Outline of microbial fuel cells technology and their significant developments, challenges, and prospects of oxygen reduction electrocatalysts
- 1Department of Chemistry, N.K.R. Government Arts College for Women, Namakkal, India
- 2Department of Physical-Chemistry, Faculty of Chemical Sciences, University of Concepcion, Concepción, Chile
- 3Electrochemical Technologies and Green Energies Laboratory, Department of Materials Engineering, Faculty of Engineering, University of Concepcion, Concepción, Chile
- 4Petroleum and Chemical Engineering Programme Area, Faculty of Engineering, Universiti Teknologi Brunei, Gadong, Brunei
- 5Faculty of Chemical and Process Engineering Technology, Universiti Malaysia Pahang Al-Sultan Abdullah, Gambang, Malaysia
- 6Department of Civil and Environmental Engineering, Faculty of Engineering, Prince of Songkla University, Hat Yai, Songkhla, Thailand
- 7Faculty of Engineering and Sciences, Adolfo Ibáñez University, Santiago, Chile
The microbial fuel cells (MFCs) which demonstrates simultaneous production of electricity and wastewater treatment have been considered as one of the potential and greener energy production technology among the available bioelectrochemical systems. The air-cathode MFCs have gained additional benefits due to using air and avoiding any chemical substances as catholyte in the cathode chamber. The sluggish oxygen reduction reaction (ORR) kinetics at the cathode is one of the main obstacles to achieve high microbial fuel cell (MFC) performances. Platinum (Pt) is one of the most widely used efficient ORR electrocatalysts due to its high efficient and more stable in acidic media. Because of the high cost and easily poisoned nature of Pt, several attempts, such as a combination of Pt with other materials, and using non-precious metals and non-metals based electrocatalysts has been demonstrated. However, the efficient practical application of the MFC technology is not yet achieved mainly due to the slow ORR. Therefore, the review which draws attention to develop and choosing the suitable cathode materials should be urgent for the practical applications of the MFCs. In this review article, we present an overview of the present MFC technology, then some significant advancements of ORR electrocatalysts such as precious metals-based catalysts (very briefly), non-precious metals-based, non-metals and carbon-based, and biocatalysts with some significant remarks on the corresponding results for the MFC applications. Lastly, we also discussed the challenges and prospects of ORR electrocatalysts for the practical application of MFCs.
1 Introduction
The shrinking of non-renewable energy sources and environmental pollution are the main critical issues the world has been facing in recent years. Therefore, the research area of energy generation by the alternative renewable sources is increasing drastically. Converting organic or inorganic waste materials into useful products and energy can better address energy and environmental problems (Babanova et al., 2022; Díaz-Vázquez et al., 2022; Gargalo et al., 2022; Kamali et al., 2023). Bioelectrochemical systems (BES) can be an effective potential candidate for the waste materials conversion and energy production (Agudelo-Escobar et al., 2022). BES is one of the emerging bioengineering technologies which show the process of electrochemical reactions, such as the conversion of chemical energy into electrical energy or vice versa, in the presence of microorganisms or biomaterials (Zheng et al., 2020). In the BES, the microorganism can exchange electrons with the electrodes directly or indirectly via a chemical compound that acts as an electron carrier (E. Logan et al., 2006). The BES can be categorized based on the task as (i) microbial fuel cells (MFCs), (ii) plant MFCs (P-MFC), (iii) constructed wetlands MFCs (CW-MFC) (iv) benthic MFCs (B-MFC), (v) microbial electrolysis cells (MEC), (vi) anaerobic digestion coupled MEC (AD-MEC), (vii) microbial electrosynthesis cells (MES), (viii) microbial desalination cells (MDC), and (ix) microbial electro-Fenton (MEF) (Zou and He, 2018; Mier et al., 2021). MFCs and P-MFC will generate electricity from an organic substrate using microorganisms and living plants with microorganisms, respectively as a catalyst. In the CW-MFC, the electricity generation and wastewater treatment occurs in MFCs integrated with constructed wetlands. In B-MFC, the electricity will be generated using sea’s inorganic and organic matter in the presence of bacterial catalysis. In MEC, the hydrogen (H2) can be produced in its cathode part by reducing H+ using small external voltage and the ammonia recovery from wastewater. By reducing carbon dioxide, the MES can generate value-added chemical products, such as formic acid, acetate, ethanol, and butanol. Besides, methane can be produced by the degradation of a substrate from the AD-MEC system. The MDC produces fresh water from seawater or brackish water with the help of self-produced electricity or applying the external electricity (Wang and Ren, 2013). In MEF, the electricity will be generated at the anode microbial chamber, and H2O2 will be generated at the cathode chamber (Mier et al., 2021). The BES has several advantages, such as the clean process, lower operational cost, flux of electrons, and the energy level can be adjusted and maintained constant. The electrical signal can be used for monitoring and high selectivity towards the target compounds. Besides, there are disadvantages of BES, such as the challenge of scale-up, the cathodic reaction in MFC may limit the anodic reaction, pH changes of the system may affect the electrochemical process and the formation of chlorine gas from marine environment (Daghio et al., 2017).
Among the above bioelectrochemical systems, microbial fuel cell (MFC) is considered one of the potential renewable energy devices where microorganisms used as biocatalysts to convert organic substrates into electricity (E. Logan et al., 2006; Slate et al., 2019; Priya et al., 2022; Abubackar et al., 2023). An MFC requires about three times less potential difference compared to a conventional electrochemical cell for the H2 production (Rozendal et al., 2006). The simultaneous treatment of wastewater during the electricity generation and the removal of metals such as chromium, copper, nickel, and zinc has gained additional importance regarding the environmental benefits (Nancharaiah et al., 2015; Hidayat et al., 2022). There are several review articles available very recently which include the focusing on functional materials (Zhu Q. et al., 2022), graphene-based materials (Aiswaria et al., 2022), carbonaceous materials (Dhilllon et al., 2022), nanomaterials (Chen et al., 2022b; Kamali et al., 2022; Dey et al., 2023; Kausar et al., 2023), anodic modification materials (Ma et al., 2023), and different types of MFCs (Gupta et al., 2023). Because the sluggish oxygen reduction reaction (ORR) kinetics is one of the main obstacles, the review which draws attention to choosing the suitable cathode materials should be urgent for the practical applications of the MFC technology. Therefore, here we present an overview of the present MFC technology, then some significant advancements of ORR electrocatalysts such as precious metals-based catalysts (very briefly), non-precious metals-based, non-metals and carbon-based, and biocatalysts with some significant remarks on the corresponding results for the MFC applications. Lastly, we also discussed the challenges and prospects of ORR electrocatalysts for the practical application of MFCs.
