Understanding the unique mechanism of ferroptosis: a promising therapeutic target
- 1Department of Hematology and Oncology, Children’s Hospital of Nanjing Medical University, Nanjing, China
- 2Department of Clinical Laboratory, Maternal and Child Health Care of Zaozhuang, Zaozhuang, China
Ferroptosis is an iron-dependent form of regulated cell death and is characterized by high concentrations of intracellular lipid peroxide and a redox imbalance in the cells. Ferroptosis shows distinct morphological and biological features compared with other prominent mechanisms of programmed cell death. The distinct characteristics of ferroptosis include the dysfunction of the lipid peroxide repair enzyme glutathione peroxidase 4, the presence of ferrous iron overload, and the lipid peroxidation of polyunsaturated fatty acids. Several other metabolic pathways (including iron, lipid, and amino acid metabolism) and ferritinophagy, as well as transcription factors, can modulate ferroptosis. However, to date, the molecular mechanism of ferroptosis has not been elucidated. This review outlines the discovery, characterization, regulatory mechanisms, and crosstalk of ferroptosis. Further, we have noted the controversial elements in the ferroptosis-related mechanisms. Our inferences may provide a partial reference for developing strategies to regulate ferroptosis.
What is already known on this topic—Ferroptosis include the dysfunction of the lipid peroxide repair enzyme glutathione peroxidase 4, the presence of ferrous iron overload, and the lipid peroxidation of polyunsaturated fatty acids.
What this study adds—Outlines the discovery, characterization, regulatory mechanisms, and crosstalk of ferroptosis.
How this study might affect research, practice or policy –May provide a partial reference for developing strategies to regulate ferroptosis.
Introduction
Cell death is an integral cellular process that occurs in living cells (Doll and Conrad, 2017). The process maintains homeostasis and prevents certain hyperproliferative diseases such as cancer (Fuchs and Steller, 2015). Under certain conditions, dead cells are continuously replaced by new ones causing aberrant cell growth in the diseased condition. Apart from accidental cell death that occurs suddenly after extremely harsh physicochemical or mechanical insults, most cell death types can be regulated by genetic or pharmacologic interferences (regulated cell death) (Galluzzi et al., 2015; Doll and Conrad, 2017). In the early 1970s, the three canonical types of cell death were defined by the structure and morphology of the dying cell; they include apoptosis (type I; physiological cell death), autophagy (type II), and necrosis (type III; pathological cell death). In addition, new non-canonical cell death modalities have been proposed, including paraptosis, pyroptosis, mitotic catastrophe, autophagy-dependent cell death, lysosome-dependent cell death, immunogenic cell death, and ferroptosis (Gilloteaux et al., 2010). Ferroptosis is a non-apoptotic mode of regulatory necrosis with distinct morphologic and biochemical features. The process is distinguished from other programmed and non-programmed cell death types by its iron dependency and intracellular lipid peroxide accumulation (Dixon et al., 2012a).
While ferroptosis was originally discovered in the context of screening for small molecules to selectively kill RAS-mutated tumor cells, its relevance has expanded to other pathologic settings as well. Ferroptosis plays a critical regulatory role in various human diseases, such as tumorigenesis, neurodegenerative diseases, ischemia-reperfusion injury, renal failure, organ fibrosis, cardiovascular diseases, and hematological diseases (Li J. et al., 2020). The initiation of ferroptosis is a desirable outcome in several carcinomas. The efficacy of chemotherapy improved in certain tumor types that were susceptible to modulations in this death pathway (Stockwell et al., 2017). However, ferroptosis is a negative outcome in several other diseases, such as neurodegeneration, type II diabetes, periventricular leukomalacia, and renal dysfunction; it contributes to enhancing injury or disease progression. Therefore, the pathophysiologic and physiologic relevance of ferroptosis in different disease contexts has created several avenues for therapeutic discovery. Herein, we reviewed the different processes involved in the regulation of ferroptosis. This information is crucial in developing novel therapeutic strategies that target ferroptosis for several human diseases, including cancer.
Overview of ferroptosis
Discovery
In 1955, human uterine carcinoma HeLa cells that were cultured without cystine revealed a unique microscopic morphology that could be restored by the addition of glutathione (GSH) (EAGLE, 1955). Later, researchers revealed that cystine could maintain the GSH levels and that there might be an accumulation of intracellular reactive oxygen species (ROS) (Bannai et al., 1977). In 1965, lipid peroxidation was considered to be the primary reason for cellular damage in the rat liver. In the 1980s, screening of the unsaturated lipid components of damaged cell membranes identified lipid peroxidation as one of the main forms of oxidative damage (Dolma et al., 2003). As such, these early studies related to the discovery of a novel mechanism of cellular damage.
Conceptualization
In 2003, Dolma et al. screened small chemical compounds to test their efficacy to kill cells overexpressing the oncogenic RAS protein (Dolma et al., 2003). This study identified a novel compound named “erastin” that selectively killed RAS-expressing tumorigenic cells in a non-apoptotic manner. In 2007, the authors further confirmed that lipophilic antioxidants (e.g., α-tocopherol) prevented erastin-induced oxidative non-apoptotic death in the HRAS- and KRAS-expressing cells (Dolma et al., 2003). In 2008, two small molecules, RAS-selective lethal molecule 3 and 5 (RSL3 and RSL5), were identified, which selectively killed human foreskin fibroblasts in a similar iron-dependent non-apoptotic manner, and this form of cell death could be inhibited by both desferrioxamine mesylate and α-tocopherol (Yang and Stockwell, 2008). Subsequent studies using other cell death inducers elaborated on the features of this mode of cell death. In 2012, Dixon et al. coined the term “ferroptosis” for this phenomenon of erastin-induced iron-dependent cell death characterized by lipid peroxidation (Dixon et al., 2012a) (Figure 1).
