneshimehr, F., et al. (2018). The extracellular vesicles-derived from mesenchymal stromal cells: A new therapeutic option in regenerative medicine. J. Cell Biochem. 119 (10), 8048–8073. doi:10.1002/jcb.26726
Oostendorp, R. A., Harvey, K. N., Kusadasi, N., de Bruijn, M. F., Saris, C., Ploemacher, R. E., et al. (2002). Stromal cell lines from mouse aorta-gonads-mesonephros subregions are potent supporters of hematopoietic stem cell activity. Blood 99 (4), 1183–1189. doi:10.1182/blood.v99.4.1183
Oskowitz, A., McFerrin, H., Gutschow, M., Carter, M. L., and Pochampally, R. (2011). Serum-deprived human multipotent mesenchymal stromal cells (MSCs) are highly angiogenic. Stem Cell Res. 6 (3), 215–225. doi:10.1016/j.scr.2011.01.004
Otte, J. M., Rosenberg, I. M., and Podolsky, D. K. (2003). Intestinal myofibroblasts in innate immune responses of the intestine. Gastroenterology 124 (7), 1866–1878. doi:10.1016/s0016-5085(03)00403-7
Paliwal, S., Chaudhuri, R., Agrawal, A., and Mohanty, S. (2018). Human tissue-specific MSCs demonstrate differential mitochondria transfer abilities that may determine their regenerative abilities. Stem Cell Res. Ther. 9 (1), 298. doi:10.1186/s13287-018-1012-0
Pant, T., Juric, M., Bosnjak, Z. J., and Dhanasekaran, A. (2021). Recent insight on the non-coding RNAs in mesenchymal stem cell-derived exosomes: Regulatory and therapeutic role in regenerative medicine and tissue Engineering. Front. Cardiovasc. Med. 8, 737512. doi:10.3389/fcvm.2021.737512
Panwar, U., Mishra, K., Patel, P., Bharadva, S., Vaniawala, S., Shah, A., et al. (2021). Assessment of long-term in vitro Multiplied human Wharton's jelly-derived mesenchymal stem cells prior to their Use in clinical administration. Cells Tissues Organs 210 (4), 239–249. doi:10.1159/000517423
Pappa, K. I., and Anagnou, N. P. (2009). Novel sources of fetal stem cells: Where do they fit on the developmental continuum? Regen. Med. 4 (3), 423–433. doi:10.2217/rme.09.12
Park, K. S., Kim, Y. S., Kim, J. H., Choi, B., Kim, S. H., Tan, A. H., et al. (2010). Trophic molecules derived from human mesenchymal stem cells enhance survival, function, and angiogenesis of isolated islets after transplantation. Transplantation 89 (5), 509–517. doi:10.1097/TP.0b013e3181c7dc99
Periera-Simon, S., Xia, X., Catanuto, P., Coronado, R., Kurtzberg, J., Bellio, M., et al. (2021). Anti-fibrotic effects of different sources of MSC in bleomycin-induced lung fibrosis in C57BL6 male mice. Respirol. Carlt. Vic. 26 (2), 161–170. doi:10.1111/resp.13928
Pittenger, M. F., Mackay, A. M., Beck, S. C., Jaiswal, R. K., Douglas, R., Mosca, J. D., et al. (1999). Multilineage potential of adult human mesenchymal stem cells. Science 284 (5411), 143–147. doi:10.1126/science.284.5411.143
Planat-Benard, V., Varin, A., and Casteilla, L. (2021). MSCs and inflammatory cells crosstalk in regenerative medicine: Concerted actions for Optimized Resolution Driven by Energy metabolism. Front. Immunol. 12, 626755. doi:10.3389/fimmu.2021.626755
Pochon, C., Notarantonio, A. B., Laroye, C., Reppel, L., Bensoussan, D., Bertrand, A., et al. (2022). Wharton's jelly-derived stromal cells and their cell therapy applications in allogeneic haematopoietic stem cell transplantation. J. Cell Mol. Med. 26 (5), 1339–1350. doi:10.1111/jcmm.17105
Prasanna, S. J., Gopalakrishnan, D., Shankar, S. R., and Vasandan, A. B. (2010). Pro-inflammatory cytokines, IFNgamma and TNFalpha, influence immune properties of human bone marrow and Wharton jelly mesenchymal stem cells differentially. PLoS One 5 (2), e9016. doi:10.1371/journal.pone.0009016
