Identification of multiple transcription factor genes potentially involved in the development of electrosensory versus mechanosensory lateral line organs
- 1Department of Physiology, Development and Neuroscience, University of Cambridge, Cambridge, United Kingdom
- 2Department of Zoology, University of Cambridge, Cambridge, United Kingdom
- 3Josephine Bay Paul Center for Comparative Molecular Biology and Evolution, Marine Biological Laboratory, Woods Hole, MA, United States
- 4Department of Genetics, University of Cambridge, Cambridge, United Kingdom
- 5Faculty of Fisheries and Protection of Waters, Research Institute of Fish Culture and Hydrobiology, University of South Bohemia in České Budějovice, Vodňany, Czechia
In electroreceptive jawed vertebrates, embryonic lateral line placodes give rise to electrosensory ampullary organs as well as mechanosensory neuromasts. Previous reports of shared gene expression suggest that conserved mechanisms underlie electroreceptor and mechanosensory hair cell development and that electroreceptors evolved as a transcriptionally related “sister cell type” to hair cells. We previously identified only one transcription factor gene, Neurod4, as ampullary organ-restricted in the developing lateral line system of a chondrostean ray-finned fish, the Mississippi paddlefish (Polyodon spathula). The other 16 transcription factor genes we previously validated in paddlefish were expressed in both ampullary organs and neuromasts. Here, we used our published lateral line organ-enriched gene-set (arising from differential bulk RNA-seq in late-larval paddlefish), together with a candidate gene approach, to identify 25 transcription factor genes expressed in the developing lateral line system of a more experimentally tractable chondrostean, the sterlet (Acipenser ruthenus, a small sturgeon), and/or that of paddlefish. Thirteen are expressed in both ampullary organs and neuromasts, consistent with conservation of molecular mechanisms. Seven are electrosensory-restricted on the head (Irx5, Irx3, Insm1, Sp5, Satb2, Mafa and Rorc), and five are the first-reported mechanosensory-restricted transcription factor genes (Foxg1, Sox8, Isl1, Hmx2 and Rorb). However, as previously reported, Sox8 is expressed in ampullary organs as well as neuromasts in a catshark (Scyliorhinus canicula), suggesting the existence of lineage-specific differences between cartilaginous and ray-finned fishes. Overall, our results support the hypothesis that ampullary organs and neuromasts develop via largely conserved transcriptional mechanisms, and identify multiple transcription factors potentially involved in the formation of electrosensory versus mechanosensory lateral line organs.
Introduction
In jawed anamniotes, mechanosensory hair cells are found in the inner ear, in the spiracular organ associated with the first pharyngeal cleft (lost in amphibians, bichirs and teleosts; see Norris and Hughes, 1920; von Bartheld and Giannessi, 2011), and in lateral line neuromasts: small sense organs distributed in lines over the head and trunk, which respond to local water movement (see, e.g., Mogdans, 2019; Webb, 2021). In electroreceptive species (e.g., cartilaginous fishes, ray-finned fishes including the chondrostean paddlefishes and sturgeons, and urodele amphibians like the axolotl), the lateral line system includes electrosensory ampullary organs containing supporting cells and electroreceptors that detect weak electric fields, primarily for hunting or avoiding predators (see, e.g., Crampton, 2019; Leitch and Julius, 2019; Chagnaud et al., 2021).
Like hair cells, electroreceptors have an apical primary cilium (lost during maturation in cochlear hair cells; Lu and Sipe, 2016; Elliott et al., 2018) and basolateral ribbon synapses with afferent nerve terminals (Jørgensen, 2005; Baker, 2019). However, electroreceptors lack the apical hair bundle (staircase array of microvilli) where mechanoelectrical transduction occurs (Ó Maoiléidigh and Ricci, 2019; Caprara and Peng, 2022). The main anamniote developmental models—the teleost zebrafish and the frog Xenopus—lack ampullary organs: electroreception was lost in the ray-finned bony fish radiation leading to teleosts, and in the lobe-finned bony fish (amphibian) lineage leading to frogs (Baker et al., 2013; Baker, 2019; Crampton, 2019). Physiologically distinct lateral line electroreceptors evolved independently in some teleost lineages (see, e.g., Baker et al., 2013; Baker, 2019; Crampton, 2019).
Neuromasts and ampullary organs, together with the neurons in lateral line ganglia that provide their afferent innervation (projecting centrally to different hindbrain nuclei; Wullimann and Grothe, 2014), develop from a series of cranial lateral line placodes (Northcutt, 1997; Baker et al., 2013; Piotrowski and Baker, 2014). These either elongate to form sensory ridges that fragment, with a line of neuromasts forming first in the ridge’s centre and ampullary organs (if present) forming later on the ridge’s flanks (Northcutt, 1997; Piotrowski and Baker, 2014). Alternatively, as in the zebrafish (a teleost ray-finned fish), lateral line primordia migrate as cell collectives, depositing neuromasts in their wake (Piotrowski and Baker, 2014). The lateral line placode origin of ampullary organs was first shown by grafting and ablation in a urodele amphibian, the axolotl (Northcutt et al., 1995). Our DiI-labelling studies in a chondrostean ray-finned fish (Mississippi paddlefish, Polyodon spathula) (Modrell et al., 2011a) and a cartilaginous fish (little skate, Leucoraja erinacea) (Gillis et al., 2012) showed that individual elongating lateral line placodes form ampullary organs and neuromasts across all non-teleost jawed vertebrates (reviewed in Baker et al., 2013).
What molecular mechanisms underlie the formation of ampullary organs versus neuromasts within the same lateral line sensory ridge? We have identified a range of ampullary organ-expressed genes in different electroreceptive vertebrates using a candidate gene approach (O’Neill et al., 2007; Modrell et al., 2011a; Modrell et al., 2011b; Gillis et al., 2012; Modrell and Baker, 2012; Modrell et al., 2017a; Modrell et al., 2017b). More recently, we took an unbiased differential RNA-seq approach, comparing the transcriptome of late-larval paddlefish gill-flaps (covered in ampullary organs, plus some neuromasts) versus fins (no lateral line organs) (Modrell et al., 2017a). The resultant lateral line organ-enriched dataset of around 500 genes (Modrell et al., 2017a) is not exhaustive: it includes most, but not all, of the genes identified in paddlefish ampullary organs via the candidate gene approach (Modrell et al., 2011a; Modrell et al., 2011b; Modrell et al., 2017a; Modrell et al., 2017b). In situ hybridization for selected candidate genes from this dataset suggested that electroreceptors and hair cells are closely related, e.g., late-larval ampullary organs express genes encoding proteins essential for neurotransmission specifically at hair-cell (but not photoreceptor) ribbon synapses in the basolateral cell membrane (Pangrsic et al., 2018; Moser et al., 2020), such as vGlut3, otoferlin and Cav1.3 (Modrell et al., 2017a). Cav1.3 has also been identified as the electrosensitive voltage-gated Ca2+ channel in the apical electroreceptor membrane (Bennett and Obara, 1986; Bodznick and Montgomery, 2005) in shark and skate species (i.e., in cartilaginous fishes) (Bellono et al., 2017; Bellono et al., 2018).
Developing ampullary organs also express key “hair cell” transcription factor genes (see Sun and Liu, 2023; Zine and Fritzsch, 2023) including Six1, Eya1, Atoh1 and Pou4f3 (Brn3c) (Modrell et al., 2011a; Butts et al., 2014; Modrell et al., 2017a). Six1 and Eya1 are jointly required to activate Sox2 in otic neurosensory progenitors (Xu et al., 2021) and cooperate with Sox2 to induce Atoh1 expression in cochlear epithelium (Ahmed et al., 2012). Six1, Atoh1 and Pou4f3 are critical for hair cell formation and, in combination with Gfi1, can drive an immature “hair cell-like” fate in mouse embryonic stem cells, adult cochlear supporting cells and fibroblasts (see Sun and Liu, 2023). Co-expression of Atoh1, Pou4f3 and Gfi1 is sufficient to drive a more mature hair cell fate in postnatal cochlear supporting cells (Chen et al., 2021; McGovern et al., 2024). The expression of Six1, Eya1, Atoh1 and Pou4f3 in developing ampullary organs, as well as neuromasts, suggests that molecular mechanisms underlying electroreceptor development are likely to be highly conserved with those underlying hair cell formation (Modrell et al., 2011a; Modrell et al., 2017a). Nevertheless, hair cells and electroreceptors are morphologically and functionally distinct (Jørgensen, 2005; Baker, 2019; Elliott and Fritzsch, 2021) and neuromasts form before ampullary organs within the same sensory ridge (Northcutt et al., 1994; Gibbs and Northcutt, 2004a; Modrell et al., 2011a). Validation of multiple candidate genes from the late-larval paddlefish lateral line organ-enriched dataset had identified only a handful of genes with expression in developing ampullary organs but not neuromasts (Modrell et al., 2017a). These were the basic helix-loop-helix (bHLH) transcription factor gene Neurod4, plus two voltage-gated potassium channel subunit genes (Kcna5, encoding Kv1.5, and Kcnab3, encoding the accessory subunit Kvβ3) and a calcium-chelating beta-parvalbumin, all presumably involved in electroreceptor function (Modrell et al., 2017a).
In recent years, another chondrostean fish, the sterlet (a sturgeon, Acipenser ruthenus), has been developed as an experimentally tractable non-teleost model (see, e.g., Saito and Psenicka, 2015; Chen et al., 2018; Baloch et al., 2019; Du et al., 2020; Stundl et al., 2020; Stundl et al., 2022; Stundl et al., 2023). In contrast to the limited Mississippi paddlefish spawning season, many hundreds of sterlet embryos are available each week for up to several months in fully equipped laboratory research facilities. We have therefore turned to the sterlet as a tractable model for studying the molecular control of lateral line hair cell and electroreceptor development. In the current study, we describe lateral line hair cell and electroreceptor differentiation in the sterlet, and the expression in sterlet and/or paddlefish of almost all of the remaining transcription factor genes from the paddlefish late-larval lateral line organ-enriched dataset (Modrell et al., 2017a), plus a few additional candidates. We report expression within the developing lateral line system of 25 novel transcription factor genes. Thirteen—including the key “hair cell” transcription factor gene Gfi1—were expressed in both ampullary organs and neuromasts, supporting conserved molecular mechanisms. Seven transcription factor genes proved to be electrosensory-restricted, while five represent the first-reported mechanosensory lateral line-restricted transcription factors. These twelve genes, plus ampullary organ-restricted Neurod4 (Modrell et al., 2017a), are good candidates to be involved in the development of electrosensory versus mechanosensory lateral line organs.
Results
Characterizing lateral line hair cell and electroreceptor development in sterlet
In order to use the sterlet as a more experimentally tractable model for lateral line organ development than the Mississippi paddlefish (Modrell et al., 2011a; Modrell et al., 2011b; Modrell et al., 2017a; Modrell et al., 2017b), we first characterized the timing and distribution of lateral line hair cell and electroreceptor differentiation (staging according to Dettlaff et al., 1993). The development of lateral line placodes, neuromasts and ampullary organs had previously been described in the shovelnose sturgeon, Scaphirhynchus platorynchus (Gibbs and Northcutt, 2004a); ampullary organ formation had also been described in a sturgeon in the same genus as the sterlet, the Adriatic sturgeon, A. naccari (Camacho et al., 2007). In S. platorynchus, all lateral line placodes are present at stage 30 and have started to elongate into sensory ridges (Gibbs and Northcutt, 2004a). By the time of hatching at stage 36, neuromast primordia are present within the sensory ridges at the centre of all the lateral line placodes but only a few mature neuromasts have formed, specifically in the otic lateral line (Gibbs and Northcutt, 2004a). At stage 41, roughly midway between hatching (stage 36) and the onset of independent feeding (stage 45), ampullary organ primordia are present in the lateral zones of the anterodorsal, anteroventral, otic and supratemporal sensory ridges (Gibbs and Northcutt, 2004a). At stage 45, mature ampullary organs are present in the infraorbital, otic and posterior preopercular fields and ampullary organs continue to develop over the next 3 weeks (Gibbs and Northcutt, 2004a). By stage 45 in A. naccari, in contrast, ampullary organs are ultrastructurally mature at almost all sites and thought to be functional (Camacho et al., 2007). Camacho et al. (2007) speculate that the difference is due to S. platorynchus reaching stage 45 on day 4 post-hatching, leaving less time for completion of ampullary organ development than in A. naccari, which reaches stage 45 on day 9 post-hatching.
To examine the formation and distribution of developing neuromasts and ampullary organs in sterlet, we focused on the stages between stage 35, i.e., the stage before hatching (stage 36), up to the onset of independent feeding at stage 45, which is reached in the sterlet at day 8 post-hatching (14 days post-fertilization, dpf) (Zeiske et al., 2003). Figure 1 shows a temporal overview of the expression by in situ hybridization (ISH) in sterlet of Eya4, an otic and lateral line primordium marker that is eventually restricted to differentiated hair cells and electroreceptors (Modrell and Baker, 2012; Baker et al., 2013) (Figures 1A–D) and Sox2, which is also expressed by lateral line primordia and is eventually restricted to supporting cells in both ampullary organs and neuromasts, with stronger expression in neuromasts (Modrell et al., 2017a) (Figures 1E–H). (Sox2 is also expressed in taste buds on the barbels and around the mouth; Figures 1E–H.) As a marker for differentiated hair cells and electroreceptors, we used Cacna1d, which encodes the pore-forming alpha subunit of Cav1.3 and is expressed in hair cells and electroreceptors across jawed vertebrates (Modrell et al., 2017a; Bellono et al., 2017; Bellono et al., 2018) (Figures 1I–L; also see Supplementary Figures S1A–H). To identify electroreceptors specifically, we cloned the two voltage-gated potassium channel subunit genes that we identified as electroreceptor-specific in paddlefish (Modrell et al., 2017a): Kcnab3, encoding the accessory subunit Kvβ3 (Figures 1M–P; also see Supplementary Figures S1I–P) and Kcna5, encoding Kv1.5, which shows the same expression pattern as Kcnab3 (data not shown.) The earliest sign of neuromast hair cell differentiation was at stage 35 (Figure 1I; Supplementary Figures S1A, B) with increasing numbers at all subsequent stages (Figures 1J–L; Supplementary Figures S1C–H). Differentiated electroreceptors were not seen until stages 40–41, in some ampullary organ fields (Figures 1I–K, M–O; Supplementary Figures S1I–N). By stage 45 (the onset of independent feeding), all cranial neuromast lines and fields of ampullary organs with differentiated electroreceptors could be identified (Figures 1D, H, L, P; Supplementary Figures S1G, H, O, P). A schematic summary is shown in Figures 1Q–T.
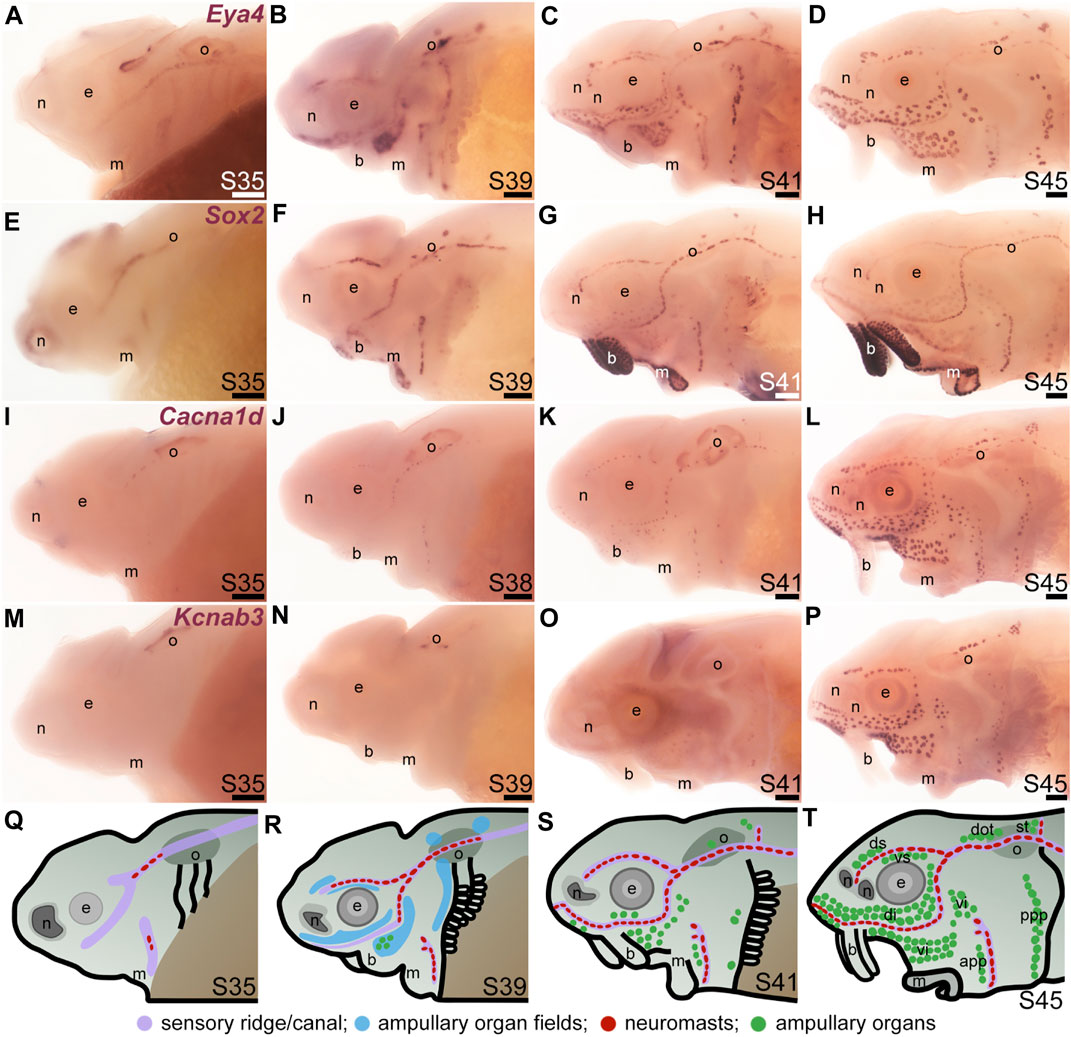
FIGURE 1. Time-course of neuromast and ampullary organ development in sterlet. In situ hybridization at selected stages in sterlet, from stage 35 (the stage before hatching occurs, at stage 36) to stage 45, the onset of independent feeding. (A–D) Eya4 expression in sensory ridges and ampullary organ fields at stages 35 and 39 subsequently resolves into individual neuromasts and ampullary organs. (E–H) A paddlefish Sox2 riboprobe reveals Sox2 expression in sensory ridges at stage 35 that later resolves into a ring-like pattern in neuromasts, with weaker expression in ampullary organs from stage 41. Very strong expression is also seen in taste buds on the barbels and around the mouth. (I–L) Expression of Cacna1d, encoding the pore-forming alpha subunit of the voltage-gated calcium channel Cav1.3, reveals differentiated hair cells in a few neuromasts already at stage 35 in the otic line, near the otic vesicle, with increasing numbers later, and some differentiated electroreceptors already at stage 41. Cacna1d is also weakly expressed in taste buds, most clearly on the barbels. (M–P) Expression of electroreceptor-specific Kcnab3 (encoding an accessory subunit for a voltage-gated K+ channel, Kvβ3) shows some differentiated electroreceptors are present by stage 41, but not earlier. (Q–T) Schematic representation of sterlet lateral line development at stages 35, 39, 41, and 45. Abbreviations: app, anterior preopercular ampullary organ field; b, barbels; di, dorsal infraorbital ampullary organ field; dot, dorsal otic ampullary organ field; ds, dorsal supraorbital ampullary organ field; e, eye; gf, gill filaments; m, mouth; n, naris; o, otic vesicle; ppp, posterior preopercular ampullary organ field; S, stage; st, supratemporal ampullary organ field; vi, ventral infraorbital ampullary organ field; vs., ventral supraorbital ampullary organ field. Scale bar: 200 μm.
