Tumour-associated macrophages: versatile players in the tumour microenvironment
- 1Department of Anatomical and Cellular Pathology, State Key Laboratory of Translational Oncology, The Chinese University of Hong Kong, Shatin, Hong Kong SAR, China
- 2Department of Applied Social Sciences, The Hong Kong Polytechnic University, Kowloon, Hong Kong SAR, China
- 3Department of Paediatrics, The Chinese University of Hong Kong, Shatin, Hong Kong SAR, China
- 4Key Laboratory for Regenerative Medicine of the Ministry of Education of China, School of Biomedical Sciences, Faculty of Medicine, The Chinese University of Hong Kong, Shatin, Hong Kong SAR, China
- 5MOE Key Laboratory of Environment and Genes Related to Diseases, School of Basic Medical Sciences, Xi’an Jiaotong University, Xi’an, China
Tumour-Associated Macrophages (TAMs) are one of the pivotal components of the tumour microenvironment. Their roles in the cancer immunity are complicated, both pro-tumour and anti-cancer activities are reported, including not only angiogenesis, extracellular matrix remodeling, immunosuppression, drug resistance but also phagocytosis and tumour regression. Interestingly, TAMs are highly dynamic and versatile in solid tumours. They show anti-cancer or pro-tumour activities, and interplay between the tumour microenvironment and cancer stem cells and under specific conditions. In addition to the classic M1/M2 phenotypes, a number of novel dedifferentiation phenomena of TAMs are discovered due to the advanced single-cell technology, e.g., macrophage-myofibroblast transition (MMT) and macrophage-neuron transition (MNT). More importantly, emerging information demonstrated the potential of TAMs on cancer immunotherapy, suggesting by the therapeutic efficiency of the checkpoint inhibitors and chimeric antigen receptor engineered cells based on macrophages. Here, we summarized the latest discoveries of TAMs from basic and translational research and discussed their clinical relevance and therapeutic potential for solid cancers.
Introduction
Tumour microenvironment (TME) is crucial for cancer initiation, progression, and drug resistance. TME is formed by various fundamental constituents including stromal cells and immune cells (Cassetta et al., 2019; Li et al., 2023; Wang et al., 2023). Cancer development can be facilitated by tissue inflammation (Nost et al., 2021; Rajamaki et al., 2021). Despite the diverse inflammatory components in various cancer types (Cheng et al., 2021), increasing evidence demonstrated the importance of macrophages in the progression of solid cancers (Christofides et al., 2022). Macrophage is the key inflammatory effector cells, better understanding its roles may uncover effective therapeutic strategy for cancer (Coussens et al., 2013).
Interestingly, macrophages are versatile in tissues under inflammation including cancer (Maier et al., 2020; Vayrynen et al., 2021; Xue et al., 2021; Nalio Ramos et al., 2022). Their phenotypes and functions are broadly categorized into pro-inflammatory M1 and anti-inflammatory M2 (Cho et al., 2022; Zhou et al., 2022). M1 macrophages eliminate cancer cells by phagocytosis, antibody-dependent cytotoxicity, vascular damage, and tumour necrosis. M2 macrophages promote tumour growth and progression via enhancing cancer cell survival, angiogenesis and immune suppression (Zhao et al., 2020; Chen et al., 2021; Ren et al., 2022). Beyond M1/M2 polarization, new transition mechanisms for TAMs have been recently identified by single-cell bioinformatic studies including MMT (Tang et al., 2022a) and MNT (Tang et al., 2022b), their roles in cancer remain unclear.
Clinical studies highlight the crucial roles of macrophages in cancer therapy response and resistance, including chemotherapy, radiotherapy, and PDL1-based immunotherapy (Furuse et al., 2020; Liu et al., 2020). Moreover, clinical trials of macrophage-targeted therapies have been started such as the engineered mononuclear phagocytes (Brempelis et al., 2020) and chimeric antigen receptor macrophages (CAR-M) (Klichinsky et al., 2020; Wang et al., 2022), these therapeutic approaches stem from bench-top discoveries like recruitment and differentiation (Hannan et al., 2023), functional reprogramming (Willingham et al., 2012), and integration (Dang et al., 2021), highlighting the importance of basic research and preclinical study for the development of effective cancer treatment.
In this review, we systematically summarized the functional roles and underlying mechanisms of macrophages in TME for cancer formation and progression, their translational potential, and related studies on patients for overcoming the barriers of conventional cancer treatments as well as the latest immunotherapy resistance in the clinic. Finally, we also discussed the prospects and further directions of TAMs in the clinical development for cancer treatment.
Physiological roles of macrophages
Macrophages release cytokines and chemokines for recruiting immune cells for wound healing and blood vessel formation (Hernandez et al., 2022), including vascular endothelial growth factor (VEGF) (Lu et al., 2020) and transforming growth factor-beta (TGF-β) (Chung et al., 2018). Macrophages maintain tissue integrity (Mosser et al., 2021), clearing apoptotic cells (Dooling et al., 2023), debris (Kim et al., 2020), and pathogens (Nau et al., 2002) via cell-mediated phagocytosis, where the targets are recognized by pattern recognition receptors (PRRs) dependent mechanisms (Li and Wu, 2021) i.e., Toll-like receptors (TLRs) (Irizarry-Caro et al., 2020) and NOD-like receptors (NLRs) (Fekete et al., 2018; Frising et al., 2022).
Furthermore, macrophages are involved in innate and adaptive immune responses by recognizing pathogen-associated molecular patterns (PAMPs) (Greene et al., 2022) and damage-associated molecular patterns (DAMPs) (Serbulea et al., 2018; Neu et al., 2022) through PRRs. Activated macrophages produce pro-inflammatory cytokines, i.e., tumour necrosis factor-alpha (TNF-α) (Lee et al., 2021; Lechner et al., 2022; Tanito et al., 2023) and interleukin-12 (IL-12) (Luo et al., 2022; Pfirschke et al., 2022), to promote inflammation and activate other immune cells. Macrophages also process and present antigens to T cells via major histocompatibility complex (MHC) molecules aiding adaptive immune response (Mascarau et al., 2023; van Elsas et al., 2023). Interestingly, tissue-specific macrophages display unique functions. For example, alveolar macrophages in lung, express high levels of surfactant protein A (SP-A) (Bain and MacDonald, 2022; Garcia-Fojeda et al., 2022; Yau et al., 2023) and surfactant protein D (SP-D) receptors (Guo et al., 2019; Hsieh et al., 2023) for clearing inhaled particles and pathogens. Liver-resident macrophages, Kupffer cells, express various scavenger receptors (Taban et al., 2022), complement receptors (Wen et al., 2021), and Fc receptors (Pfefferle et al., 2023), filtering blood-borne pathogens (Zhao et al., 2022a), toxins (Kermanizadeh et al., 2019), and debris (Liu and Sun, 2023).
Macrophages are classified into M1 and M2 phenotypes (Guilliams and Svedberg, 2021; De Vlaminck et al., 2022). M1 macrophages express high level of pro-inflammatory cytokines like Interleukin-1β (IL-1β), Interleukin-6 (IL-6), IL-12, Interleukin-23 (IL-23), and TNF-α (Hou et al., 2018; Akhtari et al., 2021; Beyranvand Nejad et al., 2021; Gunassekaran et al., 2021) polarized by Th1 cytokines including GM-CSF, TNF-α, and interferon-gamma (IFN-γ) (Wu et al., 2022a; Zhao et al., 2022b; Cho et al., 2022; Zhang et al., 2023), whereas, M2 macrophages actively produce anti-inflammatory cytokines Interleukin-10 (IL-10) and TGF-β (Nagata et al., 2019; Yang et al., 2023a) and polarized by Th2 cytokines like Interleukin-4 (IL-4) and Interleukin-13 (IL-13) (Celik et al., 2020; Lundahl et al., 2022). For metabolism, M1 macrophages rely on glycolysis (Yu et al., 2020; Mouton et al., 2023), while M2 macrophages depend on oxidative phosphorylation (Xu et al., 2021a; Zhou et al., 2022). During tissue repair, macrophages switch from an M1-like to an M2-like phenotype (Kim et al., 2019a; Alhamdi et al., 2019; Kohno et al., 2021). Interestingly, M1/M2 homeostasis is disrupted by inhibition of aspartate-aminotransferase (Wu et al., 2020a) and N-glycosylation (Wu et al., 2020a; Hu et al., 2023), altering immune responses and tissue damage. Moreover, various polarization and activation markers coexist in tissues, and factors like the macrophage-inducible C-type lectin (MINCLE) (Maier et al., 2020; Xue et al., 2021) or TLRs (Vidyarthi et al., 2018; Zhou et al., 2022) impact their balance. TAMs play multifaceted roles in cancer progression that are both beneficial and detrimental, highlighting the dual nature of their involvement (Figure 1).
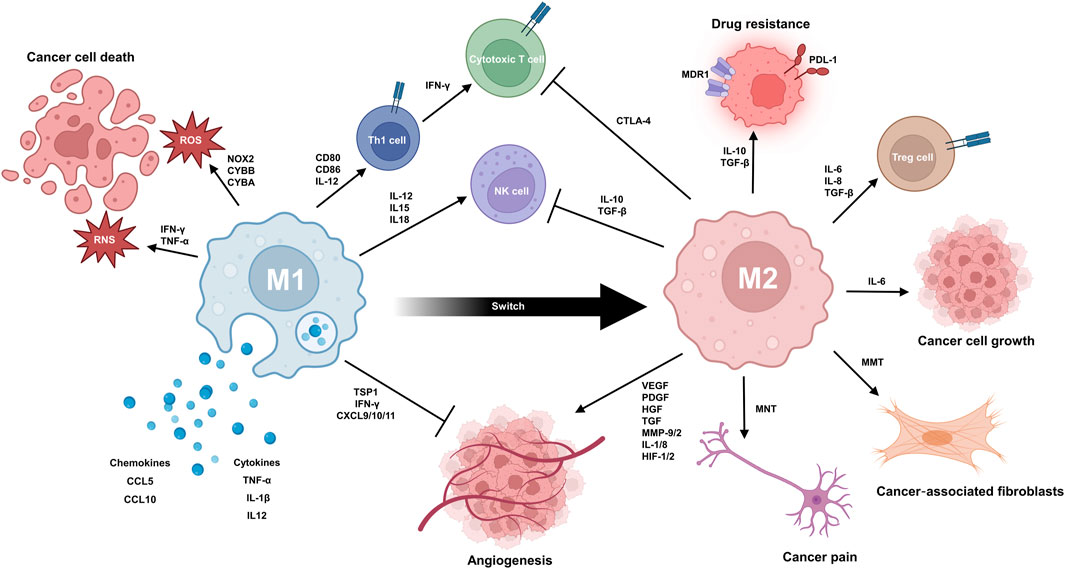
FIGURE 1. TAMs play a complex dual role in the progression of cancer. M1 TAMs contribute to the anticancer response via multiple mechanisms. They can produce reactive oxygen species (ROS) and reactive nitrogen species (RNS) to cause oxidative damage and kill cancer cells. The secretion of pro-inflammatory cytokines and chemokines (e.g., TNF-α, IL1B, IL12A/B, CCL5, and CXCL10) can mobilize other anticancer immune cells, like T cells and NK cells, into the TME. Anti-angiogenesis is promoted by secretion of thrombospondin-1 and angiostatic chemokines like CXCL9, CXCL10, and CXCL11. TAMs also express MHC class I and II molecules for antigen presentation to further priming and activation of T cells. The interaction between CD80/CD86 on TAMs and CD28 on T cells provides a second signal for T cell activation. M2 TAMs promote immunosuppression, angiogenesis, and tumour growth/metastasis while contributing to drug resistance. Immunosuppression involves secretion of TGF-β and IL-10, expression of PD-L1, and CCL22-induced Treg activation. In angiogenesis, TAMs secrete factors like VEGF, FGFs, PDGF, HGF, MMPs, and IL-8/1. During tumour growth and metastasis, M2 TAMs enhance proliferation, migration, and invasion. Factors like EGF, PDGF, VEGF, CCL-10, and MMPs play key roles. TAM can also undergo transformation to MNT and MMT, resulting in the generation of cancer pain and cancer-associated fibroblast. In drug resistance, TAM-derived TGF-β, IL-6/8, and PDGF stimulate survival pathways and enhance DNA repair in cancer cells. It is noteworthy that macrophages can switch from M1 phenotype to M2 phenotype during tissue repair.
Anticancer effects of TAMs
Reactive species production
M1 TAMs produce reactive oxygen species (ROS), mediated by NADPH oxidase (Fang et al., 2022; Tlili et al., 2023), causing cancer cell death. Activation by IFN-γ and TNF-α prompts TAMs to generate reactive nitrogen species (RNS) via nitric oxide synthase (iNOS) (Zhang et al., 2021a; Wei et al., 2022). Collectively, these ROS and RNS induce oxidative damage on cancer cells, leading to direct cancer cell-killing effect (Liang et al., 2019; Huang et al., 2022; Qi et al., 2022; Kidwell et al., 2023).
Pro-inflammatory cytokine and chemokine
TAMs secrete pro-inflammatory cytokines for mobilizing anticancer cells (e.g., T cells and natural killer cells) into TME, including TNF-α (Jiang et al., 2019; Kaplanov et al., 2019; Tu et al., 2021a), IL1B (interleukin-1 beta) (Revu et al., 2018), IL12A and IL12B (subunits of IL-12) (Yen et al., 2022). TAMs also produce chemokines, e.g., C-C Motif Chemokine Ligand 5 (CCL5) and C-X-C motif chemokine ligand 10 (CXCL10) to recruit and activate other immune cells to TME, driven by pro-inflammatory transcription factor NF-κB (nuclear factor kappa-light-chain-enhancer of activated B cells) (Taki et al., 2018). Furthermore, M1 macrophages produce IL-12, prompting CD4+ T cells towards Th1 phenotype (Zhao et al., 2022b), these Th1 cells will produce IFN-γ to activate cytotoxic CD8+ T cells in TME (Greaney et al., 2020; Liu et al., 2022). M1 macrophages also stimulate NK cell activation by IL-12, IL-15 and IL-18 (Mattiola et al., 2015).
Anti-angiogenesis
M1 macrophages secrete angiostatic factor thrombospondin-1(TSP1) (Yang et al., 2019; Kumar et al., 2020) for inhibiting angiogenesis by interacting with an endothelial cell receptor CD36 in various cancers, including hepatocellular carcinoma (Aburima et al., 2021). Moreover, M1 macrophages produce additional angiostatic chemokines to block vessel formation via CXCR3 (C-X-C Motif Chemokine Receptor 3) dependent mechanism, including CXCL9, 10, 11 (C-X-C Motif Chemokine Ligand 9, 10, 11) (Romagnani et al., 2004; Sahraei et al., 2019).
Antigen presentation
M1 macrophages express MHC class I and II molecules (Haloul et al., 2019; Ahmed and Ismail, 2020) to present cancer antigens, involving several genes, including MHC class I (Yao et al., 2020; Desterke et al., 2021; Piatakova et al., 2021) and II (He et al., 2021; Tang et al., 2022c; Scavuzzi et al., 2022). The interaction of MHC molecules with T cell receptors amplifies anti-tumour host immune response (Guerriero, 2019; Kawasaki et al., 2022). Interaction between CD80 and CD86 on the M1 macrophage and CD28 on the T cell also provides crucial second signal for T cell activation (Trzupek et al., 2020).