Characterization
The ferroptotic cells have distinct morphological and biochemical features and phenotypes that distinguish them from cells undergoing other forms of cell death (Table 1). Treatment with a ferroptosis inducer (e.g., erastin) results in small-sized mitochondria with reduced or vanished cristae, condensed mitochondrial densities, and ruptured outer mitochondrial membrane (Dixon et al., 2012a; Xie et al., 2016; Li J. et al., 2020). The biochemical features of ferroptotic cells include accumulation of iron, lipid peroxidation, inhibition of cystine/glutamate antiporter system (system Xc−) and glutathione peroxidase 4 (GPX4), and reduction in cystine uptake and GSH (Dixon et al., 2012a; Xie et al., 2016; Li J. et al., 2020). Six mitochondrial regulator genes specific for ferroptosis, namely, ATP5G3, CS, RPL8, IREB2, TTC35, and ACSF2, were detected using shRNA in HRAS-mutant Calu-1 cells and NRAS-mutant HT-1080 cells (Dixon et al., 2012a). Moreover, the specific ferroptosis inhibitors (such as ferrostatin-1 and deferoxamine) do not affect other types of programmed cell death. However, it is controversial whether the other cell death inhibitors can regulate ferroptosis. Therefore, further studies are required to explore the mechanism of ferroptosis.
Regulation mechanisms of ferroptosis
Iron metabolism
Ferroptosis is an iron-dependent form of regulated cell death. Iron is an important component in several intracellular processes, including DNA synthesis, mitochondrial respiration, cellular respiration, and cell signaling. Iron exists in two forms in the human body, ferrous (Fe2+) and ferric (Fe3+) ions; the majority of iron is used for metalloprotein synthesis or stored by ferritin to form inorganic (e.g., Fe/S cluster) or organic cofactors (e.g., heme) (Daher et al., 2017). The uptake, transport, storage, and metabolism of iron are precisely regulated because excessive cellular iron [redox-active iron (Fe2+)] can increase the production of ROS through the Fenton reaction, thereby promoting lipid peroxidation. Extracellular Fe3+ is absorbed from the diet by duodenal villous epithelial cells, binds to transferrin, and is transported by combining with the transferrin receptor (TFR) (Li et al., 2020b). Once internalized, the complex dissolves in the late endosome/lysosome owing to the acidic environment. Free Fe3+ is reduced to Fe2+ by a six-transmembrane epithelial antigen of prostate 3 metalloreductase (STEAP3) and transferred out of the endosome/lysosome into the cytosol by the apical divalent metal transporter 1 (DMT1) (Li J. et al., 2020). In the cytosol, the acquired iron can be transported to the site of its use. It may also be stored by ferritin as the Fe3+ irons or in the transient labile iron pool (LIP) to avoid cell toxicity. The surplus intracellular iron is exported out of the cell by ferroportin (FPN1/SLC40A1) (Kakhlon and Cabantchik, 2002).
High levels of free intracellular iron in the cells are a prerequisite for triggering ferroptosis. Excess cellular iron increases the levels of the Fe2+ irons; the resulting increase in the LIP subsequently promotes lipid peroxidation via the Fenton reaction and activity of ROS-generating enzymes which triggers ferroptosis (Hassannia et al., 2019). The iron metabolism genes probably regulate the susceptibility to ferroptosis and its occurrence and development. The most crucial route for cells to import iron is mediated by transferrin and its carrier protein TFR1, which is encoded by the TFR1 gene. Notably, silencing the TFR1 gene inhibited erastin-induced ferroptosis (Kuang et al., 2020). Furthermore, ferroptosis induction or iron-responsive element-binding protein 2 (IREB2) silencing modulates iron content, which is mediated by several genes such as the TFR, ferritin heavy chain, and ferritin light chain genes, rendering the cell more sensitive to ferroptosis (Dixon et al., 2012a). Artemisinin derivatives, which rely on free Fe2+ iron to mediate their activity, regulate the expression of iron metabolism genes such as TFR, IREB2, and other specific ferroptosis-related genes. In Hela cells, heat shock factor-binding protein 1 (HSPB1) protects the actin cytoskeleton to obstruct iron uptake by inhibiting the TRF1 gene expression (Sun et al., 2015). In a recent study, excessive expression of iron-sulfur cluster proteins, such as CDGSH iron sulfur domain 1 (CISD1), an iron-containing export mitochondrial membrane protein desensitized tumor cells to ferroptosis (Alvarez et al., 2017). However, the role of mitochondria in ferroptosis remains controversial and further research is needed to explore this phenomenon. Overall, alterations in the expression of iron metabolism genes or inhibition of HSPB1 and CISD1 can significantly lower the free intracellular iron content and trigger iron overload-induced ferroptosis.
Ferritinophagy is the autophagic depletion of ferritin by nuclear receptor coactivator 4 (NCOA4). It is a recently identified process that contributes to iron accumulation and free radical damage (Mancias et al., 2014). Ferritin degradation results in the release of iron, thereby increasing LIP and subsequent cellular toxicity. Ferritinophagy plays a critical role in maintaining iron homeostasis. The autophagic degradation of ferritin via NCOA4-mediated ferritinophagy was found to initiate ferroptosis by increasing the levels of free iron, promoting ROS accumulation, and driving the ferroptotic process in the cytoplasm (Lei et al., 2019). The genetic ablation of NCOA4 inhibits erastin-induced ferroptosis, whereas its overexpression significantly increases sensitivity to ferroptosis in HT-1080 fibrosarcoma and PANC1 pancreatic adenocarcinoma cell lines (Hou et al., 2016). Therefore, NCOA4-mediated ferritin degradation is closely related to ferroptosis. Overall, these studies suggest that free intracellular iron levels are tightly regulated and dysregulation of iron metabolism is a key trigger of ferroptosis (Figure 2).
Depletion of glutathione and ferroptosis
Ferroptosis is an iron-dependent form of cell death; it occurs through the accumulation of ROS when GSH-dependent lipid peroxide repair systems are compromised. System Xc− is a disulfide-linked heterodimer comprising SLC3A2 and SLC7A11; it imports one molecule of cystine (the oxidized dimer form of cysteine) in exchange for the equivalent molecule of intracellular glutamate antiporter (Tsurusaki et al., 2019). GSH is an antioxidant comprising glutamate, cysteine, and glycine. GPX4 utilizes GSH as a cofactor and is the only selenoenzyme that catabolizes the reduction of lipid hydroperoxide to alcohol (Lei et al., 2019). Consequently, the inhibition of system Xc− activity by erastin causes the intracellular depletion and inhibition of GSH levels by decreasing the influx of cysteine. This depletes GPX activity and results in the reduction in cell antioxidant capacity, lethal accumulation of ROS, and initiation of ferroptosis by erastin-induced oxidative stress (Yang et al., 2014).