Praveen Kumar, L., Kandoi, S., Misra, R., Vijayalakshmi, S., Rajagopal, K., and Verma, R. S. (2019). The mesenchymal stem cell secretome: A new paradigm towards cell-free therapeutic mode in regenerative medicine. Cytokine Growth Factor Rev. 46, 1–9. doi:10.1016/j.cytogfr.2019.04.002
Qi, Y., Ma, J., Li, S., and Liu, W. (2019). Applicability of adipose-derived mesenchymal stem cells in treatment of patients with type 2 diabetes. Stem Cell Res. Ther. 10 (1), 274. doi:10.1186/s13287-019-1362-2
Qiu, B., Wei, F., Sun, X., Wang, X., Duan, B., Shi, C., et al. (2014). Measurement of hydroxyproline in collagen with three different methods. Mol. Med. Rep. 10 (2), 1157–1163. doi:10.3892/mmr.2014.2267
Quan, T. E., Cowper, S. E., and Bucala, R. (2006). The role of circulating fibrocytes in fibrosis. Curr. Rheumatol. Rep. 8 (2), 145–150. doi:10.1007/s11926-006-0055-x
Rajan, T. S., Diomede, F., Bramanti, P., Trubiani, O., and Mazzon, E. (2017). Conditioned medium from human gingival mesenchymal stem cells protects motor-neuron-like NSC-34 cells against scratch-injury-induced cell death. Int. J. Immunopathol. Pharmacol. 30 (4), 383–394. doi:10.1177/0394632017740976
Ranganath, S. H., Levy, O., Inamdar, M. S., and Karp, J. M. (2012). Harnessing the mesenchymal stem cell secretome for the treatment of cardiovascular disease. Cell stem cell 10 (3), 244–258. doi:10.1016/j.stem.2012.02.005
Raut, A., and Khanna, A. (2016). Enhanced expression of hepatocyte-specific microRNAs in valproic acid mediated hepatic trans-differentiation of human umbilical cord derived mesenchymal stem cells. Exp. Cell Res. 343 (2), 237–247. doi:10.1016/j.yexcr.2016.03.015
Rengasamy, M., Singh, G., Fakharuzi, N. A., Siddikuzzaman, , Balasubramanian, S., Swamynathan, P., et al. (2017). Transplantation of human bone marrow mesenchymal stromal cells reduces liver fibrosis more effectively than Wharton’s jelly mesenchymal stromal cells. Stem Cell Res. Ther. 8 (1), 143. doi:10.1186/s13287-017-0595-1
Rosenbloom, J., Macarak, E., Piera-Velazquez, S., and Jimenez, S. A. (2017). Human fibrotic diseases: Current challenges in fibrosis research. Methods Mol. Biol. 1627, 1–23. doi:10.1007/978-1-4939-7113-8_1
Rushkevich, Y. N., Kosmacheva, S. M., Zabrodets, G. V., Ignatenko, S. I., Goncharova, N. V., Severin, I. N., et al. (2015). The Use of autologous mesenchymal stem cells for cell therapy of patients with amyotrophic lateral sclerosis in Belarus. Bull. Exp. Biol. Med. 159 (4), 576–581. doi:10.1007/s10517-015-3017-3
Russo, F. P., Alison, M. R., Bigger, B. W., Amofah, E., Florou, A., Amin, F., et al. (2006). The bone marrow functionally contributes to liver fibrosis. Gastroenterology 130 (6), 1807–1821. doi:10.1053/j.gastro.2006.01.036
Rustad, K. C., and Gurtner, G. C. (2012). Mesenchymal stem cells home to sites of injury and inflammation. Adv. wound care 1 (4), 147–152. doi:10.1089/wound.2011.0314
Saleh, M., Fotook Kiaei, S. Z., and Kavianpour, M. (2022a). Application of Wharton jelly-derived mesenchymal stem cells in patients with pulmonary fibrosis. Stem Cell Res. Ther. 13 (1), 71. doi:10.1186/s13287-022-02746-x
Saleh, M., Vaezi, A. A., Aliannejad, R., Sohrabpour, A. A., Kiaei, S. Z. F., Shadnoush, M., et al. (2021). Cell therapy in patients with COVID-19 using Wharton's jelly mesenchymal stem cells: A phase 1 clinical trial. Stem Cell Res. Ther. 12 (1), 410. doi:10.1186/s13287-021-02483-7