“Hair cell” transcription factor genes expressed in developing ampullary organs include Gfi1, Sox4 and Sox3
We previously showed that various transcription factor genes essential for hair cell development—Six1, Eya1, Sox2, Atoh1, Pou4f3 (see Sun and Liu, 2023; Zine and Fritzsch, 2023)—are expressed in developing paddlefish ampullary organs, as well as neuromasts (Modrell et al., 2011a; Modrell et al., 2011b; Butts et al., 2014; Modrell et al., 2017a). We confirm here that, in addition to Sox2 (Figures 1E–H; Figures 2A, B), Atoh1 and Pou4f3 are also expressed in both types of lateral line organs in sterlet (Figures 2C–F). As noted earlier, Sox2 was expressed more strongly in neuromasts than ampullary organs (Figures 1G, H; Figures 2A, B). However, Atoh1 showed the converse pattern, with stronger expression in ampullary organs than in neuromasts (Figures 2C, D). These differential expression patterns were also seen at earlier stages (for Sox2, see Figures 1E–G; for Atoh1, see Supplementary Figures S2A–D).
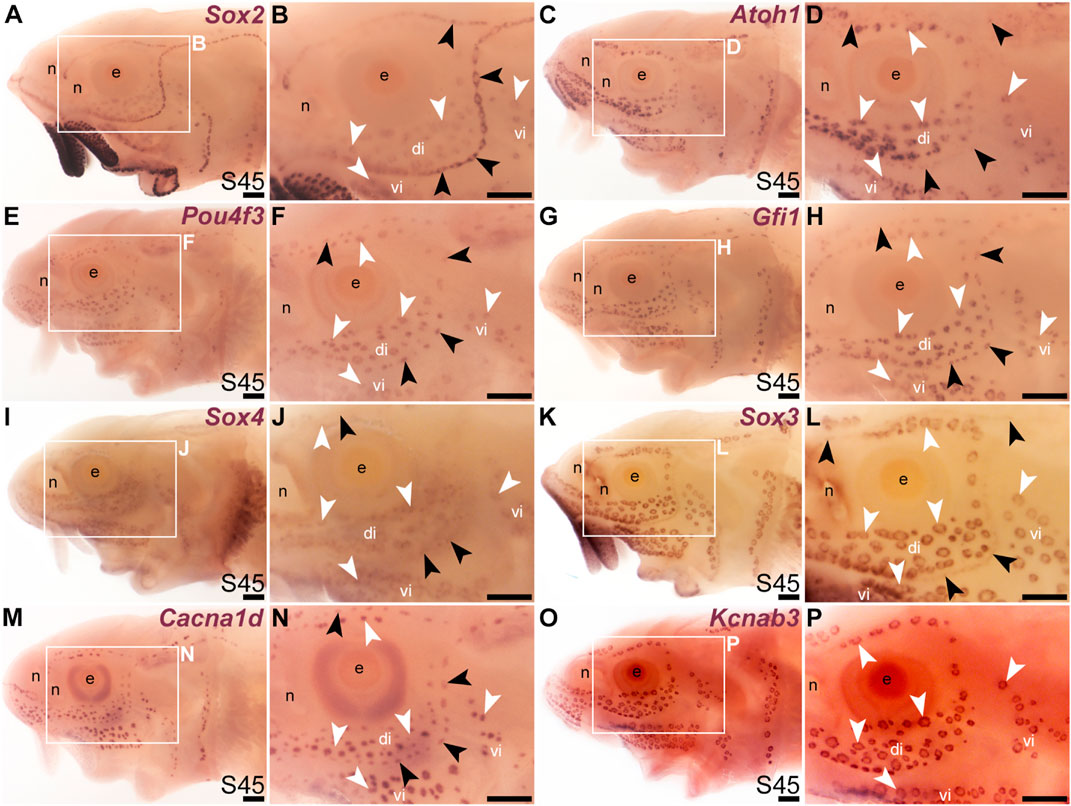
FIGURE 2. Transcription factor genes essential for hair cell development, including Gfi1, are expressed in ampullary organs as well as neuromasts. In situ hybridization in sterlet at stage 45 (the onset of independent feeding). Black arrowheads indicate examples of neuromasts; white arrowheads indicate examples of ampullary organs. (A,B) A paddlefish Sox2 riboprobe reveals strong Sox2 expression in neuromasts and weaker expression in ampullary organs (plus very strong expression in taste buds on the barbels and around the mouth). (C,D) Atoh1 is expressed more strongly in ampullary organs than in neuromasts. (E,F) Pou4f3 and (G,H) Gfi1 are expressed in both neuromasts and ampullary organs. (I,J) Sox4 is expressed in ampullary organs and very weakly in neuromasts. (K,L) Sox3 expression is weaker in neuromasts than in ampullary organs [the opposite to Sox2; compare with (A,B)]. (M,N) For comparison, the differentiated hair cell and electroreceptor marker Cacna1d is expressed in both neuromasts and ampullary organs (and also weakly in taste buds). (O,P) For comparison, the electroreceptor marker Kcnab3 is expressed in ampullary organs only. Abbreviations: di, dorsal infraorbital ampullary organ field; e, eye; n, naris; S, stage; vi, ventral infraorbital ampullary organ field. Scale bar: 200 μm.
A key “hair cell” transcription factor gene whose expression we had not previously examined is the zinc-finger transcription factor gene Gfi1 (see Sun and Liu, 2023; McGovern et al., 2024). Gfi1 was 12.0-fold enriched in late-larval paddlefish operculum versus fin tissue (Modrell et al., 2017a). Sterlet Gfi1 proved also to be expressed in developing ampullary organs, as well as neuromasts (Figures 2G, H).
In the mouse inner ear, the SoxC subfamily members Sox4 and Sox11 are co-expressed in proliferating hair cell progenitor cells and newly born hair cells, and in combination are essential for hair cell formation (Gnedeva and Hudspeth, 2015; Wang et al., 2023). Ectopic expression of either gene converts supporting cells to hair cells (Gnedeva and Hudspeth, 2015; Wang et al., 2023). A recent study showed that Sox4 confers hair-cell competence by binding lineage-specific regulatory elements and making these accessible (Wang et al., 2023). Although neither Sox4 nor Sox11 was present in the paddlefish lateral line-enriched gene-set (Modrell et al., 2017a), we cloned sterlet Sox4, which proved to be expressed in both ampullary organs and neuromasts, though more strongly in ampullary organs (Figures 2I, J). This differential expression pattern was also seen at earlier stages (Supplementary Figures S2E–H).
It has been reported that proliferative stem cells in zebrafish neuromasts express the SoxB1 subfamily member Sox3, as well as Sox2, and that Sox3 is important for the formation of the correct number of neuromast hair cells (preprint: Undurraga et al., 2019). A recent single-cell RNA sequencing (scRNA-seq) study showed Sox3 expression at homeostasis in multiple neuromast support cell types (Baek et al., 2022) including central cells, the immediate precursors of regenerating hair cells (Romero-Carvajal et al., 2015; Lush et al., 2019). We had previously used a candidate gene approach for lateral line placode markers to identify Sox3 expression in paddlefish lateral line primordia, neuromasts and also ampullary organs (Modrell et al., 2011b). (Sox3 was 5.2-fold enriched in late-larval paddlefish operculum versus fin tissue; Modrell et al., 2017a). As expected, sterlet Sox3 was also expressed in both types of lateral line organs, though much more strongly in ampullary organs than in neuromasts (Figures 2K, L). Intriguingly, this was the opposite pattern to the other SoxB1 family member, Sox2 (Figures 2A, B). This differential expression pattern was also seen at earlier stages (Supplementary Figures S2I–L). For comparison, Figures 2M, N show the hair cell and electroreceptor marker Cacna1d, and Figures 2O, P show electroreceptor-specific Kcnab3 expression.
Thus, all the key “hair cell” transcription factors are expressed in developing ampullary organs as well as neuromasts (although several show differing levels of expression between the two sensory organ types). These results provide further support for the hypothesis that electroreceptors evolved as transcriptionally related sister cell types to lateral line hair cells (Baker and Modrell, 2018).
Additional transcription factor genes expressed in developing ampullary organs and neuromasts
We cloned and analysed the expression of paddlefish and/or sterlet homologues of a further 33 transcription factor genes present in the late-larval paddlefish lateral line organ-enriched dataset (Modrell et al., 2017a), plus five others. (The paddlefish lateral line-enriched dataset also includes fifteen other loci assigned to specific transcription factor/co-factor genes, for which cloning and/or ISH failed in sterlet, or expression was inconsistent: Akna, Barx1, Egr2, Fev, Fhl2, Fhl5, Litaf, Meis3, Nkx3-1, Not2, Osr1, Pou3f1, Spdef, Tbx22 and Vgll3.) Eleven of the transcription factor genes examined were expressed in developing ampullary organs as well as neuromasts, like Gfi1 (see previous section). One was the zinc-finger transcription factor gene Insm2 (19.9-fold lateral line-enriched in late-larval paddlefish; Modrell et al., 2017a), which was expressed in both ampullary organs and neuromasts in paddlefish and sterlet (Figures 3A–D). However, Insm2 expression was much stronger in ampullary organs than in neuromasts; indeed in sterlet, Insm2 expression in neuromasts was often undetectable except in some parts of the neuromast lines (Figures 3C, D). The PRD class homeobox transcription factor gene Otx1 (18.7-fold lateral line-enriched; Modrell et al., 2017a) similarly showed much stronger expression in ampullary organs than in neuromasts in both paddlefish and sterlet (Figures 3E–H).
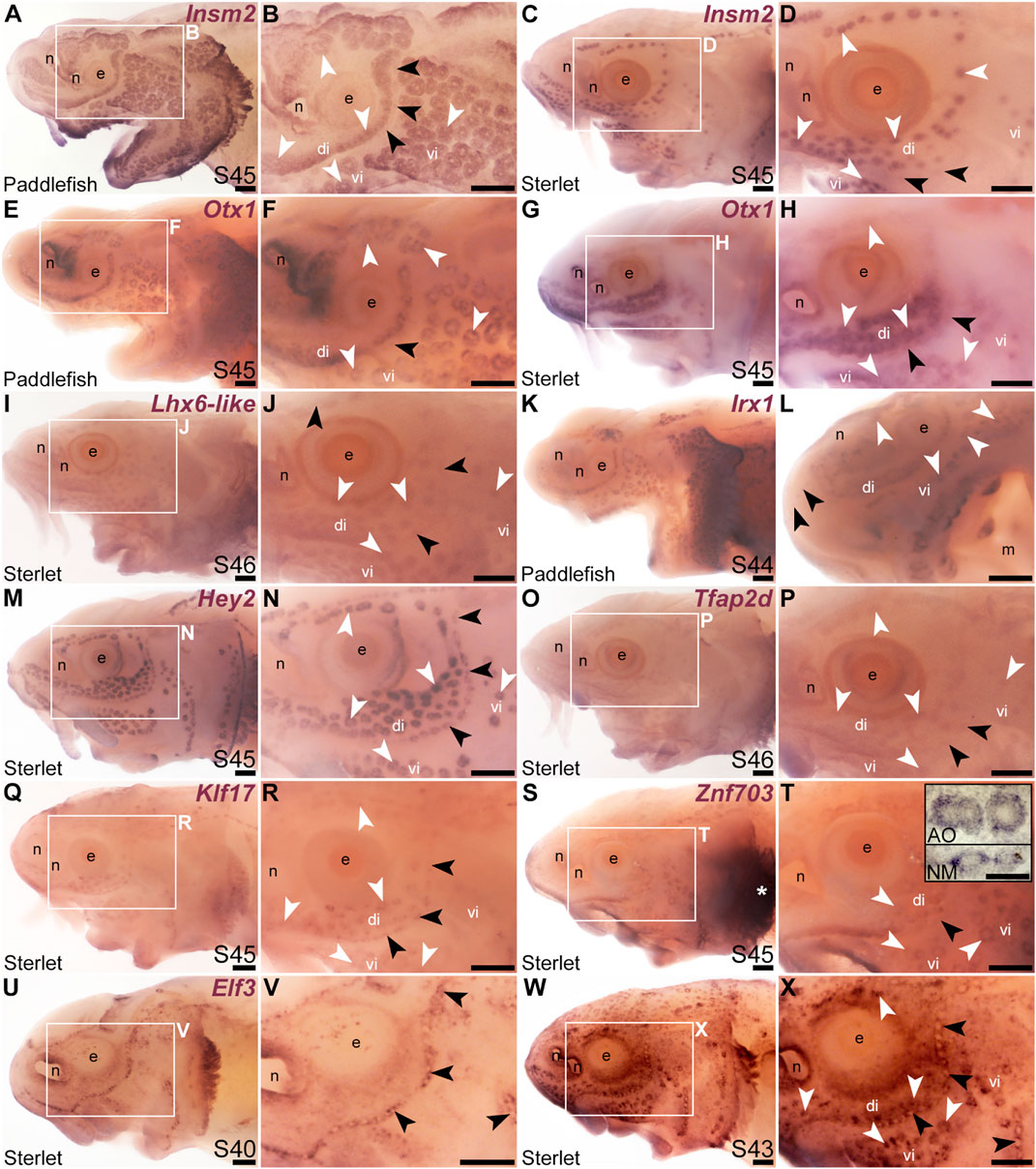
FIGURE 3. Other transcription factor genes expressed in ampullary organs and neuromasts. In situ hybridization in paddlefish or sterlet showing genes expressed in both ampullary organs (white arrowheads indicate examples) and neuromasts (black arrowheads indicate examples). Higher power views in each case are of the same embryo shown in the preceding panel. (Note: Paddlefish have many more ampullary organs than sterlet.) (A–D) Insm2 at stage 45 in paddlefish (A,B) and sterlet (C,D). Neuromast expression is only detectable in some parts of the neuromast lines and is noticeably weaker than in ampullary organs. (E–H) Otx1 at stage 45 in paddlefish (E,F) and sterlet (G,H). Neuromast expression is considerably weaker than ampullary organ expression (almost undetectable in paddlefish). (I,J) Sterlet Lhx6-like at stage 46. (K,L) Irx1 at stage 45 in paddlefish. Neuromast expression is weak and can be seen most clearly on the tip of the rostrum (black arrowheads in L). (M,N) Sterlet Hey2 at stage 45. (O,P) Sterlet Tfap2 at stage 45. (Q,R) Sterlet Klf17 at stage 45. (S,T) Sterlet Znf703 at stage 45. Expression in lateral line organs was often hard to detect in wholemount but expression in both neuromasts (NM) and ampullary organs (AO) was clear in skinmount (examples shown in inset in T). Strong expression is seen in gill filaments (white asterisk). (U–X) Sterlet Elf3. At stage 40 (U,V), Elf3 is expressed in a “ring” pattern in neuromasts, and in scattered cells in the skin. By stage 43 (W,X), Elf3 expression is also seen in ampullary organs and more broadly throughout the skin. Abbreviations: AO, ampullary organ; di, dorsal infraorbital ampullary organ field; e, eye; m, mouth; n, naris; NM, neuromast; S, stage; vi, ventral infraorbital ampullary organ field. Scale bars: 200 μm except for inset in T: 50 μm.
The LIM class homeobox transcription factor gene Lhx6 was also expressed in both ampullary organs and neuromasts (Figures 3I, J; originally unassigned locus 12855; 3.5-fold lateral line-enriched, Modrell et al., 2017a). The Lhx6-like riboprobe recognizes sequence from the 3′untranslated region of Lhx6-like mRNA, as annotated in the sterlet reference genome (NCBI RefSeq assembly GCF_902713425.1). Ampullary organs and neuromasts also expressed TALE class homeobox transcription factor gene Irx1 (Figures 3K, L; originally unassigned locus 111072; 8.3-fold lateral line-enriched, Modrell et al., 2017a). Vertebrate Iroquois-family transcription factor genes are found in clusters (IrxA: Irx1, Irx2, and Irx4; IrxB: Irx3, Irx5, and Irx6; Gómez-Skarmeta and Modolell, 2002) and the expression patterns of the IrxA-cluster genes Irx1 and Irx2 are identical in some mouse tissues (Houweling et al., 2001). Although Irx2 was not present in the late-larval paddlefish lateral line organ-enriched dataset (Modrell et al., 2017a), we cloned Irx2 and similarly saw expression in ampullary organs and neuromasts (Supplementary Figures S3A–D).
The Notch target and effector gene Hey2 (2.9-fold lateral line-enriched, Modrell et al., 2017b) was also expressed by both ampullary organs and neuromasts (Figures 3M, N). Three originally unassigned loci in the paddlefish lateral line organ-enriched dataset (Modrell et al., 2017a), all of whose closest UniProt matches had Pfam Hairy Orange and helix-loop-helix DNA-binding domains (locus 52662, 2.7-fold lateral line-enriched; locus 27975, 2.9-fold lateral line-enriched; locus 26264, 2.3-fold lateral line-enriched), proved to represent the related Notch target and effector gene Hes5. We had previously published the expression of Hes5 in both ampullary organs and neuromasts in a study on the role of Notch signalling in ampullary organ versus neuromast development in paddlefish (Modrell et al., 2017b).
Expression in developing ampullary organs and neuromasts was also seen for Tfap2d (Figures 3O, P; 6.0-fold lateral line-enriched, Modrell et al., 2017a). This gene encodes transcription factor AP-2 delta, which is a direct activator of Pou4f3 in retinal ganglion cell progenitors (Hesse et al., 2011; Li et al., 2016). The Krüppel-like transcription factor gene Klf17 (2.1-fold lateral line-enriched, originally annotated in our transcriptome as Klf4; Modrell et al., 2017a; also see Kotkamp et al., 2014) was also expressed in both types of lateral line organs (Figures 3Q, R), as was the zinc finger transcription factor gene Znf703 (2.3-fold lateral line-enriched, Modrell et al., 2017a), although neuromast expression was often at the limits of detection in wholemount (Figures 3S, T). However, Znf703 expression in neuromasts as well as ampullary organs was clear in skinmount (Figure 3T, inset). The E74-like Ets domain transcription factor gene Elf3 (2.1-fold lateral line-enriched, Modrell et al., 2017a) showed a “ring-like” expression pattern in both neuromasts and ampullary organs that was clearer prior to stage 45 as general expression gradually developed throughout the skin (Figures 3U–X).