2 Microbial fuel cells (MFCs)
In the early 20th century, Potter reported the first bioelectrochemical reaction to generate electricity with some live microbial cultures such as Escherichia coli and Saccharomyces spp. and Pt macro-electrodes in a battery style system (Potter M. C., 1911). Later in 1931, Cohen confirmed this reaction by generating 0.2 mA current and 35 V voltage with a stacked bacterial fuel cell arrangement (Cohen, 1931). Interestingly after a few years, in 1963, electricity generated using human waste during space flight was demonstrated by the National Aeronautics and Space Administration (NASA) space program (Canfield et al., 1963; Slate et al., 2019). Recently, the MFC technology has been one of the best emerging eco-friendly research areas, as can be witnessed from the extensive reports (Gude, 2016; Zhang Y. et al., 2019; Kaur et al., 2020; Huang et al., 2021; Munoz-Cupa et al., 2021). A typical MFC contains anodic and cathodic chambers, which are separated by a proton exchange membrane (PEM). In the anodic chamber, microbes degrade the organic substances and produce electrons, protons (H+), and carbon dioxide (CO2). Then, the produced electrons and H+ are transported through an external circuit and PEM, respectively to the cathodic chamber and react with oxygen (O2) to form water.
Typical electrode reactions of MFC with acetic acid (CH3COOH) as a model organic substance are shown below:
The potentials of −0.300, 0.805, and 1.105 V (vs. NHE) correspond to the anode, cathode, and the cell, respectively, for the production of electricity from the above electrochemical cell reaction (Obileke et al., 2021).
The main classification of MFC includes double-chamber MFC, single-chamber MFC, and stacked MFC. The double chamber MFC will consist of two chambers separated by a cation exchange membrane (CEM) to separate the different electrolytes in the compartments and only to allow H+. The distance between the two electrodes and the small surface area of the membrane causes high internal resistance of these types of MFCs, hence limiting the output power density. Chemical substances like permanganate, ferricyanides, and dichromates act as oxidizing agents, and no catalysts are needed for cathodic reactions in double-chamber MFCs (Yu et al., 2017; Fang and Achal, 2019; Hidayat et al., 2022). Double-chamber MFCs are suitable for laboratory research as these are run in batch mode. Different double-chamber MFCs are available such as H-type MFC, cube-type MFC, flate-type MFC, tubular upflow MFC, and miniature MFC, which have decreased internal resistance and therefore increasing power generation(Kun et al., 2012). In a single chamber MFC, the cathode can be in direct contact with air and with the presence or absence of a membrane(Logan and Regan, 2006). The anode and cathode in a single-chamber MFCs are separated by a PEM in a single compartment and it is not necessary that the cathode part have to be filled with catholyte (electrolyte on the cathode part) when O2 was used at the cathode. Single-chamber MFC is also called air-cathode MFC since the cathode is directly exposed to air. The single chamber MFC has emerged into several variants, such as cube-type MFC (Liu and Logan, 2004), horizontal tube-type MFC (Wang and Ren, 2013), side-arm bottle MFC (Logan et al., 2007), and upflow-type MFC (You et al., 2007). The single-chamber MFCs have several advantages over the double-chamber MFCs, such as cost-effective, simple construction, sustainable sources, and better performance. Directly harvesting electricity from the biodegradable organic substances using a single MFC is lower. This problem can be addressed by using another type of MFC called stacked MFCs, in which the MFCs are stacked in series or parallel or a combination of series and parallel (Aelterman et al., 2006; Oh and Logan, 2007; An et al., 2015; Santoro et al., 2019; Dziegielowski et al., 2021; Mukherjee et al., 2022). There are 4-module sedimentMFC (Prasad and Tripathi, 2021), 6-cell stacked MFC (Aelterman et al., 2006), bipolar type of stacked MFC (Shin et al., 2006), stacked MFCs bridged internally through an extra CEM (Liu et al., 2008) and the MFC stack assembled from two single MFCs (Oh and Logan, 2007).
3 Air-cathode MFC
The single-chamber or air-cathode MFCs demonstrated higher efficiency than double-chamber MFCs. However, the efficiency of air-cathode MFCs can be fine-tuned by controlling many factors such as substrates, inoculums, electrodes materials, PEMs, and operating conditions (Bagchi and Behera, 2019; Merino-Jimenez et al., 2019; Vilas Boas et al., 2019). The structures and the types of single-chamber MFCs were discussed earlier in Section 2.
3.1 Limitations of air-cathode MFCs performances
The limitations of the MFC technology for the industrial and social applications include using high-cost materials such as electrodes and proton exchange membranes (PEMs), low life spans, and low energy outputs.
3.1.1 Thermodynamic factors
Generally, the cathodic reactions take place in the aerobic or anaerobic conditions. The anaerobic cathodic reactions will have occurred in double-chamber MFCs where a chemical substance acts as an oxidizing agent. The aerobic condition usually occurs in the cathodic part of air-cathode MFCs. The potential generated from an MFC can be thermodynamically derived using the Nernst equation (Eq. 4) given below (Rismani-Yazdi et al., 2008),
Where,
The potential of an ideal MFC is always higher than the actual MFC because of irreversible losses such as activation losses, ohmic losses, and transport losses (Rabaey and Verstraete, 2005). The potential required for redox (oxidation-reduction) reactions to occur is the activation loss, also known as activation over potential. The overpotential is a limiting step, and this can be reduced by increasing operating temperature, efficient electrocatalysts, electrode surface area, the concentration of redox shuttles, etc. The electrical resistance between anode and cathode, solution and electrode interfaces, and the electrolyte and membrane interfaces cause ohmic losses. Ohmic losses can be avoided by using appropriate electrolyte and electrode materials which are having high electrical conductivity. Generally, at high current densities, mass transport losses occur due to the limited mass transport of species from or to the electrode. This limited mass transport causes product depletion or accumulation. These losses can be minimized by maintaining high bulk concentrations and distribution of oxidants such as O2 at the cathode compartment (Oguz Koroglu et al., 2019).
3.1.2 Other factors
In addition to the above thermodynamic factors, several other factors such as biofouling, catalyst inactivation (if existing), and excessive biofilm growth are the main obstacles to the real-world applications of the technology (Sun M. et al., 2016; Li et al., 2023). The factors such as microbial electron transfer, supply of oxygen, fuel oxidation, circuit resistance, proton transfer via the PEM, pH, concentration, and reduction at the cathode influenced the MFCs’ performances (Woodward et al., 2010; Jatoi et al., 2021). The crossover of electron acceptors or organic compounds from the cathode compartment to the anode compartment and vice versa also decreases the efficiency of the MFCs (Harnisch et al., 2009; Winfield et al., 2013). The biofouling involves the generation of insulating materials such as polymeric and/or dead cells, which can separate the dynamic biofilm from the surface of the electrode or blockage the porous electrode surface. This results in the reduction of efficiently active sites of the electrodes and ultimately decrease the overall performance of MFCs (Amirul Islam et al., 2016; Sun M. et al., 2016; Blanchet et al., 2016).