GPX4 is a monomeric glutathione peroxidase responsible for peroxide detoxification in complex lipids and is the main protector of cells from ferroptotic cell death (Yang and Stockwell, 2008). Deficiency and inhibition of GPX4 induce cell death in a ferroptotic manner (Kim et al., 2020). A decrease in GPX4 activity is mediated by direct or indirect mechanisms (such as GSH depletion). In the modulatory profiling strategy, erastin and RSL3 (the first identified ferroptotic compounds) are xCT antiporter (consisting of two subunits SLC3A2 and SLC7A11) and GPX4 inhibitors, respectively. A mechanism underlying erastin-mediated inhibition of the system Xc− transporter has been shown to initiate ferroptosis (Dixon et al., 2012a) (Figure 2). Other than diminishing the abundance of GPX4 through GSH depletion, the direct inactivation or depletion of GPX4 is mediated by RSL3 and wathaferin A (Hassannia et al., 2018). The ferroptosis-inducing agent 56 (FIN56) directly inhibits GPX4 activity at the posttranslational level without GSH depletion (Shimada et al., 2016a). Moreover, GPX4 inactivation can be induced by several compounds, such as sorafenib, sulfasalazine, L-buthionine sulfoximine, glutamate, and artesunate (Li et al., 2020c). These compounds are involved in GSH depletion and ROS accumulation in the form of lipid hydroperoxides, ultimately due to oxidative imbalance and ferroptosis.
Recently, several studies on ferroptosis have suggested the mutational or chemical inhibition of the cystine-glutamate antiporter in diverse cancers. Glutamate, by preventing cystine import, could initiate ferroptosis at high extracellular concentrations (Dixon et al., 2012a). Cysteine, imported into cells through the SLC7A10 transporter, is also essential for ferroptotic cell death (Felley-Bosco and Gray, 2019). Some cells synthesize cysteine from methionine through methionine-R-sulfide reductase B2 by trans-sulfuration pathway. This process bypasses the requirement for cystine import via system Xc− (Stockwell et al., 2017; Felley-Bosco and Gray, 2019). Moreover, the conditional ablation of SLC7A11 initiates tumor-selective ferroptosis, which inhibited pancreatic ductal adenocarcinoma growth (Badgley et al., 2020). Consistent with the above findings, GPX4 inactivation using ferroptosis inhibitors such as ferrostatin-1 and liproxstatin-1 was lethal in xenograft models in many human diseases including acute renal failure, ischemia/reperfusion injury, neurodegenerative diseases, and cancers (Shi et al., 2021). Nicotinamide adenine dinucleotide phosphate (NADPH), which is involved in lipid peroxidation and synthesis of GSH, can promote sensitivity to ferroptosis-inducing agents and is essential for the malfunctioning of GSH metabolism (Shimada et al., 2016b). The nucleophile catalytic moiety of the selenocysteine on the active site of GPX4 plays a pivotal role in these pathologies and the modulation of ferroptosis. GSH is involved in the critical role of maintaining membrane integrity favored by inhibition and impairment of cysteine oxidation and the elimination of electrophiles involved in lipid peroxidation.
Lipid peroxidation
Lipid peroxidation is a free radical or non-radical-driven chain reaction that is initiated by the interaction of activated ROS with polyunsaturated fatty acids (PUFAs), which are susceptible to oxygenation to generate lipid peroxyl radicals and hydroperoxides (Friedmann et al., 2014). It occurs in three phases: initiation, propagation, and termination (reviewed in detail in (Yin et al., 2011)). PUFAs, the substrates for iron-catalyzed lipid peroxidation are present in phospholipids. The peroxidation is ultimately catalyzed by lysophosphatidylcholine acyltransferase 3 (LPCAT3) after the acetylation of acyl-CoA synthetase long-chain member 4 (ACSL4) (Dixon et al., 2015). LPCAT3 and ACSL4 are the biomarkers that correlate with the biosynthesis and remodeling of lipid peroxides while executing ferroptosis (Doll et al., 2017) (Figure 3). Lipoxygenases (LOXs) are the other key drivers of lipid peroxidation. LOXs induce the loss of membrane stability and integrity, catalyze the oxidation of PUFAs, and release noxious aldehydes (Hassannia et al., 2019), thereby modulating ferroptosis. The knockdown or inhibition of LOX activity inhibited the occurrence of ferroptosis in some contexts. ROS accumulation is caused by either an increase in their generation or a decrease in their elimination. However, the mechanism by which the ferroptotic death cascade occurs remains unclear. Recent studies on the transcriptional regulation underlying ferroptotic cell death have revealed that several transcription factors (including TP53, NFE2L2/NRF2, YAP1, TAZ, ATF3, ATF4, and HNF4A) can recognize specific DNA sequences to mediate the expression of multiple genes and play multiple roles in determining ferroptosis sensitivity (Dai et al., 2020). These transcription factors may exert their effects through either transcription-dependent or -independent mechanisms (Kang et al., 2019). The function of transcription factors generally depends on the targeted genes, and the same regulators sometimes may regulate both ferroptosis and other cell death forms (Tang et al., 2019). Overall, targeting the ferroptotic network appears to be a new therapeutic strategy for several diseases or pathological conditions.
Diseases associated with ferroptosis
Ferroptosis and cancer
Research suggests that the accumulation of iron ions within tumor cells is closely associated with tumor growth and metastasis in cancer (Figure 4). Excessive accumulation of iron ions may induce oxidative stress in certain types of tumors, leading to apoptosis or necrosis of cancer cells (Chen et al., 2015). Additionally, various tumor suppressor factors have been found to enhance cell sensitivity to ferroptosis, implying that ferroptosis might contribute to the anti-tumor activity of these factors. For instance, studies indicate that p53 promotes ferroptosis by inhibiting the transcription of SLC7A11 (Wang et al., 2016). Furthermore, a particular single nucleotide polymorphism P47S of p53, which is more prevalent in certain African populations, has been associated with increased susceptibility to cancer and resistance of cancer cells to p53 (Jennis et al., 2016). However, further research is needed to explore other functions of these specific mutations in addition to impairing the pro-ferroptotic activity of p53. Moreover, tumor suppressor factor BAP1, an epigenetic regulator, has also been shown to downregulate SLC7A11 expression to promote ferroptosis, but the extent of BAP1’s pro-ferroptotic activity in tumor suppression remains unclear (Zhang et al., 2018).
Ischemia-reperfusion injury and iron death
Ischemia-reperfusion injury (IRI) results in widespread cell death and inflammatory responses in affected organs, leading to severe conditions such as stroke, ischemic heart disease, liver, and kidney damage. Ischemic heart disease remains one of the deadliest diseases globally.