Electrosensory-restricted cranial lateral line expression: Irx5, Irx3, Insm1, Sp5, Satb2, Mafa and Rorc
Our original analysis of candidates from the late-larval paddlefish lateral line organ-enriched dataset identified the bHLH gene Neurod4 as the first-reported transcription factor gene restricted within the paddlefish lateral line to developing ampullary organs (Modrell et al., 2017a). Here, we identified seven more transcription factor genes whose cranial lateral line expression is restricted to ampullary organs. The TALE class homeobox transcription factor gene Irx5 (1.9-fold lateral line-enriched in paddlefish, Modrell et al., 2017a) was expressed in ampullary organs but not neuromasts on the head (paddlefish: Figures 4A, B; sterlet: Figures 4C, D). However, Irx5 expression was seen in trunk neuromasts as well as the migrating posterior lateral line primordium (Figures 4E, F). The expression patterns of the IrxB-cluster genes Irx5 and Irx3 are very similar in both mouse and zebrafish (Houweling et al., 2001; Gómez-Skarmeta and Modolell, 2002; Lecaudey et al., 2005). Although Irx3 was not present in the late-larval paddlefish lateral line organ-enriched dataset (Modrell et al., 2017a), we cloned Irx3 and similarly saw expression in ampullary organs but not neuromasts on the head, and in the migrating posterior lateral line primordium and neuromasts on the trunk (Supplementary Figures S3E–J). Expression of the zinc-finger transcription factor gene Insm1 (3.1-fold lateral line-enriched, Modrell et al., 2017a) was similarly restricted to ampullary organs on the head (Figures 4G, H), but was seen in trunk neuromasts and migrating lateral line primordia (Figure 4I; compare with Sox2 immunostaining of trunk neuromasts and migrating lateral line primordia at the same stage, Figure 4J). (Insm1 was also expressed in cells scattered throughout the skin; these are likely to be Merkel cells, which were shown to express Insm1 in differential RNA-seq data from mouse; Hoffman et al., 2018.)
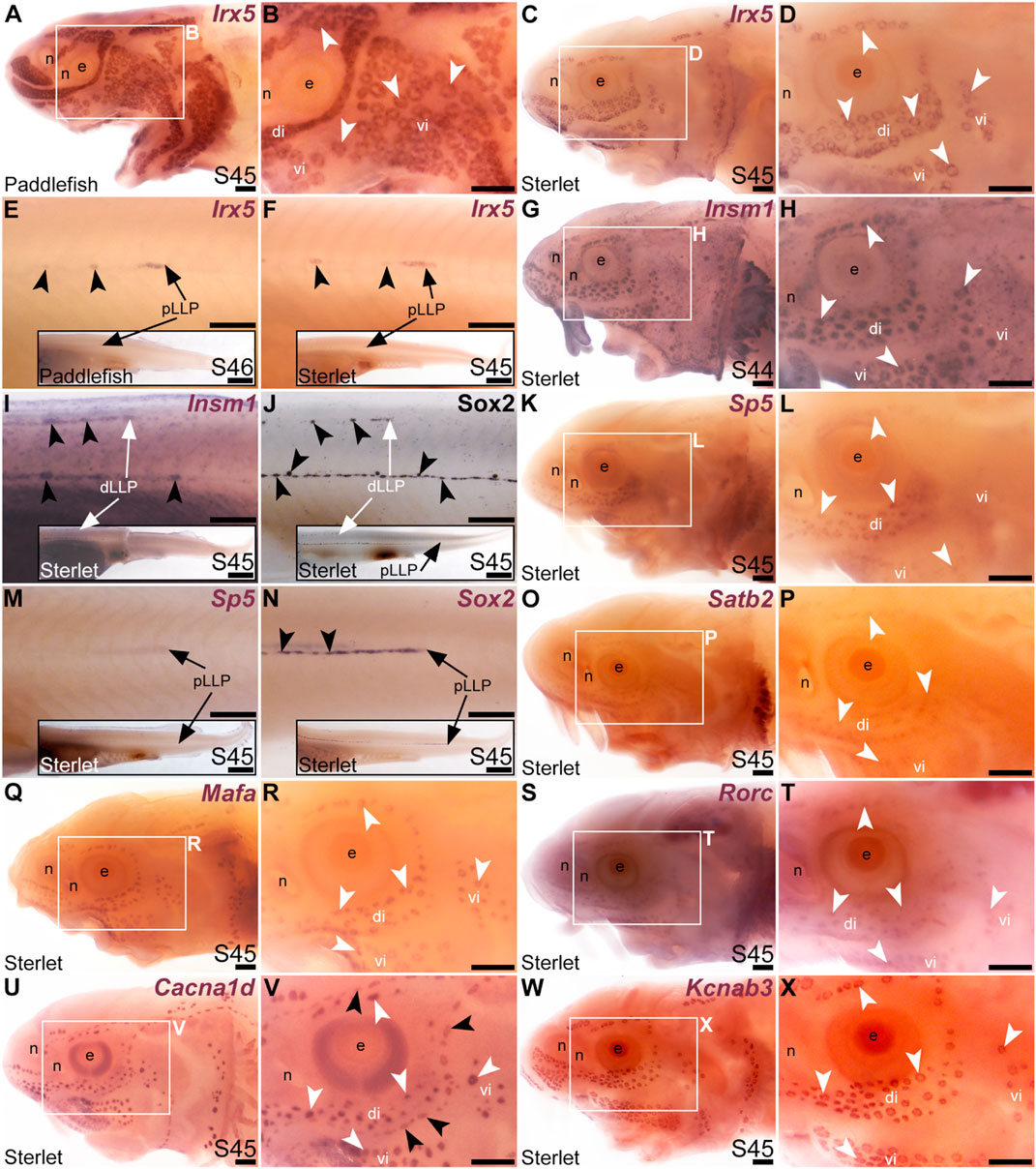
FIGURE 4. Transcription factor genes expressed in ampullary organs but not neuromasts on the head. In situ hybridization in paddlefish or sterlet. White arrow- heads indicate examples of ampullary organs; black arrowheads indicate examples of neuromasts. (A–F) Irx5 at stage 45–46 in paddlefish (A,B,E) and at stage 45 in sterlet (C,D,F). Expression is seen in ampullary organs but not neuromasts on the head (A–D); on the trunk, expression is also visible in developing neuromasts and the migrating posterior lateral line primordium [black arrows in (E,F)]. The insets in E,F show the position of the migrating primordia on the larval tail. (G–I) Insm1 in sterlet at stages 44–45. Cranial expression is detected in ampullary organs but not neuromasts (G,H); on the trunk, expression is also seen in developing neuromasts and the migrating posterior lateral line primordia (white arrow in I shows the dorsal trunk primordium). (Insm1 is also expressed in scattered cells throughout the skin, most likely Merkel cells.) (J) For comparison with (I): Sox2 immunostaining also labels developing neuromasts and the migrating posterior lateral line primordia (white arrow: dorsal trunk line primordium; black arrow: primary posterior lateral line primordium). The inset shows the position of the migrating primordia on the larval tail. (K–M) Sp5 at stage 45 in sterlet. Expression on the head is detected in ampullary organs but not neuromasts; on the trunk, weak expression is also visible in the migrating posterior lateral line primordium (arrow in K, different larva). The inset shows the position of the migrating primordium on the larval tail. (N) For comparison with panel M: Sox2 expression (using a paddlefish Sox2 riboprobe) is also seen in the migrating posterior lateral line primordium (arrow), as well as in developing neuromasts (black arrowheads). The inset shows the position of the migrating primordium on the larval tail. (O,P) Sterlet Satb2 at stage 45. (Q,R) Sterlet Mafa at stage 45. (S,T) Sterlet Rorc at stage 45. (U,V) Sterlet Cacna1d at stage 45 for comparison, showing differentiated hair cells in neuromasts and electroreceptors in ampullary organs. (W,X) Sterlet Kcnab3 at stage 45 for comparison, showing differentiated electroreceptors only. Abbreviations: di, dorsal infraorbital ampullary organ field; dLLP, dorsal trunk lateral line primordium; e, eye; n, naris; pLLP, posterior lateral line primordium; S, stage; vi, ventral infraorbital ampullary organ field. Scale bars: 200 μm except for insets in E,F,I,J,M,N: 1000 μm.
Another zinc-finger transcription factor gene, Sp5 (2.6-fold lateral line-enriched, Modrell et al., 2017a), was expressed in ampullary organs but not neuromasts (Figures 4K–M), although it was expressed in the migrating posterior lateral line primordium (Figure 4M: compare with Sox2 expression in developing neuromasts and the migrating primordium at the same stage, Figure 4N). Three other transcription factor genes were fully electrosensory-restricted: the CUT class (SATB subclass) homeobox transcription factor gene Satb2 (4.8-fold lateral line-enriched in paddlefish, Modrell et al., 2017a), although its expression was weak (Figures 4O, P); the bZIP transcription factor gene Mafa (2.6-fold lateral line-enriched, Modrell et al., 2017a; Figures 4Q, R) and a retinoic acid receptor (RAR)-related orphan nuclear receptor gene, Rorc (14.3-fold lateral line-enriched, Modrell et al., 2017a; Figures 4S, T). For comparison with the ampullary organ-restricted cranial expression of the above-listed transcription factor genes, Figures 4U, V show Cacna1d expression in hair cells and electroreceptors, while Figures 4W, X show electroreceptor-specific Kcnab3 expression.
Sox8 is restricted to the mechanosensory lateral line in bony fishes but not in cartilaginous fishes
The paddlefish lateral line organ-enriched gene-set included a single SoxE-class high-mobility group (HMG)-box transcription factor gene, Sox10 (3.9-fold lateral line-enriched, Modrell et al., 2017a). Comparison with the hair cell and electroreceptor marker Cacna1d (Figures 5A, B) and the supporting cell marker Sox2 (Figures 5C, D) shows that paddlefish Sox10 was not expressed within neuromasts or ampullary organs, but instead along nerves (Figures 5E, F). Sox10 expression would be expected in nerve-associated Schwann cells, as these neural crest-derived glial cells express Sox10 throughout their development and into the adult (see, e.g., Jessen and Mirsky, 2019) and Sox10 is required for Schwann cell differentiation in zebrafish, including on lateral line nerves (Kelsh and Eisen, 2000; Grant et al., 2005; López-Schier and Hudspeth, 2005).
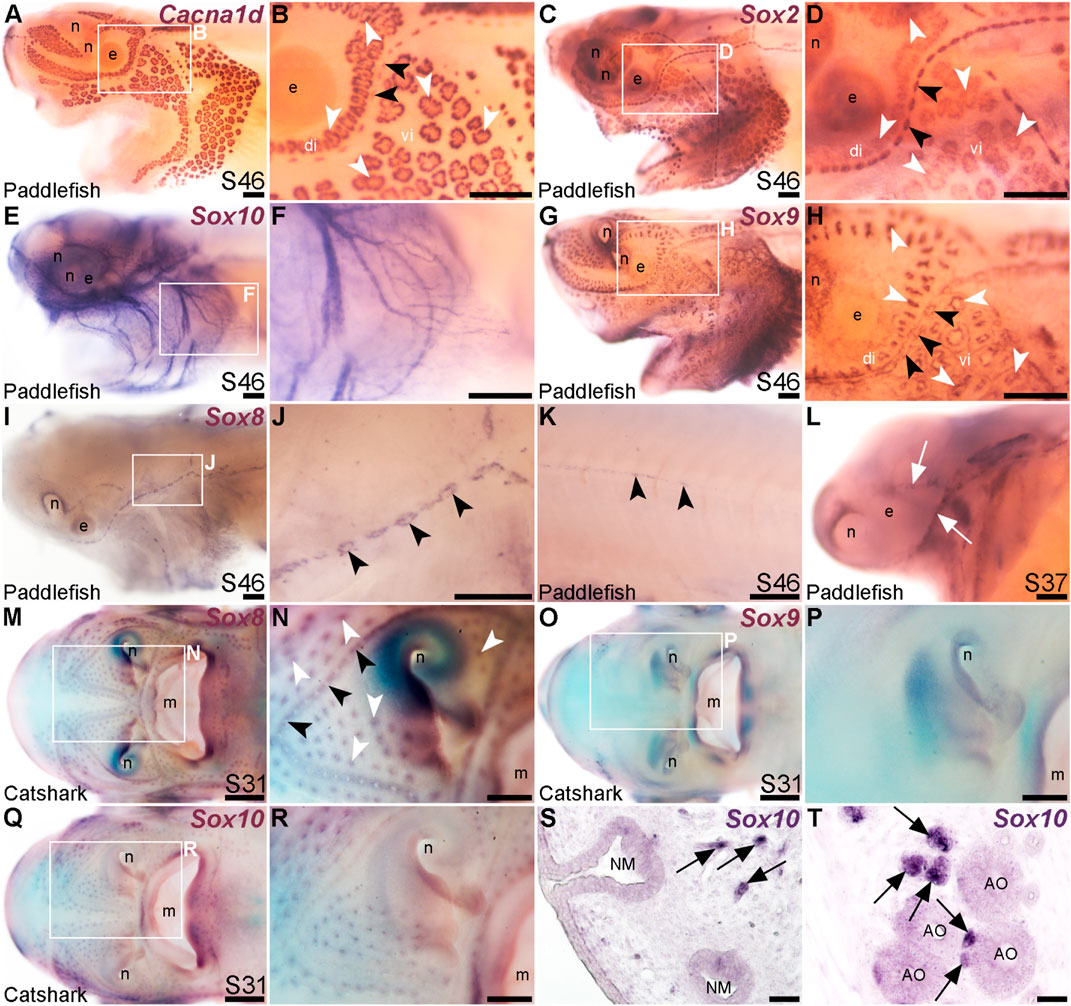
FIGURE 5. Lateral line expression of SoxE genes differs between chondrostean ray-finned bony fishes and cartilaginous fishes. In situ hybridization in late-larval paddlefish or catshark (S. canicula). White arrowheads indicate examples of ampullary organs; black arrowheads indicate examples of neuromasts. (A,B) For comparison, paddlefish Cacna1d expression at stage 46 reveals differentiated hair cells in neuromasts and electroreceptors in ampullary organs. (Note: The image in panel A was previously published in a review; Baker and Modrell, 2018.) (C,D) For comparison, paddlefish Sox2 expression at stage 46 identifies support cells in neuromasts and ampullary organs. (E,F) Paddlefish Sox10 expression at stage 46 is associated with cranial nerves, not in lateral line organs (compare with Cacna1d expression in panel A). (G,H) Paddlefish Sox9 at stage 46 is expressed in a “ring”-like pattern in neuromasts and ampullary organs [compare with panels (A–D), especially in the ventral infraorbital ampullary organ field]. (I–K) Paddlefish Sox8 expression at stage 46 is seen in a ring pattern in neuromasts only [compare panel J with panels (B,D)], including in neuromasts developing on the trunk (K). (L) At stage 37, paddlefish Sox8 is expressed in sensory ridges (white arrows). (M,N) Catshark Sox8 at stage 31 is expressed in both neuromasts and ampullary organs. (O,P) Catshark Sox9 expression at stage 31 is not seen in lateral line organs (compare with Sox8 in panels O,P). (Q–T) Catshark Sox10 expression at stage 31 seems to be in or near individual lateral line organs in wholemount (Q,R). However, in situ hybridization on sections shows that Sox10 is strongly expressed in cells (arrows) adjacent to neuromasts (S) and ampullary organs (T) that are likely associated with nerves. Above-background Sox10 expression is not seen in the lateral line organs themselves. Abbreviations: di, dorsal infraorbital ampullary organ field; e, eye; m, mouth; n, naris; S, stage; vi, ventral infraorbital ampullary organ field. Scale bars: A-L,N,P,R, 200 μm; M,O,Q, 500 μm; S,T, 10 μm.
Another SoxE-class HMG-box transcription factor gene, Sox8, was previously reported to be expressed in developing ampullary organs in a catshark, Scyliorhinus canicula (Freitas et al., 2006). Given this, we also cloned Sox8 and the remaining SoxE class gene, Sox9, to test their expression in paddlefish. Sox9 was expressed at late-larval stages in both neuromasts and ampullary organs with a “ring-like” distribution (Figures 5G, H). This is also consistent with Sox9 expression in the developing mouse inner ear, where initial broad expression becomes restricted to supporting cells, co-expressed with Sox2 (Mak et al., 2009; Jan et al., 2021).
Paddlefish Sox8 expression, in contrast to Sox9, was restricted to neuromasts at late-larval stages (Figures 5I, J), also in a ring pattern suggestive of supporting cells rather than centrally clustered hair cells (compare neuromast expression of Sox8 in Figure 5J with Cacna1d in hair cells in Figure 5B and Sox2 expression in supporting cells in Figure 5D). Sox8 was also expressed in neuromasts on the trunk (Figure 5K) and, at earlier stages, in the central region of sensory ridges where neuromasts form (Figure 5L).
The mechanosensory lateral line-restricted expression of paddlefish Sox8 contrasts with the reported expression of Sox8 in catshark ampullary organs (Freitas et al., 2006). To test this further, we cloned all three SoxE genes from the lesser-spotted catshark (Scyliorhinus canicula). We confirmed that Sox8 is expressed in catshark ampullary organs, as previously reported (Freitas et al., 2006), as well as in neuromasts (Figures 5M, N), unlike the neuromast-specific Sox8 expression seen in late-larval paddlefish (Figures 5K, L). Sox9 was not expressed in catshark lateral line organs at all (Figures 5O, P), in striking contrast to paddlefish Sox9 expression in both neuromasts and ampullary organs (Figures 5G, H). The only conserved SoxE lateral line expression pattern between catshark and paddlefish was that of Sox10, which ISH on sections confirmed to be restricted to axon-associated Schwann cells (Figures 5Q–T). Overall, these data reveal lineage-specific differences in SoxE transcription factor gene expression within late-larval lateral line organs in a ray-finned chondrostean fish versus a cartilaginous fish.
Foxg1 is restricted to the mechanosensory lateral line
The winged-helix transcription factor gene Foxg1 was 11.4-fold enriched in late-larval (stage 46) paddlefish operculum vs. fin (Modrell et al., 2017a). Foxg1 proved to be restricted to the mechanosensory lateral line in ray-finned chondrostean fishes. For comparison, Figures 6A–D show Sox2 protein expression in supporting cells in neuromasts and (more weakly) in ampullary organs in late-larval paddlefish (Figures 6A, B) and sterlet (Figures 6C, D). Sox2 immunostaining also labels taste buds, and individual cells scattered throughout the skin (most likely Merkel cells, which express Sox2 in zebrafish, as well as mouse; Brown et al., 2023; Bardot et al., 2013; Lesko et al., 2013; Perdigoto et al., 2014). Paddlefish Foxg1 expression in the lateral line system at stage 45 (Figures 6E, F) was restricted to neuromast lines, but excluded from the central domain of individual neuromasts where hair cells are found (compare Figure 6F with Sox2 in Figure 6B). (Foxg1 expression was also seen in the olfactory system, as expected; e.g., Kawauchi et al., 2009.) Paddlefish Foxg1 was expressed in the migrating posterior lateral line primordium on the trunk, and in trunk neuromasts deposited by the primordium (Figure 6G; compare with Sox2 immunostaining at the same stage, Figure 6H). Sterlet Foxg1 was expressed in the same pattern at stage 45 as in paddlefish (Figures 6I–K; Figure 6L shows Sox2 immunostaining in the sterlet posterior lateral line primordium and trunk neuromasts for comparison with sterlet Foxg1 expression in Figure 6K). Analysis at earlier stages in sterlet showed that Foxg1 expression was restricted to the central zone of sensory ridges where neuromasts form (Figures 6M–P). Thus, Foxg1 expression in the developing lateral line system is restricted to the mechanosensory division, although it seems to be excluded from differentiated hair cells.