Saleh, M., Vaezi, A. A., Sohrabpour, A. A., Barkhordar, M., Aghaghazvini, L., Alijani, N., et al. (2022b). Wharton's jelly-mesenchymal stem cells treatment for severe COVID 19 patients: 1-year follow-up. Gene Rep. 29, 101691. doi:10.1016/j.genrep.2022.101691
Salehipour Bavarsad, S., Jalali, M. T., Bijan Nejad, D., Alypoor, B., Babaahmadi Rezaei, H., and Mohammadtaghvaei, N. (2022). TGFβ1-Pretreated exosomes of Wharton jelly mesenchymal stem cell as a therapeutic strategy for improving liver fibrosis. Hepat. Mon. 22 (1), e123416. doi:10.5812/hepatmon-123416
Sandonà, M., Di Pietro, L., Esposito, F., Ventura, A., Silini, A. R., Parolini, O., et al. (2021). Mesenchymal stromal cells and their secretome: New therapeutic perspectives for skeletal muscle regeneration. Front. Bioeng. Biotechnol. 9, 652970. doi:10.3389/fbioe.2021.652970
Sato, F., Tsuchiya, S., Meltzer, S. J., and Shimizu, K. (2011). MicroRNAs and epigenetics. Febs J. 278 (10), 1598–1609. doi:10.1111/j.1742-4658.2011.08089.x
Schuppan, D., Ruehl, M., Somasundaram, R., and Hahn, E. G. (2001). Matrix as a modulator of hepatic fibrogenesis. Semin. Liver Dis. 21 (3), 351–372. doi:10.1055/s-2001-17556
Sedgwick, A. E., and D'Souza-Schorey, C. (2018). The biology of extracellular microvesicles. Traffic (Copenhagen, Den. 19 (5), 319–327. doi:10.1111/tra.12558
Selkoe, D. J., and Hardy, J. (2016). The amyloid hypothesis of Alzheimer's disease at 25 years. EMBO Mol. Med. 8 (6), 595–608. doi:10.15252/emmm.201606210
Sharif, S., Ghahremani, M. H., and Soleimani, M. (2018). Delivery of Exogenous miR-124 to glioblastoma Multiform cells by Wharton's jelly mesenchymal stem cells Decreases cell proliferation and migration, and Confers chemosensitivity. Stem Cell Rev. Rep. 14 (2), 236–246. doi:10.1007/s12015-017-9788-3
Shi, Q., Sun, B., Wang, D., Zhu, Y., Zhao, X., Yang, X., et al. (2020). Circ6401, a novel circular RNA, is implicated in repair of the damaged endometrium by Wharton’s jelly-derived mesenchymal stem cells through regulation of the miR-29b-1-5p/RAP1B axis. Stem Cell Res. Ther. 11 (1), 520. doi:10.1186/s13287-020-02027-5
Shi, Y., Hu, G., Su, J., Li, W., Chen, Q., Shou, P., et al. (2010). Mesenchymal stem cells: A new strategy for immunosuppression and tissue repair. Cell Res. 20 (5), 510–518. doi:10.1038/cr.2010.44
Shin, S., Lee, J., Kwon, Y., Park, K. S., Jeong, J. H., Choi, S. J., et al. (2021). Comparative proteomic analysis of the mesenchymal stem cells secretome from adipose, bone marrow, placenta and Wharton's jelly. Int. J. Mol. Sci. 22 (2), 845. doi:10.3390/ijms22020845
Singh, R. P., Massachi, I., Manickavel, S., Singh, S., Rao, N. P., Hasan, S., et al. (2013). The role of miRNA in inflammation and autoimmunity. Autoimmun. Rev. 12 (12), 1160–1165. doi:10.1016/j.autrev.2013.07.003
Soder, R. P., Dawn, B., Weiss, M. L., Dunavin, N., Weir, S., Mitchell, J., et al. (2020). A phase I study to evaluate two Doses of Wharton's jelly-derived mesenchymal stromal cells for the treatment of de Novo high-risk or Steroid-Refractory acute graft versus host disease. Stem Cell Rev. Rep. 16 (5), 979–991. doi:10.1007/s12015-020-10015-8
Subramanian, A., Fong, C. Y., Biswas, A., and Bongso, A. (2015). Comparative characterization of cells from the various compartments of the human umbilical cord shows that the Wharton's jelly compartment provides the best source of clinically utilizable mesenchymal stem cells. PLoS One 10 (6), e0127992. doi:10.1371/journal.pone.0127992
Suk, K. T., and Kim, D. J. (2015). Staging of liver fibrosis or cirrhosis: The role of hepatic venous pressure gradient measurement. World J. hepatology 7 (3), 607–615. doi:10.4254/wjh.v7.i3.607
