STING in tumors: a focus on non-innate immune pathways
- 1Department of Plastic Surgery, China-Japan Union Hospital, Jilin University, Changchun, China
- 2Key Laboratory of Pathobiology, Ministry of Education, and Department of Biomedical Science, College of Basic Medical Sciences, Jilin University, Changchun, China
Cyclic GMP-AMP synthase (cGAS) and downstream stimulator of interferon genes (STING) are involved in mediating innate immunity by promoting the release of interferon and other inflammatory factors. Mitochondrial DNA (mtDNA) with a double-stranded structure has greater efficiency and sensitivity in being detected by DNA sensors and thus has an important role in the activation of the cGAS-STING pathway. Many previous findings suggest that the cGAS-STING pathway-mediated innate immune regulation is the most important aspect affecting tumor survival, not only in its anti-tumor role but also in shaping the immunosuppressive tumor microenvironment (TME) through a variety of pathways. However, recent studies have shown that STING regulation of non-immune pathways is equally profound and also involved in tumor cell progression. In this paper, we will focus on the non-innate immune system pathways, in which the cGAS-STING pathway also plays an important role in cancer.
1 Introduction
cGAS is highly conserved and is the double-stranded DNA (dsDNA) sensor, which is responsible for monitoring the changes in cytoplasmic DNA content in most mammalian cells (Sun et al., 2013; Ablasser and Chen, 2019). In contrast to Toll-like receptor 9 and NLRP3, whose expression appears to be restricted to immune cells, cGAS and the downstream STING signaling are widely expressed in most cell types (Chen et al., 2016a; Riley and Tait, 2020). It has been shown that cytoplasmic leakage of mtDNA is the major source of cytoplasmic dsDNA in ATM-deficient tumor cells (Hu et al., 2021a), suggesting that mtDNA may play an important role in the innate immunity of tumor cells. The mtDNA is located near the five complexes that transfer electrons across the inner mitochondrial membrane (IMM) to ensure efficient synthesis of the proteins encoded by the mitochondrial genes, but the electron transport chain as a major source of mitochondrial reactive oxygen species (ROS) will result in mtDNA being more susceptible to oxidative damage and leakage into the cytoplasm. The cyclic structure of mtDNA and the presence of unmethylated CpG motifs allow cGAS to recognize mtDNA leakage into the cytoplasm as a “foreign” molecule that triggers an inflammatory response (West and Shadel, 2017; Kausar et al., 2020; De Gaetano et al., 2021), which is then involved in tumorigenesis through subsequent STING signaling. By analyzing The Cancer Genome Atlas of 18 malignancies, several scientists found that the expression of four key molecules in the cGAS-STING pathway, MB21D1 which encodes cGAS, TMEM173 which encodes STING, as well as TANK-binding kinase 1 (TBK1) and interferon-regulating factor 3 (IRF3), are significantly upregulated in almost all detected cancer types compared to normal control tissues, suggesting that cGAS-STING and the signaling molecules may be activated in all cancer processes (An et al., 2019).
It is now widely accepted that the cGAS-STING signaling cascade may rely primarily on synthetic interferons (IFNs) to exert anti-tumor effects, but recent studies have found that it also has significant regulatory effects on non-immune pathways and influences tumor progression. At the same time, it has been found that there is significant heterogeneity in the outcomes exhibited by tumor cells upon STING activation, and the mechanism of this double-edged role in tumor progression deserves to be explored. Therefore, in this review, we summarize the main mechanisms of mtDNA escaping from mitochondria and the recent advances in the impact on tumor cells after the occurrence of horizontal metastasis, with a focus on the role of the subsequent non-innate immunoregulatory pathways of the cGAS-STING pathway in tumor progression and the potential factors that influence STING bi-directionality.
2 Overview of cGAS-STING
cGAS is a member of the nucleotidyltransferase family and is normally present in the cytoplasm as an inactive protein, and current evidence suggests that cGAS also has a diverse cellular distribution (Kuchta et al., 2009; Sun et al., 2013; Wu et al., 2013; Hopfner and Hornung, 2020). Jiang et al. showed that cGAS is also constitutively present in the nucleus. When it is activated, it accelerates genomic instability, the formation of micronuclei (MNi), and cell death under stress conditions by inhibiting homologous recombination pathways (Jiang et al., 2019). This dual function of cGAS as an innate immune sensor in the cytoplasm and a negative regulator of DNA repair in the nucleus highlights the importance of cGAS in cells. Briefly, After the recognition and binding of free cytoplasmic DNA to form the complexes in the cytoplasm, there has been a conformational change in the active site of cGAS, which causes cGAS activation. Then the cyclic guanosine monophosphate-adenosine monophosphate (cGAMP) is synthesized by cGAS with intracytoplasmic ATP and GTP as raw materials. As the secondary messenger, cGAMP or cyclic dinucleotides (CDNs) bind to the adapter protein STING anchored to the endoplasmic reticulum (ER) (Ablasser et al., 2013; Gao et al., 2013a; Diner et al., 2013; Sun et al., 2013; Zhang et al., 2013). Upon binding to cGAMP, STING undergoes extensive conformational rearrangements (Huang et al., 2012; Shang et al., 2012; Shu et al., 2012; Gao et al., 2013b; Ergun et al., 2019; Shang et al., 2019). After conformational rearrangement, STING is translocated from the ER to the Golgi through the ER-Golgi intermediate compartment (Dobbs et al., 2015). Upon arrival at the Golgi compartment, STING is palmitoylated at two cysteine residues (Cys88 and Cys91) and recruits TBK1 (Mukai et al., 2016). TBK1 then phosphorylates itself. STING and IRF3 dimerize and enter the nucleus, triggering type I interferon production (Hopfner and Hornung, 2020). Phosphorylated TBK1 and its cognate IκB kinase (IKK) also mediate activation of the IKK complex, which in turn activates the atypical NF-κB pathway to induce the production of other inflammatory cytokines (Smale, 2010). For detailed mechanisms, see the excellent review by Hopfner and Hornung (2020).
3 Regulation and modification of cGAS and STING expression
cGAS is constitutively expressed in most cell types. Thus, unlike other pattern recognition receptor systems, the levels of cGAS and its ability to be activated are not regulated at the transcriptional level. Conversely, many post-translational mechanisms have been shown to play an important role in regulating the activity of this receptor toward its ligand, enzymatic activity, or half-life (Hopfner and Hornung, 2020). cGAS and STING undergo several types of post-translational modifications, including protein hydrolysis (Wang et al., 2017), acetylation (Dai et al., 2019), glutamylation (Xia et al., 2016a), ubiquitination (Zhang et al., 2012), sumoylation (Hu et al., 2016), and phosphorylation (Tanaka and Chen, 2012). These post-translational modifications affect cGAS and STING either by direct modification or by cleavage of active site residues. In particular, STING signaling is defective in a variety of cancers (e.g., colon cancer and melanoma), and its behavior may allow damaged cells to escape immune surveillance (Xia et al., 2016b; Xia et al., 2016c). Konno et al. found that cGAS or STING genes were mutated in a variety of human tumors by searching the cBioPortal database. Among them, cGAS missense mutants R376Q and E383K lost the ability to produce cGAMP upon binding to dsDNA, while STING mutants (R169W and P203S) were unable to promote the production of oncogene-induced pro-inflammatory cytokines. Hypermethylation of cGAS or STING promoter regions is present in several types of tumor samples, resulting in cGAS or STING repression (Konno et al., 2018). It has been shown that this methylation process is reversible, and in STING-deficient melanoma cell lines, demethylation-mediated restoration of STING signaling can increase the antigenicity of MHC-like molecules by upregulating them, thereby enhancing their recognition and killing by cytotoxic T cells (Falahat et al., 2021).
4 mtDNA is a potent inducer of the cGAS-STING pathway
One of the characteristics of tumor cells is the massive replication of chromosomes in the nucleus, which can lead to the leakage of genomic DNA into the cytoplasm (Chin et al., 2020). When the mitotic process of cells is damaged endogenously or exogenously, some chromatin segments may be missegregated into the cytoplasm, either passively or actively, forming one or more spatially separated MNi (Guo et al., 2019). Gupta et al. found Cisplatin-induced massive MNi production in cutaneous squamous carcinoma (Gupta et al., 2011). Using high-resolution live-cell imaging, Hatch et al. found that spontaneous rupture of the micronuclear envelope can occur in the majority of MNi (Hatch et al., 2013), and that ruptured MNi are an important source of cytoplasmic self-DNA (mainly dsDNA) (Harding et al., 2017). Approximately 55% of metastatic tumor-derived cells were reported to be cGAS positive (Bakhoum et al., 2018). Similarly, cytoplasmic translocation of mtDNA has been detected in many disease states and has been associated with cancer progression (Liu et al., 2019b; Piantadosi, 2020). In particular, when tumor cells are often subjected to multiple physicochemical insults during treatment, their mitochondrial damage is further aggravated and more mtDNA is released into the cytoplasm (Cheng et al., 2020; Zhu et al., 2022). Numerous studies have shown that mtDNA is a potent inducer of cGAS-STING signaling (Wu et al., 2021b). Interestingly, Tigano et al. used the inducible restriction endonuclease AsiSi to selectively induce DNA double-strand breaks (DSBs) in the nucleus and ionization radiation (IR) to induce DSBs in both mtDNA and nuclear DNA. The results showed that although AsiSi and IR caused the cells to produce similar levels of cGAS-positive MNi (∼15%), the induction of nuclear DNA and mtDNA co-damage showed stronger interferon-stimulated gene activation and significantly increased transcript levels than the induction of nuclear DNA damage alone. This suggests that damaged mtDNA synergizes with MNi to produce a stronger type I IFN response (Tigano et al., 2021). The reason for the strong activation of cGAS by mtDNA may be related to the fact that mtDNA is more susceptible to damage due to its high copy number and inefficient repair system (Wu et al., 2021a). In addition, the structural features of mtDNA, i.e., shorter circular DNA, and no histone hindrance, seem to be the critical factors (Andreeva et al., 2017). However, it has also been shown that cytoplasmic chromatin is equally competent for cGAS-STING activation (Dou et al., 2017). Structurally, the N-terminus of cGAS is required for nuclear chromatin sensing, not mtDNA (Li et al., 2021c). Compared with naked dsDNA, reconstituted nucleosomes in vitro have higher affinities for cGAS, but lower capacities to activate it (Wang et al., 2020a). The reason for this may be that nucleosomes interfere to some extent with the formation of this DNA structure, and thus some studies have used nucleosomes as markers to distinguish their DNA from pathogenic DNA (Zierhut et al., 2014; Zierhut and Funabiki, 2015). Given that mtDNA is an important component of dsDNA and plays an important role in cGAS-STING activation, the next section will explore the main mechanisms of mtDNA leakage into the cytosol in the context of the current findings.