Pro-tumour effects of TAM
Immunosuppression
TAMs contribute to immunosuppression in TME, including lung adenocarcinoma (LUAD) and bladder cancer (BLCA). They inhibit the anticancer activities of NK cells primarily through producing TGF-β (Nunez et al., 2018) and IL-10 (Xu et al., 2022). TGF-β hampers NK cell cytotoxicity by downregulating NKG2D receptor expression (Lazarova and Steinle, 2019). IL-10 inhibits the production of the anticancer cytokine IFN-γ in NK cells (Wang et al., 2021a). TAMs in these diverse cancer types express programmed death-ligand 1 (PD-L1) (Sumitomo et al., 2019; Shinchi et al., 2022; Xia et al., 2022; Elomaa et al., 2023), which interacts with the PD-1 receptor on T cells (Pereira et al., 2023; Puig-Saus et al., 2023) and NK cells (Zhou et al., 2023a; van der Sluis et al., 2023), leading to their exhaustion and promoting tumour immune evasion. TAM-derived CCL22 (C-C Motif Chemokine Ligand 22) contributes to the recruitment and activation of regulatory T cells (Tregs) (Rapp et al., 2019; Chen et al., 2022a), inducing immunosuppression in TME (Kraaij et al., 2010; Erlandsson et al., 2019). TAMs also enhance immunosuppressive function of Tregs, promote the transition of conventional CD4+ T cells into Tregs (Morhardt et al., 2019; Saraiva et al., 2020; Maldonado et al., 2022), and activate myeloid-derived suppressor cells (MDSCs) via IL-10 (Yu et al., 2018; Yogev et al., 2022) and TGF-β (Becker et al., 2018; Astarita et al., 2023). Furthermore, TAMs express immune checkpoint molecule cytotoxic T-lymphocyte-associated protein 4 (CTLA-4) (Guan et al., 2021), interacting with CD80/CD86 of Tregs to amplify their immunosuppressive effects (Zappasodi et al., 2021; Kennedy et al., 2022).
Angiogenesis
TAMs play pivotal role in augmenting angiogenesis within the TME, integral to cancer progression (Cheng et al., 2021). Essential for tumor growth and metastasis (Liu et al., 2023a; Natale and Bocci, 2023), angiogenesis provides TME with necessary nutrients and oxygen, aiding in the growth of cancer cells (Schaaf et al., 2018; Lugano et al., 2020; Schito and Rey, 2020). TAMs secret factors for promoting angiogenesis, including VEGF (Schaaf et al., 2018), fibroblast growth factors (FGF1 and FGF2) (Schaaf et al., 2018; Im et al., 2020), platelet-derived growth factor (PDGF) (Ntokou et al., 2021), hepatocyte growth factor (HGF) (Choi et al., 2019; Dong et al., 2019), matrix metalloproteinases (MMP-9, MMP-2) (Diwanji and Bergmann, 2020; Tian et al., 2022), and cytokines like IL-8 and IL-1 (Liu et al., 2023b; Yang et al., 2023b). VEGF is crucial for tumoural angiogenesis (Lai et al., 2019; Hwang et al., 2020). Moreover, TAMs are concentrated in the hypoxic zones of tumours (Bai et al., 2022), where they upregulate the expression of numerous angiogenic genes including Hypoxia-inducible factors (HIF)-1 and −2 (Jeong et al., 2019; Cowman et al., 2020) for enhancing the production of angiogenic factors like VEGF in TME (Roda et al., 2012).
Cancer growth and metastasis
M2 TAMs promote primary tumour development and metastasis (Yao et al., 2018; Li et al., 2019a; Tu et al., 2021b). They increase tumour proliferation in breast cancer (Chen et al., 2022b; Zhou et al., 2023b), endometrial cancer (Xiao et al., 2020; Gu et al., 2021), and renal cell carcinoma (Xie et al., 2021; Ishii et al., 2022). Furthermore, M2 TAMs secrete Epidermal Growth Factor (EGF) (Zeng et al., 2019; Wu et al., 2020b), which binds to EGFR on cancer cells, for activating their growth signaling including MAPK/ERK (Liang et al., 2022) and PI3K/Akt pathways (Zhang et al., 2021b), promoting cell motility and invasion (Haque et al., 2019; Zeng et al., 2019; Onal et al., 2021). Growth Factor PDGF (Turrell et al., 2023) secreted from TAMs also contributes to tumour cell proliferation. Tumour metastasis is defining characteristic of advanced cancer stage, TAM-derived EGF accelerates metastasis by activating the EGFR-ERK signaling and inhibiting the expression of lncRNA LIMT (Zeng et al., 2019) in the epithelial ovarian cancer.
At the pre-metastasis stage, TAMs secrete VEGF, CCL-10 and MMPs, which remodel distant tissues to create pre-metastatic niche (Kim et al., 2019b; Winkler et al., 2020). TAMs release inflammatory factors TNF-α, IL-6, and IL-11 (Kaplanov et al., 2019; Yu et al., 2019; Beyranvand Nejad et al., 2021) to enhance cancer cell survival and proliferation by activating NF-κB and STAT3 pathways (Dorrington and Fraser, 2019; Balic et al., 2020). TGF-β from TAMs activates TGF receptors on cancer cells, initiating SMAD signaling for their growth (Chung et al., 2023; Lv et al., 2023). Importantly, TAM-derived TGF-β induces epithelial-to-mesenchymal transition (EMT) of cancer cells (Cai et al., 2019; Tiwari et al., 2021), allowing them to migrate into surrounding tissue and vasculature (Dongre and Weinberg, 2019; Wang et al., 2021b). Additionally, TAMs-secreted MMPs, such as MMP2 and MMP9 (Wang and Khalil, 2018; Liu et al., 2019; Muniz-Bongers et al., 2021), degrade the ECM in TME (Marigo et al., 2020), enabling metastasis into the bloodstream or lymphatic system (Winkler et al., 2020). TAMs produce chemokines like CCL18 and CCL22 (She et al., 2018; Kimura et al., 2019; Zhou et al., 2019; Chen et al., 2022a) to promote tumour cell migration. TAMs also release proteases like cathepsins (CTSB, CTSD) (Loeuillard et al., 2020; Shi et al., 2022) to stimulate tumour cells to produce tissue inhibitors of metalloproteinases, enhancing ECM degradation and metastasis (Bissinger et al., 2021).
TAMs transformation also contributes to cancer progression. Besides M1/M2 polarization, single-cell RNA-sequencing revealed new TAM phenomena. Macrophage to MNT, a process where TAMs transform into neuron-like cells contributing to the formation of cancer pain (Tang et al., 2022b). MMT, where TAMs trans-differentiate into myofibroblasts for increasing abundance of pro-tumour cancer-associated fibroblasts (CAFs) in TME, enhancing the progression of non-small-cell lung carcinoma (NSCLC) (Tang et al., 2022a).
Drug resistance
TAMs are associated with resistance of cancer therapy (Mantovani et al., 2022). TAM-derived TGF-β upregulates the expression of multidrug resistance protein 1 (MDR1) in cancer cells (Badmann et al., 2020), leading to drug resistance. TAMs secrete IL-6 and IL-8 (Ahmed et al., 2021; Radharani et al., 2022), associated with resistance to therapies including EGFR tyrosine kinase inhibitors. TAMs-secreted PDGF enhances DNA repair in cancer cells against radiation therapy (Sakama et al., 2021).
Interplay between TME and cancer stem cells
The dynamic relationship between the TME and cancer stem cells (CSCs) is central to understanding the roles of TAMs. CSCs, distinguished by their pronounced expression of stemness markers like SOX2, NANOG, and OCT4 (Zhou et al., 2021), actively drive self-renewal, differentiation, and are influenced by signals from TME (Yang et al., 2020). Key pathways such as TGF-β, Wnt, and Hedgehog (Li et al., 2019b; Zhu et al., 2019; Wu et al., 2022b) mold the genetic landscape of CSCs. The crosstalk between CSCs and TME involves factors including IL-6 (Orange et al., 2023), IL-8 (Sun et al., 2018), IL-1β (Eyre et al., 2019), MMPs (Jin and Jin, 2020), VEGF (Lopez de Andres et al., 2020), and TGF-β1 (Yuan et al., 2022), which are encapsulated within extracellular vehicles (EVs) (Su et al., 2021; Cao et al., 2022). Given the immunomodulatory role of CSCs, further studies are essential to understand the clinical implications.
Importantly, interaction between TAMs and CSCs fosters an immunosuppressive TME (Wu et al., 2023). CSCs promote macrophage recruitment and polarization by ILs, ECM, TGF-β, and periostin (Ning et al., 2018; Kesh et al., 2020; Taniguchi et al., 2020; Li et al., 2022a; Lin et al., 2022). Moreover, TAMs increase CD47 expression in pancreatic, liver and lung cancer stem cells (Cioffi et al., 2015; Liu et al., 2017; Ruiz-Blazquez et al., 2021). When linked to SIRPα on macrophages, CD47 expression protects CSCs against immune cell-mediated phagocytosis (Li et al., 2018). TAM-secreted factors also upregulate immunological checkpoints like PD-L1 (Muraoka et al., 2019; Pu and Ji, 2022). The intricate interplay between CSCs and TAMs creates immunosuppressive TME, enhancing the survival of CSC and hindering tumour eradication post-immunotherapy.
Macrophage-targeted antitumour therapy
TAMs are essential for cancer immunotherapy (Lin et al., 2019). Macrophage-targeted treatments often deplete macrophages, modify their phenotypes, or enhance antigen presentation activity of TAM (Cassetta and Pollard, 2018). Combined with chemotherapy, radiation, or immunotherapy, these techniques may increase host antitumor immunity. They have been studied in animal models and clinical studies with immunological checkpoints and other immunotherapies (Table 1).
Depletion of macrophages
TAM recruitment by CCL2 and CCR2 is critical to tumour invasion and metastasis (Xu et al., 2021b). CCL2-CCR2 signaling controls the supply of circulating inflammatory monocytes (Argyle and Kitamura, 2018) and inhibiting CCR2 keeps monocytes in bone marrow, reducing TAMs at cancer sites (Flores-Toro et al., 2020). Blocking CCL2-CCR2 axis also hinders TAM recruitment, decreasing tumour incidence and enhancing CD8+ T cells anti-tumour activity (Teng et al., 2017; Tu et al., 2020). Another target is CSF-1, which promotes monocyte and macrophage differentiation, proliferation, and function (Stanley and Chitu, 2014). Mouse models with CSF-1R inhibition had smaller tumors and better survival (Tan et al., 2021). Small molecule inhibitors of CSF1-R have also been shown to deplete some TAMs, enhancing tumour sensitivity to chemotherapy (O'Brien et al., 2021).
Alteration of macrophage phenotypes
TAMs change into a tumour-suppressing phenotype (Liu et al., 2021) which is a promising clinical strategy for cancer treatment. Inducing M1 macrophage phenotype through the use of selective class IIa HDAC inhibitors (Li et al., 2021a) enhances T cell responses to chemotherapy and immune checkpoint blockades (McCaw et al., 2019). The CD47/SIRP-α pathway is crucial for tumour immune escape, and blocking it enhances macrophages immune killing against tumours (Wang et al., 2020; Jia et al., 2021). Cancer immunotherapy research has also focused on anti-PD-1/PD-L1 treatment (Tomlins et al., 2023). TAMs, particularly M2 TAMs, express PD-L1 on their surface and contribute to immunosuppression by promoting T-cell apoptosis (Li et al., 2022b; Shinchi et al., 2022). In vitro-transcribed mRNA could stimulate effector molecule synthesis or cell reprogramming. mRNA in an injectable nanocarrier genetically reprogrammed TAMs into antitumour effectors. Nanoparticles formulated with mRNAs encoding the transcription factor interferon regulatory factor 5 (IRF5) and its activating kinase, inhibitor of NF-B kinase subunit-β (IKKβ), reversed the immunosuppressive TME and reprogrammed TAMs, regressing tumours in mouse cancer models (Zhang et al., 2019; Petty et al., 2021). The LILRB family, specifically LILRB2, is integral to the immune evasion strategies of cancer cells (Chen et al., 2018). LILRB2, an MHC-binding protein rich in TAMs, interacts with MHC class I molecules, which cancer cells often downregulate to dodge T cell recognition (Liu et al., 2023c). Blocking LILRB2 enhances macrophage pro-inflammatory and phagocytic activity. Its effect on macrophage activation and phagocytosis is unknown (Chen et al., 2018). MK-4830, an antibody against LILRB2, showed promising results in early trials with advanced-stage tumours (Siu et al., 2022). Responses correlated with the expression of pro-inflammatory cytokines and enhanced cytotoxic T cell-mediated anti-tumour immune response (Sharma et al., 2021). These approaches have been tested with other clinical used immunotherapies like immune checkpoints for their clinical potential with animal models and clinical trials.
Antigen presentation enhancement
Scavenger receptors on TAMs are becoming therapeutic targets for their role in promoting TME pro-inflammatory shifts. Scavenger receptor CD163 is associated to tumour progression in several malignancies but the mechanism is unclear (Xie et al., 2022). However, CD163+ macrophage depletion causes tumor regression and re-establish anti-PD1 treatment response (Etzerodt et al., 2019). Macrophage mannose receptor 1 (MRC1), also known as CD206, affects tumour immunity (Rahabi et al., 2020). Its activation induces immunosuppressive macrophages. Intriguingly, MRC1-binding peptide RP-182 converts TAMs into anti-tumour M1-like effector cells (Jaynes et al., 2020). The collagenous macrophage receptor (MARCO) is abundantly present on TAMs. Targeting MARCO potentially reprogrammes TAMs from tumour-supportive to pro-inflammatory effectors (Sa et al., 2020; La Fleur et al., 2021). Another scavenger receptor Clever 1 also suppresses macrophages and T helper 1 lymphocytes (Virtakoivu et al., 2021). Blocking it switches TAMs from immunosuppressive to pro-inflammatory (Viitala et al., 2019). Triggering receptor expressed on myeloid cells 2 (TREM2), upregulated on TAMs in human and mouse tumours, is a potential target (Katzenelenbogen et al., 2020; Molgora et al., 2020). Blocking TREM2+ macrophages limit tumour growth and augment anti-PD1 therapy (Binnewies et al., 2021). PSGL1, highly expressed in TAMs, represents a valuable target for TAMs re-education (Johnston et al., 2019). Using anti-PSGL1 monoclonal antibody potentially triggers a pro-inflammatory response in tumour tissues, exhibiting notable antitumour activity (DeRogatis et al., 2022; Lin et al., 2023).
Innovative strategies for TAM modulation
Recent strategies explore TAM modulation. One approach involves the engineering of T cells with chimeric antigen receptors (CAR) (Maalej et al., 2023) specifically tailored to recognize and eliminate TAMs. Research shows CAR T cells targeting macrophages are effective against various solid organ tumours, including ovarian and pancreatic cancer (Sanchez-Paulete et al., 2022). Eliminating M2-like FRβ+ TAMs in the murine models of ovarian cancer, colon cancer and melanoma TME through FR-specific CAR-T cells delay tumour progression and prolong life (Rodriguez-Garcia et al., 2021). These CAR-engineered T cells show potential in redirecting immune responses against the tumour. Another method focuses on harnessing invariant natural killer T (iNKT) cells (Li et al., 2021b). These cells possess innate and adaptive immune properties, CAR-iNKT cells use iNKT TCR/CD1d and CAR recognition to deplete TAMs and tumours (Simonetta et al., 2021). Recent studies harness iNKT cells to modulate TAMs, boosting antitumour responses. Other innate T cells, including MAIT, and γδT cells, have potential clinical applications as they target and eliminate TAMs (Li et al., 2022c). In synthesis, these innovative strategies signify a shift in tumour immunotherapy (Table 2).