Sun, B., Shi, L., Shi, Q., Jiang, Y., Su, Z., Yang, X., et al. (2018). Circular RNAs are abundantly expressed and upregulated during repair of the damaged endometrium by Wharton’s jelly-derived mesenchymal stem cells. Stem Cell Res. Ther. 9 (1), 314. doi:10.1186/s13287-018-1046-3
Taghavi-Farahabadi, M., Mahmoudi, M., Rezaei, N., and Hashemi, S. M. (2021). Wharton’s jelly mesenchymal stem cells exosomes and conditioned media increased neutrophil lifespan and Phagocytosis capacity. Immunol. Investig. 50 (8), 1042–1057. doi:10.1080/08820139.2020.1801720
Tang, Y., Zhou, Y., and Li, H. J. (2021). Advances in mesenchymal stem cell exosomes: A review. Stem Cell Res. Ther. 12 (1), 71. doi:10.1186/s13287-021-02138-7
Tavian, M., Hallais, M. F., and Peault, B. (1999). Emergence of intraembryonic hematopoietic precursors in the pre-liver human embryo. Development 126 (4), 793–803. doi:10.1242/dev.126.4.793
Thery, C., Boussac, M., Veron, P., Ricciardi-Castagnoli, P., Raposo, G., Garin, J., et al. (2001). Proteomic analysis of dendritic cell-derived exosomes: A secreted subcellular compartment distinct from apoptotic vesicles. J. Immunol. 166 (12), 7309–7318. doi:10.4049/jimmunol.166.12.7309
Théry, C., Witwer, K. W., Aikawa, E., Alcaraz, M. J., Anderson, J. D., Andriantsitohaina, R., et al. (2018). Minimal information for studies of extracellular vesicles 2018 (MISEV2018): A position statement of the international society for extracellular vesicles and update of the MISEV2014 guidelines. J. Extracell. Vesicles 7 (1), 1535750. doi:10.1080/20013078.2018.1535750
Thomi, G., Joerger-Messerli, M., Haesler, V., Muri, L., Surbek, D., and Schoeberlein, A. (2019b). Intranasally administered exosomes from umbilical cord stem cells have preventive neuroprotective effects and contribute to functional recovery after perinatal brain injury. Cells 8 (8), 855. doi:10.3390/cells8080855
Thomi, G., Surbek, D., Haesler, V., Joerger-Messerli, M., and Schoeberlein, A. (2019a). Exosomes derived from umbilical cord mesenchymal stem cells reduce microglia-mediated neuroinflammation in perinatal brain injury. Stem Cell Res. Ther. 10 (1), 105. doi:10.1186/s13287-019-1207-z
Ting, Z., Zhi-Xin, Y., You-Wen, T., Fu-Ji, Y., Hui, S., Fei, M., et al. (2021). Exosomes derived from human umbilical cord Wharton's jelly mesenchymal stem cells ameliorate experimental lymphedema. Clin. Transl. Med. 11 (7), e384. doi:10.1002/ctm2.384
Tipnis, S., Viswanathan, C., and Majumdar, A. S. (2010). Immunosuppressive properties of human umbilical cord-derived mesenchymal stem cells: Role of B7-H1 and Ido. Immunol. Cell Biol. 88 (8), 795–806. doi:10.1038/icb.2010.47
Tjalsma, H., Bolhuis, A., Jongbloed, J. D., Bron, S., and van Dijl, J. M. (2000). Signal peptide-dependent protein transport in Bacillus subtilis: A genome-based survey of the secretome. Microbiol. Mol. Biol. Rev. 64 (3), 515–547. doi:10.1128/mmbr.64.3.515-547.2000
Tomasek, J. J., Gabbiani, G., Hinz, B., Chaponnier, C., and Brown, R. A. (2002). Myofibroblasts and mechano-regulation of connective tissue remodelling. Nat. Rev. Mol. Cell Biol. 3 (5), 349–363. doi:10.1038/nrm809
Tomé, M., López-Romero, P., Albo, C., Sepúlveda, J. C., Fernández-Gutiérrez, B., Dopazo, A., et al. (2011). miR-335 orchestrates cell proliferation, migration and differentiation in human mesenchymal stem cells. Cell Death Differ. 18 (6), 985–995. doi:10.1038/cdd.2010.167