4 Mechanisms of oxygen reduction reaction (ORR)
The ORR is an essential reaction in many systems, such as biological respiration, fuel cells, and metal-air batteries. In an aqueous solution, the ORR occurs mainly by two pathways which are a) direct four-electron transfer from O2 to H2O (Equations 5–9) and b) the two-electron transfer from O2 to hydrogen peroxide (H2O2) (Equations 10–12). The one-electron transfer pathway from O2 to superoxide (O2-) could also occur in alkaline solutions and/or in non-aqueous aprotic solvents (Oguz Koroglu et al., 2019).




The ORR occurs in the cathodic part under ambient temperature and pH, involving complicated interfacial electron and mass transfer processes. The kinetics of the ORR is generally sluggish and result in a high overpotential at the cathode which decreases the MFC efficiency (Gao et al., 2020). Platinum (Pt) is one of the most widely used and the efficient ORR electrocatalysts. The ORR can occur through three possible mechanistic pathways such as dissociation of O2, dissociation of OOH, and dissociation of H2O2, as shown in Figure 1 (Haile et al., 2020). The ORR in the presence of a Pt catalyst takes place predominately through a four-electron transfer pathway as shown below (Equations 13–17) (Si et al., 2014),
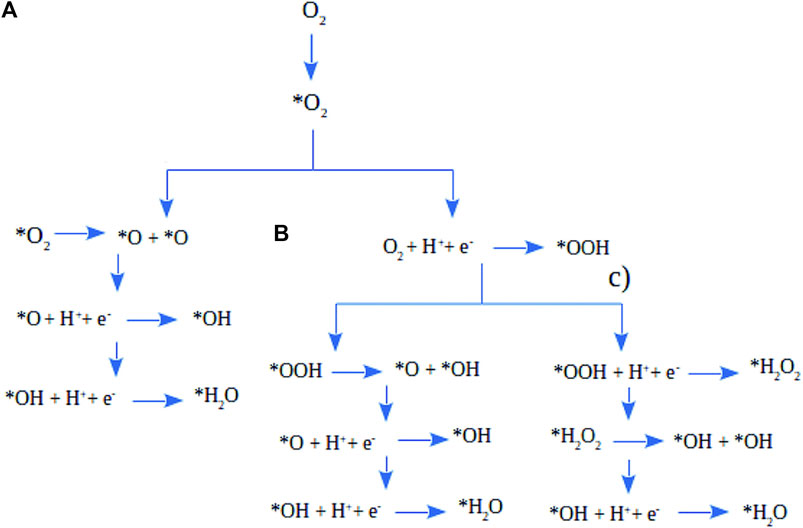
FIGURE 1. Possible mechanisms of ORR: (A) dissociation of O2, (B) dissociation of OOH, and (C) dissociation of H2O2 (Haile et al., 2020).
In the case of other electrocatalysts such as carbon based, transition metal oxides and hybrid nanomaterials may follow two-electron transfer or a combination of two- and four-electron transfer pathways. The pH (acidic or basic) also influences the ORR pathway as given below (Equations 18–25), In acidic pH,
In basic pH,
The formation of OH− in the basic media accumulated at the cathode may result in a lower kinetic performance of ORR. Therefore, the effective elimination of OH− at the catalyst active sites is a central challenge in the ORR. Besides, the functional groups on the surface of the catalyst (if present) can assist in the elimination of OH− for ease of ORR (Hou et al., 2016). The electrocatalysts’ performance for the ORR is generally studied by rotating ring disc electrode (RRDE). The Koutecky-Levich equation can be used to measure the number of electron transfers in ORR as given below (Eq. 26),
Where j, jk and ɷ are the total current density, kinetic current density, and electrode rotation rate (rpm) respectively. The coefficient, B, can be obtained from the Levich equation (Eq. 27) and the slope of the Koutecky-Levich plots.
Where n, F, DO2, ν, and C O2 are the number of electron transfer per O2 molecule, Faraday constant (96,485 C/mol), the diffusion coefficient of O2 in 0.1 M KOH (1.9 × 10−5 cm2/s), kinetic viscosity (0.01 cm2/s) and bulk concentration of O2 (1.2 × 10−6 mol/cm3) respectively. The constant 0.2 in the above equation is assumed when the rotation speed is mentioned in rpm (Gautam and Verma, 2019).
5 Importance of ORR electrocatalysts
However, the infinite source of O2 makes the air-cathode MFCs a potential eco-friendly technology; incomplete reduction of O2 at the cathodic part produces some destructive reactive intermediates and free radicals. The performance of the MFC for the current generation depends on the ORR at the surface of the cathode, which is restricted by the activation barrier (Sawant et al., 2017; Liu et al., 2022). The large activation energy loss due to the sluggish ORR results in high overpotential at the cathode. The use of mediators, optimization of MFC operating conditions, and cathode modification with catalysts are adopted to enhance the ORR kinetics. Among these, using the ORR electrocatalysts is the most feasible way to facilitate the fast ORR kinetics (Kamali et al., 2022). The ORR electrocatalyst should possess excellent catalytic activity (including good electrical conductivity, high surface area, functional groups, and surface morphology), cost-effectiveness, and higher stability. Pt has been proven to be the most widely used conventional electrocatalyst for ORR activity due to the superior activity, and highly stable in acidic media when compared to other non-precious ORR electrocatalysts (Sui et al., 2017; Ma et al., 2020). The high cost and easily poisoned by anions such as carbon monoxide and sulphide limits its large-scale applications (Chaturvedi and Kundu, 2021). The fabrication cost of a Pt cathode is more than half of the total cost of a lab-scale MFC. Therefore, the research on cost-effective, efficient ORR electrocatalysts has become an attractive and vital research field (Chaturvedi and Kundu, 2021; Peera et al., 2021). There are several types of electrocatalysts have been reported to decrease and/or to replace the usage of Pt for the ORR activity which include Pt-based, non-precious metals-based, and carbon-based electrocatalysts (Chaturvedi and Kundu, 2021; Peera et al., 2021; Priyadarshini et al., 2021; Kamali et al., 2022).