5 The main mechanism of mtDNA leakage
Mitochondrial outer membrane permeability (MOMP), initiated by endogenous or mitochondrial apoptotic processes, is one of the major forms of mtDNA release. Previous studies have long shown that free Bax and Bak oligomerize in the outer mitochondrial membrane (OMM) during apoptosis to form a proteic pore, which can lead to the release of mitochondrial contents such as cyt C, mtDNA, etc., into the cytosol (Chipuk et al., 2006). However, since the IMM is thought to remain intact during apoptosis (Bernardi et al., 2015), it is particularly mysterious how mtDNA escapes from the mitochondria during this process. McArthur et al. examined mitochondria from mouse embryonic fibroblasts using live-cell lattice light-sheet microscopy and found that BAX/BAK activation and subsequent cyt C release are followed by mitochondrial lattice rupture and the appearance of large BAX/BAK pores on the OMM. These large pores allow IMM-carrying mitochondrial matrix components, including the mitochondrial genome, to protrude into the cytosol, ultimately leading to the release of contents such as mtDNA (McArthur et al., 2018). The study by Riley et al. also confirmed the above and indicated that after the occurrence of BAX-dependent MOMP, mitochondrial inner membrane permeability (MIMP) may also follow (Riley et al., 2018). It is noteworthy that tBID, a member of the BH3-only family, can mediate MOMP in a BAX/BAK-independent manner. The finding defines tBID as an effector of mitochondrial permeability in apoptosis (Flores-Romero et al., 2022) and may influence the process of mtDNA release through MOMP.
Another important pathway for mtDNA escape from mitochondria is the opening of the mitochondrial permeability transition pore (mPTP). The opening of mPTP leads to increased IMM permeability and thus to changes in mitochondrial permeability (Bernardi and Di Lisa, 2015; Bernardi, 2018). It has been shown that mtDNA fragments are released from the brains of irradiated mice by briefly opening the mPTP (Patrushev et al., 2006). Under oxidative stress conditions, DNA from liver mitochondria can also be released by nonspecific mPTP. In the presence of Ca2+, the addition of Fe2+ and H2O2 induces the opening of mPTP, and mtDNA is observed to be hydrolyzed after oxidative stress, with partial release of mDNA fragments into the cytoplasm (Garcia et al., 2005). Different studies have shown differences in the amount of mtDNA fragments released. Garcia et al. found that the DNA content in the mitochondrial matrix decreased to 42% ± 6% and the number of MTCO1, MTND3, and MTCYB genes in the mitochondria decreased by approximately 46%, 22%, and 54%, respectively, and mtDNA release could be inhibited in the presence of cyclosporin A, an mPTP inhibitor (Garcia and Chavez, 2007). In summary, mtDNA can be released by mPTP, and incomplete mtDNA fragments are released, which is consistent with the fact that mPTP only allows the transport of molecules<1.5 kDa (Halestrap et al., 2002). Recent studies have revealed the mechanism by which this process occurs. When mitochondrial stress occurs, ROS induces the production of oxidized mtDNA (Ox-mtDNA), which can be repaired by the DNA glycosylase OGG1, and when the repair mechanism is limited, Ox-mtDNA is cleaved within the mitochondria by the nucleic acid endonuclease FEN1 into 500–650 bp fragments, which leave the mPTP- and voltage-dependent anion channel-(VDAC) dependent channels through the mitochondria, thus initiating the activation of inflammation (Xian et al., 2022; Cabral et al., 2023). During this time, VDAC plays a role in helping mtDNA open the door to the cytoplasm. VDAC, also known as mitochondrial porin, is the major protein involved in transporting OMM (Shoshan-Barmatz et al., 2006). One of the VDAC-1 isoforms can form oligomers on the OMM (Keinan et al., 2010). Kim et al. found in the mice model of systemic lupus erythematosus (SLE) that when mitochondria are stressed in multiple ways, the fragmented mtDNA binds to the VDAC on the OMM, which causes multiple VDAC monomers to cluster together and form a mesopore in their middle, allowing mtDNA to be released into the cytoplasm through this mesopore by direct interaction between the three positively charged residues at the N-terminal end of the VDAC and mtDNA (Kim et al., 2019). Researchers applied the oligomerization inhibitor VBIT-4 to block only one of the channel forms, VDAC1, to reduce mtDNA release in the mice model of SLE, demonstrating the potential role of VDAC in mtDNA release (Kim et al., 2019). In conclusion, when oxidative stress damages mitochondria, it triggers the opening of mPTP on IMM while oxidizing mtDNA. Ox-mtDNA undergoes shearing by nucleic acid endonucleases and then enters cytoplasmic lysis through mPTP and VDAC on OMM in sequence, which in turn triggers subsequent inflammatory responses. It is worth mentioning that the structural opening of the pores may also lead to mitochondrial swelling, resulting in IMM disruption and thus cytosolic release of mtDNA (Riley and Tait, 2020). Whether this effect of mPTP on IMM is related to MIMP needs to be further explored. Li et al. also showed that the inherent tripartite motif 21 of tumor cells promotes VDAC2 degradation through K48-linked ubiquitination, which inhibits the pore-forming capacity of VDAC2 oligomers and reduces mtDNA leakage, which in turn inhibits the type I interferon response following exposure to IR (Li et al., 2023). Notably, the structural opening of the pore can also lead to mitochondrial swelling and IMM disruption, which promotes the release of mtDNA into the cytosol (Riley and Tait, 2020). Whether this effect of mPTP on IMM is related to MIMP deserves further investigation. It has been shown that chitosan, a vaccine adjuvant, activates the cGAS-STING pathway and induces the type I IFN response via mPTP-dependent release of mtDNA (Carroll et al., 2016). These results suggest that mPTP may play a more important role in activating the cGAS-STING pathway, and although the structural integrity of mtDNA is difficult to maintain, this fragmented DNA does not affect the recognition of cGAS.
Gasdermins (GSDMs) play a key role in pyroptosis. Its N-terminal structural domain can form transmembrane pores in the plasma membrane, leading to changes in membrane permeability (Chen et al., 2016b; Ding et al., 2016; Liu et al., 2016). Besides the plasma membrane, there is evidence that the GSDM family can form pores in the mitochondrial membrane. Weindel et al. also found that in macrophages, elevated mitochondrial ROS (mtROS) direct the binding of GSDMD to the mitochondrial membrane, followed by the formation of mitochondrial GSDMD pores and then the release of mtROS (Weindel et al., 2022). Huang et al. showed that lipopolysaccharide (LPS) activates GSDMD and forms a mitochondrial pore that induces the release of mtDNA into the cytoplasm of endothelial cells to be recognized by cGAS (Huang et al., 2020). These findings suggest that GSDM may be involved in the release of mtDNA as a novel pathway.
In addition, changes in mitochondrial dynamics contribute to the release of mtDNA from the mitochondria. Mitochondrial transcription factor A (TFAM) plays an important role in mitochondrial quality control, and imbalances in TFAM and/or mtDNA homeostasis are frequently observed in tumor cells (Wallace, 2012). West et al. showed that TFAM-deficient fibroblasts display elongated and highly fused mitochondria and increased cytosolic mtDNA (West et al., 2015). Similarly, inhibition of ataxia-mutated protein (ATM) leads to downregulation of TFAM, allowing mtDNA to escape to the cytoplasm (Hu et al., 2021b). Mitochondrial division is important to ensure proper nucleoid distribution and removal of damaged mtDNA (Meyer et al., 2017). It has been shown that activation of STING signaling in Kupffer cells after LPS stimulation may be associated with enhanced mitochondrial division and increased mtDNA release caused by upregulation of dynamin-related protein 1 (DRP1) (Zhang et al., 2023c). Furthermore, LPS enhanced DRP1-dependent mtROS production, which further enhanced STING signaling by promoting mtDNA leakage into the cytosol (Li et al., 2022; Zhang et al., 2023c). Similarly, a recent study showed that the knockdown of GTPase—MxB, a member of the membrane-deforming dynamin family located in the IMM, resulted in mitochondrial fragmentation, IMM breakage, and increased cytoplasmic mtDNA levels (Cao et al., 2020). This suggests that homeostatic imbalance is likely another factor in the release of mtDNA into the cytoplasm.
6 Presence of intercellular transfer of mtDNA, cGAS, and STING
In recent years, horizontal transfer of mitochondria and mtDNA between tumor cells and surrounding non-tumor cells has been reported. Tumor cells acquire functional mitochondria and mtDNA from healthy host cells to repair or even enhance impaired mitochondrial function, thereby restoring tumorigenic potential. Tumor cells show a significant delay in tumor growth in the absence of mtDNA (Rebbeck et al., 2011). Salaud et al. found that mitochondria can be transferred from tumor-activated stromal cells present in the glioblastoma (GBM) microenvironment to GBM, and this transfer increases the proliferation of GBM and its resistance to radiotherapy and chemotherapy (Salaud et al., 2020). This transfer of intact mitochondria from host cells may be an important way for tumor cells to acquire mtDNA (Tan et al., 2015; Dong et al., 2017) (Figure 1). Interestingly, Kleih et al. found that cisplatin-sensitive high-grade plasmacytoid ovarian cancer cell lines contained higher mitochondrial content than cisplatin-resistant cells (Kleih et al., 2019). This suggests that mtDNA or mitochondria may play different roles depending on the specific context and stage of tumor progression.
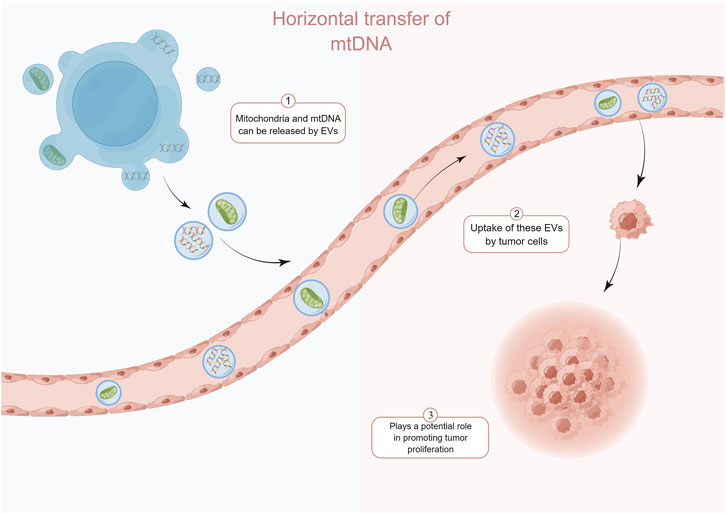
FIGURE 1. Normal cell-derived mtDNA and mitochondria can be transferred to tumor cells via the extracellular vesicle pathway and enhance the recovery of their tumorigenic potential. By Figdraw.