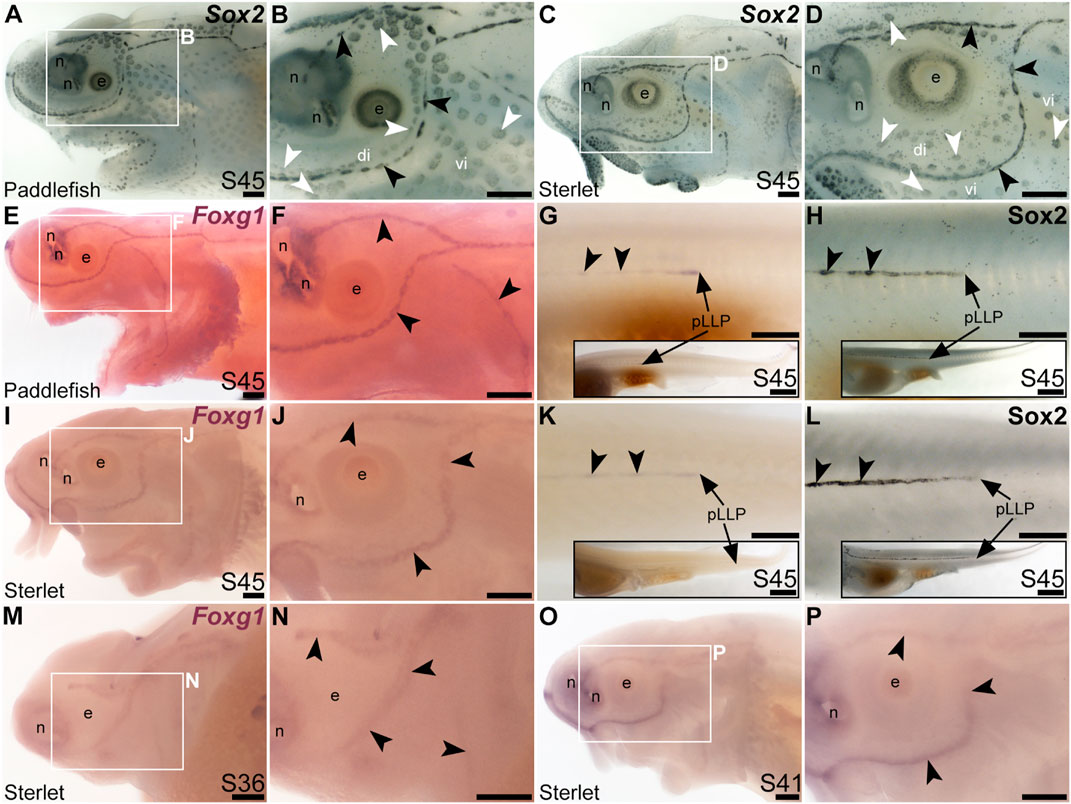
FIGURE 6. Foxg1 is mechanosensory-restricted within the developing lateral line system. Black arrowheads indicate examples of neuromasts; white arrowheads indicate examples of ampullary organs. (A–D) For comparison, Sox2 immunostaining at stage 45 in paddlefish (A,B) and sterlet (C,D) shows support cells in neuromasts (stronger staining) and ampullary organs (weaker staining). (Strong Sox2 expression is also seen in taste buds on the barbels and around the mouth, and in scattered cells in the skin, most likely Merkel cells.) (E–G) In situ hybridization for Foxg1 at stage 45 in paddlefish, showing a ring-like expression pattern in the neuromast lines (compare panel F with paddlefish Sox2 in B), as well as expression in the migrating posterior lateral line primordium (arrow in G, different larva) and developing trunk neuromasts (black arrowheads). Expression is also seen in the nares. The inset in G shows the position of the migrating primordium on the larval tail. (H) For comparison with G, Sox2 immunostaining on the trunk at stage 45 in paddlefish shows the migrating posterior lateral line primordium (arrow) and developing neuromasts. The inset shows the position of the migrating primordium on the larval tail. (I–K) In situ hybridization for Foxg1 at stage 45 in sterlet similarly shows a ring pattern in neuromasts (compare panel J with sterlet Sox2 in D), as well as expression in the migrating posterior lateral line primordium (arrow in K, different larva) and developing trunk neuromasts (black arrowheads). Expression is also seen in the nares. The inset in K shows the position of the migrating primordium on the larval tail. (L) For comparison with K, Sox2 immunostaining on the trunk at stage 45 in sterlet shows weak expression in the migrating posterior lateral line primordium (arrow) and developing neuromasts. The inset shows the position of the migrating primordium on the larval tail. (M–P) In situ hybridization for sterlet Foxg1 at stage 36 (M,N) and stage 42 (O,P) shows ring-like expression already in developing neuromasts in sensory ridges, and no expression in developing ampullary organ fields (compare with sterlet Eya4 expression at stages 35 and 41 in Figures 1A, C). Abbreviations: di, dorsal infraorbital ampullary organ field; e, eye; n, naris; pLLP, posterior lateral line primordium; S, stage; vi, ventral infraorbital ampullary organ field. Scale bars 200 μm except for insets in G,H,K,L: 1000 μm.
Mechanosensory-restricted lateral line expression of Hmx2, Isl1 and Rorb
The NKL class homeobox transcription factor gene Hmx2 (also known as Nkx5-2), which was 5.8-fold lateral line-enriched in late-larval paddlefish (Modrell et al., 2017a), also proved to be restricted to the mechanosensory lateral line. For comparison, Figures 7A, B show Sox2 immunostaining at stage 45. At this stage, Hmx2 was expressed in a ring-like pattern at the outer edge of developing neuromasts (Figures 7C, D; compare with Sox2 in Figures 7A, B). However, no expression was seen in ampullary organs (Figures 7C, D), confirmed in skinmounts after post-ISH Sox2 immunostaining to identify ampullary organs (Figure 7E). As an aside, Hmx2 expression was also seen in scattered skin cells (Figures 7C–E). Hmx2 was not among the genes reported in a differential RNA sequencing study of adult mouse Merkel cells (Hoffman et al., 2018). However, examination of sterlet skinmounts after post-ISH Sox2 immunostaining suggested the Hmx2-expressing skin cells may co-express Sox2 (Figure 7E), which would support their being Merkel cells. Alternatively, other scRNA-seq studies in mouse and human have shown that Hmx2 is expressed by tuft (brush) cells in gut and airway epithelia (Haber et al., 2017; Deprez et al., 2020), so it is possible the Hmx2-expressing skin cells in paddlefish are chemosensory epithelial cells, like tuft cells (Kotas et al., 2023).
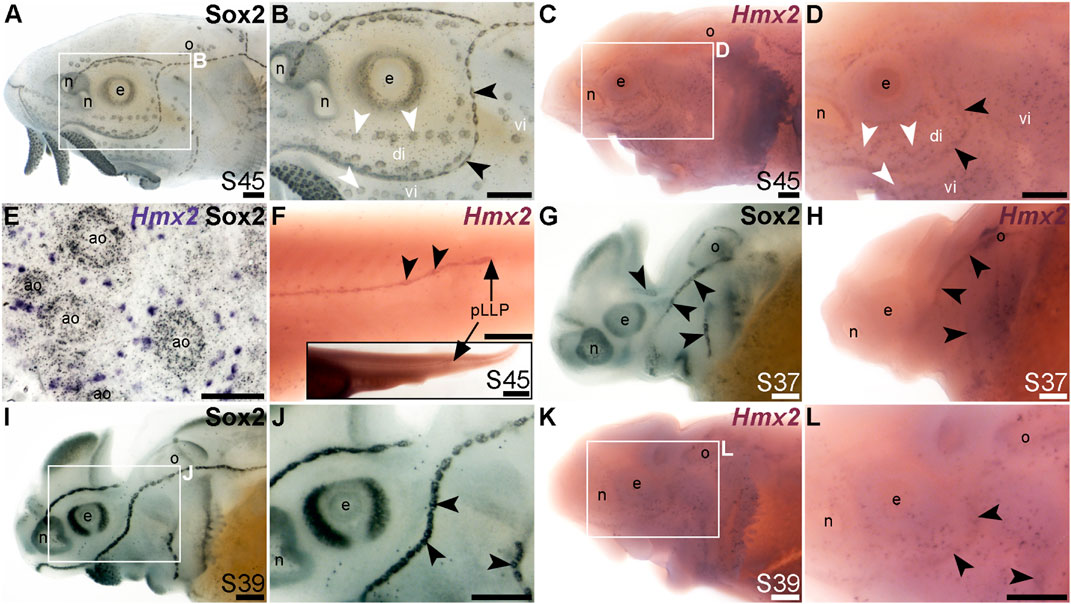
FIGURE 7. Hmx2 is mechanosensory-restricted within the developing lateral line system. Black arrowheads indicate examples of neuromasts; white arrowheads indicate examples of ampullary organs. (A,B) For comparison, Sox2 immunostaining in sterlet is shown at stage 45. Sox2 labels developing neuromasts (stronger staining) and scattered cells in the skin, most likely Merkel cells, as well as taste buds on the barbels and around the mouth. Expression is also seen in ampullary organs (weaker than in neuromasts). A patch of Sox2 expression at the spiracular opening (first gill cleft) may represent the spiracular organ. (C–F) In situ hybridization for sterlet Hmx2 at stage 45 in wholemount (C,D), Hmx2 is weakly expressed in a ring-like pattern in the neuromast lines, as well as in scattered cells in the skin, but appears to be absent from ampullary organs (compare D with Sox2 expression in B). A skinmount (E) with several ampullary organs from a stage 45 embryo revealed by post-ISH immunostaining for Sox2 (black metallographic deposits) confirms that ampullary organs do not express Hmx2 (purple). (The scattered Hmx2-positive skin cells may co-express Sox2, suggesting they are likely to be Merkel cells.) Hmx2 is also expressed in the trunk neuromast line (F), including the migrating posterior lateral line primordium (black arrow in J). The inset shows the position of the migrating primordium on the larval tail. (G) For comparison with H, Sox2 immunostaining at stage 37 labels developing neuromasts. Expression is also seen in the nasal capsule and otic vesicle, as well as eye. (H) Hmx2 expression at stage 37 is detected in neuromast lines, as well as in the otic vesicle. (I,J) For comparison with K and L, Sox2 immunostaining at stage 39 labels developing neuromasts and scattered cells in the skin, most likely Merkel cells, as well as taste buds on the barbels. Expression is also seen in the nasal capsule and the eye. (K,L) Hmx2 expression at stage 39 is detected in neuromast lines and scattered cells in the skin. Abbreviations: ao, ampullary organ; di, dorsal infraorbital ampullary organ field; e, eye; n, naris; o, otic vesicle; pLLP, posterior lateral line primordium; S, stage; vi, ventral infraorbital ampullary organ field. Scale bars: 200 μm except for (E): 50 μm and inset in F, 1000 μm.
Hmx2 was also expressed in the migrating posterior lateral line primordium at stage 45 (Figure 7F), like Foxg1 (Figure 6K) and Sox2 (Figure 6L). Analysis at earlier stages, with Sox2 immunostaining for comparison (Figures 7G–L), showed that Hmx2 was weakly expressed in neuromast lines (and in the otic vesicle) at stage 37 (Figure 7H; compare with Sox2 in Figure 7G) and at stage 39 (Figures 7K, L; compare with Sox2 in Figures 7I, J).
The LIM class homeobox transcription factor Isl1 was recently reported to promote a more complete conversion by Atoh1 of mouse cochlear supporting cells to hair cells than does Atoh1 alone (Yamashita et al., 2018). In the mouse inner ear, Isl1 is expressed in sensory patches but downregulated as hair cells differentiate (Radde-Gallwitz et al., 2004; Huang et al., 2008). The only LIM homeobox genes in the paddlefish lateral line organ-enriched dataset (Modrell et al., 2017a) are Lhx3, which we previously reported to be expressed in ampullary organs as well as neuromasts (Modrell et al., 2017a); Lhx6-like (originally unassigned locus 12855), with the same expression pattern (Figure 3K), and Lhx8, which proved to be expressed in gill filaments, not lateral line organs (Supplementary Figure S4J). Nevertheless, given the demonstrated role for Isl1 in promoting cochlear hair cell formation (Yamashita et al., 2018), we cloned sterlet Isl1. For comparison, Figures 8A, B show Sox2 immunostaining at stage 45, and Figures 8C, D show Cacna1d-expressing hair cells and electroreceptors at stage 45. At this stage, Isl1 expression was weak, but restricted within the lateral line system to neuromasts (Figures 8E, F). Examination at earlier stages revealed no detectable expression at stage 36 (Figures 8G, H) and neuromast-restricted expression at stages 39 and 40, when Isl1 seemed to be more strongly expressed than at stage 45 (Figures 8I–L). The weaker expression at later stages is consistent with the downregulation of Isl1 expression in differentiating hair cells in the mouse inner ear (Radde-Gallwitz et al., 2004; Huang et al., 2008).
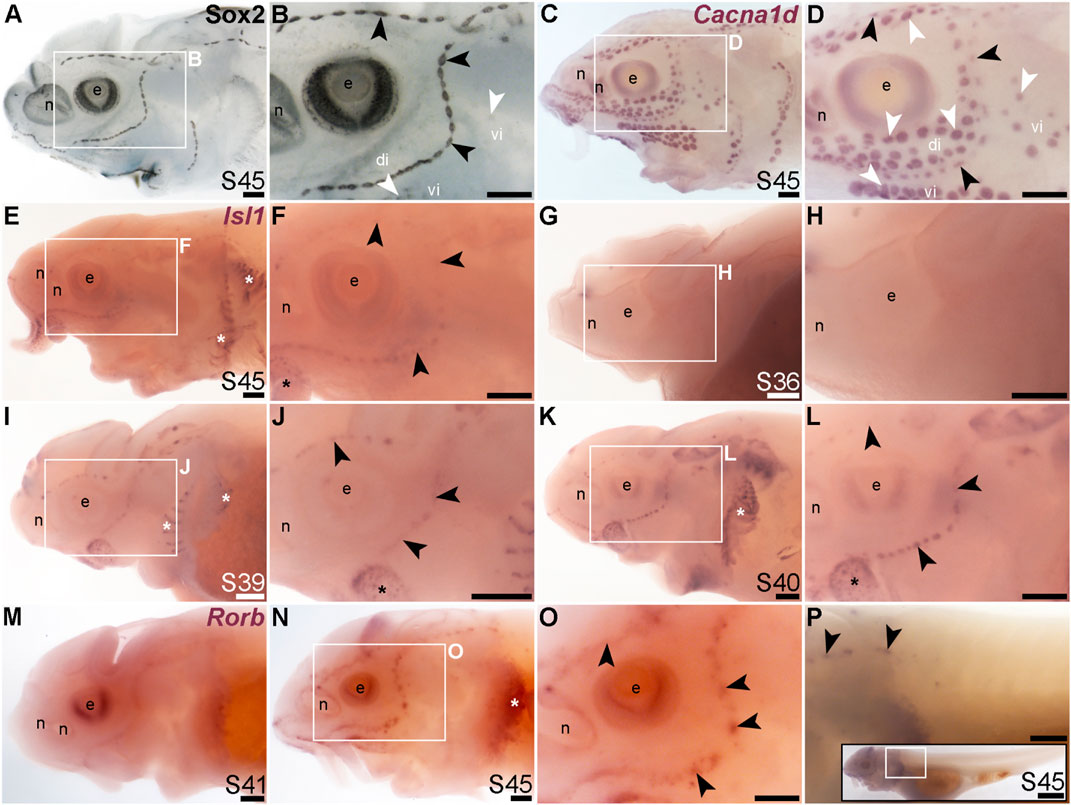
FIGURE 8. Isl1 and Rorb are mechanosensory-restricted within the developing lateral line system. Black arrowheads indicate examples of neuromasts; white arrowheads indicate examples of ampullary organs. (A,B) For comparison, Sox2 immunostaining in sterlet is shown at stage 45. The colour reaction for this larva was stopped early, highlighting the mechanosensory system more prominently. Sox2 labels developing neuromasts (stronger staining) and scattered cells in the skin, most likely Merkel cells, as well as taste buds on the barbels and around the mouth. Expression is also seen in ampullary organs (weaker than in neuromasts). A patch of Sox2 expression at the spiracular opening (first gill cleft) may represent the spiracular organ. (C,D) For comparison, in situ hybridization for sterlet Cacna1d at stage 45 shows expression in hair cells in neuromasts and electroreceptors in ampullary organs (and weak expression in taste buds on the barbels). (E,F) In situ hybridization for sterlet Isl1 at stage 45 shows weak spots of expression in neuromasts but not ampullary organs (compare with Cacna1d in C,D). Stronger expression is seen in taste buds on the barbels (black asterisk) and in gill filaments (white asterisk). (G–L) In situ hybridization for sterlet Isl1 at earlier stages shows no expression at stage 36 (G,H), and expression in neuromasts (but not ampullary organs) at stage 39 (I,J), and stage 40 (K,L). From stage 39, Isl1 expression is also seen in taste buds on the barbels (asterisk in J) and in gill filaments. (M–P) In situ hybridization for sterlet Rorb at stage 41 (M), and stage 45 (N–P) shows cranial neuromast-specific expression within the lateral line (i.e., without expression in either trunk neuromasts or the migrating posterior lateral line primordium). Abbreviations: di, dorsal infraorbital ampullary organ field; e, eye; n, naris; pLLP, posterior lateral line primordium; S, stage; vi, ventral infraorbital ampullary organ field. Scale bar: 200 μm.
Finally, we identified the RAR-related orphan nuclear receptor beta gene, Rorb (6.2-fold lateral line-enriched; Modrell et al., 2017a), as being restricted to cranial neuromasts, with no detectable expression in ampullary organs or trunk neuromasts (Figures 8M–P). The onset of Rorb expression in neuromasts was later even than Isl1, starting only at stage 41 (Figures 8M, N). It was intriguing to see the mutually exclusive expression of Rorb in cranial neuromasts (Figures 8O, P) and Rorc in ampullary organs (Figures 4O, P).
Taken together, we have identified Sox8, Foxg1, Hmx2, Isl1 and Rorb as the first-reported transcription factor genes restricted to the mechanosensory division of the lateral line system in ray-finned fishes. Sox8 and Foxg1 are expressed in the central zone of sensory ridges where neuromasts form and maintained in neuromasts, though apparently excluded from differentiated hair cells. Hmx2 is expressed in sensory ridges and retained in neuromasts, whereas Isl1 and Rorb are restricted to neuromasts (specifically cranial neuromasts, for Rorb) as early as they can be detected.
The remaining transcription factor genes from the paddlefish lateral line organ-enriched gene-set that we examined proved not to be expressed in lateral line organs, but instead, e.g., in ectoderm around ampullary organs (Supplementary Figures S4A–D: Ehf, Foxi2 and Nkx2-3), or at the edge of the operculum, in taste buds and/or in developing gill filaments (Supplementary Figures S4E–P: Foxe1, Foxl2, Gcm2, Hoxa2, Lhx8, Pou3f4, Sim2, Tbx1, Tlx1, Tlx2 and Rax2).
Discussion
In this study, we used our paddlefish lateral line organ-enriched gene-set (generated from differential bulk RNA-seq at late larval stages; Modrell et al., 2017a), together with a candidate gene approach, to identify 25 novel transcription factor genes expressed in developing lateral line organs in sterlet and/or paddlefish. These data, together with our previous work in paddlefish (Modrell et al., 2011a; Modrell et al., 2011b; Modrell et al., 2017a; Modrell et al., 2017b), suggest extensive conservation of molecular mechanisms involved in electrosensory and mechanosensory lateral line organ development. However, they also reveal a set of transcription factor genes with restricted expression that may be involved in the development of mechanosensory versus electrosensory organs. Of the 42 transcription factor genes with validated expression during lateral line organ development in paddlefish and/or sterlet, 29 (69%) were expressed in both ampullary organs and neuromasts (Table 1). These include the key “hair cell” transcription factor genes Six1, Eya1, Sox2, Atoh1, Pou4f3 and Gfi1 (see Sun and Liu, 2023; Zine and Fritzsch, 2023). We also identified eight electrosensory-restricted and five mechanosensory-restricted transcription factor genes (Table 1), as discussed further below.