6 Types of ORR electrocatalysts
The types of ORR electrocatalysts can be broadly categorized as (i) precious metals-based ORR electrocatalysts, (ii) non-precious metals-based ORR electrocatalysts, (iii) non-metals and/or carbon based ORR electrocatalysts and (iv) biocatalysts.
6.1 Precious metals-based ORR electrocatalysts
Due to the high-cost nature and aim toward the practical applications of the MFC technology, the precious metal-based catalysts are reviewed very briefly in this section. Pt is the most widely used and potential electrocatalyst for ORR applications. The Pt loaded on carbon cathode in MFC generated a maximum of 1,553 mW cm-2 power with simultaneously treating piggery waste (Chandrasekhar and Ahn, 2017). The carbon paper electrode contains lower loading of Pt fabricated using an e-beam evaporation technique performed 2.5 times higher than the commercial Pt catalyst in MFC applications (Park et al., 2007). Similarly, the lower loading of Pt on carbon cloth using electrodeposition showed superior performances in the MFC applications (Yen et al., 2013). Zerrouki et al. demonstrated the Pt-PANI composite catalyst showed better ORR activity and a maximum power density of 1,510 mW cm-2 with 88% of COD removal efficiency in MFC. The enhanced activity was ascribed to the presence of conducting polymer PANI, which enhanced the electron cloud on the catalyst (Zerrouki et al., 2022). The Pt-boron-nitride-carbon electrocatalyst recently showed superior stable MFC performances over 2 months with 936.31 mW cm-2 of maximum power density. The superior performance was due to the availability of more and uniformly dispersed active sites as well as the strong coordination structure of Pt-N4 (Shixuan et al., 2023).
The palladium (Pd) on the Si nanowire exhibited 84.5% methyl orange degradation with simultaneous generation of 0.119 W/m2 of maximum output power density (Han et al., 2017). The Pd supported on stainless steel fiber felt prepared by simple water bath method having macropores showed a higher power density of 390.79 mW m−2, comparable to the efficiency of conventional Pt/C ORR electrocatalyst (405.47 mW m−2) (Chen et al., 2019). Wang et al. demonstrated around 1.8 times enhanced MFC performance of 901 mW m-2 maximum power density using Pd/GO-C catalyst than the other Pd catalysts. They used a novel method to prepare the Pd/GO-C catalyst by simply mixing GO, carbon block, and PdCl2 as shown in Figure 2 (Wang et al., 2021). Remarkably, beyond 100 days, the MFC performance was demonstrated using sub-5nm Pd nanocrystals in FeN3-Pd@NC NBs composite ORR catalyst. The active sites of Fe and Pd, and the structural properties of the catalyst resulted the higher MFC performances (Lin et al., 2022). The silver (Ag) based electrocatalysts show the good performances in the MFC applications (Dai et al., 2017). Ag2O/Ag cathode showed stable maximum power output of 1.796 W m-3 in MFC applications (Dai et al., 2017). The Ag performance in MFC was also enhanced by hybridizing with tungsten carbide, which showed better efficiency than the commercial Pt/C electrocatalyst (Gong et al., 2013). The Ag−Fe−N/C ORR catalyst derived from a zeolitic imidazole framework displayed a higher power density of 523 mW m−2 than the commercial Pt/C (358 mW m−2) owing to the synergistic effects of Ag nanoparticles, Fe and N-doped porous carbon (Lai et al., 2022). Recently, Sun et al. reported CNFs-Ag/Fe based catalyst derived using metal organic framework material showed a higher maximum power density of 737.45 mW m−2 than the commercial Pt/C (457.99 mW m−2), which was due to the incorporation of Fe in the catalyst (Sun et al., 2023).
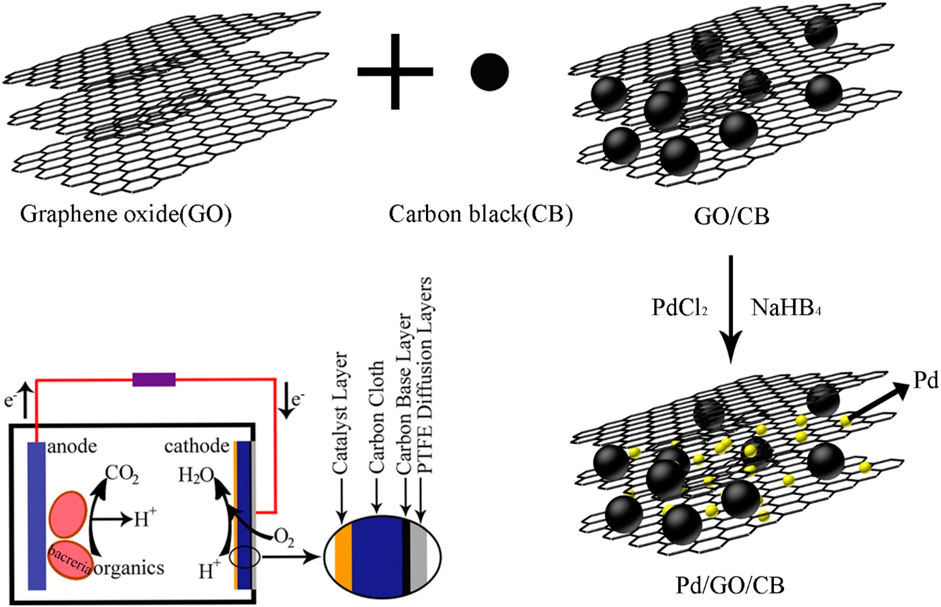
FIGURE 2. Schematic diagram of the preparation and application of Pd/GO-C in microbial fuel cells (MFCs) (Wang et al., 2021).
The combination of different transition and/or non-precious metals with Pt and forming alloys provides high surface area by decreasing particle size and, thus, more active sites for ORR in MFCs. The Pt-Co alloy supported on carbon demonstrated more elevated and more stable MFC performances when compared with the commercial Pt/C ORR electrocatalyst due to the presence of Co with optimized composition (Yan et al., 2014b). The Pt-Ni alloy showed higher power generation efficiency of 0.637 W m−2 in MFC than that of the Pt cathode of 0.180 W m−2 due to its increased oxygen adsorption and reduction on the more active sites (Cetinkaya et al., 2015). The optimized Pt-Pd alloy coated on carbon paper via the electrodeposition method demonstrated 1,274 m Wm−2 of maximum power density comparable to that of commercial Pt/C catalyst in air-cathode MFC application (Quan et al., 2015). The PtSn/C ORR electrocatalyst showed a maximum power density of 336 mW m−2 in MFC application, while the commercial Pt/C catalyst showed lower efficiency of 307 mW m−2. The modification of the electronic structure of the Pt by the introduction of Sn enhanced the MFC performances of the catalyst (Li et al., 2017). The Pt3-Fe/C ORR electrocatalyst showed a 18% enhanced performance of 1,680 mW m−2 power generation with outstanding durability compared with the commercial Pt/C (1,422 mW m−2) in MFC application (Yan et al., 2014a). The Pt coated onto carbon paper (CP) as cathode generated the maximum power density of 84.01 mW/m2 in the MFC, which is approximately two times higher than neat CP (44.7 mW/m2). The performance of the MFC was then increased to about 147 mW/m2 by incorporating carbon nanotube (CNT) with Pt electrocatalyst, which was attributed to the high surface area of the CNT(Halakoo et al., 2015). The summary of maximum power density, wastewater, and cell configuration with significant remarks corresponding to a few essential precious metals-based ORR electrocatalysts for the MFC applications are given in Table 1.