The transfer of mtDNA through “tunneling nanotubes” (TnTs) may be an important way of cross-talk between stromal cells and cancer cells (Pasquier et al., 2013). In addition to the TnTs pathway of cellular level translocation of mtDNA, the extracellular vesicle (EV) pathway is another important way of mtDNA level transfer. Circulating EVs from breast cancer patients identify complete mitochondrial genomes (Sansone et al., 2017). Rabas et al. also found that in breast cancer cells, PINK1 drives the production of mtDNA-loaded EVs and transfers invasive properties to “recipient” tumor cells (Rabas et al., 2021). Recent studies have identified mechanisms that regulate the translocation of mitochondrial components, including mtDNA, into EVs. Mitochondria-derived vesicles can transport components of the mitochondrial interior to nearby organelles. This process is dependent on the proteins optic atrophy 1 and categorical connexin 9 (Todkar et al., 2021). Notably, the presence of mitochondrial proteins in EVs has been demonstrated in the absence of pro-inflammatory stimuli (Hurwitz et al., 2016; Kowal et al., 2016). So, is it possible that mitochondria regulate tumor cells survival by actively releasing mtDNA-containing EVs, or is the acquisition of mtDNA by tumor cells a “voluntary act” that occurs when the tumor cells force the normal cells through certain pathways? Besides mitochondria and mtDNA, activated cGAS and STING can be transferred to recipient cells via EVs. Clancy et al. found that a portion of the dsDNA coupled to activated cGAS was encapsulated in tumor microvesicles (TMVs) and that the TMVs transferred both to the recipient cells and influenced the behavior of the recipient cells (Clancy et al., 2022). In addition, RAB22A mediates the formation of atypical autophagosomes containing STING activated by agonists or radiotherapy, while RAB22A inactivates RAB7 and inhibits the fusion of formed autophagosomes with lysosomes, resulting in the release of endo-vesicular vesicles of autophagosomes with activated STING into the extracellular space (Gao et al., 2022). It is foreseeable that this direct cell-to-cell transfer of activators may have a faster and more direct effect than the transfer of DNA, leading to different consequences.
7 STING exerts anti-tumor effects through the innate immune pathway
Given the role of the cGAS-STING pathway in activating immune surveillance, current studies have focused on innate immune function to exert anti-tumor effects (Li et al., 2019; Kwon and Bakhoum, 2020). Due to the inherent genomic instability of cancer cells, they can display constitutive activation of intrinsic immunity and cGAS-STING pathway-mediated IFN signaling, with the production of IFN-a and IFN-b that bind to IFN receptors on themselves, neighboring cells or immune cells, mobilizing immune cells such as dendritic cells (DCs) and TME infiltration of CD8+T cells to eliminate the tumor (Diamond et al., 2011; Fuertes et al., 2011; Reislander et al., 2020). Grabosch et al. activated the cGAS-STING pathway in ovarian cancer mice using the DNA damage inducer cisplatin and increased CD8+T cells infiltration (Grabosch et al., 2019). Chemosuppression of ATM activated the cGAS-STING signaling pathway by downregulating TFAM to promote mtDNA release, which similarly enhanced T lymphocytes infiltration into TME (Hu et al., 2021b). Harabuchi and others used a combination of cisplatin and cGAMP to increase chemokine expression in tumor tissue, transform tumors from “cold” to “hot” via STING, increase CD8+T cells infiltration, and significantly inhibit tumor growth in tumor-bearing mice (Harabuchi et al., 2020). This transformation to “hot” tumors may be associated with the expression of chemokines, such as CXCL9, CXCL10, CCL5, CCL20, etc., which recruit antigen-presenting cells (APCs), T cells, and NK cells to infiltrate the TME and kill tumor cells (Corbera-Bellalta et al., 2016; Hu et al., 2023).
8 STING exerts anti-tumor effects through non-innate immune pathways
8.1 STING regulated autophagy
Induction of autophagy is one of the important functions of STING and may play a role in the interferon signaling process before STING (Gui et al., 2019; Nassour et al., 2019). Nassour et al. showed that STING-driven macroautophagy, a subtype of autophagy involving autophagic lysosomes, is a critical step in preventing cancer development (Nassour et al., 2019). Liu et al. reported STING-induced atypical autophagy dependent on ATG5 (Liu et al., 2019a). Typical autophagy can also be triggered by the interaction of cGAS and Beclin-1, fluid-phase separation of cGAS-DNA complexes, and STING-triggered ER stress-mTOR signaling (Zhang et al., 2023c). Autophagy-dependent cell death can be triggered when DNA damage-induced STING activation clears cancer cell (Zhang et al., 2021).
8.2 STING-regulated cell death pathways
The molecular mechanisms behind STING-associated cell death involve multiple signaling cascades. Ferroptosis is a form of cell death characterized by iron-mediated membrane lipid peroxidation and dysregulation of anti-oxidant levels (Tang et al., 2021). The nucleoside analog zalcitabine induces mitochondrial damage and mtDNA release, activates the cGAS-STING pathway, which in turn induces autophagy-dependent ferroptosis and inhibits pancreatic tumor growth in mice (Li et al., 2021b). STING also promotes ferroptosis in human pancreatic cancer cell lines by increasing mitofusin 1/2-dependent mitochondrial fusion, which induces massive ROS production and ultimately membrane lipid peroxidation (Li et al., 2021a). Zierhut et al. showed that cGAS activation is inhibited by nucleosomes during normal mitosis, but during prolonged mitotic arrest, cGAS promotes a slow accumulation of IRF3 phosphorylation, which in turn may contribute to apoptosis by inhibiting Bcl-xL. This ability to exert inflammatory signaling in transcriptionally competent interphase cells while inducing apoptosis in transcriptionally attenuated mitotic cells contributes to increased sensitivity to paclitaxel, a chemotherapeutic agent that acts by inhibiting cell mitosis, in patients with lung and ovarian cancer (Zierhut et al., 2019). Since certain types of DNA damage can lead to mitotic blockage and mitotic cell death (Garner et al., 2013), cGAS may also affect other types of anti-tumor responses that use DNA damage as a mechanism of action. For example, Banerjee et al. found that STING affects cell death through the DNA damage response (DDR) system independently of the typical IFN pathway. The STING-TBK1 axis stimulates phosphorylation of the kinase ATM, which activates the CHK2-p53-p21 pathway and induces apoptosis by blocking the cellular G1 phase (Banerjee et al., 2021). In addition, a genome-wide CRISPR-Cas9 screen by Hayman et al. revealed that STING acts as a regulator of ROS homeostasis that deletion of STING leads to enhanced cellular ROS metabolism and therapeutic resistance to DNA damaging agents in head and neck squamous cell carcinoma, and that pharmacological activation of STING enhances the anti-tumor effects of IR in vivo. A rationale for the therapeutic combination of STING agonists and DNA-damaging agents is provided (Hayman et al., 2021).
8.3 STING in metabolism
In the presence of Brucella infection, STING regulates the metabolic reprogramming of macrophages via HIF-1α involving oxidative phosphorylation and glycolysis (Gomes et al., 2021). It is then worth exploring whether the restoration of impaired respiratory function by tumor cells through horizontal transfer of mtDNA to enhance tumor-promoting behavior may also be related to STING, as mentioned above. The current study demonstrated the regulatory role of STING in glucose metabolism. Rong et al. found that in an environment of adequate nutrition and unactivated innate immunity, STING molecules could inhibit the autophagosome-lysosome fusion process involved in STX17 and downregulate autophagic flux by binding to STX17, an important protein for the fusion of autophagic membranes, and allowing it to reside in the ER. In the absence of STING, or upon activation by cGAMP, and ER-GIC translocation, STX17 is released from STING, leading to an upregulation of autophagy levels, which enhances AMPK activity in skeletal muscle cells (Rong et al., 2022). This reveals a novel non-immune function of STING, i.e., spatial modulation of the classical autophagy pathway induced by energy deprivation, suggesting a new link between innate immunity and energy metabolism. Tumor cells promote proliferation through aerobic glycolysis, or the Warburg effect, which is another hallmark of cancer. Tumor aerobic glycolysis produces an unfavorable TME, resulting in impaired anti-tumor immunity. Local nutrient depletion inhibits anti-tumor T-cells proliferation and activation, while accumulation of waste products (e.g., lactate) enhances the immunosuppressive TME (Wang et al., 2020b). Zhang et al. found that STING can target hexokinase II to block its activity, thereby promoting anti-tumor immunity by limiting aerobic glycolysis in tumor cells. In human colorectal cancer samples, lactate, which can be used as a proxy for aerobic glycolysis, was negatively correlated with STING expression levels and anti-tumor immunity (Zhang et al., 2023a). Collectively, these findings reveal a role for STING in the regulation of cellular metabolism and establish a critical link between the glycolytic regulation of STING and tumor suppressor activity.
8.4 STING in senescence
STING, through activation of the downstream NF-κB pathway, also mediates the secretion of pro-inflammatory cytokines, chemokines, proteases, and growth factors, collectively referred to as SASPs (senescence-associated secretory phenotypes), which attenuate tumor growth by promoting cell cycle arrest in tumor cells (Dou et al., 2017; Yang et al., 2017). Yang et al. found that cGAS deletion accelerated spontaneous immortalization of mouse embryonic fibroblasts, and cGAS deletion also eliminated SASPs induced by multiple factors, including radiation and etoposide (Yang et al., 2017). These cytokines signaling programs can also induce enhanced inflammatory responses. For example, both IL-1β and IFN-α disrupt mitochondrial homeostasis to induce enhanced immune responses, demonstrating a novel function of IL-1β in initiating or enhancing STING-mediated intrinsic cellular immunity (Aarreberg et al., 2019). In this perspective, STING-mediated IFNs production and inflammatory factors generated by activation of the NF-κB pathway may further amplify the damaging effects of mitochondria, leading to increased mtDNA release and enhanced cGAS recognition, resulting in a cascade of amplified responses to inflammatory signals. Interestingly, it has recently been shown that NF-κB activation enhances STING signaling by modulating microtubule-mediated STING transport and that this synergistic interaction between NF-κB and STING triggers a cascade-amplified interferon response and robust host antiviral defense (Zhang et al., 2023b). In addition, Zhu et al. found that VDAC2 is a novel STING-binding ligand. STING deficiency or inhibition of the STING palmitoyltransferase ZDHHCs by 2-BP enhanced VDAC2/GRP75-mediated mitochondria-ER contact, increased mtROS/calcium levels, impaired mitochondrial function, and inhibited signaling in the mTOR pathway, which ultimately led to growth retardation in renal cell carcinoma (Zhu et al., 2023) (Figure 2). Table 1 summarizes the non-innate immunoregulatory pathways mediated by STING.