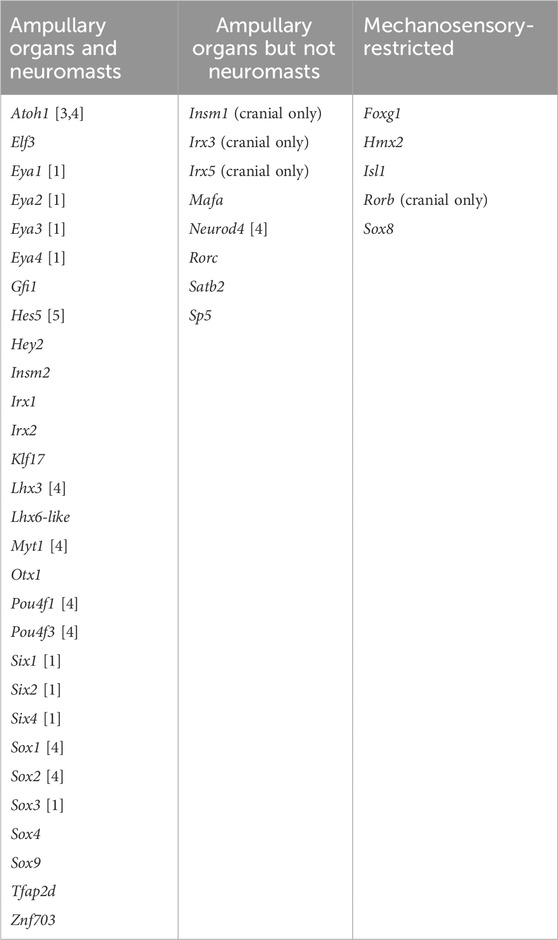
TABLE 1. Transcription factor genes expressed in developing lateral line organs in paddlefish and/or sterlet. Lateral line expression was reported either in this study or in previous papers, denoted by numbers in brackets: [1] Modrell et al. (2011b); [2] Modrell et al. (2011a); [3] Butts et al. (2014); [4] Modrell et al. (2017a); [5] Modrell et al. (2017b).
While this work was ongoing, a differential RNA-seq study of regenerating ampullary organs and neuromasts in the Siberian sturgeon (Acipenser baerii) was published (Wang et al., 2020). This study compared dissected tissue samples containing stage 45 ampullary organs or neuromasts relative to general epidermis, which identified 2074 lateral line organ-enriched genes, of which 1418 were shared by ampullary organs and neuromasts; 539 were ampullary organ-enriched, and 117 were neuromast-enriched (Wang et al., 2020). The “common” stage 45 lateral line-organ dataset from the Siberian sturgeon (Wang et al., 2020) included many of the candidate genes encoding transcription factors and differentiation markers whose expression we have validated in both ampullary organs and neuromasts at stage 45–46 in paddlefish and/or sterlet, e.g., Six1, Eya1, Atoh1, Pou4f3, Gfi1, Otof, and Cacna1d (Modrell et al., 2011b; Modrell et al., 2011a; Modrell et al., 2017a; this study). The ampullary organ-enriched dataset from the Siberian sturgeon (Wang et al., 2020) included Sp5, which we identified here in sterlet as ampullary organ-restricted, but also Hmx2 and Rorb, which we identified here as neuromast-restricted. Conversely, the neuromast-enriched dataset (Wang et al., 2020) included Insm1, which we found in sterlet to be ampullary organ-restricted on the head (though expressed in trunk neuromasts). Furthermore, the “common” dataset (Wang et al., 2020) included genes whose expression in sterlet and paddlefish was either ampullary organ-specific (e.g., the voltage-gated K+ channel genes Kcna5 and Kcnab3, as well as Neurod4; this study; Modrell et al., 2017a), or mechanosensory-specific (e.g., Foxg1 and Isl1; this study).
Overall, we think that the stage 45 Siberian sturgeon tissue dissections (Wang et al., 2020) were unable to separate ampullary organs and neuromasts completely. Like our own stage 46 paddlefish lateral line organ-enriched dataset (Modrell et al., 2017a), the stage 45 Siberian sturgeon datasets are not exhaustive (Wang et al., 2020): some of the genes whose expression we have validated in stage 45–46 sterlet and/or paddlefish lateral line organs were missing (e.g., Satb2, Sox2 and Sox8; this study; Modrell et al., 2017a). Nevertheless, this differential RNA-seq study in late-larval Siberian sturgeon embryos (Wang et al., 2020) provides an invaluable, independent resource from which to identify additional candidate genes for future validation and functional investigation in vivo.
Conserved molecular mechanisms are likely involved in the formation of hair cells and electroreceptors
In this study, we identified 13 novel transcription factor genes expressed in both types of lateral line organs in chondrostean ray-finned fishes, consistent with conservation of molecular mechanisms. In particular, we highlight Gfi1 (see Sun and Liu, 2023; McGovern et al., 2024) as being another key “hair cell” transcription factor gene expressed in developing ampullary organs as well as neuromasts, together with Atoh1, Pou4f3 and Six1 (Modrell et al., 2011a; Modrell et al., 2017a). Gfi1-deficient hair cells fail to mature and also upregulate neuronal differentiation genes such as Neurod1 and Pou4f1 (and Insm1, which is important for otic neurogenesis as well as outer hair cell formation; Lorenzen et al., 2015), suggesting that a key function of Gfi1 in hair cells is to repress neuronal genes that are initially also expressed in hair cell progenitors (Matern et al., 2020). Gfi1 also acts indirectly to increase Atoh1 transcriptional activity by forming part of a transcriptional complex with Atoh1 and E proteins in which neither Atoh1 nor Gfi1 binds the other directly and Gfi1 does not bind DNA (Jen et al., 2022). Given the shared expression in ampullary organs and neuromasts, it seems likely that Gfi1 plays these roles in both developing electroreceptors and hair cells. Intriguingly, however, Insm2 was recently reported as a direct target of both Atoh1 and Gfi1 in mouse cochlear hair cells, and one of only a handful of genes (including Atoh1 itself) to be repressed by Gfi1 during hair-cell maturation (Jen et al., 2022). Repression of Insm2 by Gfi1 in mature hair cells, but not electroreceptors, could explain the much weaker expression of Insm2 that we saw in neuromasts versus ampullary organs at stage 45 (the onset of independent feeding). This suggests the existence of both shared and divergent functions of the same transcription factor within hair cells versus electroreceptors.
Seven novel transcription factor genes with ampullary organ-restricted cranial expression
We have identified seven novel transcription factor genes expressed in developing ampullary organs but not cranial neuromasts in chondrostean ray-finned fishes, in addition to previously published Neurod4 (Modrell et al., 2017a). Three of these, Irx5, Irx3 and Satb2, encode homeodomain transcription factors. In C. elegans, unique combinations of homeodomain transcription factors define all 118 neuron classes (Reilly et al., 2020) (also see Vidal et al., 2022). Hence, members of this class of transcription factors are potentially good candidates to be involved in controlling divergent fate specification and/or maintenance in closely related cell types.
Irx5 is required for the terminal differentiation of a subset of cone bipolar cells in the mouse retina (Cheng et al., 2005). In mouse and chicken, Irx5 and Irx3 are expressed within the otic vesicle epithelium, including some prospective sensory patches (Bosse et al., 2000; Cardeña-Núñez et al., 2016). However, by stage 34 in chicken (embryonic day 8), when hair cells are fully differentiated, Irx5 is not expressed in any sensory patch, unlike some other Irx family members (for example, Irx2 is expressed in all sensory patches; Irx1 and Irx3 are expressed in subsets) (Cardeña-Núñez et al., 2016). In mouse, chicken and zebrafish, Irx5 (together with other Irx family members) is expressed in otic placode-derived neurons (Bosse et al., 2000; Houweling et al., 2001; Lecaudey et al., 2005; Cardeña-Núñez et al., 2016). In zebrafish, the only reported expression of Irx5 or Irx3 genes in the lateral line system is that of Irx5a in the secondary posterior lateral line primordium (prim II) (Lecaudey et al., 2005). This migrates later than the primary posterior lateral line primordium and contributes post-embryonically to the trunk lateral line (Sapède et al., 2002). However, the function of Irx5a in the primordium is not known (Lecaudey et al., 2005). Furthermore, the lack of reported expression in zebrafish neuromasts contrasts with Irx5 expression in developing trunk (but not cranial) neuromasts in paddlefish and sterlet, suggesting lineage-specific differences.
Ampullary organ-restricted Satb2 encodes a homeodomain transcription factor and chromatin remodeller that is important for craniofacial development, including osteoblast differentiation (reviewed by Huang et al., 2022). Its expression has not been reported in the inner ear or lateral line system. The Satb2 gene is directly bound by Smad1/5 and upregulated following over-expression of Bmp4 in cranial neural crest cells, suggesting that Satb2 is a direct target of the Bmp signalling pathway (Bonilla-Claudio et al., 2012). This raises the possibility that Bmp signalling may be important for ampullary organ development. Indeed, Bmp4, Bmp5, Brinp3 (encoding BMP/retinoic acid-inducible neural-specific protein 3) and Bambi (encoding a Bmp/activin inhibitor) are present in the “common” lateral line organ-enriched gene-set from stage 45 Siberian sturgeon (Wang et al., 2020). Brinp3 is also in the ampullary organ-enriched gene-set (Wang et al., 2020). Our stage 46 paddlefish lateral line organ-enriched gene-set also includes Bmp5, together with genes encoding the dual Bmp/Wnt inhibitors Sostdc1 and Apcdd1 (Modrell et al., 2017a). Thus, the Bmp pathway is a promising target for studies of ampullary organ development.
The other electrosensory-restricted transcription factor genes on the head were Insm1, Mafa, Rorc and Sp5. In zebrafish, Insm1a is expressed in the migrating posterior lateral line primordium and neuromasts on the trunk (cranial lateral line expression was not reported), and morphants showed defects in primordium migration, proliferation and neuromast formation (He et al., 2017). In the inner ear, transient expression of Insm1 in developing outer hair cells prevents them from transdifferentiating into inner hair cells, by repressing a set of genes usually enriched in early inner hair cells (Wiwatpanit et al., 2018). It is possible, therefore, that Insm1 also acts in developing ampullary organs to repress hair cell-specific genes.
MafA synergises with Neurod1 (and Pdx1) to activate the insulin promoter in pancreatic beta-cells (reviewed in Liang et al., 2022). Given the ampullary organ-restricted expression of Neurod4 in the paddlefish lateral line system (Modrell et al., 2017a), this raises the possibility that MafA could similarly synergise with Neurod4 to activate ampullary organ-specific target genes.
Rorc encodes two isoforms of a ligand-dependent transcription factor, RAR-related orphan nuclear receptor gamma (RORγ and RORγt), primarily studied for its roles in regulating Th17 cell differentiation and thus autoimmune and inflammatory diseases (see Fauber and Magnuson, 2014; Meijer et al., 2020; Ladurner et al., 2021). Endogenous ligands for RORγ have not been confirmed, but it responds to sterols including the cholesterol precursor, desmosterol (see Hu et al., 2015; Meijer et al., 2020). Retinoic acid has also been reported to inhibit RORγ activity (Stehlin-Gaon et al., 2003). In the axolotl, ampullary organs were missing and far fewer cranial neuromasts formed after retinoic acid treatment for 1 h at late gastrula/early neurula stages (Gibbs and Northcutt, 2004b). However, this most likely reflects an effect on the lateral line placodes themselves, rather than organ formation directly (Gibbs and Northcutt, 2004b). In any case, the mutually exclusive expression of ampullary organ-restricted Rorc and cranial neuromast-restricted Rorb is particularly intriguing (also see next section).
Finally, Sp5 encodes a Wnt/β-catenin effector (Kennedy et al., 2016), suggesting that this signalling pathway might be important for ampullary organ development. Indeed, one of the other ampullary organ-restricted genes, Irx5, is directly upregulated by Wnt/β-catenin signalling in somatic cells of the gonad (Koth et al., 2020).
Overall, the ampullary organ-restricted cranial expression of these six transcription factor genes, as well as Neurod4 (Modrell et al., 2017a), provides a starting point for identifying molecular mechanisms that may be important for the formation of electrosensory lateral line organs.
Five novel mechanosensory lateral line-restricted transcription factor genes
We identified five mechanosensory lateral line-restricted transcription factor genes: the first-such genes reported in electroreceptive vertebrates. Of these, Hmx2, Isl1 and Rorb are expressed in zebrafish lateral line placodes and/or neuromasts (Dufourcq et al., 2006; Bertrand et al., 2007; Feng and Xu, 2010). Hmx2 and Isl1 both encode homeodomain transcription factors. In zebrafish, Hmx2 is expressed throughout lateral line placode development, together with the related gene Hmx3 (Feng and Xu, 2010). Morpholino knockdown experiments suggested a redundant requirement for Hmx2 and Hmx3 for cell proliferation in the migrating posterior lateral line primordium, and for normal neuromast formation (Feng and Xu, 2010). Double mutant analysis of Hmx2 and Hmx3a suggested that the loss of neuromasts arises from stalling of the migrating primordium adjacent to the first few somites, hence failure to deposit neuromasts (England et al., 2020). Recent scRNA-seq data from zebrafish also show that Hmx2 is expressed specifically in anterior-posterior (A/P) support cells in neuromasts (Baek et al., 2022).
We cloned Isl1 because it promotes a more complete conversion by Atoh1 of mouse cochlear supporting cells to hair cells than does Atoh1 alone (Yamashita et al., 2018). In zebrafish neuromasts, Isl1 is expressed in multiple support cell types including central support cells (Lush et al., 2019; Baek et al., 2022), which divide symmetrically to form new hair cells after hair cells are ablated (Romero-Carvajal et al., 2015; Lush et al., 2019). Isl1 is important for aspects of otic placode-derived auditory neuron differentiation (Filova et al., 2022). In neural crest-derived sensory ganglia, Isl1 is expressed in all neurons and is necessary for nociceptor lineage-specific gene expression, for repressing earlier-acting neurogenic transcription factors—including direct repression of Neurod4, which is ampullary organ-specific in the paddlefish lateral line (Modrell et al., 2017a)—and for repressing lineage-inappropriate genes (Sun et al., 2008; Dykes et al., 2011). In the pancreas, Isl1 is a direct transcriptional repressor of Mafa (Du et al., 2009), which we identified here as ampullary organ-restricted (see previous section). We hypothesize that, in electroreceptive species, Isl1 may promote a hair cell fate within neuromasts at least in part by repressing an electroreceptor fate, including by repressing Neurod4 and Mafa.
Rorb, encoding RAR-related orphan nuclear receptor beta (RORβ), is expressed by supporting cells in adult, regenerating and embryonic neuromasts in zebrafish (Dufourcq et al., 2006; Bertrand et al., 2007). Retinoic acid is a confirmed inhibitory ligand for RORβ (Stehlin-Gaon et al., 2003). However, in sterlet, we only identified Rorb expression in cranial neuromasts, suggesting lineage-specific differences. The reciprocal expression of Rorb in cranial neuromasts and Rorc in ampullary organs (see previous section) suggests that these ligand-dependent transcription factors play specific roles in the development of mechanosensory versus electrosensory organs.
In contrast to Hmx2, Isl1 and Rorb, mechanosensory lateral line-restricted Foxg1 and Sox8 are not expressed in the developing lateral line system of zebrafish or Xenopus (e.g., Dirksen and Jamrich, 1995; Papalopulu and Kintner, 1996; Toresson et al., 1998; Eagleson and Dempewolf, 2002; Duggan et al., 2008; Zhao et al., 2009; O'Donnell et al., 2006; Martik et al., 2019). As zebrafish and Xenopus only have a mechanosensory lateral line system (Baker et al., 2013; Baker, 2019), this suggests Foxg1 and Sox8 may play specific roles in the developing mechanosensory lateral line system of electroreceptive bony vertebrates, rather than in lateral line primordium or neuromast development per se. (In cartilaginous fishes, i.e., sharks, Sox8 is expressed in ampullary organs as well as neuromasts; this study and Freitas et al., 2006).
In paddlefish and sterlet, Foxg1 was expressed in the central zones of lateral line sensory ridges where neuromasts form, though excluded from the central domains of neuromasts where hair cells differentiate. In the mouse olfactory epithelium, Foxg1 maintains a proliferative Sox2+ progenitor state (Kawauchi et al., 2009). Similarly, in the inner ear, Foxg1 is expressed by Sox2+ hair cell progenitors and supporting cells in sensory epithelia (Kiernan et al., 2005; Dabdoub et al., 2008; Tasdemir-Yilmaz et al., 2021) (also see Dvorakova et al., 2020), although it is also expressed by a subset of hair cells (Pauley et al., 2006). Foxg1 mouse mutants have reduced inner ear sensory epithelia and a shortened cochlea with numerous additional rows of disorganized hair cells (Pauley et al., 2006; Hwang et al., 2009). Conditional knockout of Foxg1 in supporting cells in the neonatal mouse inner ear resulted in increased numbers of hair cells, potentially by transdifferentiation of supporting cells (Zhang et al., 2019; Zhang et al., 2020). Overall, Foxg1 is an exciting candidate for further investigation at the functional level.
Recent work on otic placode development in chicken embryos (Buzzi et al., 2022) suggests that another of the chondrostean lateral line mechanosensory-restricted transcription factor genes we identified, Sox8, could play an even earlier role than Foxg1. (Sox8 was expressed in ampullary organs as well as neuromasts in cartilaginous fishes, however, suggesting lineage-specific divergence of expression between cartilaginous and bony vertebrates.) Sox8 in chicken lies upstream of all other transcription factor genes in the otic gene regulatory network, including Foxg1 (Buzzi et al., 2022). Ectopic expression of Sox8 in cranial ectoderm drives the formation of ectopic otic vesicles and neurons (Buzzi et al., 2022). In paddlefish, Sox8 displays a similar expression pattern to Foxg1 in elongating lateral line primordia and neuromasts. As mentioned, Sox8 expression has not been reported in the developing lateral line system in either Xenopus or zebrafish (O’Donnell et al., 2006; Martik et al., 2019). Given the “master regulator” role of Sox8 in otic placode development (Buzzi et al., 2022), it is possible that Sox8 lies upstream of Foxg1 in lateral line primordium development specifically in electroreceptive vertebrates (although it may play a separate, later role in ampullary organ development in cartilaginous fishes).
Conclusion
The data presented here, taken together with our previous results in paddlefish (Modrell et al., 2011a; Modrell et al., 2011b; Modrell et al., 2017a; Modrell et al., 2017b), show that most transcription factor genes expressed in developing lateral line organs in chondrostean ray-finned fishes, including many that are required for hair cell development, are expressed in both ampullary organs and neuromasts. This supports the hypothesis that the molecular mechanisms underlying electrosensory and mechanosensory lateral line organ development are highly conserved, and that electroreceptors likely evolved as transcriptionally related sister cell types to lateral line hair cells (Modrell et al., 2017a; Baker and Modrell, 2018). Moreover, in addition to electrosensory-restricted Neurod4 (Modrell et al., 2017a), we have identified a further 12 transcription factors (seven that are electrosensory-restricted on the head; five that are mechanosensory-restricted) that could be involved in the formation of electrosensory versus mechanosensory organs. These are good candidates for functional experiments using CRISPR/Cas9-mediated mutagenesis in sterlet (e.g., Chen et al., 2018; Baloch et al., 2019; Stundl et al., 2022), the next step to further our understanding of the development of these sensory (sister) cell types.