Tsai, P. C., Fu, T. W., Chen, Y. M., Ko, T. L., Chen, T. H., Shih, Y. H., et al. (2009). The therapeutic potential of human umbilical mesenchymal stem cells from Wharton's jelly in the treatment of rat liver fibrosis. Liver Transplant. 15 (5), 484–495. doi:10.1002/lt.21715
Turinetto, V., Vitale, E., and Giachino, C. (2016). Senescence in human mesenchymal stem cells: Functional changes and implications in stem cell-based therapy. Int. J. Mol. Sci. 17 (7), 1164. doi:10.3390/ijms17071164
Uccelli, A., Moretta, L., and Pistoia, V. (2006). Immunoregulatory function of mesenchymal stem cells. Eur. J. Immunol. 36 (10), 2566–2573. doi:10.1002/eji.200636416
Uder, C., Brückner, S., Winkler, S., Tautenhahn, H. M., and Christ, B. (2018). Mammalian MSC from selected species: Features and applications. Cytom. A 93 (1), 32–49. doi:10.1002/cyto.a.23239
Udler, M. S., and Florez, J. C. (2017). “Chapter 14 - diabetes,” in Genomic and Precision medicine. Editor S. P. David Third Edition (Boston: Academic Press), 245–282.
van Buul, G. M., Villafuertes, E., Bos, P. K., Waarsing, J. H., Kops, N., Narcisi, R., et al. (2012). Mesenchymal stem cells secrete factors that inhibit inflammatory processes in short-term osteoarthritic synovium and cartilage explant culture. Osteoarthr. Cartil. 20 (10), 1186–1196. doi:10.1016/j.joca.2012.06.003
Van Linthout, S., Miteva, K., and Tschöpe, C. (2014). Crosstalk between fibroblasts and inflammatory cells. Cardiovasc. Res. 102 (2), 258–269. doi:10.1093/cvr/cvu062
Varaa, N., Azandeh, S., Khodabandeh, Z., and Gharravi, A. M. (2019). Wharton’s jelly mesenchymal stem cell: Various Protocols for isolation and differentiation of hepatocyte-like cells; Narrative review. Iran. J. Med. Sci. 44 (6), 437–448. doi:10.30476/ijms.2019.44952
Vega, A., Martín-Ferrero, M. A., Del Canto, F., Alberca, M., García, V., Munar, A., et al. (2015). Treatment of knee osteoarthritis with allogeneic bone marrow mesenchymal stem cells: A randomized controlled trial. Transplantation 99 (8), 1681–1690. doi:10.1097/TP.0000000000000678
Velnar, T., Bailey, T., and Smrkolj, V. (2009). The wound healing process: An overview of the cellular and molecular mechanisms. J. Int. Med. Res. 37 (5), 1528–1542. doi:10.1177/147323000903700531
Vieira Paladino, F., de Moraes Rodrigues, J., da Silva, A., and Goldberg, A. C. (2019). The immunomodulatory potential of Wharton's jelly mesenchymal stem/stromal cells. Stem Cells Int. 2019, 3548917. doi:10.1155/2019/3548917
Vizoso, F. J., Eiro, N., Cid, S., Schneider, J., and Perez-Fernandez, R. (2017). Mesenchymal stem cell secretome: Toward cell-free therapeutic strategies in regenerative medicine. Int. J. Mol. Sci. 18 (9), 1852. doi:10.3390/ijms18091852
Wagner, W., Horn, P., Castoldi, M., Diehlmann, A., Bork, S., Saffrich, R., et al. (2008). Replicative senescence of mesenchymal stem cells: A continuous and organized process. PLoS One 3 (5), e2213. doi:10.1371/journal.pone.0002213
Wang, H., Qiu, X., Ni, P., Qiu, X., Lin, X., Wu, W., et al. (2014). Immunological characteristics of human umbilical cord mesenchymal stem cells and the therapeutic effects of their transplantion on hyperglycemia in diabetic rats. Int. J. Mol. Med. 33 (2), 263–270. doi:10.3892/ijmm.2013.1572
Wang, J., Liu, Y., Ding, H., Shi, X., and Ren, H. (2021b). Mesenchymal stem cell-secreted prostaglandin E(2) ameliorates acute liver failure via attenuation of cell death and regulation of macrophage polarization. Stem Cell Res. Ther. 12 (1), 15. doi:10.1186/s13287-020-02070-2