9 The intensity of STING activation in tumor cells influences their outcome
Recent evidence suggests that cGAS-STING signaling may also be involved in the promotion of tumor proliferation and metastasis. Cheng et al. showed that in tumor cells, ROS induced by mitochondrial Lon, a chaperone and DNA-binding protein involved in protein quality control and stress response pathways, triggers mtDNA damage, and Ox-mtDNA is released into the cytoplasm to activate IFN signaling, which further upregulates the expression of programmed death ligand-1 (PD-L1) and indoleamine 2,3-dioxygenase (IDO-1) to inhibit T-cells activation. In addition, Lon upregulation induced the secretion of loaded mtDNA and PD-L1 EVs, which further induced the production of IFNs and IL-6 by macrophages, thereby attenuating innate and CD8+T cells immunity in the TME (Cheng et al., 2020). This process may be the mechanism for the formation of immunosuppressive TME in tumors, and mtDNA levels may serve as a potential diagnostic biomarker for anti-PD-L1 immunotherapy (Cheng et al., 2020). Zeng et al. also found that IL-6-induced ROS production in endometrial cancer (EC) cells and high levels of ROS cause cytoplasmic leakage of mtDNA and activate downstream cGAS-STING signaling, which increased PD-L1 levels. In IL-6-induced EC cell-derived EVs, mtDNA and PD-L1 expression levels were similarly significantly elevated (Zeng et al., 2022). Similarly, IR of radiotherapy activates innate immune pathways, leading to the upregulation of PD-L1 in hepatocellular carcinoma (HCC), inhibition of cytotoxic T lymphocytes activity and immune infiltration, and protection of tumor cells from cellular immune-mediated killing (Du et al., 2022).
The emergence of this paradoxical phenomenon of STING may be related to its activation state, and its chronic activation may induce the formation of immunosuppressive TME (Coussens and Werb, 2002; Kwon and Bakhoum, 2020) (Figure 3). It has been shown that STING-deficient mice often develop more and larger tumors in advanced stages of HCC and show reduced levels of phosphorylated STAT1, autophagy, and cleaved caspase-3. Treatment with CDNs, a STING agonist, activates these responses in the liver, and CDNs treatment of advanced HCC in mice effectively reduces the size of most tumors (Thomsen et al., 2020). Application of engineered EVs exogenously loaded with CDNs preferentially activated APCs in the TME, enhanced local Th1 response and CD8+T cells infiltration in tumors as well as systemic anti-tumor immunity (Jang et al., 2021). Wang-Bishop et al. researchers mediated effective cytoplasmic delivery of the endogenous STING ligand cGAMP via intratumoral nanoparticle delivery, which enhanced STING activation relative to free cGAMP and improved response to PD-L1 immune checkpoint blockade, inducing immunogenic death in neuroblastoma (Wang-Bishop et al., 2020). Furthermore, the combination treatment of cGAMP with cisplatin exerted a stronger anti-tumor effect (Harabuchi et al., 2020).
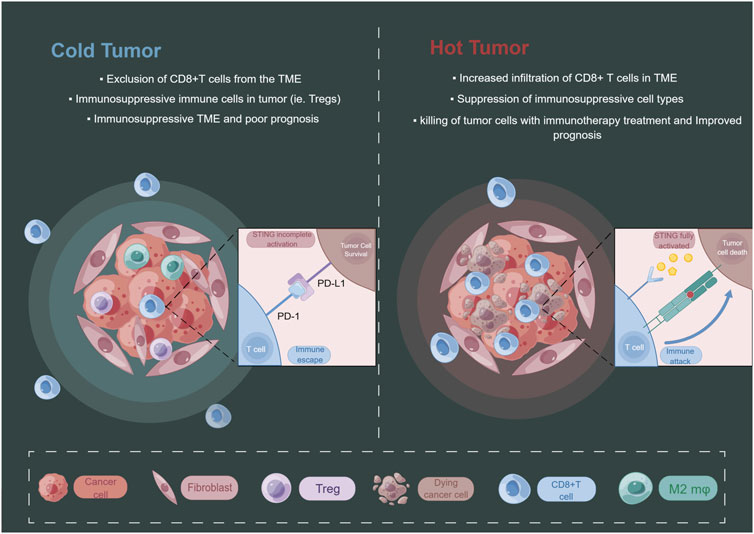
FIGURE 3. The level of STING activation in tumor cells may have an impact on the state of the tumor immune microenvironment. By Figdraw.
Furthermore, the levels of intracellularly detectable DNA fragments are even more important for the degree of activation of the cGAS-STING pathway. Liu et al. recently found that STING signaling in CD11c+ dendritic cells is required for the anti-tumor effects of CD47 blockade therapy and that tumor cells clearance by CD47 blockade is dependent on the recognition of tumor cells cytoplasmic DNA. Impaired recognition of endogenous DNA, including mtDNA, would result in ineffective tumor cells clearance (Liu et al., 2015). Gusho et al. found significantly elevated cGAS levels in human papillomavirus (a dsDNA virus) positive cells that stably maintained viral-free bodies (Gusho and Laimins, 2022). Given the complex regulatory roles of STING and its downstream pathways in immunotherapy targeting tumor cells, the combination of STING agonists with immune checkpoint blockade therapies may be a better approach. This combination application could reduce the use of one drug and provide an economical, beneficial, and safe option for patients. For example, the combination of STING agonists and IDO inhibitors significantly inhibited tumor progression in a mouse colorectal cancer model compared to immune checkpoint therapy alone (Shi et al., 2021). Yi et al. combined the STING agonists and the anti-TGF-β/PD-L1 bispecific antibody successfully enhanced innate and acquired immunity in mice, promoted antigen presentation, improved T-cells migration and chemotaxis, and upregulated the number and activity of tumor-infiltrating lymphocytes, demonstrating new perspectives for overcoming immunotherapy resistance (Yi et al., 2022). Since mtDNA acts as a potent agonist of cGAS, facilitating the release of mtDNA into the cytoplasm may be another promising direction. However, this places greater demands on the understanding of the temporal and spatial mechanisms involved in the tumor-promoting or inhibitory effects of cGAS-STING, as well as on the ability to correctly localize this pathway. Since whether horizontally transferred mtDNA is “friend” or “foe” is determined by the role played by STING at the stage of tumor progression, if the release of mtDNA is not high enough to reach the threshold for full activation of STING, it is more likely that the tumor will choose the path of no return, driving malignant programs and inhibiting their anti-tumor functions, producing counterproductive effects. Therefore, it may be possible to choose to combine with STING agonists and immune checkpoint inhibitors for better therapeutic effects when perhaps necessary.
10 Conclusion
Under conditions of oxidative stress or impaired mitochondrial quality control, mtDNA escapes into intra- or extracellular compartments of the cytoplasm. Once shed, mtDNA acts as damage-associated molecular patterns (DAMPs) that bind danger-signaling receptors to trigger an innate immune response (Picca et al., 2020). The consequences of mtDNA also depend on the cellular context and cover a wide range of states, including triggering an innate immune response, inducing cell death, or acting as a tumorigenic agent. mtDNA follows MOMP into the cytoplasm. In the past, MOMP appeared to play a bipolar role in cells, i.e., as long as apoptotic signals are generated, they can be propagated to all mitochondria in cells, leading to apoptotic cell death. However, under sublethal stress conditions, a fraction of mitochondria undergoe MOMP without inducing cell death, the phenomenon known as minority MOMP (miMOMP) (Ichim et al., 2015). The escape of mtDNA from mitochondria under miMOMP conditions is worth exploring because tumor cells are constantly exposed to chronic stress conditions such as hypoxia, and thus mtDNA leakage induced by miMOMP under such sublethal stress conditions may be involved in the maintenance of cytoplasmic dsDNA in tumor cells as well as its delivery to the outside of the cells. This chronic stimulation of the cGAS-STING pathway of appropriate intensity in tumor cells may mediate tumor cells survival and metastasis. In addition, the transfer of mtDNA from tumor cells to surrounding immune cells via EVs or TnTs may trigger an apoptotic cascade in host immune cells, thus creating an “immunoparalyzing” microenvironment for tumor cells, which may partly explain the depletion of CD8+T cells in the immunosuppressive TME. There are still many unanswered questions about the pathway of mtDNA escape, including the lack of conclusive evidence for the increase in MIMP when mtDNA is extruded from MOMP. Further studies are needed on the influence of the GSDM family and mitochondrial dynamics aspects on mtDNA release.
The fact that cGAS-STING has complex consequences during tumor progression suggests that our understanding of the two sides of cGAS-STING in cancer progression is important for advancing cancer therapeutic strategies. As mentioned above, the use of STING agonists to induce tumor immunogenicity for anti-tumor therapy has achieved some efficacy. However, there is still a lack of detailed studies on individual differences in different tumors, stages, genotypes, etc., as well as large sample data. These will determine the therapeutic response of tumor cells to STING agonists or antagonists. Admittedly, these studies have focused on the role of STING in innate immunity, and the exploration of the non-immune direction is currently very limited. Since STING modulation of processes such as tumor glycolysis, autophagy, and cell cycle has also shown anti-tumor effects, attempting to bypass the innate immunity aspect could prevent its potential for immune escape. For example, the atypical pathway autophagy process induced by STING participation is relatively independent and can be generated in the absence of TBK1 and IRF3 activation, and blocking IKK activation does not impair STING-induced autophagy (Gui et al., 2019). The cGAS-STING pathway can exert anti-tumor effects independent of IFNs and autophagy, which mainly depends on the recruitment ability of STING to TBK1. This suggests that the recruitment function of TBK1 to STING may be broader than the activation of IRF3 to promote IFNs synthesis (Yum et al., 2021). Overall, targeting STING will be a promising therapeutic prospect, whether focusing on the traditional innate immune aspects of STING or focusing on the emerging non-innate immune aspects of STING.
Author contributions
JY: Writing–original draft. MY: Writing–original draft. YW: Visualization, Writing–review and editing. JS: Investigation, Writing–review and editing. YL: Writing–review and editing. LZ: Conceptualization, Writing–review and editing. BG: Supervision, Writing–review and editing.