In studies related to the brain and heart, ferroptosis plays a crucial role in neurotoxicity and brain injury, suggesting that inhibiting ferroptosis might have therapeutic potential. Experiments in vitro have demonstrated that ferroptosis inhibitor, ferrostatin-1, can block glutamate-induced neurotoxic cell death (Lau and Tymianski, 2010). As glutamate-induced neurotoxicity is associated with conditions like stroke and various neurodegenerative diseases, and high extracellular glutamate concentrations induce ferroptosis by inhibiting the Xc-system, it suggests that ferroptosis might be one of the pathological mechanisms in these brain-related diseases (Dixon et al., 2012b). Genetic studies have shown that conditional deletion of GPX4 in mice leads to symptoms resembling neurodegenerative diseases. Iron chelators and lipophilic radical-trapping antioxidants (RTAs) have been used in various experimental systems to test their effectiveness in improving stroke and neurodegeneration (Wirth et al., 2010; Chen et al., 2015; Hambright et al., 2017). While systemic use of iron chelators poses serious side effects, these studies confirm the importance of ferroptosis in these diseases.
Regarding ischemic heart disease, extensive research has been conducted on the role of ferroptosis. Studies indicate that in an ex vivo system simulating ischemia-reperfusion injury in mouse hearts, iron ion chelators and glutamine breakdown inhibitors significantly reduce myocardial cell death, alleviate cardiac tissue damage, and improve its function (Gao et al., 2015). This suggests the potential value of targeting ferroptosis for treating ischemic heart disease, a view supported by recent in vivo studies in mouse models. Designing effective ferroptosis-targeted therapies for acute diseases like stroke and ischemic heart disease requires prompt application, rapid efficacy, and the development of specific inhibitors to minimize side effects on other organs.
Furthermore, in ischemia-reperfusion injury associated with organ transplantation, ferroptosis appears to particularly affect the kidneys (Gao et al., 2015). Survival of proximal renal tubular cells depends on functional GPX4, making these cells highly susceptible during kidney transplantation-associated IRI. Studies have demonstrated that ferroptosis inhibitors alleviate renal tubular cell death and acute kidney failure in models of renal tubular cell IRI in mice, GPX4 knockout mice, and folate-induced acute kidney injury models (Friedmann Angeli et al., 2014; Linkermann et al., 2014). The liver and heart are also associated with organ transplantation (Gao et al., 2015). Overall, ferroptosis inhibitors may contribute to effective treatments for successful organ transplantation across various organs. These findings suggest that inhibiting ferroptosis could be a potential therapeutic method for treating IRI-related diseases.
Significance and outlook: regulating ferroptosis in tumor immunotherapy
Given the complexity of ferroptosis and its direct impact on various metabolic pathways, it is highly probable that the immune system targets certain crucial steps within the ferroptotic process. In the realm of combining immunotherapy with cysteine deprivation, research suggests that CD8+ T cells activated by anti-PD-L1 immunotherapy secrete IFN-γ, promoting ferroptosis in tumor cells post PD-L1 blockade (Wang et al., 2019). IFN-γ secretion notably downregulates the expression of SLC3A2 and SLC7A11 genes in tumor cells, leading to reduced cysteine intake, enhanced lipid peroxidation, and subsequent ferroptosis. Therefore, the combined application of cysteine/cystine dioxygenase with anti-PD-L1 immunotherapy might elicit effective anti-tumor immune responses by inducing ferroptosis.
Regarding the combination of immunotherapy with targeted treatments, recent studies highlight overcoming the resistance to anti-PD-L1 therapy through a combined approach involving TYR03 receptor tyrosine kinase (RTK) inhibitors. TYR03 inhibitors facilitate ferroptosis by modulating the expression of ferroptosis-related genes such as SLC3A2, particularly noted in PD-1-resistant tumors (Xu et al., 2021a). In mouse models of breast cancer, inhibiting TYR03 promotes ferroptosis and heightens the sensitivity of tumors to PD-1 therapy. This research indicates that disrupting ferroptosis via TYR03 inhibitors might be an effective strategy to overcome immunotherapy resistance.
Additionally, evidence suggests a synergistic effect between immunotherapy and radiotherapy (Sun et al., 2018), where radiotherapy enhances sensitivity to ferroptosis. Radiation has been shown to induce ferroptosis by inducing ROS production and upregulating ACSL4, enhancing lipid synthesis, lipid peroxidation, and subsequent membrane damage (Lei et al., 2020; Ye et al., 2020; Lei et al., 2021). The combination of radiotherapy and immunotherapy downregulates SLC7A11 gene expression, mediated by ATM and IFN-γ induced by DNA damage, resulting in reduced cysteine intake, increased ferroptosis, and enhanced tumor control (Jiang et al., 2021). These studies reveal the significance of ferroptosis in the synergistic effects of immunotherapy and radiotherapy.
Finally, immunotherapy might also combine with inhibitors of T-cell ferroptosis, as the ferroptosis of T cells themselves might weaken their immune responses (Xu et al., 2021a; Xu H. et al., 2021). T cells lacking GPX4 accumulate membrane lipid peroxides, leading to ferroptosis. Increased expression of CD36 in tumor-infiltrating CD8+ T cells might induce lipid peroxidation, causing functional impairments in these T cells (Xu et al., 2021c; Ma et al., 2021). These findings underscore CD8+ T cell ferroptosis as a new mode of tumor immune suppression, highlighting the potential therapeutic benefit of blocking CD36 to enhance anti-tumor immunity. However, GPX4 inhibitors might adversely affect T cells in inducing ferroptosis, leading to unnecessary targeted effects and toxicities.
These latest findings underscore the importance of ferroptosis in immunotherapy and how modulating ferroptosis can enhance or diminish the effectiveness of immunotherapy. Considering the role of ferroptosis in immunotherapy holds significant clinical implications in devising strategies for tumor treatment.