Wang, L-T., Liu, K-J., Sytwu, H-K., Yen, M-L., and Yen, B. L. (2021a). Advances in mesenchymal stem cell therapy for immune and inflammatory diseases: Use of cell-free products and human pluripotent stem cell-derived mesenchymal stem cells. Stem Cells Transl. Med. 10 (9), 1288–1303. doi:10.1002/sctm.21-0021
Wang, S., Lei, B., Zhang, E., Gong, P., Gu, J., He, L., et al. (2022). Targeted therapy for inflammatory diseases with mesenchymal stem cells and their derived exosomes: From basic to Clinics. Int. J. nanomedicine 17, 1757–1781. doi:10.2147/IJN.S355366
Wang, S., Qu, X., and Zhao, R. C. (2012a). Clinical applications of mesenchymal stem cells. J. Hematol. Oncol. 5, 19. doi:10.1186/1756-8722-5-19
Wang, X. S., Gong, J. N., Yu, J., Wang, F., Zhang, X. H., Yin, X. L., et al. (2012b). MicroRNA-29a and microRNA-142-3p are regulators of myeloid differentiation and acute myeloid leukemia. Blood 119 (21), 4992–5004. doi:10.1182/blood-2011-10-385716
Wang, X. Y., Lan, Y., He, W. Y., Zhang, L., Yao, H. Y., Hou, C. M., et al. (2008). Identification of mesenchymal stem cells in aorta-gonad-mesonephros and yolk sac of human embryos. Blood 111 (4), 2436–2443. doi:10.1182/blood-2007-07-099333
Waterman, R. S., Tomchuck, S. L., Henkle, S. L., and Betancourt, A. M. (2010). A new mesenchymal stem cell (MSC) paradigm: Polarization into a pro-inflammatory MSC1 or an immunosuppressive MSC2 phenotype. PLoS One 5 (4), e10088. doi:10.1371/journal.pone.0010088
Weiss, M. L., Anderson, C., Medicetty, S., Seshareddy, K. B., Weiss, R. J., Vanderwerff, I., et al. (2008). Immune properties of human umbilical cord Wharton's jelly-derived cells. Stem Cells 26 (11), 2865–2874. doi:10.1634/stemcells.2007-1028
Wernig, G., Chen, S-Y., Cui, L., Van Neste, C., Tsai, J. M., Kambham, N., et al. (2017). Unifying mechanism for different fibrotic diseases. Proc. Natl. Acad. Sci. 114 (18), 4757–4762. doi:10.1073/pnas.1621375114
White, I. J., Bailey, L. M., Aghakhani, M. R., Moss, S. E., and Futter, C. E. (2006). EGF stimulates annexin 1-dependent inward vesiculation in a multivesicular endosome subpopulation. Embo J. 25 (1), 1–12. doi:10.1038/sj.emboj.7600759
Wickman, G., Julian, L., and Olson, M. F. (2012). How apoptotic cells aid in the removal of their own cold dead bodies. Cell Death Differ. 19 (5), 735–742. doi:10.1038/cdd.2012.25
Willis, B. C., duBois, R. M., and Borok, Z. (2006). Epithelial origin of myofibroblasts during fibrosis in the lung. Proc. Am. Thorac. Soc. 3 (4), 377–382. doi:10.1513/pats.200601-004TK
Willms, E., Cabanas, C., Mager, I., Wood, M. J. A., and Vader, P. (2018). Extracellular vesicle Heterogeneity: Subpopulations, isolation techniques, and Diverse functions in cancer progression. Front. Immunol. 9, 738. doi:10.3389/fimmu.2018.00738
Witwer, K. W., and Théry, C. (2019). Extracellular vesicles or exosomes? On primacy, precision, and popularity influencing a choice of nomenclature. J. Extracell. Vesicles 8 (1), 1648167. doi:10.1080/20013078.2019.1648167
Wolf, P. (1967). The nature and significance of platelet products in human plasma. Br. J. Haematol. 13 (3), 269–288. doi:10.1111/j.1365-2141.1967.tb08741.x
Wu, L. F., Wang, N. N., Liu, Y. S., and Wei, X. (2009). Differentiation of Wharton's jelly primitive stromal cells into insulin-producing cells in comparison with bone marrow mesenchymal stem cells. Tissue Eng. Part A 15 (10), 2865–2873. doi:10.1089/ten.TEA.2008.0579
Wu, Z. H., Ji, C. L., Li, H., Qiu, G. X., Gao, C. J., and Weng, X. S. (2013). Membrane microparticles and diseases. Eur. Rev. Med. Pharmacol. Sci. 17 (18), 2420–2427.