Funding
The author(s) declare financial support was received for the research, authorship, and/or publication of this article. This study research was funded by The National Natural Science Foundation of China (Grant No. 82273479 to LZ), The Research Fund of Jilin Provincial Science and Technology Department (Grant No. 20210204072YY to BG), and China-Japan Union Hospital and College of Basic Medical Sciences, Jilin University Union Project (Grant No. KYXZ2022JC05 to LZ).
Acknowledgments
Thanks to Jian Wang in the lab for his help with this paper and to the institution Home for Researchers (www.home-for-researchers.com).
Conflict of interest
The authors declare that the research was conducted in the absence of any commercial or financial relationships that could be construed as a potential conflict of interest.
Publisher’s note
All claims expressed in this article are solely those of the authors and do not necessarily represent those of their affiliated organizations, or those of the publisher, the editors and the reviewers. Any product that may be evaluated in this article, or claim that may be made by its manufacturer, is not guaranteed or endorsed by the publisher.
References
Aarreberg, L. D., Esser-Nobis, K., Driscoll, C., Shuvarikov, A., Roby, J. A., and Gale, M. (2019). Interleukin-1β induces mtDNA release to activate innate immune signaling via cGAS-STING. Mol. Cell. 74, 801–815. doi:10.1016/j.molcel.2019.02.038
Ablasser, A., and Chen, Z. J. J. (2019). cGAS in action: expanding roles in immunity and inflammation. Science 363, eaat8657. doi:10.1126/science.aat8657
Ablasser, A., Goldeck, M., Cavlar, T., Deimling, T., Witte, G., Rohl, I., et al. (2013). cGAS produces a 2 '-5 '-linked cyclic dinucleotide second messenger that activates STING. Nature 498, 380–384. doi:10.1038/nature12306
An, X., Zhu, Y. Y., Zheng, T. S., Wang, G. Y., Zhang, M. H., Li, J. D., et al. (2019). An analysis of the expression and association with immune cell infiltration of the cGAS/STING pathway in pan-cancer. Mol. Therapy-Nucleic Acids 14, 80–89. doi:10.1016/j.omtn.2018.11.003
Andreeva, L., Hiller, B., Kostrewa, D., Lassig, C., Mann, C. C. D., Drexler, D. J., et al. (2017). cGAS senses long and HMGB/TFAM-bound U-turn DNA by forming protein-DNA ladders. Nature 549, 394–398. doi:10.1038/nature23890
Bakhoum, S., Ngo, B., Bakhoum, A., Cavallo-Fleming, J. A., Murphy, C. W., Powell, S. N., et al. (2018). Chromosomal instability drives metastasis through a cytosolic DNA response. Int. J. Radiat. Oncol. Biol. Phys. 102, S118. doi:10.1016/j.ijrobp.2018.06.295
Banerjee, D., Langberg, K., Abbas, S., Odermatt, E., Yerramothu, P., Volaric, M., et al. (2021). A non-canonical, interferon-independent signaling activity of cGAMP triggers DNA damage response signaling. Nat. Commun. 12, 6207. doi:10.1038/s41467-021-26240-9
Bernardi, P., and Di Lisa, F. (2015). The mitochondrial permeability transition pore: molecular nature and role as a target in cardioprotection. J. Mol. Cell. Cardiol. 78, 100–106. doi:10.1016/j.yjmcc.2014.09.023
Bernardi, P., Rasola, A., Forte, M., and Lippe, G. (2015). The mitochondrial permeability transition pore: channel formation by F-atp synthase, integration in signal transduction, and role in pathophysiology. Physiol. Rev. 95, 1111–1155. doi:10.1152/physrev.00001.2015
Bernardi, P. (2018). Why F-ATP synthase remains a strong candidate as the mitochondrial permeability transition pore. Front. Physiology 9, 1543. doi:10.3389/fphys.2018.01543
Cabral, A., Cabral, J. E., Wang, A. E. L. A., Zhang, Y. Y., Liang, H. L., Nikbakht, D., et al. (2023). Author correction: differential binding of NLRP3 to non-oxidized and ox-mtDNA mediates NLRP3 inflammasome activation. Commun. Biol. 6, 632. doi:10.1038/s42003-023-05019-2
Cao, H., Krueger, E. W., Chen, J., Drizyte-Miller, K., Schulz, M. E., and Mcniven, M. A. (2020). The anti-viral dynamin family member MxB participates in mitochondrial integrity. Nat. Commun. 11, 1048. doi:10.1038/s41467-020-14727-w
Carroll, E. C., Jin, L., Mori, A., Munoz-Wolf, N., Oleszycka, E., Moran, H. B. T., et al. (2016). The vaccine adjuvant chitosan promotes cellular immunity via DNA sensor cGAS-STING-dependent induction of type I interferons. Immunity 44, 597–608. doi:10.1016/j.immuni.2016.02.004
Chen, Q., Sun, L. J., and Chen, Z. J. J. (2016a). Regulation and function of the cGAS-STING pathway of cytosolic DNA sensing. Nat. Immunol. 17, 1142–1149. doi:10.1038/ni.3558
Chen, X., He, W. T., Hu, L. C., Li, J. X., Fang, Y., Wang, X., et al. (2016b). Pyroptosis is driven by non-selective gasdermin-D pore and its morphology is different from MLKL channel-mediated necroptosis. Cell. Res. 26, 1007–1020. doi:10.1038/cr.2016.100
Cheng, A. N., Cheng, L. C., Kuo, C. L., Lo, Y. K., Chou, H. Y., Chen, C. H., et al. (2020). Mitochondrial Lon-induced mtDNA leakage contributes to PD-L1-mediated immunoescape via STING-IFN signaling and extracellular vesicles. J. Immunother. Cancer 8, e001372. doi:10.1136/jitc-2020-001372
Chin, E. N., Yu, C. G., Vartabedian, V. F., Jia, Y., Kumar, M., Gamo, A. M., et al. (2020). Antitumor activity of a systemic STING-activating non-nucleotide cGAMP mimetic. Science 369, 993–999. doi:10.1126/science.abb4255
Chipuk, J. E., Bouchier-Hayes, L., and Green, D. R. (2006). Mitochondrial outer membrane permeabilization during apoptosis: the innocent bystander scenario. Cell. Death Differ. 13, 1396–1402. doi:10.1038/sj.cdd.4401963
Clancy, J. W., Sheehan, C. S., Boomgarden, A. C., and D'Souza-Schorey, C. (2022). Recruitment of DNA to tumor-derived microvesicles. Cell. Rep. 38, 110443. doi:10.1016/j.celrep.2022.110443
Corbera-Bellalta, M., Planas-Rigol, E., Lozano, E., Terrades-Garcia, N., Alba, M. A., Prieto-Gonzalez, S., et al. (2016). Blocking interferon gamma reduces expression of chemokines CXCL9, CXCL10 and CXCL11 and decreases macrophage infiltration in ex vivo cultured arteries from patients with giant cell arteritis. Ann. Rheumatic Dis. 75, 1177–1186. doi:10.1136/annrheumdis-2015-208371
Coussens, L. M., and Werb, Z. (2002). Inflammation and cancer. Nature 420, 860–867. doi:10.1038/nature01322
Dai, J., Huang, Y. J., He, X. H., Zhao, M., Wang, X. Z., Liu, Z. S., et al. (2019). Acetylation blocks cGAS activity and inhibits self-DNA-induced autoimmunity. Cell. 176, 1447–1460. doi:10.1016/j.cell.2019.01.016
De Gaetano, A., Solodka, K., Zanini, G., Selleri, V., Mattioli, A. V., Nasi, M., et al. (2021). Molecular mechanisms of mtDNA-mediated inflammation. Cells 10, 2898. doi:10.3390/cells10112898
Diamond, M. S., Kinder, M., Matsushita, H., Mashayekhi, M., Dunn, G. P., Archambault, J. M., et al. (2011). Type I interferon is selectively required by dendritic cells for immune rejection of tumors. J. Exp. Med. 208, 1989–2003. doi:10.1084/jem.20101158
Diner, E. J., Burdette, D. L., Wilson, S. C., Monroe, K. M., Kellenberger, C. A., Hyodo, M., et al. (2013). The innate immune DNA sensor cGAS produces a noncanonical cyclic dinucleotide that activates human STING. Cell. Rep. 3, 1355–1361. doi:10.1016/j.celrep.2013.05.009
Ding, J. J., Wang, K., Liu, W., She, Y., Sun, Q., Shi, J. J., et al. (2016). Pore-forming activity and structural autoinhibition of the gasdermin family. Nature 535, 111–116. doi:10.1038/nature18590
Dobbs, N., Burnaevskiy, N., Chen, D. D., Gonugunta, V. K., Alto, N. M., and Yan, N. (2015). STING activation by translocation from the ER is associated with infection and autoinflammatory disease. Cell. Host Microbe 18, 157–168. doi:10.1016/j.chom.2015.07.001
Dong, L.-F., Kovarova, J., Bajzikova, M., Bezawork-Geleta, A., Svec, D., Endaya, B., et al. (2017). Horizontal transfer of whole mitochondria restores tumorigenic potential in mitochondrial DNA-deficient cancer cells. ELife 6, e22187. doi:10.7554/eLife.22187
Dou, Z. X., Ghosh, K., Vizioli, M. G., Zhu, J. J., Sen, P., Wangensteen, K. J., et al. (2017). Cytoplasmic chromatin triggers inflammation in senescence and cancer. Nature 550, 402–406. doi:10.1038/nature24050
Du, S. S., Chen, G. W., Yang, P., Chen, Y. X., Hu, Y., Zhao, Q. Q., et al. (2022). Radiation therapy promotes hepatocellular carcinoma immune cloaking via PD-L1 upregulation induced by cGAS-STING activation. Int. J. Radiat. Oncol. Biol. Phys. 112, 1243–1255. doi:10.1016/j.ijrobp.2021.12.162
Ergun, S. L., Fernandez, D., Weiss, T. M., and Li, L. Y. (2019). STING polymer structure reveals mechanisms for activation, hyperactivation, and inhibition. Cell. 178, 290–301. doi:10.1016/j.cell.2019.05.036