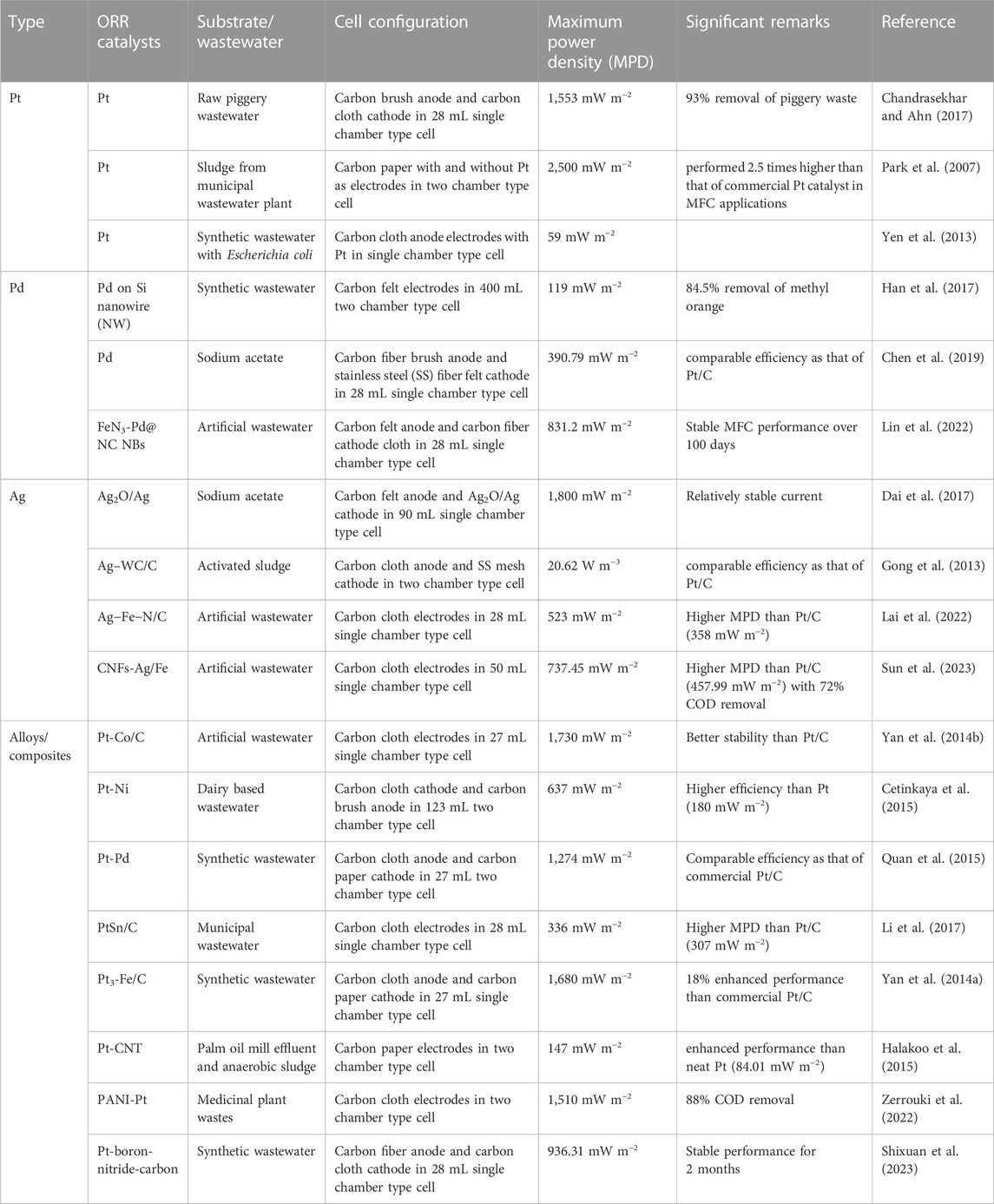
TABLE 1. Summary of substrates or wastewater, cell configuration, and maximum power density with significant remarks corresponds to a few essential precious metals-based ORR electrocatalysts for the MFC applications.
6.2 Non-precious metals-based ORR electrocatalysts
Several non-precious metals-based materials have been studied as the ORR electrocatalysts in air-cathode MFC. The main concern of using non-precious metals is to reduce the cost of electrocatalysts and aim for the future potential of scaling-up applications. The transition metal compounds were found to have higher activity as ORR electrocatalysts. Plentiful research articles displayed that properly designed non-precious metal-based ORR electrocatalysts can perform comparable to, or even higher than, the commercial Pt/C in MFC applications with high stability. The summary of maximum power density, wastewater, and cell configuration with important remarks corresponding to some significant non-precious metals-based ORR electrocatalysts for the MFC applications are given in Table 2.