Conclusion and perspectives
Since its discovery in 2012, ferroptosis has become a promising therapeutic target in cancer research. Several researchers are exploring its clinical roles and pathogenesis, and new ferroptosis-inducing agents are being used for therapy. Ferroptosis is a novel mode of iron-dependent programmed cell death, which is induced by small molecules such as erastin and RSL3 (Table 2). The process is regulated by multiple metabolic pathways such as iron metabolism, GSH metabolism and lipid metabolism, and is accompanied by lethal ROS accumulation, which leads to lipid peroxidation of the cell membrane. However, specific molecular markers for ferroptosis are not available, and the relevant mechanisms need to be further studied. This unique cell death pattern has generated numerous chemotherapeutic possibilities. However, the molecular mechanism of ferroptosis in cancer and its association with other diseases remains to be clarified. Several questions are unanswered in this context. For example, are there other pathways of ferroptosis regulation in addition to the classical pathway? Which is the main pathway that catalyzes the generation of lipid peroxides, the nonenzymatic or enzymatic pathway? Is there any mechanism of ferroptosis resistance? What are the molecular targets of ferroptosis-inducing therapy? How can ferroptosis-inducing antitumor drugs be used in clinical practice? Therefore, further research is required to comprehensively explore the mechanism of ferroptosis for eliminating aggressive tumors.
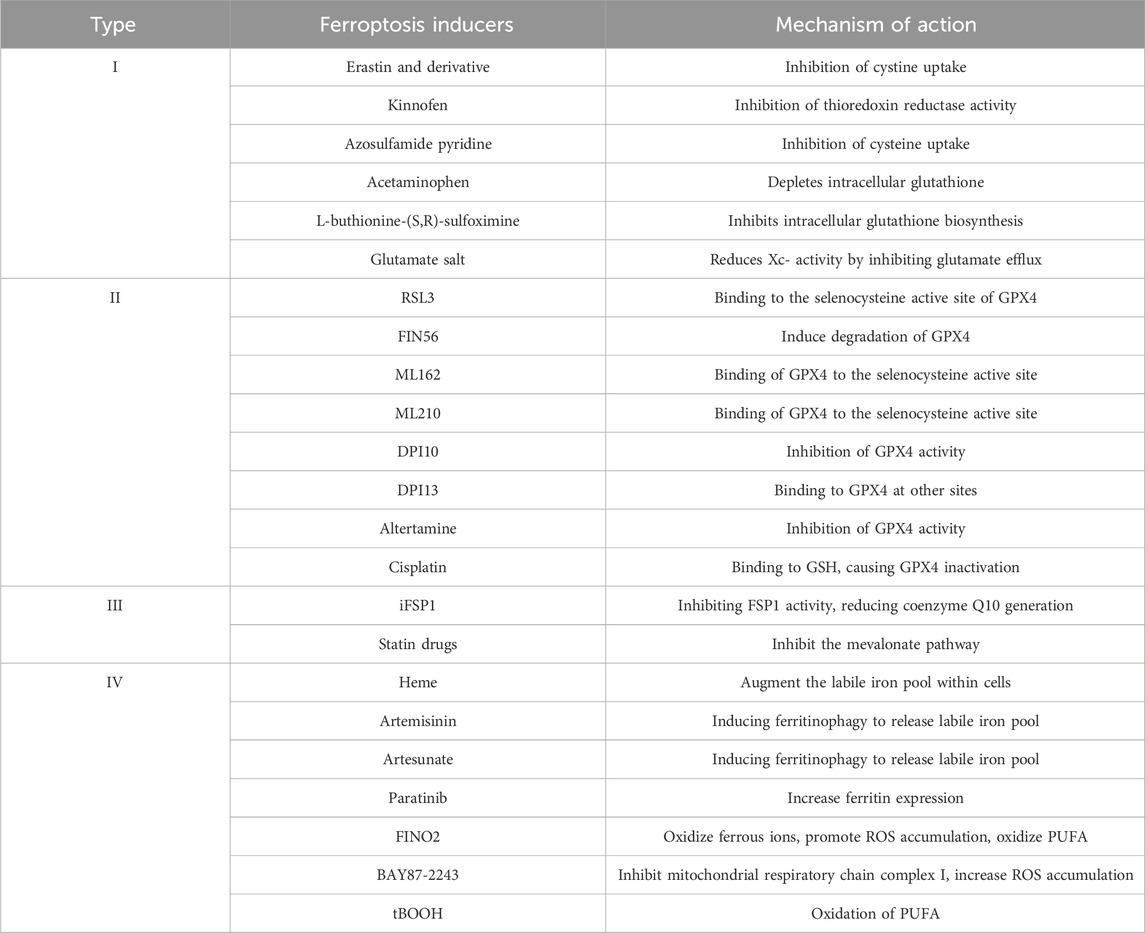
TABLE 2. Based on different mechanisms, currently known iron-induced cell death inducers can be broadly categorized into four types: Class I: Inhibitors of Xc-system; Class II: Inhibitors or degraders of GPX4; Class III: Depleters of coenzyme Q10; Class IV: Inducers of lipid peroxidation via iron or polyunsaturated fatty acid (PUFA) overload. These four types exhibit high specificity in inducing iron-dependent cell death, meaning that during the induction of iron-induced cell death, there is minimal activation of other types of cell death markers.
Taken together, multi-directional insights into the underlying regulatory mechanism of ferroptosis have great significance in understanding the pathogenesis, preventing the diseases, and designing therapeutic interventions. Therefore, new studies dedicated to the fundamental research of ferroptosis should be encouraged. The crosstalk between ferroptosis and other forms of cell deaths is another potential research area to determine their cooperation and modulation in cells and tissues. These studies will provide clues for the regulatory mechanism of ferroptosis, and ferroptosis will be a promising therapeutic target in translational medicine.
Five references that we consider to be key references:
1. Dixon SJ, Lemberg KM, Lamprecht MR, Skouta R, Zaitsev EM, Gleason CE, Patel DN, et al. Ferroptosis: an iron-dependent form of nonapoptotic cell death. CELL 2012, 149: 1060-1072
2. Bannai S, Tsukeda H, Okumura H. Effect of antioxidants on cultured human diploid fibroblasts exposed to cystine-free medium. Biochem Biophys Res Commun 1977, 74: 1582-1588
3. Alvarez SW, Sviderskiy VO, Terzi EM, Papagiannakopoulos T, Moreira AL, Adams S, Sabatini DM, et al. NFS1 undergoes positive selection in lung tumours and protects cells from ferroptosis. NATURE 2017, 551: 639-643
4. Yang WS, SriRamaratnam R, Welsch ME, Shimada K, Skouta R, Viswanathan VS, Cheah JH, et al. Regulation of ferroptotic cancer cell death by GPX4. CELL 2014, 156: 317-331
5. Badgley MA, Kremer DM, Maurer HC, DelGiorno KE, Lee HJ, Purohit V, Sagalovskiy IR, et al. Cysteine depletion induces pancreatic tumor ferroptosis in mice. SCIENCE 2020, 368: 85-89
Author contributions
YK: Conceptualization, Data curation, Formal Analysis, Funding acquisition, Investigation, Methodology, Project administration, Resources, Software, Supervision, Validation, Visualization, Writing–original draft, Writing–review and editing. JL: Investigation, Methodology, Writing–review and editing. RL: Software, Writing–review and editing. SL: Software, Writing–review and editing. LR: Resources, Writing–review and editing. YX: Resources, Writing–review and editing. YF: Conceptualization, Data curation, Formal Analysis, Funding acquisition, Investigation, Methodology, Project administration, Resources, Software, Supervision, Validation, Visualization, Writing–review and editing.