Falahat, R., Berglund, A., Putney, R. M., Perez-Villarroel, P., Aoyama, S., Pilon-Thomas, S., et al. (2021). Epigenetic reprogramming of tumor cell-intrinsic STING function sculpts antigenicity and T cell recognition of melanoma. Proc. Natl. Acad. Sci. U. S. A. 118, e2013598118. doi:10.1073/pnas.2013598118
Flores-Romero, H., Hohorst, L., John, M., Albert, M. C., King, L. E., Beckmann, L., et al. (2022). BCL-2-family protein tBID can act as a BAX-like effector of apoptosis. Embo J. 41, e108690. doi:10.15252/embj.2021108690
Fuertes, M. B., Kacha, A. K., Kline, J., Woo, S. R., Kranz, D. M., Murphy, K. M., et al. (2011). Host type I IFN signals are required for antitumor CD8+ T cell responses through CD8{alpha}+ dendritic cells. J. Exp. Med. 208, 2005–2016. doi:10.1084/jem.20101159
Gao, P., Ascano, M., Wu, Y., Barchet, W., Gaffney, B. L., Zillinger, T., et al. (2013a). Cyclic [G(2 ',5 ') pA(3 ',5 ')p] is the metazoan second messenger produced by DNA-activated cyclic GMP-AMP synthase. Cell. 153, 1094–1107. doi:10.1016/j.cell.2013.04.046
Gao, P., Ascano, M., Zillinger, T., Wang, W. Y., Dai, P. H., Serganov, A. A., et al. (2013b). Structure-function analysis of STING activation by c[G(2 ',5 ') pA(3 ',5 ')p] and targeting by antiviral DMXAA. Cell. 154, 748–762. doi:10.1016/j.cell.2013.07.023
Gao, Y., Zheng, X. P., Chang, B. Y., Lin, Y. J., Huang, X. D., Wang, W., et al. (2022). Intercellular transfer of activated STING triggered by RAB22A-mediated non-canonical autophagy promotes antitumor immunity. Cell. Res. 32, 1086–1104. doi:10.1038/s41422-022-00731-w
Garcia, N., and Chavez, E. (2007). Mitochondrial DNA fragments released through the permeability transition pore correspond to specific gene size. Life Sci. 81, 1160–1166. doi:10.1016/j.lfs.2007.08.019
Garcia, N., Garcia, J. J., Correa, F., and Chavez, E. (2005). The permeability transition pore as a pathway for the release of mitochondrial DNA. Life Sci. 76, 2873–2880. doi:10.1016/j.lfs.2004.12.012
Garner, E., Kim, Y., Lach, F. P., Kottemann, M. C., and Smogorzewska, A. (2013). Human GEN1 and the SLX4-associated nucleases MUS81 and SLX1 are essential for the resolution of replication-induced Holliday junctions. Cell. Rep. 5, 207–215. doi:10.1016/j.celrep.2013.08.041
Gomes, M. T. R., GuimarãES, E. S., Marinho, F. V., Macedo, I., Aguiar, E. R. G. R., Barber, G. N., et al. (2021). STING regulates metabolic reprogramming in macrophages via HIF-1α during Brucella infection. PLoS Pathog. 17, e1009597. doi:10.1371/journal.ppat.1009597
Grabosch, S., Bulatovic, M., Zeng, F. T. Z., Ma, T. Z., Zhang, L. X., Ross, M., et al. (2019). Cisplatin-induced immune modulation in ovarian cancer mouse models with distinct inflammation profiles. Oncogene 38, 2380–2393. doi:10.1038/s41388-018-0581-9
Gui, X., Yang, H., Li, T., Tan, X. J., Shi, P. Q., Li, M. H., et al. (2019). Autophagy induction via STING trafficking is a primordial function of the cGAS pathway. Nature 567, 262–266. doi:10.1038/s41586-019-1006-9
Guo, X. H., Ni, J., Liang, Z. Q., Xue, J. L., Fenech, M. F., and Wang, X. (2019). The molecular origins and pathophysiological consequences of micronuclei: new insights into an age-old problem. Mutat. Research-Reviews Mutat. Res. 779, 1–35. doi:10.1016/j.mrrev.2018.11.001
Gupta, V., Agrawal, R. C., and Trivedi, P. (2011). Reduction in cisplatin genotoxicity (micronucleus formation) in non target cells of mice by protransfersome gel formulation used for management of cutaneous squamous cell carcinoma. Acta Pharm. 61, 63–71. doi:10.2478/v10007-011-0004-8
Gusho, E., and Laimins, L. A. (2022). Human papillomaviruses sensitize cells to DNA damage induced apoptosis by targeting the innate immune sensor cGAS. Plos Pathog. 18, e1010725. doi:10.1371/journal.ppat.1010725
Halestrap, A. P., Mcstay, G. P., and Clarke, S. J. (2002). The permeability transition pore complex: another view. Biochimie 84, 153–166. doi:10.1016/s0300-9084(02)01375-5
Harabuchi, S., Kosaka, A., Yajima, Y., Nagata, M., Hayashi, R., Kumai, T., et al. (2020). Intratumoral STING activations overcome negative impact of cisplatin on antitumor immunity by inflaming tumor microenvironment in squamous cell carcinoma. Biochem. Biophysical Res. Commun. 522, 408–414. doi:10.1016/j.bbrc.2019.11.107
Harding, S. M., Benci, J. L., Irianto, J., Discher, D. E., Minn, A. J., and Reenberg, R. A. G. (2017). Mitotic progression following DNA damage enables pattern recognition within micronuclei. Nature 548, 466–470. doi:10.1038/nature23470
Hatch, E. M., Fischer, A. H., Deerinck, T. J., and Hetzer, M. W. (2013). Catastrophic nuclear envelope collapse in cancer cell micronuclei. Cell. 154, 47–60. doi:10.1016/j.cell.2013.06.007
Hayman, T. J., Baro, M., Macneil, T., Phoomak, C., Aung, T. N., Cui, W., et al. (2021). STING enhances cell death through regulation of reactive oxygen species and DNA damage. Nat. Commun. 12, 2327. doi:10.1038/s41467-021-22572-8
Hopfner, K. P., and Hornung, V. (2020). Molecular mechanisms and cellular functions of cGAS-STING signalling. Nat. Rev. Mol. Cell. Biol. 21, 501–521. doi:10.1038/s41580-020-0244-x
Hu, M. M., Yang, Q., Xie, X. Q., Liao, C. Y., Lin, H., Liu, T. T., et al. (2016). Sumoylation promotes the stability of the DNA sensor cGAS and the adaptor STING to regulate the kinetics of response to DNA virus. Immunity 45, 555–569. doi:10.1016/j.immuni.2016.08.014
Hu, M., Zhou, M., Bao, X., Pan, D., Jiao, M., Liu, X., et al. (2021a). ATM inhibition enhances cancer immunotherapy by promoting mtDNA leakage and cGAS/STING activation. J. Clin. Invest. 131, e139333. doi:10.1172/JCI139333
Hu, M. J., Zhou, M., Bao, X. H., Pan, D., Jiao, M., Liu, X. J., et al. (2021b). ATM inhibition enhances cancer immunotherapy by promoting mtDNA leakage and cGAS/STING activation. J. Clin. Investigation 131, e139333. doi:10.1172/JCI139333
Hu, J., Sanchez-Rivera, F. J., Wang, Z. H., Johnson, G. N., Ho, Y. J., Ganesh, K., et al. (2023). STING inhibits the reactivation of dormant metastasis in lung adenocarcinoma. Nature 616, 806–813. doi:10.1038/s41586-023-05880-5
Huang, Y. H., Liu, X. Y., Du, X. X., Jiang, Z. F., and Su, X. D. (2012). The structural basis for the sensing and binding of cyclic di-GMP by STING. Nat. Struct. Mol. Biol. 19, 728–730. doi:10.1038/nsmb.2333
Huang, L. S., Hong, Z. G., Wu, W., Xiong, S. Q., Zhong, M., Gao, X. P., et al. (2020). mtDNA activates cGAS signaling and suppresses the YAP-mediated endothelial cell proliferation program to promote inflammatory injury. Immunity 52, 475–486. doi:10.1016/j.immuni.2020.02.002
Hurwitz, S. N., Rider, M. A., Bundy, J. L., Liu, X., Singh, R. K., and Meckes, D. G. (2016). Proteomic profiling of NCI-60 extracellular vesicles uncovers common protein cargo and cancer type-specific biomarkers. Oncotarget 7, 86999–87015. doi:10.18632/oncotarget.13569
Ichim, G., Lopez, J., Ahmed, S. U., Muthalagu, N., Giampazolias, E., Delgado, M. E., et al. (2015). Limited mitochondrial permeabilization causes DNA damage and genomic instability in the absence of cell death. Mol. Cell. 58, 900. doi:10.1016/j.molcel.2015.05.028
Jang, S. C., Economides, K. D., Moniz, R. J., Sia, C. L., Lewis, N., Mccoy, C., et al. (2021). ExoSTING, an extracellular vesicle loaded with STING agonists, promotes tumor immune surveillance. Commun. Biol. 4, 497. doi:10.1038/s42003-021-02004-5
Jiang, H., Xue, X. Y., Panda, S., Kawale, A., Hooy, R. M., Liang, F. S., et al. (2019). Chromatin-bound cGAS is an inhibitor of DNA repair and hence accelerates genome destabilization and cell death. Embo J. 38, e102718. doi:10.15252/embj.2019102718
Kausar, S., Yang, L. Q., Abbas, M. N., Hu, X., Zhao, Y. J., Zhu, Y., et al. (2020). Mitochondrial DNA: a key regulator of anti-microbial innate immunity. Genes 11, 86. doi:10.3390/genes11010086
Keinan, N., Tyomkin, D., and Shoshan-Barmatz, V. (2010). Oligomerization of the mitochondrial protein voltage-dependent anion channel is coupled to the induction of apoptosis. Mol. Cell. Biol. 30, 5698–5709. doi:10.1128/MCB.00165-10
Kim, J., Gupta, R., Blanco, L. P., Yang, S. T., Shteinfer-Kuzmine, A., Wang, K. N., et al. (2019). VDAC oligomers form mitochondrial pores to release mtDNA fragments and promote lupus-like disease. Science 366, 1531–1536. doi:10.1126/science.aav4011
Kleih, M., Bopple, K., Dong, M., Gaissler, A., Heine, S., Olayioye, M. A., et al. (2019). Direct impact of cisplatin on mitochondria induces ROS production that dictates cell fate of ovarian cancer cells. Cell. Death Dis. 10, 851. doi:10.1038/s41419-019-2081-4
Konno, H., Yamauchi, S., Berglund, A., Putney, R. M., Mule, J. J., and Barber, G. N. (2018). Suppression of STING signaling through epigenetic silencing and missense mutation impedes DNA damage mediated cytokine production. Oncogene 37, 2037–2051. doi:10.1038/s41388-017-0120-0