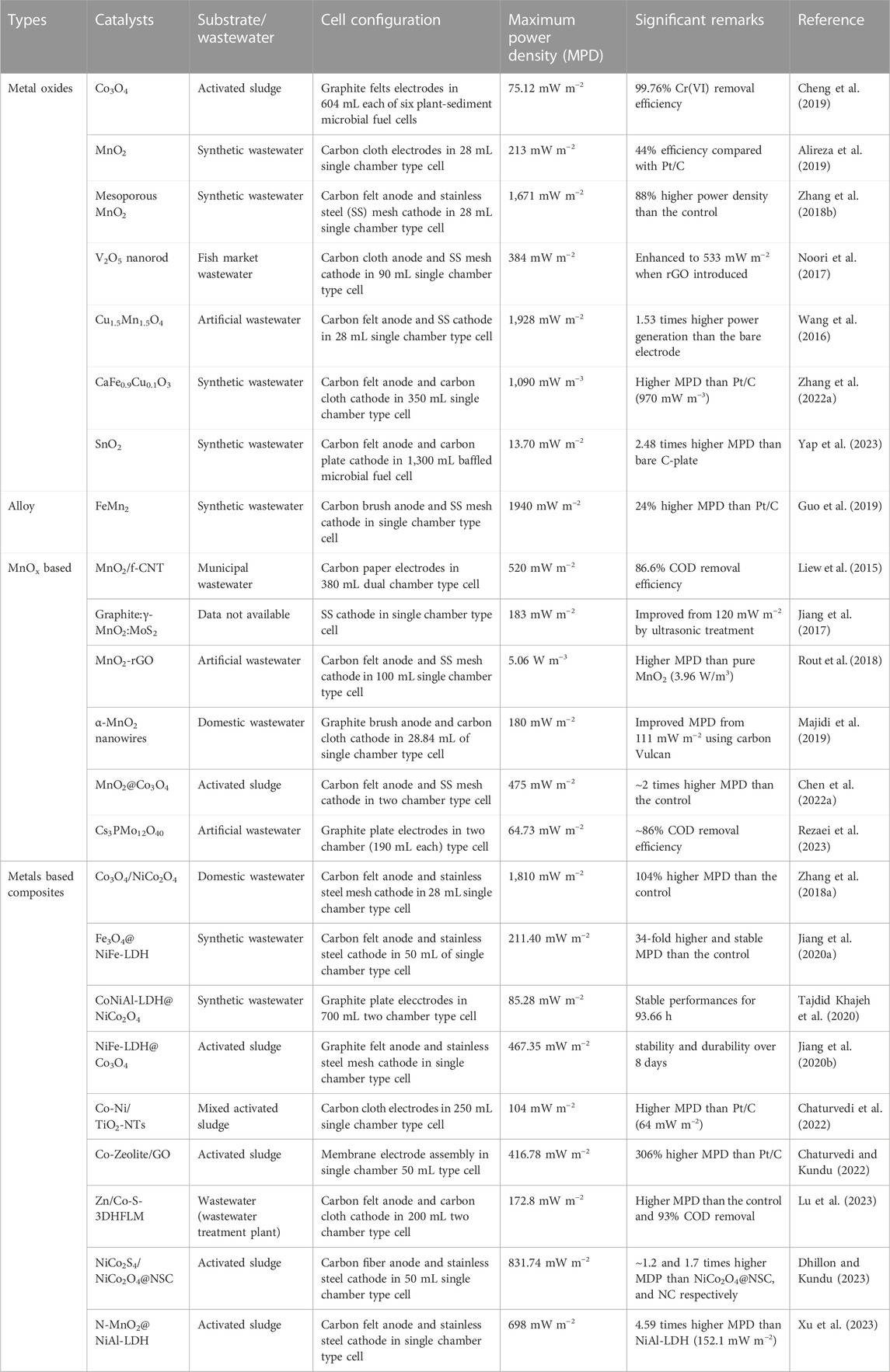
TABLE 2. Summary of the MFC configurations and performances of some significant non-precious metals-based ORR electrocatalysts.
The transition metal oxide ORR electrocatalysts such as Co3O4 (maximum power density of 75.12 mW m−2 with 99.76% Cr(VI) removal efficiency) (Cheng et al., 2019), MnO2 (maximum power density of 213 mW m−2) (Alireza et al., 2019) (maximum power density of 1,671 mW m−2) (Zhang et al., 2018b), V2O5 (maximum power density of 384 mW/m2) (Noori et al., 2017), TiO2 nanotubes (15.16 mWm−2) (Yahia et al., 2016), TiO2 nanoparticles (15.2 Wm−3) (Kumar A. et al., 2023), SnO2 (Yap et al., 2023), etc., showed better activity in MFC applications. Among these metal oxides, manganese oxides (MnOx) are a potential candidate for ORR due to their high chemical stability, low cost, and environmental benignity. However, they showed relatively less activity (Gao et al., 2020). The performance of MnOx was improved by adopting several ways, such as introducing oxygen vacancies, doping with other metals, combining with carbon-based materials, etc. The oxygen-deficient nest-like Cu1.5Mn1.5O4 ORR electrocatalyst showed 1.53 times higher power generation of 1,928 mW m−2 than that of the bare electrode. The enhanced activity with the four electron transfer oxygen reduction mechanism was due to the presence of oxygen deficient in the catalyst (Wang et al., 2016). The MnO2/functionalized carbon nanotubes (f-CNT) exhibited a 86.6% of COD removal efficiency and a higher power density of 520 mW m−2 than the individual components (Liew et al., 2015). The mixture of graphite, γ-MnO2, and MoS2 can achieve a higher maximum power density of up to 183 mW m−2 due to the high surface area and porous structural properties of the catalyst(Jiang et al., 2017). Rout et al. reported that the higher charge transfer property of rGO was enhanced the power density of 5.06 W/m3 of MnO2-reduced graphene oxide (rGO) ORR electrocatalysts than that of pure MnO2 (3.96 W m−3) (Rout et al., 2018). The α-MnO2 nanowires showed a power density of 111 mW m−2, which was increased to 180 mW/m2 when supported on carbon Vulcan which provides high surface area and surface structure (Majidi et al., 2019). The MnO2@Co3O4 composite catalyst showed more than 2-fold higher maximum power generation efficiency than that of the individual components in MFC due to their multiple active sites, high surface area, and high conductivity (Chen et al., 2022a). The Cs3PMo12O40 ORR electrocatalyst showed a maximum power density of 64.73 mW m−2 with around 86% COD removal efficiency in MFC application which was higher than that of the bare graphite electrode. The higher efficiency of the catalyst was due to the mesoporous structure, good electrochemical active sites, electron and proton sink ability, and the presence of Mo (Rezaei et al., 2023). Likewise, the other mixed metal oxide CaFe0.9Cu0.1O3 catalyst showed a higher maximum power density of 1,090 mW m−3 than the commercial Pt/C (970 mW m−3). The Fe3+ content was increased by introducing Cu in the catalyst which resulted in the higher MFC performances (Zhang H. et al., 2022). Besides, Pema et al. very recently reported BiFe1−xLixO3-graphene (G) composite as a low-cost catalyst. They achieved a higher ORR activity of 8.1 W/m3 compared with GO in MFC applications, with a more stable COD removal efficiency of 78.5%. The BiFe1−xLixO3-G composite favors mainly the four electron pathway for ORR in the single chamber MFC as shown in Figure 3 (Pema et al., 2023).