Materials and methods
Embryo collection, staging, and fixation
Fertilized sterlet (Acipenser ruthenus) eggs were obtained from adults bred at the Research Institute of Fish Culture and Hydrobiology (RIFCH), Faculty of Fisheries and Protection of Waters, University of South Bohemia in České Budějovice, Vodňany, Czech Republic. Sterlet animal husbandry, in vitro fertilization and the rearing of embryos and yolk-sac larvae are described in detail in Stundl et al. (2022). Sterlet embryos were staged according to Dettlaff et al. (1993). Animal care was approved by the Ministry of Agriculture of the Czech Republic (MSMT-12550/2016-3), followed the principles of the European Union Harmonized Animal Welfare Act of the Czech Republic, and Principles of Laboratory Animal Care and National Laws 246/1992 “Animal Welfare”, and was conducted in accordance with the Animal Research Committee of RIFCH.
Mississippi paddlefish (Polyodon spathula) embryos were purchased from Osage Catfisheries Inc. (Osage Beach, MO, United States) and reared at approximately 22°C in tanks with filtered and recirculating water (pH 7.2 ± 0.7, salinity of 1.0 ± 0.2 ppt). Paddlefish embryos were staged according to Bemis and Grande (1992). Lesser-spotted catshark (Scyliorhinus canicula) egg cases were reared in a flow-through seawater system at the Station Biologique de Roscoff, France. Catshark embryos were staged according to Ballard et al. (1993).
Upon reaching desired developmental stages, embryos/larvae of all three species were euthanized via overdose of MS-222 (Sigma-Aldrich). Paddlefish and sterlet embryos/yolk-sac larvae were fixed in modified Carnoy’s fixative (6 volumes 100% ethanol: 3 volumes 37% formaldehyde: 1 volume glacial acetic acid) for 3 h at room temperature or for 12–24 h at 4°C, then dehydrated stepwise into ethanol and stored at −20°C. Catshark embryos were fixed overnight at 4°C in 4% paraformaldehyde in phosphate-buffered saline (PBS), washed three times in PBS, dehydrated stepwise into methanol and stored at −20°C.
Generation of de novo transcriptome assemblies from late-larval sterlet heads
Sterlet yolk-sac larvae intended for RNA isolation were preserved in RNAlater (Invitrogen, Thermo Fisher Scientific) and stored at −80°C until processed. Prior to RNA isolation, RNAlater was removed, and heads were manually dissected from sterlet yolk-sac larvae: two at stage 40, two at stage 42, three at stage 45. RNA was then extracted using TRIzol reagent (Invitrogen, Thermo Fisher Scientific) according to the manufacturer’s instructions. RNA concentration was assessed using a Nanodrop N1000 spectro- photometer and integrity using an Agilent 2100 Bioanalyzer (Cambridge Genomic Services, Department of Pathology, University of Cambridge, United Kingdom). Samples with an RNA integrity number (RIN) greater than 9 were submitted for next-generation sequencing at The Centre for Applied Genomics, The Hospital for Sick Children, Toronto, Canada. Libraries were prepared using the NEBNExt Ultra Directional RNA library prep kit and sequenced on an Illumina HiSeq 2500, using Illumina v3 chemistry, following the multiplex paired-end protocol (2 × 125 bases).
Reads were subjected to various quality controls, including high-quality read filtering based on the score value given in fastq files (FastQC version 0.10.1; http://www.bioinformatics.babraham.ac.uk/projects/fastqc/), removal of reads containing primer/adaptor sequences and read-length trimming using Trimmomatic-0.30 (Bolger et al., 2014). De novo assembly was performed using Velvet version 1.2.10 (Zerbino and Birney, 2008) and Oases version 0.2.08 (Schulz et al., 2012). Velvet was run using different k-mer lengths, k31, k43, k47, k53, and k63 along with other default parameters. Oases was run using the same k-mer range. Results from these assemblies were merged, using Velvet and Oases k-mer of k43. All assemblies were performed on a server with 64 cores and 512 GB of RAM. A second de novo assembly was carried out using Trinity version 2.6.6 (Grabherr et al., 2011) using default parameters. This Transcriptome Shotgun Assembly project has been deposited at DDBJ/EMBL/GenBank under the accessions GKLU00000000 (Velvet-Oases assembly) and GKEF00000000 (Trinity assembly). The versions described in this paper are the first versions, GKLU00000000 and GKEF01000000.
Gene cloning and sequence verification
Total RNA was isolated from embryos using Trizol (Invitrogen, Thermo Fisher Scientific), following the manufacturer’s protocol, and cDNA made using the Superscript III First Strand Synthesis kit (Invitrogen, Thermo Fisher Scientific). To design gene-specific PCR primers or synthetic gene fragments to use as riboprobe templates for in situ hybridisation for paddlefish or sterlet, we used the previously published paddlefish transcriptome assembly (NCBI Gene Expression Omnibus accession code GSE92470; Modrell et al., 2017a) or the sterlet transcriptome assemblies reported here (deposited at DDBJ/EMBL/GenBank under the accessions GKLU00000000 and GKEF01000000). Gene-specific primers (Supplementary Table S1) were used to amplify cDNA fragments under standard PCR conditions from cDNA and cloned into the pDrive cloning vector (Qiagen) as previously described (Modrell et al., 2011a). Alternatively, synthetic gene fragments based on paddlefish or sterlet transcriptome data, with added M13 forward and reverse primer adaptors, were ordered from Twist Bioscience. To design gene-specific PCR primers for lesser-spotted catshark, we used S. canicula RNAseq data, publicly available via the Skatebase website (http://skatebase.org/skateblast-skatebase%e2%80%8b/). Catshark cDNA fragments were cloned into the pGEM-T Easy vector (Promega).
The sterlet and paddlefish riboprobe template sequences were designed prior to the publication of chromosome-level genome assemblies for sterlet (Du et al., 2020; Vertebrate Genomes Project NCBI RefSeq assembly GCF_902713425.1) and paddlefish (NCBI RefSeq assembly GCF_017654505.1; Cheng et al., 2021). In sterlet, roughly 70% of ohnologues (i.e., gene paralogs resulting from an independent whole-genome duplication in the sterlet lineage) proved to have been retained (Du et al., 2020). The paddlefish underwent an independent species-specific whole-genome duplication relatively recently (Cheng et al., 2021). Both ohnologues have been retained for all genes described here except sterlet Foxi2 and paddlefish Sox10. Supplementary Table S1 includes each riboprobe’s percentage match with each ohnologue, obtained using the National Center for Biotechnology Information (NCBI) Basic Local Alignment Search Tool (BLAST; https://blast.ncbi.nlm.nih.gov/Blast.cgi; McGinnis and Madden, 2004) by performing a nucleotide BLAST search against the respective reference genome assemblies (sterlet: GCF_902713425.1; paddlefish, GCF_017654505.1). The percentage match with the “targeted” ohnologue ranged from 97.5% to 100% for sterlet (mean ± s.d. 99.7% ± 0.50; n = 42) and from 98.7% to 100% for paddlefish (mean ± s.d. 99.5% ± 0.40; n = 12). The percentage match with the second ohnologue was also high, ranging from 87.4% to 100% for sterlet (mean ± s.d. 97.1% ± 2.65; n = 41) and from 90.7% to 99.0% for paddlefish (mean ± s.d. 95.1% ± 2.63, n = 11) (Supplementary Table S1), suggesting that our riboprobes most likely also target transcripts from the second ohnologue, where present. Indeed, three of our paddlefish riboprobes (Irx5, Lhx8 and Sox2) also worked well in sterlet; the percentage match with the top-match sterlet ohnologue ranged from 93.5% to 96.8% (Supplementary Table S1).
GenBank accession numbers for sterlet (A. ruthenus), paddlefish (P. spathula) and catshark (S. canicula) cDNA fragments, synthetic gene fragments or predicted transcripts from the sterlet or paddlefish genomes are given in Supplementary Table S1, as are the nucleotide ranges targeted by our riboprobes. The sterlet Rorc sequence was absent from the GCA_010645085.2 assembly (Du et al., 2020), but present in the reference genome (NCBI RefSeq assembly GCF_902713425.1).
Individual clones were verified by sequencing (Department of Biochemistry Sequencing Facility, University of Cambridge, United Kingdom, or Genewiz, Azenta Life Sciences, United Kingdom). Sequence identity was checked using the NCBI BLAST tool. Sequences whose identity was still inconclusive following a general BLAST search were checked against the sterlet reference genome (GCF_902713425.1) or paddlefish reference genome (GCF_017654505.1; Cheng et al., 2021) using BLAST. However, we note here that this approach did not result in conclusive identification of our Insm family, Klf or Ror gene transcripts. We thus performed phylogenetic analysis of these gene families using predicted protein sequences from reference genome assemblies of a range of species of deuterostomes. The accession numbers for these sequences are listed in Supplementary Table S2. The sequences were aligned using MAFFT (Katoh and Standley, 2013) and trimmed using TrimAL (Capella-Gutiérrez et al., 2009) before using IQ-TREE2 (Minh et al., 2020) with Model Finder (Kalyaanamoorthy et al., 2017) for phylogenetic tree inference and bootstrap analysis. Trees were then visualised using TreeGraph 2 (Stöver and Müller, 2010). Our phylogenetic analysis of Insm family genes revealed that the Insm2 ohnologues in the reference sterlet genome (GCF_902713425.1) have been mis-annotated as Insm1 and Insm1-like, while in the reference paddlefish genome (Cheng et al., 2021), one of the Insm2 ohnologues has been mis-annotated as Insm1a-like (Supplementary Figure S5; Supplementary Table S1). Similarly, our phylogenetic analysis of Klf family genes revealed that one of the Klf17 ohnologues in the reference sterlet genome (GCF_902713425.1) has been mis-annotated as Klf4 (Supplementary Figures S6, S7; Supplementary Table S1). Finally, our phylogenetic analysis of RAR-related orphan nuclear receptor (Ror) genes suggested that two of the three Rorc genes in the reference sterlet genome (GCF_902713425.1) have been mis-annotated as Rora-like and Rorab-like (Supplementary Figure S8; Supplementary Table S1).
In situ hybridization and immunohisto- chemistry
Digoxigenin-labelled antisense riboprobes were synthesized from cloned cDNA fragments (Supplementary Table S1) using T7 or SP6 polymerases (Promega) and digoxigenin-labeled dUTPs (Roche). Alternatively, synthetic gene fragments (Twist Bioscience) with added M13 forward and reverse primer adaptors were PCR-amplified under standard conditions using the M13 forward primer, and the M13 reverse primer containing an overhang with the SP6 polymerase promoter. The PCR product was then used as a template for riboprobe synthesis by in vitro transcription using SP6 polymerase and digoxigenin-labelled dUTPs (Roche). Each riboprobe was tested a minimum of two times, using at least three embryos per stage.
Wholemount in situ hybridization (ISH) was performed as previously described (Modrell et al., 2011a). In some cases, sterlet and paddlefish yolk-sac larvae were processed into pre-hybridization buffer as described (Modrell et al., 2011a), then stored at −20°C for up to a month in this solution before continuing the protocol. For weaker riboprobes, overnight incubations at 4°C in MABT (0.1 M maleic acid, 150 mM NaCl, 0.1% Tween-20, pH 7.5) and/or NTMT (100 mM NaCl, 100 mM Tris, pH 9.5, 50 mM MgCl2, 0.1% Tween-20) were added prior to the colour reaction, to increase the signal to background staining ratio.
Wholemount immunostaining was performed as previously described (Metscher and Müller, 2011). When using sterlet embryos or yolk-sac larvae that had not already been subject to ISH, bleaching and proteinase K treatment were performed prior to immunostaining, as described for ISH (Modrell et al., 2011a). A primary antibody against Sox2 (rabbit monoclonal, ab92494; Abcam) was used at 1:200 and a horseradish peroxidase-conjugated goat anti-rabbit antibody (Jackson ImmunoResearch) at 1:300. For the histochemical reaction, the metallographic peroxidase substrate EnzMet kit (Nanoprobes) was used according to the manufacturer’s instructions.
For sPTkinmounts after wholemount ISH and/or immunostaining, skin samples were dissected using forceps and microcapillary needles and mounted on Superfrost Plus slides (VWR) using Fluoroshield mounting medium with DAPI (Sigma-Aldrich).
For ISH on sections, embryos were embedded in paraffin wax and sectioned at 10 μm as previously described (O'Neill et al., 2007). ISH on sections was performed as previously described (O’Neill et al., 2007; Miller et al., 2017) except that slides were not treated with proteinase K prior to hybridization and BMP Purple (Roche) was used for the colour reaction.
Imaging and image processing
Wholemount embryos and larvae were positioned in a slit in an agar-coated Petri dish with PBS and imaged using a Leica MZFLIII dissecting microscope equipped with a MicroPublisher 5.0 RTV camera (QImaging) or a MicroPublisher 6 color CCD camera (Teledyne Photometrics). Skinmounts and sections were imaged using a Zeiss AxioSkop 2 microscope equipped with a Retiga 2000R camera and RGB pancake (QImaging) or a MicroPublisher 6 color CCD camera (Teledyne Photometrics). Images were acquired using QCapture Pro 6.0 or 7.0 software (QImaging) or Ocular software (Teledyne Photometrics). For most wholemount embryos and larvae, as well as skinmounts, a stack of images was taken by manually focusing through the sample, then focus stacking was performed using Helicon Focus software (Helicon Soft Limited). Images were processed in Adobe Photoshop (Adobe Systems Inc.).
Data availability statement
The Transcriptome Shotgun Assembly project has been deposited at DDBJ/EMBL/GenBank under the accessions GKLU00000000 (https://www.ncbi.nlm.nih.gov/nuccore/GKLU00000000) and GKEF01000000 (https://www.ncbi.nlm.nih.gov/nuccore/GKEF00000000.1). The versions described in this paper are the first versions, GKLU00000000 and GKEF01000000. The publication and associated Supplementary Figures include representative example images of embryos from each experiment. Additional data underlying this publication consist of further images of these and other embryos from each experiment. Public sharing of these images is not cost-efficient, but they are available from the corresponding author upon reasonable request.
Ethics statement
The animal study was approved by the Animal Research Committee of Research Institute of Fish Culture and Hydrobiology, Faculty of Fisheries and Protection of Waters, University of South Bohemia in České Budějovice, Vodňany, Czech Republic and Ministry of Agriculture of the Czech Republic (MSMT-12550/2016-3). Experimental fish were maintained according to the principles of the European Union (EU) Harmonized Animal Welfare Act of the Czech Republic, and Principles of Laboratory Animal Care and National Laws 246/1992 “Animal Welfare” on the protection of animals. The study was conducted in accordance with the local legislation and institutional requirements.
Author contributions
MM: Conceptualization, Investigation, Project Administration, Visualization, Writing–original draft, Writing–review and editing. MSM: Conceptualization, Investigation, Writing–review and editing. JAG: Conceptualization, Funding acquisition, Investigation, Writing–review and editing. AC: Investigation, Writing–review and editing. IF: Investigation, Writing–review and editing. RL: Investigation, Writing–review and editing. GM: Supervision, Writing–review and editing. DG: Resources, Writing–review and editing. MP: Funding acquisition, Resources, Writing–review and editing. CB: Conceptualization, Funding acquisition, Project administration, Writing–original draft, Writing–review and editing.
Funding
The authors declare that financial support was received for the research, authorship, and/or publication of this article. This work was supported by the Biotechnology and Biological Sciences Research Council (BB/F00818X/1 and BB/P001947/1 to CB), the Leverhulme Trust (RPG-383 to CB) and the Royal Society (Newton International Fellowship to JAG). Additional support for MM was provided by the Cambridge Isaac Newton Trust (grant 20.07 [c] to CB) and by the School of the Biological Sciences, University of Cambridge. AC was supported by a PhD research studentship from the Anatomical Society, with additional funding from the Cambridge Philosophical Society. The work of MP was supported by the Ministry of Education, Youth and Sports of the Czech Republic, projects CENAKVA (LM2018099), Biodiversity (CZ.02.1.01/0.0/0.0/16_025/0007370) and Czech Science Foundation (22-31141J). Collection of S. canicula embryos was funded by an Association of European Marine Biological Laboratories ASSEMBLE award to JAG.
Acknowledgments
Thanks to Marek Rodina and Martin Kahanec for their help with sterlet spawns, and Michaela Vazačová for her help with embryo incubation and fixation. Thanks to Tatjana Piotrowski and her lab at the Stowers Institute for Medical Research (Kansas City, MO, United States) and Steve and Pete Kahrs and the Kahrs family (Osage Catfisheries, Inc.) for hosting MSM during paddlefish spawning seasons. Thanks to Christine Hirschberger and Rolf Ericsson for their help with some of the in situ hybridization rounds. Thanks to Nathanael Walker-Hale for advice on phylogenetic analysis.
Conflict of interest
The authors declare that the research was conducted in the absence of any commercial or financial relationships that could be construed as a potential conflict of interest.
Publisher’s note
All claims expressed in this article are solely those of the authors and do not necessarily represent those of their affiliated organizations, or those of the publisher, the editors and the reviewers. Any product that may be evaluated in this article, or claim that may be made by its manufacturer, is not guaranteed or endorsed by the publisher.
Supplementary material
The Supplementary Material for this article can be found online at: https://www.frontiersin.org/articles/10.3389/fcell.2024.1327924/full#supplementary-material
References
Ahmed, M., Wong, E. Y. M., Sun, J., Xu, J., Wang, F., and Xu, P.-X. (2012). Eya1-Six1 interaction is sufficient to induce hair cell fate in the cochlea by activating Atoh1 expression in cooperation with Sox2. Dev. Cell 22, 377–390. doi:10.1016/j.devcel.2011.12.006
Baek, S., Tran, N. T. T., Diaz, D. C., Tsai, Y.-Y., Acedo, J. N., Lush, M. E., et al. (2022). Single-cell transcriptome analysis reveals three sequential phases of gene expression during zebrafish sensory hair cell regeneration. Dev. Cell 57, 799–819. doi:10.1016/j.devcel.2022.03.001
Baker, C. V. H. (2019). “The development and evolution of lateral line electroreceptors: insights from comparative molecular approaches,” in Electroreception: Fundamental Insights from Comparative Approaches. Editors B. A. Carlson, J. A. Sisneros, A. N. Popper, and R. R. Fay (Cham: Springer), 25–62. Available at: https://link.springer.com/chapter/10.1007/978-3-030-29105-1_2.
Baker, C. V. H., and Modrell, M. S. (2018). Insights into electroreceptor development and evolution from molecular comparisons with hair cells. Integr. Comp. Biol. 58, 329–340. doi:10.1093/icb/icy037
Baker, C. V. H., Modrell, M. S., and Gillis, J. A. (2013). The evolution and development of vertebrate lateral line electroreceptors. J. Exp. Biol. 216, 2515–2522. doi:10.1242/jeb.082362
Ballard, W. W., Mellinger, J., and Lechenault, H. (1993). A series of normal stages for development of Scyliorhinus canicula, the lesser spotted dogfish (Chondrichthyes: Scylorhinidae). J. Exp. Zool. 267, 318–336. doi:10.1002/jez.1402670309
Baloch, A. R., Franěk, R., Tichopád, T., Fučíková, M., Rodina, M., and Pšenička, M. (2019). Dnd1 knockout in sturgeons by CRISPR/Cas9 generates germ cell free host for surrogate production. Animals 9, 174. doi:10.3390/ani9040174
Bardot, E. S., Valdes, V. J., Zhang, J., Perdigoto, C. N., Nicolis, S., Hearn, S. A., et al. (2013). Polycomb subunits Ezh1 and Ezh2 regulate the Merkel cell differentiation program in skin stem cells. EMBO J. 32, 1990–2000. doi:10.1038/emboj.2013.110
Bellono, N. W., Leitch, D. B., and Julius, D. (2017). Molecular basis of ancestral vertebrate electroreception. Nature 543, 391–396. doi:10.1038/nature21401
Bellono, N. W., Leitch, D. B., and Julius, D. (2018). Molecular tuning of electroreception in sharks and skates. Nature 558, 122–126. doi:10.1038/s41586-018-0160-9
Bemis, W. E., and Grande, L. (1992). Early development of the actinopterygian head. I. External development and staging of the paddlefish Polyodon spathula. J. Morphol. 213, 47–83. doi:10.1002/jmor.1052130106
Bennett, M. V. L., and Obara, S. (1986). “Ionic mechanisms and pharmacology of electroreceptors,” in Electroreception. Editors T. H. Bullock, and W. Heiligenberg (New York: Wiley), 157–181.