Prospects of macrophages in cancer
TAMs are an important immune cell type that shapes TME properties. Targeting TAMs effectively blocks the progression of various cancer types. Moreover, popularity of single-cell RNA-sequencing analysis enhances the mechanistic study and preclinical research of TAMs in TME (Tang et al., 2020; Tang et al., 2021a; Chung et al., 2023). Dissecting the heterogeneity and regulatory mechanism of macrophages in cancer at single-cell resolution leads to the discovery of novel macrophage-specific therapeutics targets from the TME, for example, MMT and MNT (Xue et al., 2021; Tang et al., 2022a; Tang et al., 2022b). They are emphasizing the adaptive plasticity of macrophages. MMTs, derived from M2 TAMs with protumour activities, lead to the formation of CAFs. These CAFs are key in driving cancer progression (Chen and Song, 2019; Li et al., 2020). The roles of MMT-derived CAFs in functions, including adaptive immunity suppression, drug resistance, metastasis, and promoting cancer cell stemness warrant investigation. Conversely, MNTs highlight the transformation of TAMs into neuron-like entities, influencing de novo neurogenesis in the TME (Tang et al., 2022b) and contributing to cancer-associated pain (Shepherd et al., 2018). This transition, while prevalent in NSCLC, is also seen in other tumours, emphasizing its importance in cancer pain and tumour innervation (Tang et al., 2022b). Given the impact of cancer pain on quality of life, especially in patients with advanced stages of the disease (Wang et al., 2021c), understanding MNT is vital for pain management strategies. Notably, these transitions were found to be mediated by a Smad3-centric gene network in TAMs, highlighting the potential of macrophage-targeted Smad3 interventions as a promising therapeutic approach in cancer immunotherapy (Tang et al., 2017; Feng et al., 2018; Tang et al., 2021b; Tang et al., 2022b). These new findings lead to the development of effective therapeutic approaches to enhance the efficiency of conventional anticancer treatments as well as the latest immunotherapies which are not primary or secondary resistant in patients with solid cancers (Kim et al., 2019b; Kim et al., 2020; Tang et al., 2020; Chung et al., 2021; Xue et al., 2021). Besides, macrophages are considered as a primary target of anti-inflammatory therapy for cancer prevention, their therapeutic potential is explored by new trials worldwide (Tang et al., 2019; Lee et al., 2021; Tang et al., 2022d). Despite the challenges, a better understanding of the immunodynamics of TAM shows a substantial potential for improving the therapeutic efficiency and clinical outcomes of cancer patients in the future.
Author contributions
ZZJ: Writing–original draft, Writing–review and editing, Visualization. MK-KC: Writing–original draft, Writing–review and editing, Visualization. AS-WC: Data curation. K-TL: Writing–review and editing. XJ: Writing–review and editing. K-FT: Writing–review and editing. YW: Writing–review and editing. PM-KT: Writing–original draft, Writing–review & editing Conceptualization, Funding acquisition, Investigation, Resources, Supervision. Validation: All authors have read and agreed to the published version.
Funding
The authors declare financial support was received for the research, authorship, and/or publication of this article. This study was supported by the Research Grants Council of Hong Kong (14106518, 14111019, 14111720, and 24102723); RGC Postdoctoral Fellowship Scheme (PDFS2122-4S06); Hong Kong Government Health and Medical Research Fund (10210726); CU Medicine Passion for Perfection Scheme (PFP202210-004) and Faculty Innovation Award (4620528), CUHK Strategic Seed Funding for Collaborative Research Scheme (178896941), Direct Grant for Research (4054722), Postdoctoral Fellowship Scheme (NL/LT/PDFS 2022/0360/22lt and WW/PDFS 2023/0640/23en).
Conflict of interest
The authors declare that the research was conducted in the absence of any commercial or financial relationships that could be construed as a potential conflict of interest.
Publisher’s note
All claims expressed in this article are solely those of the authors and do not necessarily represent those of their affiliated organizations, or those of the publisher, the editors and the reviewers. Any product that may be evaluated in this article, or claim that may be made by its manufacturer, is not guaranteed or endorsed by the publisher.
References
Aburima, A., Berger, M., Spurgeon, B. E. J., Webb, B. A., Wraith, K. S., Febbraio, M., et al. (2021). Thrombospondin-1 promotes hemostasis through modulation of cAMP signaling in blood platelets. Blood 137 (5), 678–689. doi:10.1182/blood.2020005382
Ahmed, I., and Ismail, N. (2020). M1 and M2 macrophages polarization via mTORC1 influences innate immunity and outcome of ehrlichia infection. J. Cell. Immunol. 2 (3), 108–115. doi:10.33696/immunology.2.029
Ahmed, S., Mohamed, H. T., El-Husseiny, N., El Mahdy, M. M., Safwat, G., Diab, A. A., et al. (2021). IL-8 secreted by tumor associated macrophages contribute to lapatinib resistance in HER2-positive locally advanced breast cancer via activation of Src/STAT3/ERK1/2-mediated EGFR signaling. Biochim. Biophys. Acta Mol. Cell. Res. 1868 (6), 118995. doi:10.1016/j.bbamcr.2021.118995
Akhtari, M., Zargar, S. J., Vojdanian, M., Jamshidi, A., and Mahmoudi, M. (2021). Monocyte-derived and M1 macrophages from ankylosing spondylitis patients released higher TNF-α and expressed more IL1B in response to BzATP than macrophages from healthy subjects. Sci. Rep. 11 (1), 17842. doi:10.1038/s41598-021-96262-2
Alhamdi, J. R., Peng, T., Al-Naggar, I. M., Hawley, K. L., Spiller, K. L., and Kuhn, L. T. (2019). Controlled M1-to-M2 transition of aged macrophages by calcium phosphate coatings. Biomaterials 196, 90–99. doi:10.1016/j.biomaterials.2018.07.012
Argyle, D., and Kitamura, T. (2018). Targeting macrophage-recruiting chemokines as a novel therapeutic strategy to prevent the progression of solid tumors. Front. Immunol. 9, 2629. doi:10.3389/fimmu.2018.02629
Astarita, J. L., Dominguez, C. X., Tan, C., Guillen, J., Pauli, M. L., Labastida, R., et al. (2023). Treg specialization and functions beyond immune suppression. Clin. Exp. Immunol. 211 (2), 176–183. doi:10.1093/cei/uxac123
Badmann, S., Heublein, S., Mayr, D., Reischer, A., Liao, Y., Kolben, T., et al. (2020). M2 macrophages infiltrating epithelial ovarian cancer express MDR1: a feature that may account for the poor prognosis. Cells 9 (5), 1224. doi:10.3390/cells9051224
Bai, R., Li, Y., Jian, L., Yang, Y., Zhao, L., and Wei, M. (2022). The hypoxia-driven crosstalk between tumor and tumor-associated macrophages: mechanisms and clinical treatment strategies. Mol. Cancer 21 (1), 177. doi:10.1186/s12943-022-01645-2
Bain, C. C., and MacDonald, A. S. (2022). The impact of the lung environment on macrophage development, activation and function: diversity in the face of adversity. Mucosal Immunol. 15 (2), 223–234. doi:10.1038/s41385-021-00480-w
Balic, J. J., Albargy, H., Luu, K., Kirby, F. J., Jayasekara, W. S. N., Mansell, F., et al. (2020). STAT3 serine phosphorylation is required for TLR4 metabolic reprogramming and IL-1β expression. Nat. Commun. 11 (1), 3816. doi:10.1038/s41467-020-17669-5
Becker, W., Nagarkatti, M., and Nagarkatti, P. S. (2018). miR-466a targeting of TGF-β2 contributes to FoxP3+ regulatory T cell differentiation in a murine model of allogeneic transplantation. Front. Immunol. 9, 688. doi:10.3389/fimmu.2018.00688
Beyranvand Nejad, E., Labrie, C., van Elsas, M. J., Kleinovink, J. W., Mittrücker, H. W., Franken, K. L. M. C., et al. (2021). IL-6 signaling in macrophages is required for immunotherapy-driven regression of tumors. J. Immunother. Cancer 9 (4), e002460. doi:10.1136/jitc-2021-002460
Binnewies, M., Pollack, J. L., Rudolph, J., Dash, S., Abushawish, M., Lee, T., et al. (2021). Targeting TREM2 on tumor-associated macrophages enhances immunotherapy. Cell. Rep. 37 (3), 109844. doi:10.1016/j.celrep.2021.109844
Bissinger, S., Hage, C., Wagner, V., Maser, I. P., Brand, V., Schmittnaegel, M., et al. (2021). Macrophage depletion induces edema through release of matrix-degrading proteases and proteoglycan deposition. Sci. Transl. Med. 13 (598), eabd4550. doi:10.1126/scitranslmed.abd4550
Brempelis, K. J., Cowan, C. M., Kreuser, S. A., Labadie, K. P., Prieskorn, B. M., Lieberman, N. A. P., et al. (2020). Genetically engineered macrophages persist in solid tumors and locally deliver therapeutic proteins to activate immune responses. J. Immunother. Cancer 8 (2), e001356. doi:10.1136/jitc-2020-001356
Cai, J., Xia, L., Li, J., Ni, S., Song, H., and Wu, X. (2019). Tumor-associated macrophages derived TGF-β‒induced epithelial to mesenchymal transition in colorectal cancer cells through smad2,3-4/snail signaling pathway. Cancer Res. Treat. 51 (1), 252–266. doi:10.4143/crt.2017.613
Cao, M., Isaac, R., Yan, W., Ruan, X., Jiang, L., Wan, Y., et al. (2022). Cancer-cell-secreted extracellular vesicles suppress insulin secretion through miR-122 to impair systemic glucose homeostasis and contribute to tumour growth. Nat. Cell. Biol. 24 (6), 954–967. doi:10.1038/s41556-022-00919-7
Cassetta, L., and Pollard, J. W. (2018). Targeting macrophages: therapeutic approaches in cancer. Nat. Rev. Drug Discov. 17 (12), 887–904. doi:10.1038/nrd.2018.169
Cassetta, L., Fragkogianni, S., Sims, A. H., Swierczak, A., Forrester, L. M., Zhang, H., et al. (2019). Human tumor-associated macrophage and monocyte transcriptional landscapes reveal cancer-specific reprogramming, biomarkers, and therapeutic targets. Cancer Cell. 35 (4), 588–602. doi:10.1016/j.ccell.2019.02.009
Celik, M. O., Labuz, D., Keye, J., Glauben, R., and Machelska, H. (2020). IL-4 induces M2 macrophages to produce sustained analgesia via opioids. JCI Insight 5 (4), e133093. doi:10.1172/jci.insight.133093
Chen, X., and Song, E. (2019). Turning foes to friends: targeting cancer-associated fibroblasts. Nat. Rev. Drug Discov. 18 (2), 99–115. doi:10.1038/s41573-018-0004-1
Chen, H. M., van der Touw, W., Wang, Y. S., Kang, K., Mai, S., Zhang, J., et al. (2018). Blocking immunoinhibitory receptor LILRB2 reprograms tumor-associated myeloid cells and promotes antitumor immunity. J. Clin. Invest. 128 (12), 5647–5662. doi:10.1172/JCI97570
Chen, J., Zhang, K., Zhi, Y., Wu, Y., Chen, B., Bai, J., et al. (2021). Tumor-derived exosomal miR-19b-3p facilitates M2 macrophage polarization and exosomal LINC00273 secretion to promote lung adenocarcinoma metastasis via Hippo pathway. Clin. Transl. Med. 11 (9), e478. doi:10.1002/ctm2.478
Chen, J., Zhao, D., Zhang, L., Zhang, J., Xiao, Y., Wu, Q., et al. (2022a). Tumor-associated macrophage (TAM)-derived CCL22 induces FAK addiction in esophageal squamous cell carcinoma (ESCC). Cell. Mol. Immunol. 19 (9), 1054–1066. doi:10.1038/s41423-022-00903-z
Chen, Z., Wu, J., Wang, L., Zhao, H., and He, J. (2022b). Tumor-associated macrophages of the M1/M2 phenotype are involved in the regulation of malignant biological behavior of breast cancer cells through the EMT pathway. Med. Oncol. 39 (5), 83. doi:10.1007/s12032-022-01670-7
Cheng, S., Li, Z., Gao, R., Xing, B., Gao, Y., Yang, Y., et al. (2021). A pan-cancer single-cell transcriptional atlas of tumor infiltrating myeloid cells. Cell. 184 (3), 792–809 e23. doi:10.1016/j.cell.2021.01.010
Cho, H., Kwon, H. Y., Sharma, A., Lee, S. H., Liu, X., Miyamoto, N., et al. (2022). Visualizing inflammation with an M1 macrophage selective probe via GLUT1 as the gating target. Nat. Commun. 13 (1), 5974. doi:10.1038/s41467-022-33526-z
Choi, W., Lee, J., Lee, J., Lee, S. H., and Kim, S. (2019). Hepatocyte growth factor regulates macrophage transition to the M2 phenotype and promotes murine skeletal muscle regeneration. Front. Physiol. 10, 914. doi:10.3389/fphys.2019.00914
Christofides, A., Strauss, L., Yeo, A., Cao, C., Charest, A., and Boussiotis, V. A. (2022). The complex role of tumor-infiltrating macrophages. Nat. Immunol. 23 (8), 1148–1156. doi:10.1038/s41590-022-01267-2
Chung, S., Overstreet, J. M., Li, Y., Wang, Y., Niu, A., Wang, S., et al. (2018). TGF-beta promotes fibrosis after severe acute kidney injury by enhancing renal macrophage infiltration. JCI Insight 3 (21), e123563. doi:10.1172/jci.insight.123563
Chung, J. Y., Chan, M. K. K., Tang, P. C. T., Chan, A. S. W., Meng, X. M., Chung, J. S. Y., et al. (2021). AANG: a natural compound formula for overcoming multidrug resistance via synergistic rebalancing the TGF-β/Smad signalling in hepatocellular carcinoma. J. Cell. Mol. Med. 25 (20), 9805–9813. doi:10.1111/jcmm.16928