Funding
The author(s) declare financial support was received for the research, authorship, and/or publication of this article. This study was supported by the Natural Science Foundation of Shandong Province, China (No. ZR2021QH244) and Clinical Medicine Science and Technology Planning Project of Jinan of China (No. 202019135).
Acknowledgments
We would like to thank Bullet Edits Limited for the linguistic editing and proofreading of the manuscript.
Conflict of interest
The authors declare that the research was conducted in the absence of any commercial or financial relationships that could be construed as a potential conflict of interest.
Publisher’s note
All claims expressed in this article are solely those of the authors and do not necessarily represent those of their affiliated organizations, or those of the publisher, the editors and the reviewers. Any product that may be evaluated in this article, or claim that may be made by its manufacturer, is not guaranteed or endorsed by the publisher.
References
Alvarez, S. W., Sviderskiy, V. O., Terzi, E. M., Papagiannakopoulos, T., Moreira, A. L., Adams, S., et al. (2017). NFS1 undergoes positive selection in lung tumours and protects cells from ferroptosis. NATURE 551, 639–643. doi:10.1038/nature24637
Badgley, M. A., Kremer, D. M., Maurer, H. C., DelGiorno, K. E., Lee, H. J., Purohit, V., et al. (2020). Cysteine depletion induces pancreatic tumor ferroptosis in mice. SCIENCE 368, 85–89. doi:10.1126/science.aaw9872
Bannai, S., Tsukeda, H., and Okumura, H. (1977). Effect of antioxidants on cultured human diploid fibroblasts exposed to cystine-free medium. Biochem. Biophys. Res. Commun. 74, 1582–1588. doi:10.1016/0006-291x(77)90623-4
Chen, L. J., Hambright, W. S., Na, R., and Ran, R. (2015). Ablation of the ferroptosis inhibitor glutathione peroxidase 4 in neurons results in rapid motor neuron degeneration and paralysis. J. Biol. Chem. 290, 28097–28106. doi:10.1074/jbc.M115.680090
Daher, R., Manceau, H., and Karim, Z. (2017). Iron metabolism and the role of the iron-regulating hormone hepcidin in health and disease. PRESSE Med. 46, e272–e278. doi:10.1016/j.lpm.2017.10.006
Dai, C., Chen, X., Li, J., Comish, P., Kang, R., and Tang, D. (2020). Transcription factors in ferroptotic cell death. CANCER GENE Ther. 27, 645–656. doi:10.1038/s41417-020-0170-2
Dixon, S. J., Lemberg, K. M., Lamprecht, M. R., Skouta, R., Zaitsev, E. M., Gleason, C. E., et al. (2012b). Ferroptosis: an iron-dependent form of nonapoptotic cell death. Cell 149, 1060–1072. doi:10.1016/j.cell.2012.03.042
Dixon, S. J., Lemberg, K. M., Lamprecht, M. R., Skouta, R., Zaitsev, E. M., Gleason, C. E., et al. (2012a). Ferroptosis: an iron-dependent form of nonapoptotic cell death. Cell 149, 1060–1072. doi:10.1016/j.cell.2012.03.042
Dixon, S. J., Winter, G. E., Musavi, L. S., Lee, E. D., Snijder, B., Rebsamen, M., et al. (2015). Human haploid cell genetics reveals roles for lipid metabolism genes in nonapoptotic cell death. ACS Chem. Biol. 10, 1604–1609. doi:10.1021/acschembio.5b00245
Doll, S., and Conrad, M. (2017). Iron and ferroptosis: a still ill-defined liaison. IUBMB LIFE 69, 423–434. doi:10.1002/iub.1616
Doll, S., Proneth, B., Tyurina, Y. Y., Panzilius, E., Kobayashi, S., Ingold, I., et al. (2017). ACSL4 dictates ferroptosis sensitivity by shaping cellular lipid composition. Nat. Chem. Biol. 13, 91–98. doi:10.1038/nchembio.2239
Dolma, S., Lessnick, S. L., Hahn, W. C., and Stockwell, B. R. (2003). Identification of genotype-selective antitumor agents using synthetic lethal chemical screening in engineered human tumor cells. CANCER Cell 3, 285–296. doi:10.1016/s1535-6108(03)00050-3
Eagle, H. (1955). The specific amino acid requirements of a human carcinoma cell (Stain HeLa) in tissue culture. J. Exp. Med. 102, 37–48. doi:10.1084/jem.102.1.37
Felley-Bosco, E., and Gray, S. G. (2019). Mesothelioma driver genes, ferroptosis, and therapy. Front. Oncol. 9, 1318. doi:10.3389/fonc.2019.01318
Friedmann, A. J., Schneider, M., Proneth, B., Tyurina, Y. Y., Tyurin, V. A., Hammond, V. J., et al. (2014). Inactivation of the ferroptosis regulator Gpx4 triggers acute renal failure in mice. Nat. Cell Biol. 16, 1180–1191. doi:10.1038/ncb3064
Friedmann Angeli, J. P., Schneider, M., Proneth, B., Tyurina, Y. Y., Tyurin, V. A., Hammond, V. J., et al. (2014). Inactivation of the ferroptosis regulator Gpx4 triggers acute renal failure in mice. Nat. Cell Biol. 16, 1180–1191. doi:10.1038/ncb3064
Fuchs, Y., and Steller, H. (2015). Live to die another way: modes of programmed cell death and the signals emanating from dying cells. Nat. Rev. Mol. Cell Biol. 16, 329–344. doi:10.1038/nrm3999
Galluzzi, L., Bravo-San, P. J., Vitale, I., Aaronson, S. A., Abrams, J. M., Adam, D., et al. (2015). Essential versus accessory aspects of cell death: recommendations of the NCCD 2015. Cell DEATH Differ. 22, 58–73. doi:10.1038/cdd.2014.137
Gao, M., Monian, P., Quadri, N., Ramasamy, R., and Jiang, X. (2015). Glutaminolysis and transferrin regulate ferroptosis. Mol. Cell 59, 298–308. doi:10.1016/j.molcel.2015.06.011
Gilloteaux, J., Jamison, J. M., Neal, D. R., Loukas, M., Doberzstyn, T., and Summers, J. L. (2010). Cell damage and death by autoschizis in human bladder (RT4) carcinoma cells resulting from treatment with ascorbate and menadione. Ultrastruct. Pathol. 34, 140–160. doi:10.3109/01913121003662304