Bertrand, S., Thisse, B., Tavares, R., Sachs, L., Chaumot, A., Bardet, P. L., et al. (2007). Unexpected novel relational links uncovered by extensive developmental profiling of nuclear receptor expression. PLoS Genet. 3, e188. doi:10.1371/journal.pgen.0030188
Bodznick, D., and Montgomery, J. C. (2005). “The physiology of low-frequency electrosensory systems,” in Electroreception. Editors T. H. Bullock, C. D. Hopkins, A. N. Popper, and R. R. Fay (New York: Springer), 132–153. Available at: https://link.springer.com/chapter/10.1007/0-387-28275-0_6.
Bolger, A. M., Lohse, M., and Usadel, B. (2014). Trimmomatic: a flexible trimmer for Illumina sequence data. Bioinformatics 30, 2114–2120. doi:10.1093/bioinformatics/btu170
Bonilla-Claudio, M., Wang, J., Bai, Y., Klysik, E., Selever, J., and Martin, J. F. (2012). Bmp signaling regulates a dose-dependent transcriptional program to control facial skeletal development. Development 139, 709–719. doi:10.1242/dev.073197
Bosse, A., Stoykova, A., Nieselt-Struwe, K., Chowdhury, K., Copeland, N. G., Jenkins, N. A., et al. (2000). Identification of a novel mouse Iroquois homeobox gene, Irx5, and chromosomal localisation of all members of the mouse Iroquois gene family. Dev. Dyn. 218, 160–174. doi:10.1002/(SICI)1097-0177(200005)218:1<160::AID-DVDY14>3.0.CO;2-2
Brown, T. L., Horton, E. C., Craig, E. W., Goo, C. E. A., Black, E. C., Hewitt, M. N., et al. (2023). Dermal appendage-dependent patterning of zebrafish atoh1a+ Merkel cells. eLife 12, e85800. doi:10.7554/eLife.85800
Butts, T., Modrell, M. S., Baker, C. V. H., and Wingate, R. J. T. (2014). The evolution of the vertebrate cerebellum: absence of a proliferative external granule layer in a non-teleost ray-finned fish. Evol. Dev. 16, 92–100. doi:10.1111/ede.12067
Buzzi, A. L., Chen, J., Thiery, A., Delile, J., and Streit, A. (2022). Sox8 remodels the cranial ectoderm to generate the ear. Proc. Natl. Acad. Sci. U.S.A. 119, e2118938119. doi:10.1073/pnas.2118938119
Camacho, S., Ostos, M. D. V., Llorente, J. I., Sanz, A., García, M., Domezain, A., et al. (2007). Structural characteristics and development of ampullary organs in Acipenser naccarii. Anat. Rec. 290, 1178–1189. doi:10.1002/ar.20569
Capella-Gutiérrez, S., Silla-Martínez, J. M., and Gabaldón, T. (2009). trimAl: a tool for automated alignment trimming in large-scale phylogenetic analyses. Bioinformatics 25, 1972–1973. doi:10.1093/bioinformatics/btp348
Caprara, G. A., and Peng, A. W. (2022). Mechanotransduction in mammalian sensory hair cells. Mol. Cell. Neurosci. 120, 103706. doi:10.1016/j.mcn.2022.103706
Cardeña-Núñez, S., Sánchez-Guardado, L. Ó., Corral-San-Miguel, R., Rodríguez-Gallardo, L., Marín, F., Puelles, L., et al. (2016). Expression patterns of Irx genes in the developing chick inner ear. Brain Struct. Funct. 222, 2071–2092. doi:10.1007/s00429-016-1326-6
Chagnaud, B. P., Wilkens, L. A., and Hofmann, M. (2021). “The ampullary electrosensory system—a paddlefish case study,” in The Senses: A Comprehensive Reference. Editor B. Fritzsch (Elsevier), 215–227. doi:10.1016/B978-0-12-809324-5.24210-7
Chen, J., Wang, W., Tian, Z., Dong, Y., Dong, T., Zhu, H., et al. (2018). Efficient gene transfer and gene editing in sterlet (Acipenser ruthenus). Front. Genet. 9, 117. doi:10.3389/fgene.2018.00117
Chen, Y., Gu, Y., Li, Y., Li, G. L., Chai, R., Li, W., et al. (2021). Generation of mature and functional hair cells by co-expression of Gfi1, Pou4f3, and Atoh1 in the postnatal mouse cochlea. Cell Rep. 35, 109016. doi:10.1016/j.celrep.2021.109016
Cheng, C. W., Chow, R. L., Lebel, M., Sakuma, R., Cheung, H.O.-L., Thanabalasingham, V., et al. (2005). The Iroquois homeobox gene, Irx5, is required for retinal cone bipolar cell development. Dev. Biol. 287, 48–60. doi:10.1016/j.ydbio.2005.08.029
Cheng, P., Huang, Y., Lv, Y., Du, H., Ruan, Z., Li, C., et al. (2021). The American paddlefish genome provides novel insights into chromosomal evolution and bone mineralization in early vertebrates. Mol. Biol. Evol. 38, 1595–1607. doi:10.1093/molbev/msaa326
Crampton, W. G. R. (2019). Electroreception, electrogenesis and electric signal evolution. J. Fish Biol. 95, 92–134. doi:10.1111/jfb.13922
Dabdoub, A., Puligilla, C., Jones, J. M., Fritzsch, B., Cheah, K. S., Pevny, L. H., et al. (2008). Sox2 signaling in prosensory domain specification and subsequent hair cell differentiation in the developing cochlea. Proc. Natl. Acad. Sci. U.S.A. 105, 18396–18401. doi:10.1073/pnas.0808175105
Deprez, M., Zaragosi, L. E., Truchi, M., Becavin, C., Ruiz García, S., Arguel, M. J., et al. (2020). A single-cell atlas of the human healthy airways. Am. J. Respir. Crit. Care Med. 202, 1636–1645. doi:10.1164/rccm.201911-2199OC
Dettlaff, T. A., Ginsburg, A. S., and Schmalhausen, O. I. (1993). Sturgeon Fishes: Developmental Biology and Aquaculture. Berlin: Springer-Verlag. Available at: https://link.springer.com/book/10.1007/978-3-642-77057-9.
Dirksen, M.-L., and Jamrich, M. (1995). Differential expression of fork head genes during early Xenopus and zebrafish development. Dev. Genet. 17, 107–116. doi:10.1002/dvg.1020170203
Du, A., Hunter, C. S., Murray, J., Noble, D., Cai, C.-L., Evans, S. M., et al. (2009). Islet-1 is required for the maturation, proliferation, and survival of the endocrine pancreas. Diabetes 58, 2059–2069. doi:10.2337/db08-0987
Du, K., Stöck, M., Kneitz, S., Klopp, C., Woltering, J. M., Adolfi, M. C., et al. (2020). The sterlet sturgeon genome sequence and the mechanisms of segmental rediploidization. Nat. Ecol. Evol. 4, 841–852. doi:10.1038/s41559-020-1166-x
Dufourcq, P., Roussigné, M., Blader, P., Rosa, F., Peyrieras, N., and Vriz, S. (2006). Mechano-sensory organ regeneration in adults: the zebrafish lateral line as a model. Mol. Cell. Neurosci. 33, 180–187. doi:10.1016/j.mcn.2006.07.005
Duggan, C. D., Demaria, S., Baudhuin, A., Stafford, D., and Ngai, J. (2008). Foxg1 is required for development of the vertebrate olfactory system. J. Neurosci. 28, 5229–5239. doi:10.1523/JNEUROSCI.1134-08.2008
Dvorakova, M., Macova, I., Bohuslavova, R., Anderova, M., Fritzsch, B., and Pavlinkova, G. (2020). Early ear neuronal development, but not olfactory or lens development, can proceed without SOX2. Dev. Biol. 457, 43–56. doi:10.1016/j.ydbio.2019.09.003
Dykes, I. M., Tempest, L., Lee, S.-I., and Turner, E. E. (2011). Brn3a and Islet1 act epistatically to regulate the gene expression program of sensory differentiation. J. Neurosci. 31, 9789–9799. doi:10.1523/JNEUROSCI.0901-11.2011
Eagleson, G. W., and Dempewolf, R. D. (2002). The role of the anterior neural ridge and Fgf-8 in early forebrain patterning and regionalization in Xenopus laevis. Comp. Biochem. Physiol. B Biochem. Mol. Biol. 132, 179–189. doi:10.1016/s1096-4959(01)00521-8
Elliott, K. L., and Fritzsch, B. (2021). “Evolution and development of lateral line and electroreception: an integrated perception of neurons, hair cells and brainstem nuclei,” in The Senses: A Comprehensive Reference. Editor B. Fritzsch (Elsevier), 95–115. doi:10.1016/B978-0-12-809324-5.24170-9
Elliott, K. L., Fritzsch, B., and Duncan, J. S. (2018). Evolutionary and developmental biology provide insights into the regeneration of organ of Corti hair cells. Front. Cell. Neurosci. 12, 252. doi:10.3389/fncel.2018.00252
England, S. J., Cerda, G. A., Kowalchuk, A., Sorice, T., Grieb, G., and Lewis, K. E. (2020). Hmx3a has essential functions in zebrafish spinal cord, ear and lateral line development. Genetics 216, 1153–1185. doi:10.1534/genetics.120.303748
Fauber, B. P., and Magnuson, S. (2014). Modulators of the nuclear receptor retinoic acid receptor-related orphan receptor-γ (RORγ or RORc). J. Med. Chem. 57, 5871–5892. doi:10.1021/jm401901d
Feng, Y., and Xu, Q. (2010). Pivotal role of hmx2 and hmx3 in zebrafish inner ear and lateral line development. Dev. Biol. 339, 507–518. doi:10.1016/j.ydbio.2009.12.028
Filova, I., Pysanenko, K., Tavakoli, M., Vochyanova, S., Dvorakova, M., Bohuslavova, R., et al. (2022). ISL1 is necessary for auditory neuron development and contributes toward tonotopic organization. Proc. Natl. Acad. Sci. U.S.A. 119, e2207433119. doi:10.1073/pnas.2207433119
Freitas, R., Zhang, G., Albert, J. S., Evans, D. H., and Cohn, M. J. (2006). Developmental origin of shark electrosensory organs. Evol. Dev. 8, 74–80. doi:10.1111/j.1525-142X.2006.05076.x
Gibbs, M. A., and Northcutt, R. G. (2004a). Development of the lateral line system in the shovelnose sturgeon. Brain Behav. Evol. 64, 70–84. doi:10.1159/000079117
Gibbs, M. A., and Northcutt, R. G. (2004b). Retinoic acid repatterns axolotl lateral line receptors. Int. J. Dev. Biol. 48, 63–66. doi:10.1387/ijdb.15005576
Gillis, J. A., Modrell, M. S., Northcutt, R. G., Catania, K. C., Luer, C. A., and Baker, C. V. H. (2012). Electrosensory ampullary organs are derived from lateral line placodes in cartilaginous fishes. Development 139, 3142–3146. doi:10.1242/dev.084046
Gnedeva, K., and Hudspeth, A. J. (2015). SoxC transcription factors are essential for the development of the inner ear. Proc. Natl. Acad. Sci. U.S.A. 112, 14066–14071. doi:10.1073/pnas.1517371112
Gómez-Skarmeta, J. L., and Modolell, J. (2002). Iroquois genes: genomic organization and function in vertebrate neural development. Curr. Opin. Genet. Dev. 12, 403–408. doi:10.1016/s0959-437x(02)00317-9
Grabherr, M. G., Haas, B. J., Yassour, M., Levin, J. Z., Thompson, D. A., Amit, I., et al. (2011). Full-length transcriptome assembly from RNA-Seq data without a reference genome. Nat. Biotechnol. 29, 644–652. doi:10.1038/nbt.1883
Grant, K. A., Raible, D. W., and Piotrowski, T. (2005). Regulation of latent sensory hair cell precursors by glia in the zebrafish lateral line. Neuron 45, 69–80. doi:10.1016/j.neuron.2004.12.020
Haber, A. L., Biton, M., Rogel, N., Herbst, R. H., Shekhar, K., Smillie, C., et al. (2017). A single-cell survey of the small intestinal epithelium. Nature 551, 333–339. doi:10.1038/nature24489
He, Y., Lu, X., Qian, F., Liu, D., Chai, R., and Li, H. (2017). Insm1a is required for zebrafish posterior lateral line development. Front. Mol. Neurosci. 10, 241. doi:10.3389/fnmol.2017.00241
Hesse, K., Vaupel, K., Kurt, S., Buettner, R., Kirfel, J., and Moser, M. (2011). AP-2δ is a crucial transcriptional regulator of the posterior midbrain. PLoS ONE 6, e23483. doi:10.1371/journal.pone.0023483
Hoffman, B. U., Baba, Y., Griffith, T. N., Mosharov, E. V., Woo, S. H., Roybal, D. D., et al. (2018). Merkel cells activate sensory neural pathways through adrenergic synapses. Neuron 100, 1401–1413. doi:10.1016/j.neuron.2018.10.034
Houweling, A. C., Dildrop, R., Peters, T., Mummenhoff, J., Moorman, A. F. M., Rüther, U., et al. (2001). Gene and cluster-specific expression of the Iroquois family members during mouse development. Mech. Dev. 107, 169–174. doi:10.1016/s0925-4773(01)00451-8
Hu, X., Wang, Y., Hao, L.-Y., Liu, X., Lesch, C. A., Sanchez, B. M., et al. (2015). Sterol metabolism controls TH17 differentiation by generating endogenous RORγ agonists. Nat. Chem. Biol. 11, 141–147. doi:10.1038/nchembio.1714
Huang, M., Sage, C., Li, H., Xiang, M., Heller, S., and Chen, Z.-Y. (2008). Diverse expression patterns of LIM-homeodomain transcription factors (LIM-HDs) in mammalian inner ear development. Dev. Dyn. 237, 3305–3312. doi:10.1002/dvdy.21735
Huang, X., Chen, Q., Luo, W., Pakvasa, M., Zhang, Y., Zheng, L., et al. (2022). SATB2: a versatile transcriptional regulator of craniofacial and skeleton development, neurogenesis and tumorigenesis, and its applications in regenerative medicine. Genes Dis. 9, 95–107. doi:10.1016/j.gendis.2020.10.003
Hwang, C. H., Simeone, A., Lai, E., and Wu, D. K. (2009). Foxg1 is required for proper separation and formation of sensory cristae during inner ear development. Dev. Dyn. 238, 2725–2734. doi:10.1002/dvdy.22111
Jan, T. A., Eltawil, Y., Ling, A. H., Chen, L., Ellwanger, D. C., Heller, S., et al. (2021). Spatiotemporal dynamics of inner ear sensory and non-sensory cells revealed by single-cell transcriptomics. Cell Rep. 36, 109358. doi:10.1016/j.celrep.2021.109358
Jen, H.-I., Singh, S., Tao, L., Maunsell, H. R., Segil, N., and Groves, A. K. (2022). GFI1 regulates hair cell differentiation by acting as an off-DNA transcriptional co-activator of ATOH1, and a DNA-binding repressor. Sci. Rep. 12, 7793. doi:10.1038/s41598-022-11931-0
Jessen, K. R., and Mirsky, R. (2019). Schwann cell precursors; multipotent glial cells in embryonic nerves. Front. Mol. Neurosci. 12, 69. doi:10.3389/fnmol.2019.00069
Jørgensen, J. M. (2005). “Morphology of electroreceptive sensory organs,” in Electroreception. Editors T. H. Bullock, C. D. Hopkins, A. N. Popper, and R. R. Fay (New York: Springer), 47–67. Available at: https://link.springer.com/chapter/10.1007/0-387-28275-0_3.
Kalyaanamoorthy, S., Minh, B. Q., Wong, T. K. F., von Haeseler, A., and Jermiin, L. S. (2017). ModelFinder: fast model selection for accurate phylogenetic estimates. Nat. Methods 14, 587–589. doi:10.1038/nmeth.4285
Katoh, K., and Standley, D. M. (2013). MAFFT multiple sequence alignment software version 7: improvements in performance and usability. Mol. Biol. Evol. 30, 772–780. doi:10.1093/molbev/mst010
Kawauchi, S., Santos, R., Kim, J., Hollenbeck, P. L. W., Murray, R. C., and Calof, A. L. (2009). The role of Foxg1 in the development of neural stem cells of the olfactory epithelium. Ann. N. Y. Acad. Sci. 1170, 21–27. doi:10.1111/j.1749-6632.2009.04372.x
Kelsh, R. N., and Eisen, J. S. (2000). The zebrafish colourless gene regulates development of non-ectomesenchymal neural crest derivatives. Development 127, 515–525. doi:10.1242/dev.127.3.515
Kennedy, M. W., Chalamalasetty, R. B., Thomas, S., Garriock, R. J., Jailwala, P., and Yamaguchi, T. P. (2016). Sp5 and Sp8 recruit β-catenin and Tcf1-Lef1 to select enhancers to activate Wnt target gene transcription. Proc. Natl. Acad. Sci. U.S.A. 113, 3545–3550. doi:10.1073/pnas.1519994113
Kiernan, A. E., Pelling, A. L., Leung, K. K., Tang, A. S., Bell, D. M., Tease, C., et al. (2005). Sox2 is required for sensory organ development in the mammalian inner ear. Nature 434, 1031–1035. doi:10.1038/nature03487
Kotas, M. E., O’Leary, C. E., and Locksley, R. M. (2023). Tuft cells: context- and tissue-specific programming for a conserved cell lineage. Annu. Rev. Pathol. 18, 311–335. doi:10.1146/annurev-pathol-042320-112212
Koth, M. L., Garcia-Moreno, S. A., Novak, A., Holthusen, K. A., Kothandapani, A., Jiang, K., et al. (2020). Canonical Wnt/β-catenin activity and differential epigenetic marks direct sexually dimorphic regulation of Irx3 and Irx5 in developing mouse gonads. Development 147, dev183814. doi:10.1242/dev.183814
Kotkamp, K., Mössner, R., Allen, A., Onichtchouk, D., and Driever, W. (2014). A Pou5f1/Oct4 dependent Klf2a, Klf2b, and Klf17 regulatory sub-network contributes to EVL and ectoderm development during zebrafish embryogenesis. Dev. Biol. 385, 433–447. doi:10.1016/j.ydbio.2013.10.025
Ladurner, A., Schwarz, P. F., and Dirsch, V. M. (2021). Natural products as modulators of retinoic acid receptor-related orphan receptors (RORs). Nat. Prod. Rep. 38, 757–781. doi:10.1039/d0np00047g
Lecaudey, V., Anselme, I., Dildrop, R., Rüther, U., and Schneider-Maunoury, S. (2005). Expression of the zebrafish Iroquois genes during early nervous system formation and patterning. J. Comp. Neurol. 492, 289–302. doi:10.1002/cne.20765
Leitch, D. B., and Julius, D. (2019). “Electrosensory transduction: comparisons across structure, afferent response properties, and cellular physiology,” in Electroreception: Fundamental Insights from Comparative Approaches. Editors B. A. Carlson, J. A. Sisneros, A. N. Popper, and R. R. Fay (Cham: Springer), 63–90. Available at: https://link.springer.com/chapter/10.1007/978-3-030-29105-1_3.