Chung, J. Y., Tang, P. C. T., Chan, M. K. K., Xue, V. W., Huang, X. R., Ng, C., et al. (2023). Smad3 is essential for polarization of tumor-associated neutrophils in non-small cell lung carcinoma. Nat. Commun. 14 (1), 1794. doi:10.1038/s41467-023-37515-8
Cioffi, M., Trabulo, S., Hidalgo, M., Costello, E., Greenhalf, W., Erkan, M., et al. (2015). Inhibition of CD47 effectively targets pancreatic cancer stem cells via dual mechanisms. Clin. Cancer Res. 21 (10), 2325–2337. doi:10.1158/1078-0432.CCR-14-1399
Coussens, L. M., Zitvogel, L., and Palucka, A. K. (2013). Neutralizing tumour-promoting chronic inflammation: a magic bullet? Science 339 (6117), 286–291. doi:10.1126/science.1232227
Cowman, S. J., Fuja, D. G., Liu, X. D., Tidwell, R. S. S., Kandula, N., Sirohi, D., et al. (2020). Macrophage HIF-1α is an independent prognostic indicator in kidney cancer. Clin. Cancer Res. 26 (18), 4970–4982. doi:10.1158/1078-0432.CCR-19-3890
Dang, M. T., Gonzalez, M. V., Gaonkar, K. S., Rathi, K. S., Young, P., Arif, S., et al. (2021). Macrophages in SHH subgroup medulloblastoma display dynamic heterogeneity that varies with treatment modality. Cell. Rep. 34 (13), 108917. doi:10.1016/j.celrep.2021.108917
De Vlaminck, K., Van Hove, H., Kancheva, D., Scheyltjens, I., Pombo Antunes, A. R., Bastos, J., et al. (2022). Differential plasticity and fate of brain-resident and recruited macrophages during the onset and resolution of neuroinflammation. Immunity 55 (11), 2085–2102 e9. doi:10.1016/j.immuni.2022.09.005
DeRogatis, J. M., Viramontes, K. M., Neubert, E. N., Henriquez, M. L., Guerrero-Juarez, C. F., and Tinoco, R. (2022). Targeting the PSGL-1 immune checkpoint promotes immunity to PD-1-resistant melanoma. Cancer Immunol. Res. 10 (5), 612–625. doi:10.1158/2326-6066.CIR-21-0690
Desterke, C., Turhan, A. G., Bennaceur-Griscelli, A., and Griscelli, F. (2021). HLA-dependent heterogeneity and macrophage immunoproteasome activation during lung COVID-19 disease. J. Transl. Med. 19 (1), 290. doi:10.1186/s12967-021-02965-5
Diwanji, N., and Bergmann, A. (2020). Basement membrane damage by ROS- and JNK-mediated Mmp2 activation drives macrophage recruitment to overgrown tissue. Nat. Commun. 11 (1), 3631. doi:10.1038/s41467-020-17399-8
Dong, N., Shi, X., Wang, S., Gao, Y., Kuang, Z., Xie, Q., et al. (2019). M2 macrophages mediate sorafenib resistance by secreting HGF in a feed-forward manner in hepatocellular carcinoma. Br. J. Cancer 121 (1), 22–33. doi:10.1038/s41416-019-0482-x
Dongre, A., and Weinberg, R. A. (2019). New insights into the mechanisms of epithelial-mesenchymal transition and implications for cancer. Nat. Rev. Mol. Cell. Biol. 20 (2), 69–84. doi:10.1038/s41580-018-0080-4
Dooling, L. J., Andrechak, J. C., Hayes, B. H., Kadu, S., Zhang, W., Pan, R., et al. (2023). Cooperative phagocytosis of solid tumours by macrophages triggers durable anti-tumour responses. Nat. Biomed. Eng. 7, 1081–1096. doi:10.1038/s41551-023-01031-3
Dorrington, M. G., and Fraser, I. D. C. (2019). NF-κB signaling in macrophages: dynamics, crosstalk, and signal integration. Front. Immunol. 10, 705. doi:10.3389/fimmu.2019.00705
Elomaa, H., Ahtiainen, M., Väyrynen, S. A., Ogino, S., Nowak, J. A., Lau, M. C., et al. (2023). Spatially resolved multimarker evaluation of CD274 (PD-L1)/PDCD1 (PD-1) immune checkpoint expression and macrophage polarisation in colorectal cancer. Br. J. Cancer 128 (11), 2104–2115. doi:10.1038/s41416-023-02238-6
Erlandsson, A., Carlsson, J., Lundholm, M., Fält, A., Andersson, S. O., Andrén, O., et al. (2019). M2 macrophages and regulatory T cells in lethal prostate cancer. Prostate 79 (4), 363–369. doi:10.1002/pros.23742
Etzerodt, A., Tsalkitzi, K., Maniecki, M., Damsky, W., Delfini, M., Baudoin, E., et al. (2019). Specific targeting of CD163+ TAMs mobilizes inflammatory monocytes and promotes T cell-mediated tumor regression. J. Exp. Med. 216 (10), 2394–2411. doi:10.1084/jem.20182124
Eyre, R., Alférez, D. G., Santiago-Gómez, A., Spence, K., McConnell, J. C., Hart, C., et al. (2019). Microenvironmental IL1β promotes breast cancer metastatic colonisation in the bone via activation of Wnt signalling. Nat. Commun. 10 (1), 5016. doi:10.1038/s41467-019-12807-0
Fang, T., Huang, Y. K., Wei, J., Monterrosa Mena, J. E., Lakey, P. S. J., Kleinman, M. T., et al. (2022). Superoxide release by macrophages through NADPH oxidase activation dominating chemistry by isoprene secondary organic aerosols and quinones to cause oxidative damage on membranes. Environ. Sci. Technol. 56 (23), 17029–17038. doi:10.1021/acs.est.2c03987
Fekete, T., Bencze, D., Szabo, A., Csoma, E., Biro, T., Bacsi, A., et al. (2018). Regulatory NLRs control the RLR-mediated type I interferon and inflammatory responses in human dendritic cells. Front. Immunol. 9, 2314. doi:10.3389/fimmu.2018.02314
Feng, M., Tang, P. M. K., Huang, X. R., Sun, S. F., You, Y. K., Xiao, J., et al. (2018). TGF-Beta mediates renal fibrosis via the smad3-erbb4-IR long noncoding RNA Axis. Mol. Ther. 26 (1), 148–161. doi:10.1016/j.ymthe.2017.09.024
Flores-Toro, J. A., Luo, D., Gopinath, A., Sarkisian, M. R., Campbell, J. J., Charo, I. F., et al. (2020). CCR2 inhibition reduces tumor myeloid cells and unmasks a checkpoint inhibitor effect to slow progression of resistant murine gliomas. Proc. Natl. Acad. Sci. U. S. A. 117 (2), 1129–1138. doi:10.1073/pnas.1910856117
Frising, U. C., Ribo, S., Doglio, M. G., Malissen, B., van Loo, G., and Wullaert, A. (2022). Nlrp3 inflammasome activation in macrophages suffices for inducing autoinflammation in mice. EMBO Rep. 23 (7), e54339. doi:10.15252/embr.202154339
Furuse, M., Kuwabara, H., Ikeda, N., Hattori, Y., Ichikawa, T., Kagawa, N., et al. (2020). PD-L1 and PD-L2 expression in the tumor microenvironment including peritumoral tissue in primary central nervous system lymphoma. BMC Cancer 20 (1), 277. doi:10.1186/s12885-020-06755-y
Garcia-Fojeda, B., Minutti, C. M., Montero-Fernández, C., Stamme, C., and Casals, C. (2022). Signaling pathways that mediate alveolar macrophage activation by surfactant protein A and IL-4. Front. Immunol. 13, 860262. doi:10.3389/fimmu.2022.860262
Greaney, S. K., Algazi, A. P., Tsai, K. K., Takamura, K. T., Chen, L., Twitty, C. G., et al. (2020). Intratumoral plasmid IL12 electroporation therapy in patients with advanced melanoma induces systemic and intratumoral T-cell responses. Cancer Immunol. Res. 8 (2), 246–254. doi:10.1158/2326-6066.CIR-19-0359
Greene, C. J., Nguyen, J. A., Cheung, S. M., Arnold, C. R., Balce, D. R., Wang, Y. T., et al. (2022). Macrophages disseminate pathogen associated molecular patterns through the direct extracellular release of the soluble content of their phagolysosomes. Nat. Commun. 13 (1), 3072. doi:10.1038/s41467-022-30654-4
Gu, X., Shi, Y., Dong, M., Jiang, L., Yang, J., and Liu, Z. (2021). Exosomal transfer of tumor-associated macrophage-derived hsa_circ_0001610 reduces radiosensitivity in endometrial cancer. Cell. Death Dis. 12 (9), 818. doi:10.1038/s41419-021-04087-8
Guan, X., Wang, Y., Sun, Y., Zhang, C., Ma, S., Zhang, D., et al. (2021). CTLA4-Mediated immunosuppression in glioblastoma is associated with the infiltration of macrophages in the tumor microenvironment. J. Inflamm. Res. 14, 7315–7329. doi:10.2147/JIR.S341981
Guerriero, J. L. (2019). Macrophages: their untold story in T cell activation and function. Int. Rev. Cell. Mol. Biol. 342, 73–93. doi:10.1016/bs.ircmb.2018.07.001
Guilliams, M., and Svedberg, F. R. (2021). Does tissue imprinting restrict macrophage plasticity? Nat. Immunol. 22 (2), 118–127. doi:10.1038/s41590-020-00849-2
Gunassekaran, G. R., Poongkavithai Vadevoo, S. M., Baek, M. C., and Lee, B. (2021). M1 macrophage exosomes engineered to foster M1 polarization and target the IL-4 receptor inhibit tumor growth by reprogramming tumor-associated macrophages into M1-like macrophages. Biomaterials 278, 121137. doi:10.1016/j.biomaterials.2021.121137
Guo, C. J., Atochina-Vasserman, E. N., Abramova, E., Smith, L. C., Beers, M. F., and Gow, A. J. (2019). Surfactant protein-D modulation of pulmonary macrophage phenotype is controlled by S-nitrosylation. Am. J. Physiol. Lung Cell. Mol. Physiol. 317 (5), L539–L549. doi:10.1152/ajplung.00506.2018
Haloul, M., Oliveira, E. R. A., Kader, M., Wells, J. Z., Tominello, T. R., El Andaloussi, A., et al. (2019). mTORC1-mediated polarization of M1 macrophages and their accumulation in the liver correlate with immunopathology in fatal ehrlichiosis. Sci. Rep. 9 (1), 14050. doi:10.1038/s41598-019-50320-y
Hannan, C. J., Lewis, D., O'Leary, C., Waqar, M., Brough, D., Couper, K. N., et al. (2023). Increased circulating chemokines and macrophage recruitment in growing vestibular schwannomas. Neurosurgery 92 (3), 581–589. doi:10.1227/neu.0000000000002252
Haque, A., Moriyama, M., Kubota, K., Ishiguro, N., Sakamoto, M., Chinju, A., et al. (2019). CD206+ tumor-associated macrophages promote proliferation and invasion in oral squamous cell carcinoma via EGF production. Sci. Rep. 9 (1), 14611. doi:10.1038/s41598-019-51149-1
He, L., Jhong, J. H., Chen, Q., Huang, K. Y., Strittmatter, K., Kreuzer, J., et al. (2021). Global characterization of macrophage polarization mechanisms and identification of M2-type polarization inhibitors. Cell. Rep. 37 (5), 109955. doi:10.1016/j.celrep.2021.109955
Hernandez, G. E., Ma, F., Martinez, G., Firozabadi, N. B., Salvador, J., Juang, L. J., et al. (2022). Aortic intimal resident macrophages are essential for maintenance of the non-thrombogenic intravascular state. Nat. Cardiovasc Res. 1 (1), 67–84. doi:10.1038/s44161-021-00006-4
Hou, Y., Zhu, L., Tian, H., Sun, H. X., Wang, R., Zhang, L., et al. (2018). IL-23-induced macrophage polarization and its pathological roles in mice with imiquimod-induced psoriasis. Protein Cell. 9 (12), 1027–1038. doi:10.1007/s13238-018-0505-z
Hsieh, M. H., Chen, P. C., Hsu, H. Y., Liu, J. C., Ho, Y. S., Lin, Y. J., et al. (2023). Surfactant protein D inhibits lipid-laden foamy macrophages and lung inflammation in chronic obstructive pulmonary disease. Cell. Mol. Immunol. 20 (1), 38–50. doi:10.1038/s41423-022-00946-2
Hu, M., Zhang, R., Yang, J., Zhao, C., Liu, W., Huang, Y., et al. (2023). The role of N-glycosylation modification in the pathogenesis of liver cancer. Cell. Death Dis. 14 (3), 222. doi:10.1038/s41419-023-05733-z
Huang, C., Hu, F., Song, D., Sun, X., Liu, A., Wu, Q., et al. (2022). EZH2-triggered methylation of SMAD3 promotes its activation and tumor metastasis. J. Clin. Invest. 132 (5), e152394. doi:10.1172/JCI152394
Hwang, I., Kim, J. W., Ylaya, K., Chung, E. J., Kitano, H., Perry, C., et al. (2020). Tumor-associated macrophage, angiogenesis and lymphangiogenesis markers predict prognosis of non-small cell lung cancer patients. J. Transl. Med. 18 (1), 443. doi:10.1186/s12967-020-02618-z
Im, J. H., Buzzelli, J. N., Jones, K., Franchini, F., Gordon-Weeks, A., Markelc, B., et al. (2020). FGF2 alters macrophage polarization, tumour immunity and growth and can be targeted during radiotherapy. Nat. Commun. 11 (1), 4064. doi:10.1038/s41467-020-17914-x
Irizarry-Caro, R. A., McDaniel, M. M., Overcast, G. R., Jain, V. G., Troutman, T. D., and Pasare, C. (2020). TLR signaling adapter BCAP regulates inflammatory to reparatory macrophage transition by promoting histone lactylation. Proc. Natl. Acad. Sci. U. S. A. 117 (48), 30628–30638. doi:10.1073/pnas.2009778117
Ishii, T., Mimura, I., Nagaoka, K., Naito, A., Sugasawa, T., Kuroda, R., et al. (2022). Effect of M2-like macrophages of the injured-kidney cortex on kidney cancer progression. Cell. Death Discov. 8 (1), 480. doi:10.1038/s41420-022-01255-3
Jaynes, J. M., Sable, R., Ronzetti, M., Bautista, W., Knotts, Z., Abisoye-Ogunniyan, A., et al. (2020). Mannose receptor (CD206) activation in tumor-associated macrophages enhances adaptive and innate antitumor immune responses. Sci. Transl. Med. 12 (530), eaax6337. doi:10.1126/scitranslmed.aax6337
Jeong, H., Kim, S., Hong, B. J., Lee, C. J., Kim, Y. E., Bok, S., et al. (2019). Tumor-associated macrophages enhance tumor hypoxia and aerobic glycolysis. Cancer Res. 79 (4), 795–806. doi:10.1158/0008-5472.CAN-18-2545
Jia, X., Yan, B., Tian, X., Liu, Q., Jin, J., Shi, J., et al. (2021). CD47/SIRPα pathway mediates cancer immune escape and immunotherapy. Int. J. Biol. Sci. 17 (13), 3281–3287. doi:10.7150/ijbs.60782
Jiang, P., Gao, W., Ma, T., Wang, R., Piao, Y., Dong, X., et al. (2019). CD137 promotes bone metastasis of breast cancer by enhancing the migration and osteoclast differentiation of monocytes/macrophages. Theranostics 9 (10), 2950–2966. doi:10.7150/thno.29617
Jin, M. Z., and Jin, W. L. (2020). The updated landscape of tumor microenvironment and drug repurposing. Signal Transduct. Target Ther. 5 (1), 166. doi:10.1038/s41392-020-00280-x