Kowal, J., Arras, G., Colombo, M., Jouve, M., Morath, J. P., Primdal-Bengtson, B., et al. (2016). Proteomic comparison defines novel markers to characterize heterogeneous populations of extracellular vesicle subtypes. Proc. Natl. Acad. Sci. U. S. A. 113, E968–E977. doi:10.1073/pnas.1521230113
Kuchta, K., Knizewski, L., Wyrwicz, L. S., Rychlewski, L., and Ginalski, K. (2009). Comprehensive classification of nucleotidyltransferase fold proteins: identification of novel families and their representatives in human. Nucleic Acids Res. 37, 7701–7714. doi:10.1093/nar/gkp854
Kwon, J., and Bakhoum, S. F. (2020). The cytosolic DNA-sensing cGAS-STING pathway in cancer. Cancer Discov. 10, 26–39. doi:10.1158/2159-8290.CD-19-0761
Li, A. P., Yi, M., Qin, S., Song, Y. P., Chu, Q., and Wu, K. M. (2019). Activating cGAS-STING pathway for the optimal effect of cancer immunotherapy. J. Hematol. Oncol. 12, 35. doi:10.1186/s13045-019-0721-x
Li, C. F., Liu, J., Hou, W., Kang, R., and Tang, D. L. (2021a). STING1 promotes ferroptosis through MFN1/2-dependent mitochondrial fusion. Front. Cell. Dev. Biol. 9, 698679. doi:10.3389/fcell.2021.698679
Li, C. F., Zhang, Y., Liu, J., Kang, R., Klionsky, D. J., and Tang, D. L. (2021b). Mitochondrial DNA stress triggers autophagy-dependent ferroptotic death. Autophagy 17, 948–960. doi:10.1080/15548627.2020.1739447
Li, T., Huang, T. Z., and Chen, Z. J. J. (2021c). Phosphorylation and chromatin tethering prevent cGAS activation during mitosis. J. Immunol. 206, 15.17. doi:10.4049/jimmunol.206.supp.15.17
Li, Y. J., Chen, H., Yang, Q., Wan, L. X., Zhao, J., Wu, Y. Y., et al. (2022). Increased Drp1 promotes autophagy and ESCC progression by mtDNA stress mediated cGAS-STING pathway. J. Exp. Clin. Cancer Res. 41, 76. doi:10.1186/s13046-022-02262-z
Li, J.-Y., Zhao, Y., Gong, S., Wang, M.-M., Liu, X., He, Q.-M., et al. (2023). TRIM21 inhibits irradiation-induced mitochondrial DNA release and impairs antitumour immunity in nasopharyngeal carcinoma tumour models. Nat. Commun. 14, 865. doi:10.1038/s41467-023-36523-y
Liu, X. J., Pu, Y., Cron, K., Deng, L. F., Kline, J., Frazier, W. A., et al. (2015). CD47 blockade triggers T cell-mediated destruction of immunogenic tumors. Nat. Med. 21, 1209–1215. doi:10.1038/nm.3931
Liu, X., Zhang, Z. B., Ruan, J. B., Pan, Y. D., Magupalli, V. G., Wu, H., et al. (2016). Inflammasome-activated gasdermin D causes pyroptosis by forming membrane pores. Nature 535, 153–158. doi:10.1038/nature18629
Liu, D., Wu, H., Wang, C. G., Li, Y. J., Tian, H. B., Siraj, S., et al. (2019a). STING directly activates autophagy to tune the innate immune response. Cell. Death Differ. 26, 1735–1749. doi:10.1038/s41418-018-0251-z
Liu, R. Q., Xu, F., Bi, S. W., Zhao, X. S., Jia, B. S., and Cen, Y. (2019b). Mitochondrial DNA-induced inflammatory responses and lung injury in thermal injury murine model: protective effect of cyclosporine-A. J. Burn Care & Res. 40, 355–360. doi:10.1093/jbcr/irz029
Mcarthur, K., Whitehead, L. W., Heddleston, J. M., Li, L., Padman, B. S., Oorschot, V., et al. (2018). BAK/BAX macropores facilitate mitochondrial herniation and mtDNA efflux during apoptosis. Science 359, eaao6047. doi:10.1126/science.aao6047
Meyer, J. N., Leuthner, T. C., and Luz, A. L. (2017). Mitochondrial fusion, fission, and mitochondrial toxicity. Toxicology 391, 42–53. doi:10.1016/j.tox.2017.07.019
Mukai, K., Konno, H., Akiba, T., Uemura, T., Waguri, S., Kobayashi, T., et al. (2016). Activation of STING requires palmitoylation at the Golgi. Nat. Commun. 7, 11932. doi:10.1038/ncomms11932
Nassour, J., Radford, R., Correia, A., Fuste, J. M., Schoell, B., Jauch, A., et al. (2019). Autophagic cell death restricts chromosomal instability during replicative crisis. Nature 565, 659–663. doi:10.1038/s41586-019-0885-0
Pasquier, J., Guerrouahen, B. S., al Thawadi, H., Abu Kaoud, N., Maleki, M., Le Foll, F., et al. (2013). Preferential transfer of mitochondria from endothelial to cancer cells through tunneling nanotubes modulates chemoresistance. Clin. Cancer Res. 19, 94. doi:10.1186/1479-5876-11-94
Patrushev, M., Kasymov, V., Patrusheva, V., Ushakova, T., Gogvadze, V., and Gaziev, A. I. (2006). Release of mitochondrial DNA fragments from brain mitochondria of irradiated mice. Mitochondrion 6, 43–47. doi:10.1016/j.mito.2005.12.001
Piantadosi, C. A. (2020). Mitochondrial DNA, oxidants, and innate immunity. Free Radic. Biol. Med. 152, 455–461. doi:10.1016/j.freeradbiomed.2020.01.013
Picca, A., Calvani, R., Coelho, H. J., Landi, F., Bernabei, R., and Marzetti, E. (2020). Mitochondrial dysfunction, oxidative stress, and neuroinflammation: intertwined roads to neurodegeneration. Antioxidants 9, 647. doi:10.3390/antiox9080647
Rabas, N., Palmer, S., Mitchell, L., Ismail, S., Gohlke, A., Riley, J. S., et al. (2021). PINK1 drives production of mtDNA-containing extracellular vesicles to promote invasiveness. J. Cell. Biol. 220, e202006049. doi:10.1083/jcb.202006049
Rebbeck, C. A., Leroi, A. M., and Burt, A. (2011). Mitochondrial capture by a transmissible cancer. Science 331, 303. doi:10.1126/science.1197696
Reislander, T., Groelly, F. J., and Tarsounas, M. (2020). DNA damage and cancer immunotherapy: a STING in the tale. Mol. Cell. 80, 21–28. doi:10.1016/j.molcel.2020.07.026
Riley, J. S., and Tait, S. W. G. (2020). Mitochondrial DNA in inflammation and immunity. Embo Rep. 21, e49799. doi:10.15252/embr.201949799
Riley, J. S., Quarato, G., Cloix, C., Lopez, J., O'Prey, J., Pearson, M., et al. (2018). Mitochondrial inner membrane permeabilisation enables mtDNA release during apoptosis. Embo J. 37, e99238. doi:10.15252/embj.201899238
Rong, Y., Zhang, S., Nandi, N., Wu, Z., Li, L., Liu, Y., et al. (2022). STING controls energy stress-induced autophagy and energy metabolism via STX17. J. Cell. Biol. 221, e202202060. doi:10.1083/jcb.202202060
Salaud, C., Alvarez-Arenas, A., Geraldo, F., Belmonte-Beitia, J., Calvo, G. F., Gratas, C., et al. (2020). Mitochondria transfer from tumor-activated stromal cells (TASC) to primary Glioblastoma cells. Biochem. Biophysical Res. Commun. 533, 139–147. doi:10.1016/j.bbrc.2020.08.101
Sansone, P., Savini, C., Kurelac, I., Chang, Q., Amato, L. B., Strillacci, A., et al. (2017). Packaging and transfer of mitochondrial DNA via exosomes regulate escape from dormancy in hormonal therapy-resistant breast cancer (vol 114, pg E9066, 2017). Proc. Natl. Acad. Sci. U. S. A. 114, E10255. doi:10.1073/pnas.1704862114
Shang, G. J., Zhu, D. Y., Li, N., Zhang, J. B., Zhu, C. Y., Lu, D. F., et al. (2012). Crystal structures of STING protein reveal basis for recognition of cyclic di-GMP. Nat. Struct. Mol. Biol. 19, 725–727. doi:10.1038/nsmb.2332
Shang, G. J., Zhang, C. G., Chen, Z. J. J., Bai, X. C., and Zhang, X. W. (2019). Cryo-EM structures of STING reveal its mechanism of activation by cyclic GMP-AMP. Nature 567, 389–393. doi:10.1038/s41586-019-0998-5
Shi, J. Q., Liu, C. Q., Luo, S. N., Cao, T. Y., Lin, B. L., Zhou, M., et al. (2021). STING agonist and Ido inhibitor combination therapy inhibits tumor progression in murine models of colorectal cancer. Cell. Immunol. 366, 104384. doi:10.1016/j.cellimm.2021.104384
Shoshan-Barmatz, V., Israelson, A., Brdiczka, D., and Sheu, S. S. (2006). The voltage-dependent anion channel (VDAC): function in intracellular signalling, cell life and cell death. Curr. Pharm. Des. 12, 2249–2270. doi:10.2174/138161206777585111
Shu, C., Yi, G. H., Watts, T., Kao, C. C., and Li, P. W. (2012). Structure of STING bound to cyclic di-GMP reveals the mechanism of cyclic dinucleotide recognition by the immune system. Nat. Struct. Mol. Biol. 19, 722–724. doi:10.1038/nsmb.2331
Smale, S. T. (2010). Selective transcription in response to an inflammatory stimulus. Cell. 140, 833–844. doi:10.1016/j.cell.2010.01.037
Sun, L. J., Wu, J. X., Du, F. H., Chen, X., and Chen, Z. J. J. (2013). Cyclic GMP-AMP synthase is a cytosolic DNA sensor that activates the type I interferon pathway. Science 339, 786–791. doi:10.1126/science.1232458
Tan, A. S., Baty, J. W., Dong, L. F., Bezawork-Geleta, A., Endaya, B., Goodwin, J., et al. (2015). Mitochondrial genome acquisition restores respiratory function and tumorigenic potential of cancer cells without mitochondrial DNA. Cell. Metab. 21, 81–94. doi:10.1016/j.cmet.2014.12.003
Tanaka, Y., and Chen, Z. J. J. (2012). STING specifies IRF3 phosphorylation by TBK1 in the cytosolic DNA signaling pathway. Sci. Signal. 5, ra20. doi:10.1126/scisignal.2002521