Hambright, W. S., Fonseca, R. S., Chen, L. J., Na, R., and Ran, S. (2017). Ablation of ferroptosis regulator glutathione peroxidase 4 in forebrain neurons promotes cognitive impairment and neurodegeneration. Redox Biol. 12, 8–17. doi:10.1016/j.redox.2017.01.021
Hassannia, B., Vandenabeele, P., and Vanden, B. T. (2019). Targeting ferroptosis to iron out cancer. CANCER Cell 35, 830–849. doi:10.1016/j.ccell.2019.04.002
Hassannia, B., Wiernicki, B., Ingold, I., Qu, F., Van Herck, S., Tyurina, Y. Y., et al. (2018). Nano-targeted induction of dual ferroptotic mechanisms eradicates high-risk neuroblastoma. J. Clin. Invest. 128, 3341–3355. doi:10.1172/JCI99032
Hou, W., Xie, Y., Song, X., Sun, X., Lotze, M. T., Zeh, H. R., et al. (2016). Autophagy promotes ferroptosis by degradation of ferritin. AUTOPHAGY 12, 1425–1428. doi:10.1080/15548627.2016.1187366
Jennis, M., Kung, C. P., Basu, S., Budina-Kolomets, A., Leu, J. I. J., Khaku, S., et al. (2016). An African-specific polymorphism in the TP53 gene impairs p53 tumor suppressor function in a mouse model. Genes Dev. 30, 918–930. doi:10.1101/gad.275891.115
Jiang, Z., Lim, S. O., Yan, M., Hsu, J. L., Yao, J., Wei, Y., et al. (2021). TYRO3 induces anti-PD-1/PD-L1 therapy resistance by limiting innate immunity and tumoral ferroptosis. J. Clin. Invest. 131, e139434. doi:10.1172/JCI139434
Kakhlon, O., and Cabantchik, Z. I. (2002). The labile iron pool: characterization, measurement, and participation in cellular processes (1). Free Radic. Biol. Med. 33, 1037–1046. doi:10.1016/s0891-5849(02)01006-7
Kang, R., Kroemer, G., and Tang, D. (2019). The tumor suppressor protein p53 and the ferroptosis network. Free Radic. Biol. Med. 133, 162–168. doi:10.1016/j.freeradbiomed.2018.05.074
Kim, K. M., Cho, S. S., and Ki, S. H. (2020). Emerging roles of ferroptosis in liver pathophysiology. Arch. Pharm. Res. 43, 985–996. doi:10.1007/s12272-020-01273-8
Kuang, F., Liu, J., Tang, D., and Kang, R. (2020). Oxidative damage and antioxidant defense in ferroptosis. Front. Cell Dev. Biol. 8, 586578. doi:10.3389/fcell.2020.586578
Lau, A., and Tymianski, M. (2010). Glutamate receptors, neurotoxicity and neurodegeneration. Pflug Arch. Eur. J. Phy 460, 525–542. doi:10.1007/s00424-010-0809-1
Lei, G., Mao, C., Yan, Y., Zhuang, L., and Gan, B. (2021). Ferroptosis, radiotherapy, and combination therapeutic strategies. Protein Cell 12, 836–857. doi:10.1007/s13238-021-00841-y
Lei, G., Zhang, Y., Koppula, P., Liu, X., Zhang, J., Lin, S. H., et al. (2020). The role of ferroptosis in ionizing radiation-induced cell death and tumor suppression. Cell Res. 30, 146–162. doi:10.1038/s41422-019-0263-3
Lei, P., Bai, T., and Sun, Y. (2019). Mechanisms of ferroptosis and relations with regulated cell death: a review. Front. Physiol. 10, 139. doi:10.3389/fphys.2019.00139
Li, J., Cao, F., Yin, H. L., Huang, Z. J., Lin, Z. T., Mao, N., et al. (2020a). Ferroptosis: past, present and future. Cell DEATH Dis. 11, 88. doi:10.1038/s41419-020-2298-2
Li, Y., Jin, C., Shen, M., Wang, Z., Tan, S., Chen, A., et al. (2020b). Iron regulatory protein 2 is required for artemether -mediated anti-hepatic fibrosis through ferroptosis pathway. Free Radic. Biol. Med. 160, 845–859. doi:10.1016/j.freeradbiomed.2020.09.008
Li, Y., Yan, H., Xu, X., Liu, H., Wu, C., and Zhao, L. (2020c). Erastin/sorafenib induces cisplatin-resistant non-small cell lung cancer cell ferroptosis through inhibition of the Nrf2/xCT pathway. Oncol. Lett. 19, 323–333. doi:10.3892/ol.2019.11066
Linkermann, A., Skouta, R., Himmerkus, N., Mulay, S. R., Dewitz, C., De Zen, F., et al. (2014). Synchronized renal tubular cell death involves ferroptosis. Proc. Natl. Acad. Sci. U. S. A. 111, 16836–16841. doi:10.1073/pnas.1415518111
Ma, X., Xiao, L., Liu, L., Ye, L., Su, P., Bi, E., et al. (2021). CD36-mediated ferroptosis dampens intratumoral CD8+ T cell effector function and impairs their antitumor ability. Cell Metab. 33, 1001–1012. doi:10.1016/j.cmet.2021.02.015
Mancias, J. D., Wang, X., Gygi, S. P., Harper, J. W., and Kimmelman, A. C. (2014). Quantitative proteomics identifies NCOA4 as the cargo receptor mediating ferritinophagy. NATURE 509, 105–109. doi:10.1038/nature13148
Shi, Z., Zhang, L., Zheng, J., Sun, H., and Ferroptosis, S. C. (2021). Ferroptosis: biochemistry and Biology in cancers. Front. Oncol. 11, 579286. doi:10.3389/fonc.2021.579286
Shimada, K., Hayano, M., Pagano, N. C., and Stockwell, B. R. (2016b). Cell-line selectivity improves the predictive power of pharmacogenomic analyses and helps identify NADPH as biomarker for ferroptosis sensitivity. Cell Chem. Biol. 23, 225–235. doi:10.1016/j.chembiol.2015.11.016
Shimada, K., Skouta, R., Kaplan, A., Yang, W. S., Hayano, M., Dixon, S. J., et al. (2016a). Global survey of cell death mechanisms reveals metabolic regulation of ferroptosis. Nat. Chem. Biol. 12, 497–503. doi:10.1038/nchembio.2079
Stockwell, B. R., Friedmann, A. J., Bayir, H., Bush, A. I., Conrad, M., Dixon, S. J., et al. (2017). Ferroptosis: a regulated cell death nexus linking metabolism, redox Biology, and disease. Cell 171, 273–285. doi:10.1016/j.cell.2017.09.021
Sun, L. L., Yang, R. Y., Li, C. W., Chen, M. K., Shao, B., Hsu, J. M., et al. (2018). Inhibition of ATR downregulates PD-L1 and sensitizes tumor cells to T cell-mediated killing. Am. J. Cancer Res. 8, 1307–1316.