Johnston, R. J., Su, L. J., Pinckney, J., Critton, D., Boyer, E., Krishnakumar, A., et al. (2019). VISTA is an acidic pH-selective ligand for PSGL-1. Nature 574 (7779), 565–570. doi:10.1038/s41586-019-1674-5
Kaplanov, I., Carmi, Y., Kornetsky, R., Shemesh, A., Shurin, G. V., Shurin, M. R., et al. (2019). Blocking IL-1β reverses the immunosuppression in mouse breast cancer and synergizes with anti-PD-1 for tumor abrogation. Proc. Natl. Acad. Sci. U. S. A. 116 (4), 1361–1369. doi:10.1073/pnas.1812266115
Katzenelenbogen, Y., Sheban, F., Yalin, A., Yofe, I., Svetlichnyy, D., Jaitin, D. A., et al. (2020). Coupled scRNA-seq and intracellular protein activity reveal an immunosuppressive role of TREM2 in cancer. Cell. 182 (4), 872–885. doi:10.1016/j.cell.2020.06.032
Kawasaki, T., Ikegawa, M., Yunoki, K., Otani, H., Ori, D., Ishii, K. J., et al. (2022). Alveolar macrophages instruct CD8(+) T cell expansion by antigen cross-presentation in lung. Cell. Rep. 41 (11), 111828. doi:10.1016/j.celrep.2022.111828
Kennedy, A., Waters, E., Rowshanravan, B., Hinze, C., Williams, C., Janman, D., et al. (2022). Differences in CD80 and CD86 transendocytosis reveal CD86 as a key target for CTLA-4 immune regulation. Nat. Immunol. 23 (9), 1365–1378. doi:10.1038/s41590-022-01289-w
Kermanizadeh, A., Brown, D. M., Moritz, W., and Stone, V. (2019). The importance of inter-individual Kupffer cell variability in the governance of hepatic toxicity in a 3D primary human liver microtissue model. Sci. Rep. 9 (1), 7295. doi:10.1038/s41598-019-43870-8
Kesh, K., Gupta, V. K., Durden, B., Garrido, V., Mateo-Victoriano, B., Lavania, S. P., et al. (2020). Therapy resistance, cancer stem cells and ECM in cancer: the matrix reloaded. Cancers (Basel) 12 (10), 3067. doi:10.3390/cancers12103067
Kidwell, C. U., Casalini, J. R., Pradeep, S., Scherer, S. D., Greiner, D., Bayik, D., et al. (2023). Transferred mitochondria accumulate reactive oxygen species, promoting proliferation. Elife 12, e85494. doi:10.7554/eLife.85494
Kim, H., Wang, S. Y., Kwak, G., Yang, Y., Kwon, I. C., and Kim, S. H. (2019a). Exosome-guided phenotypic switch of M1 to M2 macrophages for cutaneous wound healing. Adv. Sci. (Weinh) 6 (20), 1900513. doi:10.1002/advs.201900513
Kim, H., Chung, H., Kim, J., Choi, D. H., Shin, Y., Kang, Y. G., et al. (2019b). Macrophages-Triggered sequential remodeling of endothelium-interstitial matrix to form pre-metastatic niche in microfluidic tumor microenvironment. Adv. Sci. (Weinh) 6 (11), 1900195. doi:10.1002/advs.201900195
Kim, S. H., Saeidi, S., Zhong, X., Gwak, S. Y., Muna, I. A., Park, S. A., et al. (2020). Breast cancer cell debris diminishes therapeutic efficacy through heme oxygenase-1-mediated inactivation of M1-like tumor-associated macrophages. Neoplasia 22 (11), 606–616. doi:10.1016/j.neo.2020.08.006
Kimura, S., Nanbu, U., Noguchi, H., Harada, Y., Kumamoto, K., Sasaguri, Y., et al. (2019). Macrophage CCL22 expression in the tumor microenvironment and implications for survival in patients with squamous cell carcinoma of the tongue. J. Oral Pathol. Med. 48 (8), 677–685. doi:10.1111/jop.12885
Klichinsky, M., Ruella, M., Shestova, O., Lu, X. M., Best, A., Zeeman, M., et al. (2020). Human chimeric antigen receptor macrophages for cancer immunotherapy. Nat. Biotechnol. 38 (8), 947–953. doi:10.1038/s41587-020-0462-y
Kohno, K., Koya-Miyata, S., Harashima, A., Tsukuda, T., Katakami, M., Ariyasu, T., et al. (2021). Inflammatory M1-like macrophages polarized by NK-4 undergo enhanced phenotypic switching to an anti-inflammatory M2-like phenotype upon co-culture with apoptotic cells. J. Inflamm. (Lond) 18 (1), 2. doi:10.1186/s12950-020-00267-z
Kraaij, M. D., Savage, N. D. L., van der Kooij, S. W., Koekkoek, K., Wang, J., van den Berg, J. M., et al. (2010). Induction of regulatory T cells by macrophages is dependent on production of reactive oxygen species. Proc. Natl. Acad. Sci. U. S. A. 107 (41), 17686–17691. doi:10.1073/pnas.1012016107
Kumar, R., Mickael, C., Kassa, B., Sanders, L., Hernandez-Saavedra, D., Koyanagi, D. E., et al. (2020). Interstitial macrophage-derived thrombospondin-1 contributes to hypoxia-induced pulmonary hypertension. Cardiovasc Res. 116 (12), 2021–2030. doi:10.1093/cvr/cvz304
La Fleur, L., Botling, J., He, F., Pelicano, C., Zhou, C., He, C., et al. (2021). Targeting MARCO and IL37R on immunosuppressive macrophages in lung cancer blocks regulatory T cells and supports cytotoxic lymphocyte function. Cancer Res. 81 (4), 956–967. doi:10.1158/0008-5472.CAN-20-1885
Lai, Y. S., Wahyuningtyas, R., Aui, S. P., and Chang, K. T. (2019). Autocrine VEGF signalling on M2 macrophages regulates PD-L1 expression for immunomodulation of T cells. J. Cell. Mol. Med. 23 (2), 1257–1267. doi:10.1111/jcmm.14027
Lazarova, M., and Steinle, A. (2019). Impairment of nkg2d-mediated tumor immunity by TGF-β. Front. Immunol. 10, 2689. doi:10.3389/fimmu.2019.02689
Lechner, A., Henkel, F. D. R., Hartung, F., Bohnacker, S., Alessandrini, F., Gubernatorova, E. O., et al. (2022). Macrophages acquire a TNF-dependent inflammatory memory in allergic asthma. J. Allergy Clin. Immunol. 149 (6), 2078–2090. doi:10.1016/j.jaci.2021.11.026
Lee, J., Son, W., Hong, J., Song, Y., Yang, C. S., and Kim, Y. H. (2021). Down-regulation of TNF-α via macrophage-targeted RNAi system for the treatment of acute inflammatory sepsis. J. Control Release 336, 344–353. doi:10.1016/j.jconrel.2021.06.022
Li, D., and Wu, M. (2021). Pattern recognition receptors in health and diseases. Signal Transduct. Target Ther. 6 (1), 291. doi:10.1038/s41392-021-00687-0
Li, F., Lv, B., Liu, Y., Hua, T., Han, J., Sun, C., et al. (2018). Blocking the CD47-SIRPα axis by delivery of anti-CD47 antibody induces antitumor effects in glioma and glioma stem cells. Oncoimmunology 7 (2), e1391973. doi:10.1080/2162402X.2017.1391973
Li, W., Zhang, X., Wu, F., Zhou, Y., Bao, Z., Li, H., et al. (2019a). Gastric cancer-derived mesenchymal stromal cells trigger M2 macrophage polarization that promotes metastasis and EMT in gastric cancer. Cell. Death Dis. 10 (12), 918. doi:10.1038/s41419-019-2131-y
Li, K., Yang, L., Li, J., Guan, C., Zhang, S., Lao, X., et al. (2019b). TGFβ induces stemness through non-canonical AKT-FOXO3a axis in oral squamous cell carcinoma. EBioMedicine 48, 70–80. doi:10.1016/j.ebiom.2019.09.027
Li, C., Xue, V. W., Wang, Q. M., Lian, G. Y., Huang, X. R., Lee, T. L., et al. (2020). The mincle/syk/NF-κB signaling circuit is essential for maintaining the protumoral activities of tumor-associated macrophages. Cancer Immunol. Res. 8 (8), 1004–1017. doi:10.1158/2326-6066.CIR-19-0782
Li, X., Su, X., Liu, R., Pan, Y., Fang, J., Cao, L., et al. (2021a). HDAC inhibition potentiates anti-tumor activity of macrophages and enhances anti-PD-L1-mediated tumor suppression. Oncogene 40 (10), 1836–1850. doi:10.1038/s41388-020-01636-x
Li, Y. R., Zhou, Y., Kim, Y. J., Zhu, Y., Ma, F., Yu, J., et al. (2021b). Development of allogeneic HSC-engineered iNKT cells for off-the-shelf cancer immunotherapy. Cell. Rep. Med. 2 (11), 100449. doi:10.1016/j.xcrm.2021.100449
Li, H., Yang, P., Wang, J., Zhang, J., Ma, Q., Jiang, Y., et al. (2022a). HLF regulates ferroptosis, development and chemoresistance of triple-negative breast cancer by activating tumor cell-macrophage crosstalk. J. Hematol. Oncol. 15 (1), 2. doi:10.1186/s13045-021-01223-x
Li, M., He, L., Zhu, J., Zhang, P., and Liang, S. (2022b). Targeting tumor-associated macrophages for cancer treatment. Cell. Biosci. 12 (1), 85. doi:10.1186/s13578-022-00823-5
Li, Y. R., Brown, J., Yu, Y., Lee, D., Zhou, K., Dunn, Z. S., et al. (2022c). Targeting immunosuppressive tumor-associated macrophages using innate T cells for enhanced antitumor reactivity. Cancers (Basel) 14 (11), 2749. doi:10.3390/cancers14112749
Li, Y. R., Dunn, Z. S., Yu, Y., Li, M., Wang, P., and Yang, L. (2023). Advancing cell-based cancer immunotherapy through stem cell engineering. Cell. Stem Cell. 30 (5), 592–610. doi:10.1016/j.stem.2023.02.009
Liang, S., Ma, H. Y., Zhong, Z., Dhar, D., Liu, X., Xu, J., et al. (2019). NADPH oxidase 1 in liver macrophages promotes inflammation and tumor development in mice. Gastroenterology 156 (4), 1156–1172. doi:10.1053/j.gastro.2018.11.019
Liang, N., Bing, Z., Wang, Y., Liu, X., Guo, C., Cao, L., et al. (2022). Clinical implications of EGFR-associated MAPK/ERK pathway in multiple primary lung cancer. Clin. Transl. Med. 12 (5), e847. doi:10.1002/ctm2.847
Lin, Y., Xu, J., and Lan, H. (2019). Tumor-associated macrophages in tumor metastasis: biological roles and clinical therapeutic applications. J. Hematol. Oncol. 12 (1), 76. doi:10.1186/s13045-019-0760-3
Lin, S. C., Liao, Y. C., Chen, P. M., Yang, Y. Y., Wang, Y. H., Tung, S. L., et al. (2022). Periostin promotes ovarian cancer metastasis by enhancing M2 macrophages and cancer-associated fibroblasts via integrin-mediated NF-κB and TGF-β2 signaling. J. Biomed. Sci. 29 (1), 109. doi:10.1186/s12929-022-00888-x
Lin, Y., Huang, S., Qi, Y., Xie, L., Jiang, J., Li, H., et al. (2023). PSGL-1 is a novel tumor microenvironment prognostic biomarker with cervical high-grade squamous lesions and more. Front. Oncol. 13, 1052201. doi:10.3389/fonc.2023.1052201
Liu, W., and Sun, Y. (2023). Epigenetics in glaucoma: a link between histone methylation and neurodegeneration. J. Clin. Invest. 133 (8), e173784. doi:10.1172/JCI173784
Liu, L., Zhang, L., Yang, L., Li, H., Li, R., Yu, J., et al. (2017). Anti-CD47 antibody as a targeted therapeutic agent for human lung cancer and cancer stem cells. Front. Immunol. 8, 404. doi:10.3389/fimmu.2017.00404
Liu, L., Ye, Y., and Zhu, X. (2019). MMP-9 secreted by tumor associated macrophages promoted gastric cancer metastasis through a PI3K/AKT/Snail pathway. Biomed. Pharmacother. 117, 109096. doi:10.1016/j.biopha.2019.109096
Liu, Y., Zugazagoitia, J., Ahmed, F. S., Henick, B. S., Gettinger, S. N., Herbst, R. S., et al. (2020). Immune cell PD-L1 colocalizes with macrophages and is associated with outcome in PD-1 pathway blockade therapy. Clin. Cancer Res. 26 (4), 970–977. doi:10.1158/1078-0432.CCR-19-1040
Liu, J., Geng, X., Hou, J., and Wu, G. (2021). New insights into M1/M2 macrophages: key modulators in cancer progression. Cancer Cell. Int. 21 (1), 389. doi:10.1186/s12935-021-02089-2
Liu, J. Q., Zhang, C., Zhang, X., Yan, J., Zeng, C., Talebian, F., et al. (2022). Intratumoral delivery of IL-12 and IL-27 mRNA using lipid nanoparticles for cancer immunotherapy. J. Control Release 345, 306–313. doi:10.1016/j.jconrel.2022.03.021
Liu, Z. L., Chen, H. H., Zheng, L. L., Sun, L. P., and Shi, L. (2023a). Angiogenic signaling pathways and anti-angiogenic therapy for cancer. Signal Transduct. Target Ther. 8 (1), 198. doi:10.1038/s41392-023-01460-1
Liu, H., Zhao, Q., Tan, L., Wu, X., Huang, R., Zuo, Y., et al. (2023b). Neutralizing IL-8 potentiates immune checkpoint blockade efficacy for glioma. Cancer Cell. 41 (4), 693–710 e8. doi:10.1016/j.ccell.2023.03.004
Liu, Y., Wang, Y., Yang, Y., Weng, L., Wu, Q., Zhang, J., et al. (2023c). Emerging phagocytosis checkpoints in cancer immunotherapy. Signal Transduct. Target Ther. 8 (1), 104. doi:10.1038/s41392-023-01365-z
Loeuillard, E., Yang, J., Buckarma, E., Wang, J., Liu, Y., Conboy, C., et al. (2020). Targeting tumor-associated macrophages and granulocytic myeloid-derived suppressor cells augments PD-1 blockade in cholangiocarcinoma. J. Clin. Invest. 130 (10), 5380–5396. doi:10.1172/JCI137110
Lopez de Andres, J., Griñán-Lisón, C., Jiménez, G., and Marchal, J. A. (2020). Cancer stem cell secretome in the tumor microenvironment: a key point for an effective personalized cancer treatment. J. Hematol. Oncol. 13 (1), 136. doi:10.1186/s13045-020-00966-3
Lu, C. Y., Santosa, K. B., Jablonka-Shariff, A., Vannucci, B., Fuchs, A., Turnbull, I., et al. (2020). Macrophage-derived vascular endothelial growth factor-A is integral to neuromuscular junction reinnervation after nerve injury. J. Neurosci. 40 (50), 9602–9616. doi:10.1523/JNEUROSCI.1736-20.2020
Lugano, R., Ramachandran, M., and Dimberg, A. (2020). Tumor angiogenesis: causes, consequences, challenges and opportunities. Cell. Mol. Life Sci. 77 (9), 1745–1770. doi:10.1007/s00018-019-03351-7
Lundahl, M. L. E., Mitermite, M., Ryan, D. G., Case, S., Williams, N. C., Yang, M., et al. (2022). Macrophage innate training induced by IL-4 and IL-13 activation enhances OXPHOS driven anti-mycobacterial responses. Elife 11, e74690. doi:10.7554/eLife.74690
Luo, W. J., Yu, S. L., Chang, C. C., Chien, M. H., Chang, Y. L., Liao, K. M., et al. (2022). HLJ1 amplifies endotoxin-induced sepsis severity by promoting IL-12 heterodimerization in macrophages. Elife 11, e76094. doi:10.7554/eLife.76094