Wynn, T. A. (2008). Cellular and molecular mechanisms of fibrosis. J. Pathol. 214 (2), 199–210. doi:10.1002/path.2277
Wynn, T. A. (2007). Common and unique mechanisms regulate fibrosis in various fibroproliferative diseases. J. Clin. Invest 117 (3), 524–529. doi:10.1172/JCI31487
Wynn, T. A., and Ramalingam, T. R. (2012). Mechanisms of fibrosis: Therapeutic translation for fibrotic disease. Nat. Med. 18 (7), 1028–1040. doi:10.1038/nm.2807
Xie, Z., Hao, H., Tong, C., Cheng, Y., Liu, J., Pang, Y., et al. (2016). Human umbilical cord-derived mesenchymal stem cells elicit macrophages into an anti-inflammatory phenotype to alleviate insulin resistance in type 2 diabetic rats. Stem Cells 34 (3), 627–639. doi:10.1002/stem.2238
Xunian, Z., and Kalluri, R. (2020). Biology and therapeutic potential of mesenchymal stem cell-derived exosomes. Cancer Sci. 111 (9), 3100–3110. doi:10.1111/cas.14563
Yáñez-Mó, M., Siljander, P. R., Andreu, Z., Zavec, A. B., Borràs, F. E., Buzas, E. I., et al. (2015). Biological properties of extracellular vesicles and their physiological functions. J. Extracell. Vesicles 4, 27066. doi:10.3402/jev.v4.27066
Yang, S., Liu, P., Jiang, Y., Wang, Z., Dai, H., and Wang, C. (2021). Therapeutic applications of mesenchymal stem cells in idiopathic pulmonary fibrosis. Front. Cell Dev. Biol. 9, 639657. doi:10.3389/fcell.2021.639657
Yin, Y., Hao, H., Cheng, Y., Gao, J., Liu, J., Xie, Z., et al. (2018). The homing of human umbilical cord-derived mesenchymal stem cells and the subsequent modulation of macrophage polarization in type 2 diabetic mice. Int. Immunopharmacol. 60, 235–245. doi:10.1016/j.intimp.2018.04.051
Yoo, K. H., Jang, I. K., Lee, M. W., Kim, H. E., Yang, M. S., Eom, Y., et al. (2009). Comparison of immunomodulatory properties of mesenchymal stem cells derived from adult human tissues. Cell Immunol. 259 (2), 150–156. doi:10.1016/j.cellimm.2009.06.010
Zaborowski, M. P., Balaj, L., Breakefield, X. O., and Lai, C. P. (2015). Extracellular vesicles: Composition, biological Relevance, and methods of study. Bioscience 65 (8), 783–797. doi:10.1093/biosci/biv084
Zanjani, E. D., Ascensao, J. L., and Tavassoli, M. (1993). Liver-derived fetal hematopoietic stem cells selectively and preferentially home to the fetal bone marrow. Blood 81 (2), 399–404. doi:10.1182/blood.v81.2.399.399
Zeddou, M., Briquet, A., Relic, B., Josse, C., Malaise, M. G., Gothot, A., et al. (2010). The umbilical cord matrix is a better source of mesenchymal stem cells (MSC) than the umbilical cord blood. Cell Biol. Int. 34 (7), 693–701. doi:10.1042/CBI20090414
Zeisberg, E. M., Tarnavski, O., Zeisberg, M., Dorfman, A. L., McMullen, J. R., Gustafsson, E., et al. (2007). Endothelial-to-mesenchymal transition contributes to cardiac fibrosis. Nat. Med. 13 (8), 952–961. doi:10.1038/nm1613
Zhang, B., Pan, X., and Anderson, T. A. (2006). MicroRNA: A new player in stem cells. J. Cell Physiol. 209 (2), 266–269. doi:10.1002/jcp.20713