Sun, X., Ou, Z., Xie, M., Kang, R., Fan, Y., Niu, X., et al. (2015). HSPB1 as a novel regulator of ferroptotic cancer cell death. ONCOGENE 34, 5617–5625. doi:10.1038/onc.2015.32
Tang, D., Kang, R., Berghe, T. V., Vandenabeele, P., and Kroemer, G. (2019). The molecular machinery of regulated cell death. Cell Res. 29, 347–364. doi:10.1038/s41422-019-0164-5
Tsurusaki, S., Tsuchiya, Y., Koumura, T., Nakasone, M., Sakamoto, T., Matsuoka, M., et al. (2019). Hepatic ferroptosis plays an important role as the trigger for initiating inflammation in nonalcoholic steatohepatitis. Cell DEATH Dis. 10, 449. doi:10.1038/s41419-019-1678-y
Wang, S. J., Li, D., Ou, Y., Jiang, L., Chen, Y., Zhao, Y., et al. (2016). Acetylation is crucial for p53-mediated ferroptosis and tumor suppression. Cell Rep. 17, 366–373. doi:10.1016/j.celrep.2016.09.022
Wang, W., Green, M., Choi, J. E., Gijón, M., Kennedy, P. D., Johnson, J. K., et al. (2019). CD8+ T cells regulate tumour ferroptosis during cancer immunotherapy. Nature 569, 270–274. doi:10.1038/s41586-019-1170-y
Wirth, E. K., Conrad, M., Winterer, J., Wozny, C., Carlson, B. A., Roth, S., et al. (2010). Neuronal selenoprotein expression is required for interneuron development and prevents seizures and neurodegeneration. Faseb J. 24, 844–852. doi:10.1096/fj.09-143974
Xie, Y., Hou, W., Song, X., Yu, Y., Huang, J., Sun, X., et al. (2016). Ferroptosis: process and function. Cell DEATH Differ. 23, 369–379. doi:10.1038/cdd.2015.158
Xu, H., Ye, D., Ren, M., Zhang, H., and Bi, F. (2021b). Ferroptosis in the tumor microenvironment: perspectives for immunotherapy. Trends Mol. Med. 27, 856–867. doi:10.1016/j.molmed.2021.06.014
Xu, S., Chaudhary, O., Rodríguez-Morales, P., Sun, X., Chen, D., Zappasodi, R., et al. (2021c). Uptake of oxidized lipids by the scavenger receptor CD36 promotes lipid peroxidation and dysfunction in CD8+ T cells in tumors. Immunity 54, 1561–1577.e7. doi:10.1016/j.immuni.2021.05.003
Xu, S., Min, J., and Wang, F. (2021a). Ferroptosis: an emerging player in immune cells. Sci. Bull. 66, 2257–2260. doi:10.1016/j.scib.2021.02.026
Yang, W. S., SriRamaratnam, R., Welsch, M. E., Shimada, K., Skouta, R., Viswanathan, V. S., et al. (2014). Regulation of ferroptotic cancer cell death by GPX4. Cell 156, 317–331. doi:10.1016/j.cell.2013.12.010
Yang, W. S., and Stockwell, B. R. (2008). Synthetic lethal screening identifies compounds activating iron-dependent, nonapoptotic cell death in oncogenic-RAS-harboring cancer cells. Chem. Biol. 15, 234–245. doi:10.1016/j.chembiol.2008.02.010
Ye, L. F., Chaudhary, K. R., Zandkarimi, F., Harken, A. D., Kinslow, C. J., Upadhyayula, P. S., et al. (2020). Radiation-induced lipid peroxidation triggers ferroptosis and synergizes with ferroptosis inducers. ACS Chem. Biol. 15, 469–484. doi:10.1021/acschembio.9b00939
Yin, H., Xu, L., and Porter, N. A. (2011). Free radical lipid peroxidation: mechanisms and analysis. Chem. Rev. 111, 5944–5972. doi:10.1021/cr200084z
Keywords: ferroptosis, cell death, iron metabolism, reactive oxygen species, GPX4
Citation: Kong Y, Li J, Lin R, Lu S, Rong L, Xue Y and Fang Y (2024) Understanding the unique mechanism of ferroptosis: a promising therapeutic target. Front. Cell Dev. Biol. 11:1329147. doi: 10.3389/fcell.2023.1329147
Received: 02 November 2023; Accepted: 31 December 2023;
Published: 18 March 2024.
Edited by:
Carsten Culmsee, University of Marburg, GermanyReviewed by:
Chuanhui Song, Nanjing Drum Tower Hospital, ChinaQingbin Cui, University of Toledo College of Medicine and Life Sciences, United States
Copyright © 2024 Kong, Li, Lin, Lu, Rong, Xue and Fang. This is an open-access article distributed under the terms of the Creative Commons Attribution License (CC BY). The use, distribution or reproduction in other forums is permitted, provided the original author(s) and the copyright owner(s) are credited and that the original publication in this journal is cited, in accordance with accepted academic practice. No use, distribution or reproduction is permitted which does not comply with these terms.
*Correspondence: Yongjun Fang, fyj322@126.com