Lv, W., Guo, H., Wang, J., Ma, R., Niu, L., and Shang, Y. (2023). PDLIM2 can inactivate the TGF-beta/Smad pathway to inhibit the malignant behavior of ovarian cancer cells. Cell. Biochem. Funct. 41, 542–552. doi:10.1002/cbf.3801
Maalej, K. M., Merhi, M., Inchakalody, V. P., Mestiri, S., Alam, M., Maccalli, C., et al. (2023). CAR-cell therapy in the era of solid tumor treatment: current challenges and emerging therapeutic advances. Mol. Cancer 22 (1), 20. doi:10.1186/s12943-023-01723-z
Maier, B., Leader, A. M., Chen, S. T., Tung, N., Chang, C., LeBerichel, J., et al. (2020). A conserved dendritic-cell regulatory program limits antitumour immunity. Nature 580 (7802), 257–262. doi:10.1038/s41586-020-2134-y
Maldonado, L. A. G., Nascimento, C. R., Rodrigues Fernandes, N. A., Silva, A. L. P., D'Silva, N. J., and Rossa, C. (2022). Influence of tumor cell-derived TGF-β on macrophage phenotype and macrophage-mediated tumor cell invasion. Int. J. Biochem. Cell. Biol. 153, 106330. doi:10.1016/j.biocel.2022.106330
Mantovani, A., Allavena, P., Marchesi, F., and Garlanda, C. (2022). Macrophages as tools and targets in cancer therapy. Nat. Rev. Drug Discov. 21 (11), 799–820. doi:10.1038/s41573-022-00520-5
Marigo, I., Trovato, R., Hofer, F., Ingangi, V., Desantis, G., Leone, K., et al. (2020). Disabled homolog 2 controls prometastatic activity of tumor-associated macrophages. Cancer Discov. 10 (11), 1758–1773. doi:10.1158/2159-8290.CD-20-0036
Mascarau, R., Woottum, M., Fromont, L., Gence, R., Cantaloube-Ferrieu, V., Vahlas, Z., et al. (2023). Productive HIV-1 infection of tissue macrophages by fusion with infected CD4+ T cells. J. Cell. Biol. 222 (5), e202205103. doi:10.1083/jcb.202205103
Mattiola, I., Pesant, M., Tentorio, P. F., Molgora, M., Marcenaro, E., Lugli, E., et al. (2015). Priming of human resting NK cells by autologous M1 macrophages via the engagement of IL-1β, IFN-β, and IL-15 pathways. J. Immunol. 195 (6), 2818–2828. doi:10.4049/jimmunol.1500325
McCaw, T. R., Li, M., Starenki, D., Liu, M., Cooper, S. J., Arend, R. C., et al. (2019). Histone deacetylase inhibition promotes intratumoral CD8+ T-cell responses, sensitizing murine breast tumors to anti-PD1. Cancer Immunol. Immunother. 68 (12), 2081–2094. doi:10.1007/s00262-019-02430-9
Molgora, M., Esaulova, E., Vermi, W., Hou, J., Chen, Y., Luo, J., et al. (2020). TREM2 modulation remodels the tumor myeloid landscape enhancing anti-PD-1 immunotherapy. Cell. 182 (4), 886–900. doi:10.1016/j.cell.2020.07.013
Morhardt, T. L., Hayashi, A., Ochi, T., Quirós, M., Kitamoto, S., Nagao-Kitamoto, H., et al. (2019). IL-10 produced by macrophages regulates epithelial integrity in the small intestine. Sci. Rep. 9 (1), 1223. doi:10.1038/s41598-018-38125-x
Mosser, D. M., Hamidzadeh, K., and Goncalves, R. (2021). Macrophages and the maintenance of homeostasis. Cell. Mol. Immunol. 18 (3), 579–587. doi:10.1038/s41423-020-00541-3
Mouton, A. J., Aitken, N. M., Moak, S. P., do Carmo, J. M., da Silva, A. A., Omoto, A. C. M., et al. (2023). Temporal changes in glucose metabolism reflect polarization in resident and monocyte-derived macrophages after myocardial infarction. Front. Cardiovasc Med. 10, 1136252. doi:10.3389/fcvm.2023.1136252
Muniz-Bongers, L. R., McClain, C. B., Saxena, M., Bongers, G., Merad, M., and Bhardwaj, N. (2021). MMP2 and TLRs modulate immune responses in the tumor microenvironment. JCI Insight 6 (12), e144913. doi:10.1172/jci.insight.144913
Muraoka, D., Seo, N., Hayashi, T., Tahara, Y., Fujii, K., Tawara, I., et al. (2019). Antigen delivery targeted to tumor-associated macrophages overcomes tumor immune resistance. J. Clin. Invest. 129 (3), 1278–1294. doi:10.1172/JCI97642
Nagata, E., Masuda, H., Nakayama, T., Netsu, S., Yuzawa, H., Fujii, N., et al. (2019). Insufficient production of IL-10 from M2 macrophages impairs in vitro endothelial progenitor cell differentiation in patients with Moyamoya disease. Sci. Rep. 9 (1), 16752. doi:10.1038/s41598-019-53114-4
Nalio Ramos, R., Missolo-Koussou, Y., Gerber-Ferder, Y., Bromley, C. P., Bugatti, M., Núñez, N. G., et al. (2022). Tissue-resident FOLR2(+) macrophages associate with CD8(+) T cell infiltration in human breast cancer. Cell. 185 (7), 1189–1207 e25. doi:10.1016/j.cell.2022.02.021
Natale, G., and Bocci, G. (2023). Discovery and development of tumor angiogenesis assays. Methods Mol. Biol. 2572, 1–37. doi:10.1007/978-1-0716-2703-7_1
Nau, G. J., Richmond, J. F. L., Schlesinger, A., Jennings, E. G., Lander, E. S., and Young, R. A. (2002). Human macrophage activation programs induced by bacterial pathogens. Proc. Natl. Acad. Sci. U. S. A. 99 (3), 1503–1508. doi:10.1073/pnas.022649799
Neu, C., Thiele, Y., Horr, F., Beckers, C., Frank, N., Marx, G., et al. (2022). DAMPs released from proinflammatory macrophages induce inflammation in cardiomyocytes via activation of TLR4 and TNFR. Int. J. Mol. Sci. 23 (24), 15522. doi:10.3390/ijms232415522
Ning, Y., Cui, Y., Li, X., Cao, X., Chen, A., Xu, C., et al. (2018). Co-culture of ovarian cancer stem-like cells with macrophages induced SKOV3 cells stemness via IL-8/STAT3 signaling. Biomed. Pharmacother. 103, 262–271. doi:10.1016/j.biopha.2018.04.022
Nost, T. H., Alcala, K., Urbarova, I., Byrne, K. S., Guida, F., Sandanger, T. M., et al. (2021). Systemic inflammation markers and cancer incidence in the UK Biobank. Eur. J. Epidemiol. 36 (8), 841–848. doi:10.1007/s10654-021-00752-6
Ntokou, A., Dave, J. M., Kauffman, A. C., Sauler, M., Ryu, C., Hwa, J., et al. (2021). Macrophage-derived PDGF-B induces muscularization in murine and human pulmonary hypertension. JCI Insight 6 (6), e139067. doi:10.1172/jci.insight.139067
Nunez, S. Y., Ziblat, A., Secchiari, F., Torres, N. I., Sierra, J. M., Raffo Iraolagoitia, X. L., et al. (2018). Human M2 macrophages limit NK cell effector functions through secretion of TGF-beta and engagement of CD85j. J. Immunol. 200 (3), 1008–1015. doi:10.4049/jimmunol.1700737
O'Brien, S. A., Orf, J., Skrzypczynska, K. M., Tan, H., Kim, J., DeVoss, J., et al. (2021). Activity of tumor-associated macrophage depletion by CSF1R blockade is highly dependent on the tumor model and timing of treatment. Cancer Immunol. Immunother. 70 (8), 2401–2410. doi:10.1007/s00262-021-02861-3
Onal, S., Turker-Burhan, M., Bati-Ayaz, G., Yanik, H., and Pesen-Okvur, D. (2021). Breast cancer cells and macrophages in a paracrine-juxtacrine loop. Biomaterials 267, 120412. doi:10.1016/j.biomaterials.2020.120412
Orange, S. T., Leslie, J., Ross, M., Mann, D. A., and Wackerhage, H. (2023). The exercise IL-6 enigma in cancer. Trends Endocrinol. Metab. 34, 749–763. doi:10.1016/j.tem.2023.08.001
Pereira, J. A., Lanzar, Z., Clark, J. T., Hart, A. P., Douglas, B. B., Shallberg, L., et al. (2023). PD-1 and CTLA-4 exert additive control of effector regulatory T cells at homeostasis. Front. Immunol. 14, 997376. doi:10.3389/fimmu.2023.997376
Petty, A. J., Owen, D. H., Yang, Y., and Huang, X. (2021). Targeting tumor-associated macrophages in cancer immunotherapy. Cancers (Basel) 13 (21), 5318. doi:10.3390/cancers13215318
Pfefferle, M., Dubach, I. L., Buzzi, R. M., Dürst, E., Schulthess-Lutz, N., Baselgia, L., et al. (2023). Antibody-induced erythrophagocyte reprogramming of Kupffer cells prevents anti-CD40 cancer immunotherapy-associated liver toxicity. J. Immunother. Cancer 11 (1), e005718. doi:10.1136/jitc-2022-005718
Pfirschke, C., Zilionis, R., Engblom, C., Messemaker, M., Zou, A. E., Rickelt, S., et al. (2022). Macrophage-targeted therapy unlocks antitumoral cross-talk between ifnγ-secreting lymphocytes and IL12-producing dendritic cells. Cancer Immunol. Res. 10 (1), 40–55. doi:10.1158/2326-6066.CIR-21-0326
Piatakova, A., Polakova, I., Smahelova, J., Johari, S. D., Nunvar, J., and Smahel, M. (2021). Distinct responsiveness of tumor-associated macrophages to immunotherapy of tumors with different mechanisms of major histocompatibility complex class I downregulation. Cancers (Basel) 13 (12), 3057. doi:10.3390/cancers13123057
Pu, Y., and Ji, Q. (2022). Tumor-associated macrophages regulate PD-1/PD-L1 immunosuppression. Front. Immunol. 13, 874589. doi:10.3389/fimmu.2022.874589
Puig-Saus, C., Sennino, B., Peng, S., Wang, C. L., Pan, Z., Yuen, B., et al. (2023). Neoantigen-targeted CD8(+) T cell responses with PD-1 blockade therapy. Nature 615 (7953), 697–704. doi:10.1038/s41586-023-05787-1
Qi, Y. T., Jiang, H., Wu, W. T., Zhang, F. L., Tian, S. Y., Fan, W. T., et al. (2022). Homeostasis inside single activated phagolysosomes: quantitative and selective measurements of submillisecond dynamics of reactive oxygen and nitrogen species production with a nanoelectrochemical sensor. J. Am. Chem. Soc. 144 (22), 9723–9733. doi:10.1021/jacs.2c01857
Radharani, N. N. V., Yadav, A. S., Nimma, R., Kumar, T. V. S., Bulbule, A., Chanukuppa, V., et al. (2022). Tumor-associated macrophage derived IL-6 enriches cancer stem cell population and promotes breast tumor progression via Stat-3 pathway. Cancer Cell. Int. 22 (1), 122. doi:10.1186/s12935-022-02527-9
Rahabi, M., Jacquemin, G., Prat, M., Meunier, E., AlaEddine, M., Bertrand, B., et al. (2020). Divergent roles for macrophage C-type lectin receptors, dectin-1 and mannose receptors, in the intestinal inflammatory response. Cell. Rep. 30 (13), 4386–4398. doi:10.1016/j.celrep.2020.03.018
Rajamaki, K., Taira, A., Katainen, R., Välimäki, N., Kuosmanen, A., Plaketti, R. M., et al. (2021). Genetic and epigenetic characteristics of inflammatory bowel disease-associated colorectal cancer. Gastroenterology 161 (2), 592–607. doi:10.1053/j.gastro.2021.04.042
Rapp, M., Wintergerst, M. W. M., Kunz, W. G., Vetter, V. K., Knott, M. M. L., Lisowski, D., et al. (2019). CCL22 controls immunity by promoting regulatory T cell communication with dendritic cells in lymph nodes. J. Exp. Med. 216 (5), 1170–1181. doi:10.1084/jem.20170277
Ren, L., Yi, J., Yang, Y., Li, W., Zheng, X., Liu, J., et al. (2022). Systematic pan-cancer analysis identifies APOC1 as an immunological biomarker which regulates macrophage polarization and promotes tumor metastasis. Pharmacol. Res. 183, 106376. doi:10.1016/j.phrs.2022.106376
Revu, S., Wu, J., Henkel, M., Rittenhouse, N., Menk, A., Delgoffe, G. M., et al. (2018). IL-23 and IL-1β drive human Th17 cell differentiation and metabolic reprogramming in absence of CD28 costimulation. Cell. Rep. 22 (10), 2642–2653. doi:10.1016/j.celrep.2018.02.044
Roda, J. M., Wang, Y., Sumner, L. A., Phillips, G. S., Marsh, C. B., and Eubank, T. D. (2012). Stabilization of HIF-2α induces sVEGFR-1 production from tumor-associated macrophages and decreases tumor growth in a murine melanoma model. J. Immunol. 189 (6), 3168–3177. doi:10.4049/jimmunol.1103817
Rodriguez-Garcia, A., Lynn, R. C., Poussin, M., Eiva, M. A., Shaw, L. C., O'Connor, R. S., et al. (2021). CAR-T cell-mediated depletion of immunosuppressive tumor-associated macrophages promotes endogenous antitumor immunity and augments adoptive immunotherapy. Nat. Commun. 12 (1), 877. doi:10.1038/s41467-021-20893-2
Romagnani, P., Lasagni, L., Annunziato, F., Serio, M., and Romagnani, S. (2004). CXC chemokines: the regulatory link between inflammation and angiogenesis. Trends Immunol. 25 (4), 201–209. doi:10.1016/j.it.2004.02.006
Ruiz-Blazquez, P., Pistorio, V., Fernández-Fernández, M., and Moles, A. (2021). The multifaceted role of cathepsins in liver disease. J. Hepatol. 75 (5), 1192–1202. doi:10.1016/j.jhep.2021.06.031
Sa, J. K., Chang, N., Lee, H. W., Cho, H. J., Ceccarelli, M., Cerulo, L., et al. (2020). Transcriptional regulatory networks of tumor-associated macrophages that drive malignancy in mesenchymal glioblastoma. Genome Biol. 21 (1), 216. doi:10.1186/s13059-020-02140-x
Sahraei, M., Chaube, B., Liu, Y., Sun, J., Kaplan, A., Price, N. L., et al. (2019). Suppressing miR-21 activity in tumor-associated macrophages promotes an antitumor immune response. J. Clin. Invest. 129 (12), 5518–5536. doi:10.1172/JCI127125
Sakama, S., Kurusu, K., Morita, M., Oizumi, T., Masugata, S., Oka, S., et al. (2021). An enriched environment alters DNA repair and inflammatory responses after radiation exposure. Front. Immunol. 12, 760322. doi:10.3389/fimmu.2021.760322
Sanchez-Paulete, A. R., Mateus-Tique, J., Mollaoglu, G., Nielsen, S. R., Marks, A., Lakshmi, A., et al. (2022). Targeting macrophages with CAR T cells delays solid tumor progression and enhances antitumor immunity. Cancer Immunol. Res. 10 (11), 1354–1369. doi:10.1158/2326-6066.CIR-21-1075
Saraiva, M., Vieira, P., and O'Garra, A. (2020). Biology and therapeutic potential of interleukin-10. J. Exp. Med. 217 (1), e20190418. doi:10.1084/jem.20190418