Tang, D. L., Chen, X., Kang, R., and Kroemer, G. (2021). Ferroptosis: molecular mechanisms and health implications. Cell. Res. 31, 107–125. doi:10.1038/s41422-020-00441-1
Thomsen, M. K., Skouboe, M. K., Boularan, C., Vernejoul, F., Lioux, T., Leknes, S. L., et al. (2020). The cGAS-STING pathway is a therapeutic target in a preclinical model of hepatocellular carcinoma. Oncogene 39, 1652–1664. doi:10.1038/s41388-019-1108-8
Tigano, M., Vargas, D. C., Tremblay-Belzile, S., Fu, Y., and Sfeir, A. (2021). Nuclear sensing of breaks in mitochondrial DNA enhances immune surveillance. Nature 591, 477–481. doi:10.1038/s41586-021-03269-w
Todkar, K., Chikhi, L., Desjardins, V., El-Mortada, F., Pepin, G., and Germain, M. (2021). Selective packaging of mitochondrial proteins into extracellular vesicles prevents the release of mitochondrial DAMPs. Nat. Commun. 12, 1971. doi:10.1038/s41467-021-21984-w
Wang, Y. T., Ning, X. H., Gao, P. F., Wu, S. X., Sha, M. Y., Lv, M. Z., et al. (2017). Inflammasome activation triggers caspase-1-mediated cleavage of cGAS to regulate responses to DNA virus infection. Immunity 46, 393–404. doi:10.1016/j.immuni.2017.02.011
Wang, H. W., Zang, C. L., Ren, M. T., Shang, M. D., Wang, Z. H., Peng, X. M., et al. (2020a). Cellular uptake of extracellular nucleosomes induces innate immune responses by binding and activating cGMP-AMP synthase (cGAS). Sci. Rep. 10, 15385. doi:10.1038/s41598-020-72393-w
Wang, J. X., Choi, S. Y. C., Niu, X. J., Kang, N., Xue, H., Killam, J., et al. (2020b). Lactic acid and an acidic tumor microenvironment suppress anticancer immunity. Int. J. Mol. Sci. 21, 8363. doi:10.3390/ijms21218363
Wang-Bishop, L., Wehbe, M., Shae, D., James, J., Hacker, B. C., Garland, K., et al. (2020). Potent STING activation stimulates immunogenic cell death to enhance antitumor immunity in neuroblastoma. J. Immunother. Cancer 8, e000282. doi:10.1136/jitc-2019-000282
Weindel, C. G., Martinez, E. L., Zhao, X., Mabry, C. J., Bell, S. L., Vail, K. J., et al. (2022). Mitochondrial ROS promotes susceptibility to infection via gasdermin D-mediated necroptosis. Cell. 185, 3214–3231.e23. doi:10.1016/j.cell.2022.06.038
West, A. P., and Shadel, G. S. (2017). Mitochondrial DNA in innate immune responses and inflammatory pathology. Nat. Rev. Immunol. 17, 363–375. doi:10.1038/nri.2017.21
West, A. P., Khoury-Hanold, W., Staron, M., Tal, M. C., Pineda, C. M., Lang, S. M., et al. (2015). Mitochondrial DNA stress primes the antiviral innate immune response. Nature 520, 553–557. doi:10.1038/nature14156
Wu, J. X., Sun, L. J., Chen, X., Du, F. H., Shi, H. P., Chen, C., et al. (2013). Cyclic GMP-AMP is an endogenous second messenger in innate immune signaling by cytosolic DNA. Science 339, 826–830. doi:10.1126/science.1229963
Wu, Z., Sainz, A. G., and Shadel, G. S. (2021a). Mitochondrial DNA: cellular genotoxic stress sentinel. Trends Biochem. Sci. 46, 812–821. doi:10.1016/j.tibs.2021.05.004
Wu, Z., Sainz, A. G., and Shadel, G. S. (2021b). Mitochondrial DNA: cellular genotoxic stress sentinel. Trends Biochem. Sci. 46, 812–821. doi:10.1016/j.tibs.2021.05.004
Xia, P. Y., Ye, B. Q., Wang, S., Zhu, X. X., Du, Y., Xiong, Z., et al. (2016a). Erratum: glutamylation of the DNA sensor cGAS regulates its binding and synthase activity in antiviral immunity. Nat. Immunol. 17, 469. doi:10.1038/ni0416-469e
Xia, T. L., Konno, H., Ahn, J., and Barber, G. N. (2016b). Deregulation of STING signaling in colorectal carcinoma constrains DNA damage responses and correlates with tumorigenesis. Cell. Rep. 14, 282–297. doi:10.1016/j.celrep.2015.12.029
Xia, T. L., Konno, H., and Barber, G. N. (2016c). Recurrent loss of STING signaling in melanoma correlates with susceptibility to viral oncolysis. Cancer Res. 76, 6747–6759. doi:10.1158/0008-5472.CAN-16-1404
Xian, H. X., Watari, K., Sanchez-Lopez, E., Offenberger, J., Onyuru, J., Sampath, H., et al. (2022). Oxidized DNA fragments exit mitochondria via mPTP- and VDAC-dependent channels to activate NLRP3 inflammasome and interferon signaling. Immunity 55, 1370–1385.e8. doi:10.1016/j.immuni.2022.06.007
Yang, H., Wang, H., Ren, J., Chen, Q., and Chen, Z. J. (2017). cGAS is essential for cellular senescence. Proc. Natl. Acad. Sci. U. S. A. 114, E4612–e4620. doi:10.1073/pnas.1705499114
Yi, M., Niu, M., Wu, Y., Ge, H., Jiao, D., Zhu, S., et al. (2022). Combination of oral STING agonist MSA-2 and anti-TGF-β/PD-L1 bispecific antibody YM101: a novel immune cocktail therapy for non-inflamed tumors. J. Hematol. Oncol. 15, 142. doi:10.1186/s13045-022-01363-8
Yum, S., Li, M., Fang, Y., and Chen, Z. J. (2021). TBK1 recruitment to STING activates both IRF3 and NF-κB that mediate immune defense against tumors and viral infections. Proc. Natl. Acad. Sci. U. S. A. 118, e2100225118. doi:10.1073/pnas.2100225118
Zeng, X., Li, X. S., Zhang, Y. D., Cao, C. X., and Zhou, Q. (2022). IL6 induces mtDNA leakage to affect the immune escape of endometrial carcinoma via cGAS-STING. J. Immunol. Res. 2022, 3815853. doi:10.1155/2022/3815853
Zhang, J., Hu, M. M., Wang, Y. Y., and Shu, H. B. (2012). TRIM32 protein modulates type I interferon induction and cellular antiviral response by targeting MITA/STING protein for K63-linked ubiquitination. J. Biol. Chem. 287, 28646–28655. doi:10.1074/jbc.M112.362608
Zhang, X., Shi, H. P., Wu, J. X., Zhang, X. W., Sun, L. J., Chen, C., et al. (2013). Cyclic GMP-AMP containing mixed phosphodiester linkages is an endogenous high-affinity ligand for STING. Mol. Cell. 51, 226–235. doi:10.1016/j.molcel.2013.05.022
Zhang, R. X., Kang, R., and Tang, D. L. (2021). The STING1 network regulates autophagy and cell death. Signal Transduct. Target. Ther. 6, 208. doi:10.1038/s41392-021-00613-4
Zhang, L., Jiang, C., Zhong, Y., Sun, K., Jing, H., Song, J., et al. (2023a). STING is a cell-intrinsic metabolic checkpoint restricting aerobic glycolysis by targeting HK2. Nat. Cell. Biol. 25, 1208–1222. doi:10.1038/s41556-023-01185-x
Zhang, L., Wei, X., Wang, Z., Liu, P., Hou, Y., Xu, Y., et al. (2023b). NF-κB activation enhances STING signaling by altering microtubule-mediated STING trafficking. Cell. Rep. 42, 112185. doi:10.1016/j.celrep.2023.112185
Zhang, Q., Wei, J. Y., Liu, Z. H., Huang, X. X., Sun, M. M., Lai, W. J., et al. (2023c). Corrigendum to "STING signaling sensing of DRP1-dependent mtDNA release in kupffer cells contributes to lipopolysaccharide-induced liver injury in mice" [Redox Biol. 54 (2022) 102367/PMID:35724543]. Redox Biol. 62, 102664. doi:10.1016/j.redox.2023.102664
Zhu, X. Q., Luo, L., Xiong, Y. Y., Jiang, N., Wang, Y. R., Lv, Y., et al. (2022). VDAC1 oligomerization may enhance DDP-induced hepatocyte apoptosis by exacerbating oxidative stress and mitochondrial DNA damage. Febs Open Bio 12, 516–522. doi:10.1002/2211-5463.13359
Zhu, Z. C., Zhou, X., Du, H. W., Cloer, E. W., Zhang, J. M., Mei, L., et al. (2023). STING suppresses mitochondrial VDAC2 to govern RCC growth independent of innate immunity. Adv. Sci. 10, e2203718. doi:10.1002/advs.202203718
Zierhut, C., and Funabiki, H. (2015). Nucleosome functions in spindle assembly and nuclear envelope formation. Bioessays 37, 1074–1085. doi:10.1002/bies.201500045
Zierhut, C., Jenness, C., Kimura, H., and Funabiki, H. (2014). Nucleosomal regulation of chromatin composition and nuclear assembly revealed by histone depletion. Nat. Struct. Mol. Biol. 21, 617–625. doi:10.1038/nsmb.2845
Keywords: mtDNA, cGAS-STING, PD-L1, TME, extracellular vesicles
Citation: Yang J, Yang M, Wang Y, Sun J, Liu Y, Zhang L and Guo B (2023) STING in tumors: a focus on non-innate immune pathways. Front. Cell Dev. Biol. 11:1278461. doi: 10.3389/fcell.2023.1278461
Received: 16 August 2023; Accepted: 13 October 2023;
Published: 25 October 2023.
Edited by:
Xiangsheng Zuo, University of Texas MD Anderson Cancer Center, United StatesReviewed by:
Khyati Maulik Kariya, Massachusetts General Hospital and Harvard Medical School, United StatesMilton Roy, Johns Hopkins Medicine, United States
Copyright © 2023 Yang, Yang, Wang, Sun, Liu, Zhang and Guo. This is an open-access article distributed under the terms of the Creative Commons Attribution License (CC BY). The use, distribution or reproduction in other forums is permitted, provided the original author(s) and the copyright owner(s) are credited and that the original publication in this journal is cited, in accordance with accepted academic practice. No use, distribution or reproduction is permitted which does not comply with these terms.
*Correspondence: Baofeng Guo, gbf@jlu.edu.cn; Ling Zhang, zhangling3@jlu.edu.cn