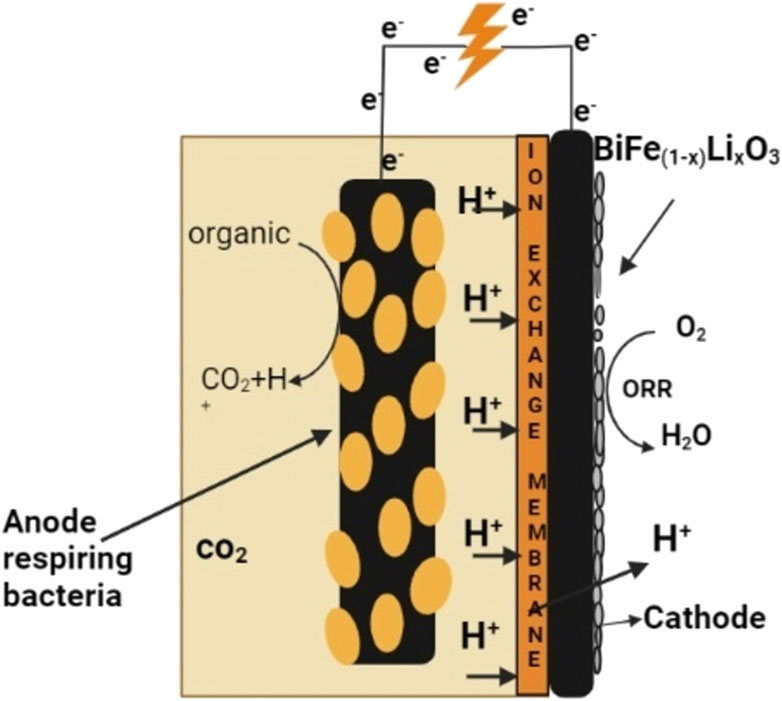
FIGURE 3. Schematic illustration of a single chamber microbial fuel cell (MFC) processes using BiFe1−xLixO3-graphene (G) composite as ORR catalyst (Pema et al., 2023).
The ORR performance of several other metal oxide electrocatalysts was significantly enhanced by adopting some modifications, especially the formation of composites. The Co3O4/NiCo2O4 double-shelled nanocage ORR electrocatalyst showed a 104% higher maximum power density of 1,810 mW m−2 than the control. This catalyst showed improved ORR activity due to its nanocage structure and the presence of Co2+/Co3+ and Ni2+/Ni3+ redox couples (Zhang et al., 2018a). The Fe3O4 supported nickel-iron layered double hydroxides (LDH) showed 34 times higher and more stable (for 110 h) power generation efficiency of about 211.40 mW/m2 than the blank control components in the MFC. The enhanced efficiency was due to its high electrochemical active sites and the excellent conductivity of Fe3O4 (Jiang et al., 2020a). The CoNiAl-LDH@NiCo2O4 composite exhibited a stable output power density of 85.28 mW m−2 for 93.66 h due to the hierarchical core-shell structure of the catalyst (Tajdid Khajeh et al., 2020). In another study, Jiang et al. displayed a remarkable stability and durability over 8 days with a maximum power density of 467.35 mW m-2 by the NiFe-LDH@Co3O4 composite due to its rich active sites and high conductivity (Jiang et al., 2020b). Besides, Guo et al. demonstrated a 39% and 24% higher power density when employing the bimetallic FeMn2 nanocatalysts than the plain AC cathode and Pt/C cathode, respectively due to the synergistic effect between Fe and Mn catalyst(Guo et al., 2019). The Co and Ni doped TiO2 nanotubes (NTs) showed enhanced ORR activity and produced ∼104 mW m-2 current density, which was higher than the commercial Pt/C catalyst. The higher performance was due to the higher specific surface area of TiO2 NTs and active sites of the catalyst (Chaturvedi et al., 2022). Recently, a 3D flower-like metal organic material (3DHFLM), Zn/Co-S-3DHFLM showed enhanced ORR activity in MFC with 172.8 maximum power density and 93% COD removal efficiency 467.35 mW m-2. The synergistic effect of the bimetallic active center and the sulfur has greatly enhanced the ORR activity of the Zn/Co-S-3DHFLM catalyst (Lu et al., 2023). Besides, the NiCo2S4/NiCo2O4@NSC electrocatalyst showed excellent ORR performances in MFC application which showed around 1.2 and 1.7 times the enhanced maximum power density of 831.74 mW m-2 compared to the NiCo2O4@NSC, and N-C catalysts, respectively. The enhanced performance of the catalyst resulted from the synergistic effect between heteroatoms and metal species, optimized amount of N and S, and better active surface area (Dhillon and Kundu, 2023). Besides, the excellent properties such as unique Ping-pong chrysanthemum-like structure, pore size distribution and electrochemical active sites of N-MnO2@NiAl-LDH ORR catalyst showed a maximum power density of 698 mW m-2 (Xu et al., 2023). Gosh et al. reported the CeO2-gC3N4 catalyst showed excellent ORR activity and a power density of 12.53 W m-3 in MFC application. The high surface area, Ce3+ content, oxygen defects, and pyridinic N of the catalyst contributed to better MFC performances (Ghosh et al., 2023). Xie et al. reported a novel MXene@NiCoP ORR electrocatalyst and achieved a maximum power density of 732 W m-3 in MFC application which was due to the presence of active NiCoP species on the MXene (Xie et al., 2023).