Scavuzzi, B. M., van Drongelen, V., and Holoshitz, J. (2022). HLA-G and the MHC cusp theory. Front. Immunol. 13, 814967. doi:10.3389/fimmu.2022.814967
Schaaf, M. B., Garg, A. D., and Agostinis, P. (2018). Defining the role of the tumor vasculature in antitumor immunity and immunotherapy. Cell. Death Dis. 9 (2), 115. doi:10.1038/s41419-017-0061-0
Schito, L., and Rey, S. (2020). Hypoxia: turning vessels into vassals of cancer immunotolerance. Cancer Lett. 487, 74–84. doi:10.1016/j.canlet.2020.05.015
Serbulea, V., Upchurch, C. M., Ahern, K. W., Bories, G., Voigt, P., DeWeese, D. E., et al. (2018). Macrophages sensing oxidized DAMPs reprogram their metabolism to support redox homeostasis and inflammation through a TLR2-Syk-ceramide dependent mechanism. Mol. Metab. 7, 23–34. doi:10.1016/j.molmet.2017.11.002
Sharma, N., Atolagbe, O. T., Ge, Z., and Allison, J. P. (2021). LILRB4 suppresses immunity in solid tumors and is a potential target for immunotherapy. J. Exp. Med. 218 (7), e20201811. doi:10.1084/jem.20201811
She, L., Qin, Y., Wang, J., Liu, C., Zhu, G., Li, G., et al. (2018). Tumor-associated macrophages derived CCL18 promotes metastasis in squamous cell carcinoma of the head and neck. Cancer Cell. Int. 18, 120. doi:10.1186/s12935-018-0620-1
Shepherd, A. J., Mickle, A. D., Golden, J. P., Mack, M. R., Halabi, C. M., de Kloet, A. D., et al. (2018). Macrophage angiotensin II type 2 receptor triggers neuropathic pain. Proc. Natl. Acad. Sci. U. S. A. 115 (34), E8057–E8066. doi:10.1073/pnas.1721815115
Shi, Q., Shen, Q., Liu, Y., Shi, Y., Huang, W., Wang, X., et al. (2022). Increased glucose metabolism in TAMs fuels O-GlcNAcylation of lysosomal Cathepsin B to promote cancer metastasis and chemoresistance. Cancer Cell. 40 (10), 1207–1222 e10. doi:10.1016/j.ccell.2022.08.012
Shinchi, Y., Ishizuka, S., Komohara, Y., Matsubara, E., Mito, R., Pan, C., et al. (2022). The expression of PD-1 ligand 1 on macrophages and its clinical impacts and mechanisms in lung adenocarcinoma. Cancer Immunol. Immunother. 71 (11), 2645–2661. doi:10.1007/s00262-022-03187-4
Simonetta, F., Lohmeyer, J. K., Hirai, T., Maas-Bauer, K., Alvarez, M., Wenokur, A. S., et al. (2021). Allogeneic CAR invariant natural killer T cells exert potent antitumor effects through host CD8 T-cell cross-priming. Clin. Cancer Res. 27 (21), 6054–6064. doi:10.1158/1078-0432.CCR-21-1329
Siu, L. L., Wang, D., Hilton, J., Geva, R., Rasco, D., Perets, R., et al. (2022). Correction: first-in-Class anti-immunoglobulin-like transcript 4 myeloid-specific antibody MK-4830 abrogates a PD-1 resistance mechanism in patients with advanced solid tumors. Clin. Cancer Res. 28 (8), 1734. doi:10.1158/1078-0432.CCR-22-0564
Stanley, E. R., and Chitu, V. (2014). CSF-1 receptor signaling in myeloid cells. Cold Spring Harb. Perspect. Biol. 6 (6), a021857. doi:10.1101/cshperspect.a021857
Su, C., Zhang, J., Yarden, Y., and Fu, L. (2021). The key roles of cancer stem cell-derived extracellular vesicles. Signal Transduct. Target Ther. 6 (1), 109. doi:10.1038/s41392-021-00499-2
Sumitomo, R., Hirai, T., Fujita, M., Murakami, H., Otake, Y., and Huang, C. L. (2019). PD-L1 expression on tumor-infiltrating immune cells is highly associated with M2 TAM and aggressive malignant potential in patients with resected non-small cell lung cancer. Lung Cancer 136, 136–144. doi:10.1016/j.lungcan.2019.08.023
Sun, L., Wang, Q., Chen, B., Zhao, Y., Shen, B., Wang, H., et al. (2018). Gastric cancer mesenchymal stem cells derived IL-8 induces PD-L1 expression in gastric cancer cells via STAT3/mTOR-c-Myc signal axis. Cell. Death Dis. 9 (9), 928. doi:10.1038/s41419-018-0988-9
Taban, Q., Mumtaz, P. T., Masoodi, K. Z., Haq, E., and Ahmad, S. M. (2022). Scavenger receptors in host defense: from functional aspects to mode of action. Cell. Commun. Signal 20 (1), 2. doi:10.1186/s12964-021-00812-0
Taki, M., Abiko, K., Baba, T., Hamanishi, J., Yamaguchi, K., Murakami, R., et al. (2018). Snail promotes ovarian cancer progression by recruiting myeloid-derived suppressor cells via CXCR2 ligand upregulation. Nat. Commun. 9 (1), 1685. doi:10.1038/s41467-018-03966-7
Tan, I. L., Arifa, R. D. N., Rallapalli, H., Kana, V., Lao, Z., Sanghrajka, R. M., et al. (2021). CSF1R inhibition depletes tumor-associated macrophages and attenuates tumor progression in a mouse sonic Hedgehog-Medulloblastoma model. Oncogene 40 (2), 396–407. doi:10.1038/s41388-020-01536-0
Tang, P. M., Zhou, S., Meng, X. M., Wang, Q. M., Li, C. J., Lian, G. Y., et al. (2017). Smad3 promotes cancer progression by inhibiting E4BP4-mediated NK cell development. Nat. Commun. 8, 14677. doi:10.1038/ncomms14677
Tang, P. M., Nikolic-Paterson, D. J., and Lan, H. Y. (2019). Macrophages: versatile players in renal inflammation and fibrosis. Nat. Rev. Nephrol. 15 (3), 144–158. doi:10.1038/s41581-019-0110-2
Tang, P. M., Zhang, Y. Y., Xiao, J., Tang, P., Chung, J. Y. F., Li, J., et al. (2020). Neural transcription factor Pou4f1 promotes renal fibrosis via macrophage-myofibroblast transition. Proc. Natl. Acad. Sci. U. S. A. 117 (34), 20741–20752. doi:10.1073/pnas.1917663117
Tang, P. M., Zhang, Y. Y., Hung, J. S. C., Chung, J. Y. F., Huang, X. R., To, K. F., et al. (2021a). DPP4/CD32b/NF-κB circuit: a novel druggable target for inhibiting CRP-driven diabetic nephropathy. Mol. Ther. 29 (1), 365–375. doi:10.1016/j.ymthe.2020.08.017
Tang, P. C., Chung, J. Y. F., Xue, V. W. W., Xiao, J., Meng, X. M., Huang, X. R., et al. (2021b). Smad3 promotes cancer-associated fibroblasts generation via macrophage-myofibroblast transition. Adv. Sci. (Weinh) 9, e2101235. doi:10.1002/advs.202101235
Tang, P. C., Chung, J. Y. F., Xue, V. W. W., Xiao, J., Meng, X. M., Huang, X. R., et al. (2022a). Smad3 promotes cancer-associated fibroblasts generation via macrophage-myofibroblast transition. Adv. Sci. (Weinh) 9 (1), e2101235. doi:10.1002/advs.202101235
Tang, P. C., Chung, J. Y. F., Liao, J., Chan, M. K. K., Chan, A. S. W., Cheng, G., et al. (2022b). Single-cell RNA sequencing uncovers a neuron-like macrophage subset associated with cancer pain. Sci. Adv. 8 (40), eabn5535. doi:10.1126/sciadv.abn5535
Tang, X. X., Shimada, H., and Ikegaki, N. (2022c). Macrophage-mediated anti-tumor immunity against high-risk neuroblastoma. Genes Immun. 23 (3-4), 129–140. doi:10.1038/s41435-022-00172-w
Tang, P. C., Zhang, Y. Y., Li, J. S. F., Chan, M., Chen, J., Tang, Y., et al. (2022d). LncRNA-dependent mechanisms of transforming growth factor-β: from tissue fibrosis to cancer progression. Noncoding RNA 8 (3), 36. doi:10.3390/ncrna8030036
Taniguchi, S., Elhance, A., Van Duzer, A., Kumar, S., Leitenberger, J. J., and Oshimori, N. (2020). Tumor-initiating cells establish an IL-33-TGF-β niche signaling loop to promote cancer progression. Science 369 (6501), eaay1813. doi:10.1126/science.aay1813
Tanito, K., Nii, T., Yokoyama, Y., Oishi, H., Shibata, M., Hijii, S., et al. (2023). Engineered macrophages acting as a trigger to induce inflammation only in tumor tissues. J. Control Release 361, 885–895. doi:10.1016/j.jconrel.2023.04.010
Teng, K. Y., Han, J., Zhang, X., Hsu, S. H., He, S., Wani, N. A., et al. (2017). Blocking the CCL2-CCR2 Axis using CCL2-neutralizing antibody is an effective therapy for hepatocellular cancer in a mouse model. Mol. Cancer Ther. 16 (2), 312–322. doi:10.1158/1535-7163.MCT-16-0124
Tian, K., Du, G., Wang, X., Wu, X., Li, L., Liu, W., et al. (2022). MMP-9 secreted by M2-type macrophages promotes Wilms' tumour metastasis through the PI3K/AKT pathway. Mol. Biol. Rep. 49 (5), 3469–3480. doi:10.1007/s11033-022-07184-9
Tiwari, J. K., Negi, S., Kashyap, M., Nizamuddin, S., Singh, A., and Khattri, A. (2021). Pan-cancer analysis shows enrichment of macrophages, overexpression of checkpoint molecules, inhibitory cytokines, and immune exhaustion signatures in EMT-high tumors. Front. Oncol. 11, 793881. doi:10.3389/fonc.2021.793881
Tlili, A., Pintard, C., Hurtado-Nedelec, M., Liu, D., Marzaioli, V., Thieblemont, N., et al. (2023). ROCK2 interacts with p22phox to phosphorylate p47phox and to control NADPH oxidase activation in human monocytes. Proc. Natl. Acad. Sci. U. S. A. 120 (3), e2209184120. doi:10.1073/pnas.2209184120
Tomlins, S. A., Khazanov, N. A., Bulen, B. J., Hovelson, D. H., Shreve, M. J., Lamb, L. E., et al. (2023). Development and validation of an integrative pan-solid tumor predictor of PD-1/PD-L1 blockade benefit. Commun. Med. (Lond) 3 (1), 14. doi:10.1038/s43856-023-00243-7
Trzupek, D., Dunstan, M., Cutler, A. J., Lee, M., Godfrey, L., Jarvis, L., et al. (2020). Discovery of CD80 and CD86 as recent activation markers on regulatory T cells by protein-RNA single-cell analysis. Genome Med. 12 (1), 55. doi:10.1186/s13073-020-00756-z
Tu, M. M., Abdel-Hafiz, H. A., Jones, R. T., Jean, A., Hoff, K. J., Duex, J. E., et al. (2020). Inhibition of the CCL2 receptor, CCR2, enhances tumor response to immune checkpoint therapy. Commun. Biol. 3 (1), 720. doi:10.1038/s42003-020-01441-y
Tu, M., Klein, L., Espinet, E., Georgomanolis, T., Wegwitz, F., Li, X., et al. (2021a). TNF-α-producing macrophages determine subtype identity and prognosis via AP1 enhancer reprogramming in pancreatic cancer. Nat. Cancer 2 (11), 1185–1203. doi:10.1038/s43018-021-00258-w
Tu, D., Dou, J., Wang, M., Zhuang, H., and Zhang, X. (2021b). M2 macrophages contribute to cell proliferation and migration of breast cancer. Cell. Biol. Int. 45 (4), 831–838. doi:10.1002/cbin.11528
Turrell, F. K., Orha, R., Guppy, N. J., Gillespie, A., Guelbert, M., Starling, C., et al. (2023). Age-associated microenvironmental changes highlight the role of PDGF-C in ER(+) breast cancer metastatic relapse. Nat. Cancer 4 (4), 468–484. doi:10.1038/s43018-023-00525-y
van der Sluis, T. C., Beyrend, G., van der Gracht, E. T. I., Abdelaal, T., Jochems, S. P., Belderbos, R. A., et al. (2023). OX40 agonism enhances PD-L1 checkpoint blockade by shifting the cytotoxic T cell differentiation spectrum. Cell. Rep. Med. 4 (3), 100939. doi:10.1016/j.xcrm.2023.100939
van Elsas, M. J., Labrie, C., Etzerodt, A., Charoentong, P., van Stigt Thans, J. J. C., Van Hall, T., et al. (2023). Invasive margin tissue-resident macrophages of high CD163 expression impede responses to T cell-based immunotherapy. J. Immunother. Cancer 11 (3), e006433. doi:10.1136/jitc-2022-006433
Vayrynen, J. P., Haruki, K., Lau, M. C., Väyrynen, S. A., Zhong, R., Dias Costa, A., et al. (2021). The prognostic role of macrophage polarization in the colorectal cancer microenvironment. Cancer Immunol. Res. 9 (1), 8–19. doi:10.1158/2326-6066.CIR-20-0527
Vidyarthi, A., Khan, N., Agnihotri, T., Negi, S., Das, D. K., Aqdas, M., et al. (2018). TLR-3 stimulation skews M2 macrophages to M1 through IFN-αβ signaling and restricts tumor progression. Front. Immunol. 9, 1650. doi:10.3389/fimmu.2018.01650
Viitala, M., Virtakoivu, R., Tadayon, S., Rannikko, J., Jalkanen, S., and Hollmén, M. (2019). Immunotherapeutic blockade of macrophage clever-1 reactivates the CD8+ T-cell response against immunosuppressive tumors. Clin. Cancer Res. 25 (11), 3289–3303. doi:10.1158/1078-0432.CCR-18-3016
Virtakoivu, R., Rannikko, J. H., Viitala, M., Vaura, F., Takeda, A., Lönnberg, T., et al. (2021). Systemic blockade of clever-1 elicits lymphocyte activation alongside checkpoint molecule downregulation in patients with solid tumors: results from a phase I/II clinical trial. Clin. Cancer Res. 27 (15), 4205–4220. doi:10.1158/1078-0432.CCR-20-4862
Wang, X., and Khalil, R. A. (2018). Matrix metalloproteinases, vascular remodeling, and vascular disease. Adv. Pharmacol. 81, 241–330. doi:10.1016/bs.apha.2017.08.002
Wang, H., Sun, Y., Zhou, X., Chen, C., Jiao, L., Li, W., et al. (2020). CD47/SIRPα blocking peptide identification and synergistic effect with irradiation for cancer immunotherapy. J. Immunother. Cancer 8 (2), e000905. doi:10.1136/jitc-2020-000905
Wang, Z., Guan, D., Huo, J., Biswas, S. K., Huang, Y., Yang, Y., et al. (2021a). IL-10 enhances human natural killer cell effector functions via metabolic reprogramming regulated by mTORC1 signaling. Front. Immunol. 12, 619195. doi:10.3389/fimmu.2021.619195
Wang, G., Xu, D., Zhang, Z., Li, X., Shi, J., Sun, J., et al. (2021b). The pan-cancer landscape of crosstalk between epithelial-mesenchymal transition and immune evasion relevant to prognosis and immunotherapy response. NPJ Precis. Oncol. 5 (1), 56. doi:10.1038/s41698-021-00200-4
Wang, K., Donnelly, C. R., Jiang, C., Liao, Y., Luo, X., Tao, X., et al. (2021c). STING suppresses bone cancer pain via immune and neuronal modulation. Nat. Commun. 12 (1), 4558. doi:10.1038/s41467-021-24867-2