Lesko, M. H., Driskell, R. R., Kretzschmar, K., Goldie, S. J., and Watt, F. M. (2013). Sox2 modulates the function of two distinct cell lineages in mouse skin. Dev. Biol. 382, 15–26. doi:10.1016/j.ydbio.2013.08.004
Li, X., Gaillard, F., Monckton, E. A., Glubrecht, D. D., Persad, A. R., Moser, M., et al. (2016). Loss of AP-2delta reduces retinal ganglion cell numbers and axonal projections to the superior colliculus. Mol. Brain 9, 62. doi:10.1186/s13041-016-0244-0
Liang, J., Chirikjian, M., Pajvani, U. B., and Bartolomé, A. (2022). MafA regulation in β-cells: from transcriptional to post-translational mechanisms. Biomolecules 12, 535. doi:10.3390/biom12040535
López-Schier, H., and Hudspeth, A. J. (2005). Supernumerary neuromasts in the posterior lateral line of zebrafish lacking peripheral glia. Proc. Natl. Acad. Sci. U.S.A. 102, 1496–1501. doi:10.1073/pnas.0409361102
Lorenzen, S. M., Duggan, A., Osipovich, A. B., Magnuson, M. A., and García-Añoveros, J. (2015). Insm1 promotes neurogenic proliferation in delaminated otic progenitors. Mech. Dev. 138 Pt 3, 233–245. doi:10.1016/j.mod.2015.11.001
Lu, X., and Sipe, C. W. (2016). Developmental regulation of planar cell polarity and hair-bundle morphogenesis in auditory hair cells: lessons from human and mouse genetics. WIREs Dev. Biol. 5, 85–101. doi:10.1002/wdev.202
Lush, M. E., Diaz, D. C., Koenecke, N., Baek, S., Boldt, H., St Peter, M. K., et al. (2019). scRNA-Seq reveals distinct stem cell populations that drive hair cell regeneration after loss of Fgf and Notch signaling. eLife 8, e44431. doi:10.7554/eLife.44431
Mak, A. C. Y., Szeto, I. Y. Y., Fritzsch, B., and Cheah, K. S. E. (2009). Differential and overlapping expression pattern of SOX2 and SOX9 in inner ear development. Gene Expr. Patterns 9, 444–453. doi:10.1016/j.gep.2009.04.003
Martik, M. L., Gandhi, S., Uy, B. R., Gillis, J. A., Green, S. A., Simoes-Costa, M., et al. (2019). Evolution of the new head by gradual acquisition of neural crest regulatory circuits. Nature 574, 675–678. doi:10.1038/s41586-019-1691-4
Matern, M. S., Milon, B., Lipford, E. L., McMurray, M., Ogawa, Y., Tkaczuk, A., et al. (2020). GFI1 functions to repress neuronal gene expression in the developing inner ear hair cells. Development 147, dev186015. doi:10.1242/dev.186015
McGinnis, S., and Madden, T. L. (2004). BLAST: at the core of a powerful and diverse set of sequence analysis tools. Nucleic Acids Res. 32, W20–W25. doi:10.1093/nar/gkh435
McGovern, M. M., Hosamani, I. V., Niu, Y., Nguyen, K. Y., Zong, C., and Groves, A. K. (2024). Expression of Atoh1, Gfi1, and Pou4f3 in the mature cochlea reprograms nonsensory cells into hair cells. Proc. Natl. Acad. Sci. U.S.A. 121, e2304680121. doi:10.1073/pnas.2304680121
Meijer, F. A., Doveston, R. G., de Vries, R. M. J. M., Vos, G. M., Vos, A. A. A., Leysen, S., et al. (2020). Ligand-based design of allosteric retinoic acid receptor-related orphan receptor γt (RORγt) inverse agonists. J. Med. Chem. 63, 241–259. doi:10.1021/acs.jmedchem.9b01372
Metscher, B. D., and Müller, G. B. (2011). MicroCT for molecular imaging: quantitative visualization of complete three-dimensional distributions of gene products in embryonic limbs. Dev. Dyn. 240, 2301–2308. doi:10.1002/dvdy.22733
Miller, S. R., Perera, S. N., and Baker, C. V. H. (2017). Constitutively active Notch1 converts cranial neural crest-derived frontonasal mesenchyme to perivascular cells in vivo. Biol. Open 6, 317–325. doi:10.1242/bio.023887
Minh, B. Q., Schmidt, H. A., Chernomor, O., Schrempf, D., Woodhams, M. D., von Haeseler, A., et al. (2020). IQ-TREE 2: new models and efficient methods for phylogenetic inference in the genomic era. Mol. Biol. Evol. 37, 1530–1534. doi:10.1093/molbev/msaa015
Modrell, M. S., and Baker, C. V. H. (2012). Evolution of electrosensory ampullary organs: conservation of Eya4 expression during lateral line development in jawed vertebrates. Evol. Dev. 14, 277–285. doi:10.1111/j.1525-142X.2012.00544.x
Modrell, M. S., Bemis, W. E., Northcutt, R. G., Davis, M. C., and Baker, C. V. H. (2011a). Electrosensory ampullary organs are derived from lateral line placodes in bony fishes. Nat. Commun. 2, 496. doi:10.1038/ncomms1502
Modrell, M. S., Buckley, D., and Baker, C. V. H. (2011b). Molecular analysis of neurogenic placode development in a basal ray-finned fish. genesis 49, 278–294. doi:10.1002/dvg.20707
Modrell, M. S., Lyne, M., Carr, A. R., Zakon, H. H., Buckley, D., Campbell, A. S., et al. (2017a). Insights into electrosensory organ development, physiology and evolution from a lateral line-enriched transcriptome. eLife 6, e24197. doi:10.7554/eLife.24197
Modrell, M. S., Tidswell, O. R. A., and Baker, C. V. H. (2017b). Notch and Fgf signaling during electrosensory versus mechanosensory lateral line organ development in a non-teleost ray-finned fish. Dev. Biol. 431, 48–58. doi:10.1016/j.ydbio.2017.08.017
Mogdans, J. (2019). Sensory ecology of the fish lateral-line system: morphological and physiological adaptations for the perception of hydrodynamic stimuli. J. Fish Biol. 95, 53–72. doi:10.1111/jfb.13966
Moser, T., Grabner, C. P., and Schmitz, F. (2020). Sensory processing at ribbon synapses in the retina and the cochlea. Physiol. Rev. 100, 103–144. doi:10.1152/physrev.00026.2018
Norris, H. W., and Hughes, S. P. (1920). The spiracular sense-organ in elasmobranchs, ganoids and dipnoans. Anat. Rec. 18, 205–209. doi:10.1002/ar.1090180210
Northcutt, R. G. (1997). Evolution of gnathostome lateral line ontogenies. Brain Behav. Evol. 50, 25–37. doi:10.1159/000113319
Northcutt, R. G., Brändle, K., and Fritzsch, B. (1995). Electroreceptors and mechanosensory lateral line organs arise from single placodes in axolotls. Dev. Biol. 168, 358–373. doi:10.1006/dbio.1995.1086
Northcutt, R. G., Catania, K. C., and Criley, B. B. (1994). Development of lateral line organs in the axolotl. J. Comp. Neurol. 340, 480–514. doi:10.1002/cne.903400404
O’Donnell, M., Hong, C.-S., Huang, X., Delnicki, R. J., and Saint-Jeannet, J.-P. (2006). Functional analysis of Sox8 during neural crest development in Xenopus. Development 133, 3817–3826. doi:10.1242/dev.02558
Ó Maoiléidigh, D., and Ricci, A. J. (2019). A bundle of mechanisms: inner-ear hair-cell mechanotransduction. Trends Neurosci. 42, 221–236. doi:10.1016/j.tins.2018.12.006
O’Neill, P., McCole, R. B., and Baker, C. V. H. (2007). A molecular analysis of neurogenic placode and cranial sensory ganglion development in the shark, Scyliorhinus canicula. Dev. Biol. 304, 156–181. doi:10.1016/j.ydbio.2006.12.029
Pangrsic, T., Singer, J. H., and Koschak, A. (2018). Voltage-gated calcium channels: key players in sensory coding in the retina and the inner ear. Physiol. Rev. 98, 2063–2096. doi:10.1152/physrev.00030.2017
Papalopulu, N., and Kintner, C. (1996). A posteriorising factor, retinoic acid, reveals that anteroposterior patterning controls the timing of neuronal differentiation in Xenopus neuroectoderm. Development 122, 3409–3418. doi:10.1242/dev.122.11.3409
Pauley, S., Lai, E., and Fritzsch, B. (2006). Foxg1 is required for morphogenesis and histogenesis of the mammalian inner ear. Dev. Dyn. 235, 2470–2482. doi:10.1002/dvdy.20839
Perdigoto, C. N., Bardot, E. S., Valdes, V. J., Santoriello, F. J., and Ezhkova, E. (2014). Embryonic maturation of epidermal Merkel cells is controlled by a redundant transcription factor network. Development 141, 4690–4696. doi:10.1242/dev.112169
Piotrowski, T., and Baker, C. V. H. (2014). The development of lateral line placodes: taking a broader view. Dev. Biol. 389, 68–81. doi:10.1016/j.ydbio.2014.02.016
Radde-Gallwitz, K., Pan, L., Gan, L., Lin, X., Segil, N., and Chen, P. (2004). Expression of Islet1 marks the sensory and neuronal lineages in the mammalian inner ear. J. Comp. Neurol. 477, 412–421. doi:10.1002/cne.20257
Reilly, M. B., Cros, C., Varol, E., Yemini, E., and Hobert, O. (2020). Unique homeobox codes delineate all the neuron classes of C. elegans. Nature 584, 595–601. doi:10.1038/s41586-020-2618-9
Romero-Carvajal, A., Navajas Acedo, J., Jiang, L., Kozlovskaja-Gumbriene, A., Alexander, R., Li, H., et al. (2015). Regeneration of sensory hair cells requires localized interactions between the Notch and Wnt pathways. Dev. Cell 34, 267–282. doi:10.1016/j.devcel.2015.05.025
Saito, T., and Psenicka, M. (2015). Novel technique for visualizing primordial germ cells in sturgeons (Acipenser ruthenus, A. gueldenstaedtii, A. baerii, and Huso huso). Biol. Reprod. 93, 96. doi:10.1095/biolreprod.115.128314
Sapède, D., Gompel, N., Dambly-Chaudière, C., and Ghysen, A. (2002). Cell migration in the postembryonic development of the fish lateral line. Development 129, 605–615. doi:10.1242/dev.129.3.605
Schulz, M. H., Zerbino, D. R., Vingron, M., and Birney, E. (2012). Oases: robust de novo RNA-seq assembly across the dynamic range of expression levels. Bioinformatics 28, 1086–1092. doi:10.1093/bioinformatics/bts094
Stehlin-Gaon, C., Willmann, D., Zeyer, D., Sanglier, S., Van Dorsselaer, A., Renaud, J.-P., et al. (2003). All-trans retinoic acid is a ligand for the orphan nuclear receptor ROR beta. Nat. Struct. Biol. 10, 820–825. doi:10.1038/nsb979
Stöver, B. C., and Müller, K. F. (2010). TreeGraph 2: combining and visualizing evidence from different phylogenetic analyses. BMC Bioinformatics 11, 7. doi:10.1186/1471-2105-11-7
Stundl, J., Pospisilova, A., Matějková, T., Psenicka, M., Bronner, M. E., and Cerny, R. (2020). Migratory patterns and evolutionary plasticity of cranial neural crest cells in ray-finned fishes. Dev. Biol. 467, 14–29. doi:10.1016/j.ydbio.2020.08.007
Stundl, J., Soukup, V., Franěk, R., Pospisilova, A., Psutkova, V., Pšenička, M., et al. (2022). Efficient CRISPR mutagenesis in sturgeon demonstrates its utility in large, slow-maturing vertebrates. Front. Cell Dev. Biol. 10, 750833. doi:10.3389/fcell.2022.750833
Stundl, J., Martik, M. L., Chen, D., Raja, D. A., Franěk, R., Pospisilova, A., et al. (2023). Ancient vertebrate dermal armor evolved from trunk neural crest. Proc. Natl. Acad. Sci. U.S.A. 120, e2221120120. doi:10.1073/pnas.2221120120
Sun, Y., Dykes, I. M., Liang, X., Eng, S. R., Evans, S. M., and Turner, E. E. (2008). A central role for Islet1 in sensory neuron development linking sensory and spinal gene regulatory programs. Nat. Neurosci. 11, 1283–1293. doi:10.1038/nn.2209
Sun, Y., and Liu, Z. (2023). Recent advances in molecular studies on cochlear development and regeneration. Curr. Opin. Neurobiol. 81, 102745. doi:10.1016/j.conb.2023.102745
Tasdemir-Yilmaz, O. E., Druckenbrod, N. R., Olukoya, O. O., Dong, W., Yung, A. R., Bastille, I., et al. (2021). Diversity of developing peripheral glia revealed by single-cell RNA sequencing. Dev. Cell 56, 2516–2535.e8. doi:10.1016/j.devcel.2021.08.005
Toresson, H., Martinez-Barbera, J. P., Bardsley, A., Caubit, X., and Krauss, S. (1998). Conservation of BF-1 expression in amphioxus and zebrafish suggests evolutionary ancestry of anterior cell types that contribute to the vertebrate telencephalon. Dev. Genes Evol. 208, 431–439. doi:10.1007/s004270050200
Undurraga, C. A., Gou, Y., Sandoval, P. C., Nuñez, V. A., Allende, M. L., Riley, B. B., et al. (2019). Sox2 and Sox3 are essential for development and regeneration of the zebrafish lateral line. bioRxiv 856088. doi:10.1101/856088
Vidal, B., Gulez, B., Cao, W. X., Leyva-Díaz, E., Reilly, M. B., Tekieli, T., et al. (2022). The enteric nervous system of the C. elegans pharynx is specified by the Sine oculis-like homeobox gene ceh-34. eLife 11, e76003. doi:10.7554/eLife.76003
votn Bartheld, C. S., and Giannessi, F. (2011). The paratympanic organ: a barometer and altimeter in the middle ear of birds? J. Exp. Zool. B Mol. Dev. Evol. 316, 402–408. doi:10.1002/jez.b.21422
Wang, J., Lu, C., Zhao, Y., Tang, Z., Song, J., and Fan, C. (2020). Transcriptome profiles of sturgeon lateral line electroreceptor and mechanoreceptor during regeneration. BMC Genomics 21, 875. doi:10.1186/s12864-020-07293-4
Wang, X., Llamas, J., Trecek, T., Shi, T., Tao, L., Makmura, W., et al. (2023). SoxC transcription factors shape the epigenetic landscape to establish competence for sensory differentiation in the mammalian organ of Corti. Proc. Natl. Acad. Sci. U.S.A. 120, e2301301120. doi:10.1073/pnas.2301301120
Webb, J. F. (2021). “Morphology of the mechanosensory lateral line system of fishes,” in The Senses: A Comprehensive Reference. Editor B. Fritzsch (Elsevier), 29–46. doi:10.1016/B978-0-12-809324-5.24162-X
Wiwatpanit, T., Lorenzen, S. M., Cantú, J. A., Foo, C. Z., Hogan, A. K., Márquez, F., et al. (2018). Trans-differentiation of outer hair cells into inner hair cells in the absence of INSM1. Nature 563, 691–695. doi:10.1038/s41586-018-0570-8
Wullimann, M. F., and Grothe, B. (2014). “The central nervous organization of the lateral line system,” in The Lateral Line System. Editors S. C. Coombs, H. Bleckmann, R. R. Fay, and A. N. Popper (New York: Springer), 195–251. Available at: https://link.springer.com/chapter/10.1007/2506_2013_18.
Xu, J., Li, J., Zhang, T., Jiang, H., Ramakrishnan, A., Fritzsch, B., et al. (2021). Chromatin remodelers and lineage-specific factors interact to target enhancers to establish proneurosensory fate within otic ectoderm. Proc. Natl. Acad. Sci. U.S.A. 118, e2025196118. doi:10.1073/pnas.2025196118
Yamashita, T., Zheng, F., Finkelstein, D., Kellard, Z., Robert, C., Rosencrance, C. D., et al. (2018). High-resolution transcriptional dissection of in vivo Atoh1-mediated hair cell conversion in mature cochleae identifies Isl1 as a co-reprogramming factor. PLoS Genet. 14, e1007552. doi:10.1371/journal.pgen.1007552
Zeiske, E., Kasumyan, A., Bartsch, P., and Hansen, A. (2003). Early development of the olfactory organ in sturgeons of the genus Acipenser: a comparative and electron microscopic study. Anat. Embryol. 206, 357–372. doi:10.1007/s00429-003-0309-6
Zerbino, D. R., and Birney, E. (2008). Velvet: algorithms for de novo short read assembly using de Bruijn graphs. Genome Res. 18, 821–829. doi:10.1101/gr.074492.107
Zhang, S., Zhang, Y., Dong, Y., Guo, L., Zhang, Z., Shao, B., et al. (2019). Knockdown of Foxg1 in supporting cells increases the trans-differentiation of supporting cells into hair cells in the neonatal mouse cochlea. Cell. Mol. Life Sci. 77, 1401–1419. doi:10.1007/s00018-019-03291-2
Zhang, Y., Zhang, S., Zhang, Z., Dong, Y., Ma, X., Qiang, R., et al. (2020). Knockdown of Foxg1 in Sox9+ supporting cells increases the trans-differentiation of supporting cells into hair cells in the neonatal mouse utricle. Aging (Albany NY) 12, 19834–19851. doi:10.18632/aging.104009
Zhao, X.-F., Suh, C. S., Prat, C. R., Ellingsen, S., and Fjose, A. (2009). Distinct expression of two foxg1 paralogues in zebrafish. Gene Expr. Patterns 9, 266–272. doi:10.1016/j.gep.2009.04.001
Keywords: lateral line organs, electrosensory, mechanosensory, ampullary organ, neuromast, paddlefish, sturgeon, sterlet
Citation: Minařík M, Modrell MS, Gillis JA, Campbell AS, Fuller I, Lyne R, Micklem G, Gela D, Pšenička M and Baker CVH (2024) Identification of multiple transcription factor genes potentially involved in the development of electrosensory versus mechanosensory lateral line organs. Front. Cell Dev. Biol. 12:1327924. doi: 10.3389/fcell.2024.1327924
Received: 25 October 2023; Accepted: 19 February 2024;
Published: 18 March 2024.
Edited by:
David Ellard Keith Ferrier, University of St Andrews, United KingdomReviewed by:
Andy Groves, Baylor College of Medicine, United StatesBernd Fritzsch, University of Nebraska Medical Center, United States
Copyright © 2024 Minařík, Modrell, Gillis, Campbell, Fuller, Lyne, Micklem, Gela, Pšenička and Baker. This is an open-access article distributed under the terms of the Creative Commons Attribution License (CC BY). The use, distribution or reproduction in other forums is permitted, provided the original author(s) and the copyright owner(s) are credited and that the original publication in this journal is cited, in accordance with accepted academic practice. No use, distribution or reproduction is permitted which does not comply with these terms.
*Correspondence: Clare V. H. Baker, cvhb1@cam.ac.uk