Zhang, T., Ma, S., Lv, J., Wang, X., Afewerky, H. K., Li, H., et al. (2021). The emerging role of exosomes in Alzheimer's disease. Ageing Res. Rev. 68, 101321. doi:10.1016/j.arr.2021.101321
Zhang, Y., Ding, J., Ren, S., Wang, W., Yang, Y., Li, S., et al. (2020). Intravenous infusion of human umbilical cord Wharton's jelly-derived mesenchymal stem cells as a potential treatment for patients with COVID-19 pneumonia. Stem Cell Res. Ther. 11 (1), 207. doi:10.1186/s13287-020-01725-4
Zhang, Y. N., Lie, P. C., and Wei, X. (2009). Differentiation of mesenchymal stromal cells derived from umbilical cord Wharton's jelly into hepatocyte-like cells. Cytotherapy 11 (5), 548–558. doi:10.1080/14653240903051533
Zhao, G., Liu, F., Liu, Z., Zuo, K., Wang, B., Zhang, Y., et al. (2020). MSC-derived exosomes attenuate cell death through suppressing AIF nucleus translocation and enhance cutaneous wound healing. Stem Cell Res. Ther. 11 (1), 174. doi:10.1186/s13287-020-01616-8
Zhao, M., Wang, L., Wang, M., Zhou, S., Lu, Y., Cui, H., et al. (2022). Targeting fibrosis: Mechanisms and clinical trials. Signal Transduct. Target. Ther. 7 (1), 206. doi:10.1038/s41392-022-01070-3
Zhdanova, D. Y., Poltavtseva, R. A., Svirshchevskaya, E. V., and Bobkova, N. V. (2021). Effect of intranasal administration of multipotent mesenchymal stromal cell exosomes on memory of mice in Alzheimer's disease model. Bull. Exp. Biol. Med. 170 (4), 575–582. doi:10.1007/s10517-021-05109-3
Zhou, H. L., Zhang, X. J., Zhang, M. Y., Yan, Z. J., Xu, Z. M., and Xu, R. X. (2016). Transplantation of human amniotic mesenchymal stem cells promotes functional recovery in a rat model of traumatic spinal cord injury. Neurochem. Res. 41 (10), 2708–2718. doi:10.1007/s11064-016-1987-9
Keywords: WJ-MSCs, secretome, exosome, Wharton’s jelly mesenchymal stem cells, extracellular vesicles, EVs
Citation: Drobiova H, Sindhu S, Ahmad R, Haddad D, Al-Mulla F and Al Madhoun A (2023) Wharton’s jelly mesenchymal stem cells: a concise review of their secretome and prospective clinical applications. Front. Cell Dev. Biol. 11:1211217. doi: 10.3389/fcell.2023.1211217
Received: 24 April 2023; Accepted: 13 June 2023;
Published: 27 June 2023.
Edited by:
Faezeh Shekari, Royan institute for Stem Cell Biology and Technology (RI-SCBT), IranReviewed by:
Ilaria Giusti, University of L'Aquila, ItalyDina H. Kassem, Ain Shams University, Egypt
Ramesh Bhonde, Dr. D. Y. Patil Vidyapeeth, India
Copyright © 2023 Drobiova, Sindhu, Ahmad, Haddad, Al-Mulla and Al Madhoun. This is an open-access article distributed under the terms of the Creative Commons Attribution License (CC BY). The use, distribution or reproduction in other forums is permitted, provided the original author(s) and the copyright owner(s) are credited and that the original publication in this journal is cited, in accordance with accepted academic practice. No use, distribution or reproduction is permitted which does not comply with these terms.
*Correspondence: Ashraf Al Madhoun, ashraf.madhoun@dasmaninstitute.org
†These authors have contributed equally to this work and share first authorship