Wang, S., Yang, Y., Ma, P., Zha, Y., Zhang, J., Lei, A., et al. (2022). CAR-macrophage: an extensive immune enhancer to fight cancer. EBioMedicine 76, 103873. doi:10.1016/j.ebiom.2022.103873
Wang, C., Barnoud, C., Cenerenti, M., Sun, M., Caffa, I., Kizil, B., et al. (2023). Dendritic cells direct circadian anti-tumour immune responses. Nature 614 (7946), 136–143. doi:10.1038/s41586-022-05605-0
Wei, Z., Oh, J., Flavell, R. A., and Crawford, J. M. (2022). LACC1 bridges NOS2 and polyamine metabolism in inflammatory macrophages. Nature 609 (7926), 348–353. doi:10.1038/s41586-022-05111-3
Wen, Y., Lambrecht, J., Ju, C., and Tacke, F. (2021). Hepatic macrophages in liver homeostasis and diseases-diversity, plasticity and therapeutic opportunities. Cell. Mol. Immunol. 18 (1), 45–56. doi:10.1038/s41423-020-00558-8
Willingham, S. B., Volkmer, J. P., Gentles, A. J., Sahoo, D., Dalerba, P., Mitra, S. S., et al. (2012). The CD47-signal regulatory protein alpha (SIRPa) interaction is a therapeutic target for human solid tumors. Proc. Natl. Acad. Sci. U. S. A. 109 (17), 6662–6667. doi:10.1073/pnas.1121623109
Winkler, J., Abisoye-Ogunniyan, A., Metcalf, K. J., and Werb, Z. (2020). Concepts of extracellular matrix remodelling in tumour progression and metastasis. Nat. Commun. 11 (1), 5120. doi:10.1038/s41467-020-18794-x
Wu, H., Zhong, Z., Wang, A., Yuan, C., Ning, K., Hu, H., et al. (2020a). LncRNA FTX represses the progression of non-alcoholic fatty liver disease to hepatocellular carcinoma via regulating the M1/M2 polarization of Kupffer cells. Cancer Cell. Int. 20, 266. doi:10.1186/s12935-020-01354-0
Wu, J., Yang, H., Cheng, J., Zhang, L., Ke, Y., Zhu, Y., et al. (2020b). Knockdown of milk-fat globule EGF factor-8 suppresses glioma progression in GL261 glioma cells by repressing microglial M2 polarization. J. Cell. Physiol. 235 (11), 8679–8690. doi:10.1002/jcp.29712
Wu, X., Wang, Z., Shi, J., Yu, X., Li, C., Liu, J., et al. (2022a). Macrophage polarization toward M1 phenotype through NF-κB signaling in patients with Behçet's disease. Arthritis Res. Ther. 24 (1), 249. doi:10.1186/s13075-022-02938-z
Wu, M., Zhang, X., Zhang, W., Chiou, Y. S., Qian, W., Liu, X., et al. (2022b). Cancer stem cell regulated phenotypic plasticity protects metastasized cancer cells from ferroptosis. Nat. Commun. 13 (1), 1371. doi:10.1038/s41467-022-29018-9
Wu, B., Shi, X., Jiang, M., and Liu, H. (2023). Cross-talk between cancer stem cells and immune cells: potential therapeutic targets in the tumor immune microenvironment. Mol. Cancer 22 (1), 38. doi:10.1186/s12943-023-01748-4
Xia, Q., Jia, J., Hu, C., Lu, J., Li, J., Xu, H., et al. (2022). Tumor-associated macrophages promote PD-L1 expression in tumor cells by regulating PKM2 nuclear translocation in pancreatic ductal adenocarcinoma. Oncogene 41 (6), 865–877. doi:10.1038/s41388-021-02133-5
Xiao, L., He, Y., Peng, F., Yang, J., and Yuan, C. (2020). Endometrial cancer cells promote M2-like macrophage polarization by delivering exosomal miRNA-21 under hypoxia condition. J. Immunol. Res. 2020, 9731049. doi:10.1155/2020/9731049
Xie, Y., Chen, Z., Zhong, Q., Zheng, Z., Chen, Y., Shangguan, W., et al. (2021). M2 macrophages secrete CXCL13 to promote renal cell carcinoma migration, invasion, and EMT. Cancer Cell. Int. 21 (1), 677. doi:10.1186/s12935-021-02381-1
Xie, T., Fu, D. J., Li, Z. M., Lv, D. J., Song, X. L., Yu, Y. Z., et al. (2022). CircSMARCC1 facilitates tumor progression by disrupting the crosstalk between prostate cancer cells and tumor-associated macrophages via miR-1322/CCL20/CCR6 signaling. Mol. Cancer 21 (1), 173. doi:10.1186/s12943-022-01630-9
Xu, M., Wang, X., Li, Y., Geng, X., Jia, X., Zhang, L., et al. (2021a). Arachidonic acid metabolism controls macrophage alternative activation through regulating oxidative phosphorylation in PPARγ dependent manner. Front. Immunol. 12, 618501. doi:10.3389/fimmu.2021.618501
Xu, M., Wang, Y., Xia, R., Wei, Y., and Wei, X. (2021b). Role of the CCL2-CCR2 signalling axis in cancer: mechanisms and therapeutic targeting. Cell. Prolif. 54 (10), e13115. doi:10.1111/cpr.13115
Xu, Y., Zeng, H., Jin, K., Liu, Z., Zhu, Y., Xu, L., et al. (2022). Immunosuppressive tumor-associated macrophages expressing interlukin-10 conferred poor prognosis and therapeutic vulnerability in patients with muscle-invasive bladder cancer. J. Immunother. Cancer 10 (3), e003416. doi:10.1136/jitc-2021-003416
Xue, V. W., Chung, J. Y. F., Tang, P. C. T., Chan, A. S. W., To, T. H. W., Chung, J. S. Y., et al. (2021). USMB-shMincle: a virus-free gene therapy for blocking M1/M2 polarization of tumor-associated macrophages. Mol. Ther. Oncolytics 23, 26–37. doi:10.1016/j.omto.2021.08.010
Yang, H. D., Kim, H. S., Kim, S. Y., Na, M. J., Yang, G., Eun, J. W., et al. (2019). HDAC6 suppresses let-7i-5p to elicit TSP1/CD47-mediated anti-tumorigenesis and phagocytosis of hepatocellular carcinoma. Hepatology 70 (4), 1262–1279. doi:10.1002/hep.30657
Yang, L., Shi, P., Zhao, G., Xu, J., Peng, W., Zhang, J., et al. (2020). Targeting cancer stem cell pathways for cancer therapy. Signal Transduct. Target Ther. 5 (1), 8. doi:10.1038/s41392-020-0110-5
Yang, K., Xie, Y., Xue, L., Li, F., Luo, C., Liang, W., et al. (2023a). M2 tumor-associated macrophage mediates the maintenance of stemness to promote cisplatin resistance by secreting TGF-β1 in esophageal squamous cell carcinoma. J. Transl. Med. 21 (1), 26. doi:10.1186/s12967-022-03863-0
Yang, Q., Dai, H., Cheng, Y., Wang, B., Xu, J., Zhang, Y., et al. (2023b). Oral feeding of nanoplastics affects brain function of mice by inducing macrophage IL-1 signal in the intestine. Cell. Rep. 42 (4), 112346. doi:10.1016/j.celrep.2023.112346
Yao, R. R., Li, J. H., Zhang, R., Chen, R. X., and Wang, Y. H. (2018). M2-polarized tumor-associated macrophages facilitated migration and epithelial-mesenchymal transition of HCC cells via the TLR4/STAT3 signaling pathway. World J. Surg. Oncol. 16 (1), 9. doi:10.1186/s12957-018-1312-y
Yao, Y., Zhang, T., Ru, X., Qian, J., Tong, Z., Li, X., et al. (2020). Constitutively expressed MHC class Ib molecules regulate macrophage M2b polarization and sepsis severity in irradiated mice. J. Leukoc. Biol. 107 (3), 445–453. doi:10.1002/JLB.1AB1219-125RR
Yau, E., Yang, L., Chen, Y., Umstead, T. M., Atkins, H., Katz, Z. E., et al. (2023). Surfactant protein A alters endosomal trafficking of influenza A virus in macrophages. Front. Immunol. 14, 919800. doi:10.3389/fimmu.2023.919800
Yen, J. H., Huang, W. C., Lin, S. C., Huang, Y. W., Chio, W. T., Tsay, G. J., et al. (2022). Metabolic remodeling in tumor-associated macrophages contributing to antitumor activity of cryptotanshinone by regulating TRAF6-ASK1 axis. Mol. Ther. Oncolytics 26, 158–174. doi:10.1016/j.omto.2022.06.008
Yogev, N., Bedke, T., Kobayashi, Y., Brockmann, L., Lukas, D., Regen, T., et al. (2022). CD4(+) T-cell-derived IL-10 promotes CNS inflammation in mice by sustaining effector T cell survival. Cell. Rep. 38 (13), 110565. doi:10.1016/j.celrep.2022.110565
Yu, L., Yang, F., Zhang, F., Guo, D., Li, L., Wang, X., et al. (2018). CD69 enhances immunosuppressive function of regulatory T-cells and attenuates colitis by prompting IL-10 production. Cell. Death Dis. 9 (9), 905. doi:10.1038/s41419-018-0927-9
Yu, Y., Ke, L., Xia, W. X., Xiang, Y., Lv, X., and Bu, J. (2019). Elevated levels of TNF-α and decreased levels of CD68-positive macrophages in primary tumor tissues are unfavorable for the survival of patients with nasopharyngeal carcinoma. Technol. Cancer Res. Treat. 18, 1533033819874807. doi:10.1177/1533033819874807
Yu, Q., Wang, Y., Dong, L., He, Y., Liu, R., Yang, Q., et al. (2020). Regulations of glycolytic activities on macrophages functions in tumor and infectious inflammation. Front. Cell. Infect. Microbiol. 10, 287. doi:10.3389/fcimb.2020.00287
Yuan, S., Stewart, K. S., Yang, Y., Abdusselamoglu, M. D., Parigi, S. M., Feinberg, T. Y., et al. (2022). Ras drives malignancy through stem cell crosstalk with the microenvironment. Nature 612 (7940), 555–563. doi:10.1038/s41586-022-05475-6
Zappasodi, R., Serganova, I., Cohen, I. J., Maeda, M., Shindo, M., Senbabaoglu, Y., et al. (2021). CTLA-4 blockade drives loss of T(reg) stability in glycolysis-low tumours. Nature 591 (7851), 652–658. doi:10.1038/s41586-021-03326-4
Zeng, X. Y., Xie, H., Yuan, J., Jiang, X. Y., Yong, J. H., Zeng, D., et al. (2019). M2-like tumor-associated macrophages-secreted EGF promotes epithelial ovarian cancer metastasis via activating EGFR-ERK signaling and suppressing lncRNA LIMT expression. Cancer Biol. Ther. 20 (7), 956–966. doi:10.1080/15384047.2018.1564567
Zhang, F., Parayath, N. N., Ene, C. I., Stephan, S. B., Koehne, A. L., Coon, M. E., et al. (2019). Genetic programming of macrophages to perform anti-tumor functions using targeted mRNA nanocarriers. Nat. Commun. 10 (1), 3974. doi:10.1038/s41467-019-11911-5
Zhang, F., Mears, J. R., Shakib, L., Beynor, J. I., Shanaj, S., Korsunsky, I., et al. (2021a). IFN-γ and TNF-α drive a CXCL10+ CCL2+ macrophage phenotype expanded in severe COVID-19 lungs and inflammatory diseases with tissue inflammation. Genome Med. 13 (1), 64. doi:10.1186/s13073-021-00881-3
Zhang, B., Zhang, Y., Jiang, X., Su, H., Wang, Q., Wudu, M., et al. (2021b). JMJD8 promotes malignant progression of lung cancer by maintaining EGFR stability and EGFR/PI3K/AKT pathway activation. J. Cancer 12 (4), 976–987. doi:10.7150/jca.50234
Zhang, S., Rautela, J., Bediaga, N. G., Kolesnik, T. B., You, Y., Nie, J., et al. (2023). CIS controls the functional polarization of GM-CSF-derived macrophages. Cell. Mol. Immunol. 20 (1), 65–79. doi:10.1038/s41423-022-00957-z
Zhao, S., Mi, Y., Guan, B., Zheng, B., Wei, P., Gu, Y., et al. (2020). Tumor-derived exosomal miR-934 induces macrophage M2 polarization to promote liver metastasis of colorectal cancer. J. Hematol. Oncol. 13 (1), 156. doi:10.1186/s13045-020-00991-2
Zhao, D., Yang, F., Wang, Y., Li, S., Li, Y., Hou, F., et al. (2022a). ALK1 signaling is required for the homeostasis of Kupffer cells and prevention of bacterial infection. J. Clin. Invest. 132 (3), e150489. doi:10.1172/JCI150489
Zhao, X., Di, Q., Liu, H., Quan, J., Ling, J., Zhao, Z., et al. (2022b). MEF2C promotes M1 macrophage polarization and Th1 responses. Cell. Mol. Immunol. 19 (4), 540–553. doi:10.1038/s41423-022-00841-w
Zhou, Z., Peng, Y., Wu, X., Meng, S., Yu, W., Zhao, J., et al. (2019). CCL18 secreted from M2 macrophages promotes migration and invasion via the PI3K/Akt pathway in gallbladder cancer. Cell. Oncol. (Dordr) 42 (1), 81–92. doi:10.1007/s13402-018-0410-8
Zhou, H. M., Zhang, J. G., Zhang, X., and Li, Q. (2021). Targeting cancer stem cells for reversing therapy resistance: mechanism, signaling, and prospective agents. Signal Transduct. Target Ther. 6 (1), 62. doi:10.1038/s41392-020-00430-1
Zhou, Y., Takano, T., Li, X., Wang, Y., Wang, R., Zhu, Z., et al. (2022). β-elemene regulates M1-M2 macrophage balance through the ERK/JNK/P38 MAPK signaling pathway. Commun. Biol. 5 (1), 519. doi:10.1038/s42003-022-03369-x
Zhou, Y., Cheng, L., Liu, L., and Li, X. (2023a). NK cells are never alone: crosstalk and communication in tumour microenvironments. Mol. Cancer 22 (1), 34. doi:10.1186/s12943-023-01737-7
Zhou, H., Gan, M., Jin, X., Dai, M., Wang, Y., Lei, Y., et al. (2023b). [Corrigendum] miR‑382 inhibits breast cancer progression and metastasis by affecting the M2 polarization of tumor‑associated macrophages by targeting PGC‑1α. Int. J. Oncol. 62 (1), 1. doi:10.3892/ijo.2022.5449
Keywords: tumour-associated macrophages, tumour microenvironment, immunotherapy, macrophage plasticity, macrophage-myofibroblast transition, macrophage-neuron transition
Citation: Ji ZZ, Chan MK-K, Chan AS-W, Leung K-T, Jiang X, To K-F, Wu Y and Tang PM-K (2023) Tumour-associated macrophages: versatile players in the tumour microenvironment. Front. Cell Dev. Biol. 11:1261749. doi: 10.3389/fcell.2023.1261749
Received: 19 July 2023; Accepted: 12 October 2023;
Published: 26 October 2023.
Edited by:
Yu Zhao, Capital Medical University, ChinaReviewed by:
Yanruide Li, University of California, Los Angeles, United StatesCopyright © 2023 Ji, Chan, Chan, Leung, Jiang, To, Wu and Tang. This is an open-access article distributed under the terms of the Creative Commons Attribution License (CC BY). The use, distribution or reproduction in other forums is permitted, provided the original author(s) and the copyright owner(s) are credited and that the original publication in this journal is cited, in accordance with accepted academic practice. No use, distribution or reproduction is permitted which does not comply with these terms.
*Correspondence: Patrick Ming-Kuen Tang, patrick.tang@cuhk.edu.hk
†These authors have contributed equally to this work