The carbon-based composite materials have attracted significant attention as ORR electrocatalysts in MFC applications due to their excellent physicochemical and electrical properties. Ge et al. reported the total resistance was reduced when doping activated carbon into the ortho-hexagon spinel nano Co3O4 which generated the maximum power density of 1,500 mW m−2 in the MFC application, which was 97.36% and 41.24% higher when compared to the bare activated carbon and the commercial Co3O4 cathodes, respectively (Ge et al., 2015). Mecheri et al. demonstrated the oxygen adsorption and ORR rate was increased by increasing the ZrO2 content on carbon which showed a maximum power generation efficiency of 600 mW m-2, which was 15 times lower in cost as compared with the commercial Pt/C catalyst in the MFC application (Mecheri et al., 2016). Zhang et al. reported a Cu2O doped activated carbon ORR electrocatalyst which showed a 59% higher power generation efficiency of 1,390 mW m–2 than that of the bare activated carbon. The lattice (111) plane and surface oxygen defects of the catalyst was enhanced the ORR performances (Zhang et al., 2015). The CoFe2O4 (CFO) supported on nitrogen doped activated carbon (N-AC) performed 2.39 times higher maximum power density of 1770.8 mW m–2 than pure activated carbon cathode catalyst in the MFC application, which was due to the synergistic effect between N-AC and CFO (Huang et al., 2017). The MnCo2O4 nanoparticles in the carbon block exhibited 545 mW m–2 of maximum power density in MFC application which was comparable to the Pt/C catalyst (689 mW m–2) and higher than the bare cathode (214 mW m–2) (Hu et al., 2015). Ge et al. reported the activated carbon modified with NiCo2O4 exhibited a maximum power generation efficiency of 1730 mW m–2, which was comparable with that of the commercial Pt/C catalyst in MFC applications (Ge et al., 2016). Noori et al. displayed the enhancement of the maximum power density of bare V2O5 of 384 mW/m2 to 533 mW m–2 by the incorporation of rGO (Noori et al., 2017). The MnO2/TiO2/g-C3N4 supported on granular activated carbon exhibited a maximum power density of 1,176.47 mW m–3 while simultaneous efficient industrial wastewater treatment of 17.77 kg COD m–3d–1 COD removal capacity (Zhang and Liu, 2020). The iron phthalocyanine (FePc) and nitrogen-doped graphene oxide ORR electrocatalyst showed the better waste treatment and power generation performances (Mecheri et al., 2018). Liu et al. reported the Fe-N derived from FePc supported on activated carbon (AC) performed for MFC of 1,092 mW m–2 maximum current density due to its porous structures and high N content (Liu et al., 2019). The Co and FePc supported in carbon derived from SiC generated 1.57 W m–2 of power with up to 86% of COD removal efficiency of wastewater. The graphitic and high mesoporous properties of the catalyst promote the good four-electron ORR pathway (Noori and Verma, 2019). Huang et al. reported the CoFe2O4 NPs/N-doped AC composite electrocatalyst generated a maximum power density in MFC of 1770.8 mW m–2, which was much greater than the AC of 741.5 mW m–2 due to its large surface area and conductivity (Huang et al., 2017). Yang et al. reported Fe(III)-chitosan hydrogel derived Fe-N-C low-cost ORR electrocatalyst exhibited a 33% higher power generation efficiency of 2.4 W m–2 than the AC control due to the improved ORR activity by higher electron transfer number of 3.4 (Yang et al., 2020b). The Fe, N co-doped graphene with CNTs generated a maximum power density of 1,210 mW m–2 which was higher than the Pt catalyst performance of 1,080 mW m–2 (Wang et al., 2018). Liang et al. achieved a maximum power density of 1,738 mW m–-2 when using the N-doped carbon incorporated with cobalt (Co) nanoparticle ORR electrocatalyst, which was higher than the commercial Pt/C (1,203 mW m–2). The higher performance of the catalyst was attributed to the high surface area and the porous carbon structure(Liang et al., 2020). The N and Co or Fe co-doped multi-walled carbon nanotubes (MWCNTs) exhibited an output power density of 5.1 W m−3 and 6 W m–3, respectively which was due to the nitrogen-metal center active sites formation in the catalysts (Türk et al., 2018). The square-like cobalt oxide nanostructures on N-doped graphene showed a higher ORR activity and exhibited a 24.9% higher power density of 713.6 mW m−2 than the commercial Pt/C (571.3 mW m-2), which was due to the synergistic effect of N-G and Co nanostructures (Cao et al., 2016). Yang et al. reported a novel N-doped molybdenum sulfide combined with CNTs and carbon atoms (N-MoS2/CNTs/C) composite showed a higher maximum power density of 987.4 mW m−2 than that of commercial Pt/C of 601.96 mW m−2 which was due to the excellent electrical properties of the CNT and N present in the catalyst (Yang et al., 2018). Jing et al. demonstrated that the porous structure, defects, heterojunctions, and N atoms in the Fe3Se4/FeSe heterojunctions in N-doped carbon achieved a higher 1,003 mW m−2 power density with good stability for 105 days of operation of air cathode MFC (Jing et al., 2019). The Co2P incorporated in a N-doped carbon nanoframework (Co2PNC-NF) composite electrocatalyst showed 2001 mW m–2 of maximum power density, which was 123% higher than the bare active carbon. The higher activity resulted from the double Co site of 001 face present in the catalyst (Lin et al., 2021). The NiCo alloy on N-doped carbon showed excellent ORR activity and showed a 2.16 times higher maximum power density of 2,325.60 mW m–2 than that of the Pt/C catalyst which was due to the synergistic effect of NiCo active sites, and graphitic and pyridinic N (Huang et al., 2022). Similarly, a novel Co/Ni@GC/NCNTs/CNFs catalyst displayed a splendid performance than the commercial Pt/C in MFC which was due to the abundant active sites and 3D structure of the catalyst(Li J. et al., 2022). Besides, a 4.5 times higher maximum power density than that of bare carbon felt was obtained by FeCoO/Go composite owing to its higher ORR activity(Zheng et al., 2022). The Co nanoparticles on zeolite-GO showed a 306% higher maximum power density of 416.78 mW m–2 than the Pt/C in the MFC application (Chaturvedi and Kundu, 2022). The N and S co-doped carbon based composite, Co9S8@HN/S-C, showed better ORR and COD removal activities in MFC application (Ding et al., 2022). Metal and N co-doped porous carbon, such as Fe-NpC (atomically dispersed Fe-N4 moieties), showed superior performances than Pt/C in MFC (Wang et al., 2023b). Liang et al. reported Fe, N codoped carbon ORR electrocatalysts derived from different Fe ligands showed a maximum power density of 2,041 mW m–2. The improved ORR and MFC activity of the catalysts was ascribed to the graphitization degree and different amounts of Fe2+ and Fe3+ of the catalysts (Liang et al., 2023). Similarly, single atom Fe sites in the N-C showed a higher MFC performance of 3,323 mW m–2 than that of the Pt/C catalyst. The higher performance was due to the optimized Fe-N-C with porous structure and efficient electron transfer properties of the catalyst (Zhao et al., 2023). Long et al. reported CoFe-LDH on partially reduced GO (p-rGO) showed 30 times higher MFC performance than the blank due to more the active sites, structural and interlayer diffusion properties of the catalyst (Long et al., 2023). Remarkably, the fantastic ORR activity in the MFC applications of carbon based composites such as Cu2O@Co/N-C (Chen et al., 2023), Fe-N-C (Kumar D. et al., 2023; Zhang et al., 2023), FeCoNi@N-C (Kaur Dhillon and Paban Kundu, 2023), Fe/Co-N-C (Liu et al., 2023; Zhuang et al., 2023), Co/CoS2@N-CNF (Guo et al., 2023), Co/CoFe NAs@NCNFs (Wang et al., 2023a), etc., were witnessed in several recent reports. The maximum power density, wastewater, and cell configuration with important remarks corresponding to some significant non-precious carbon-based ORR electrocatalysts for the MFC applications are summarized in Table 3.
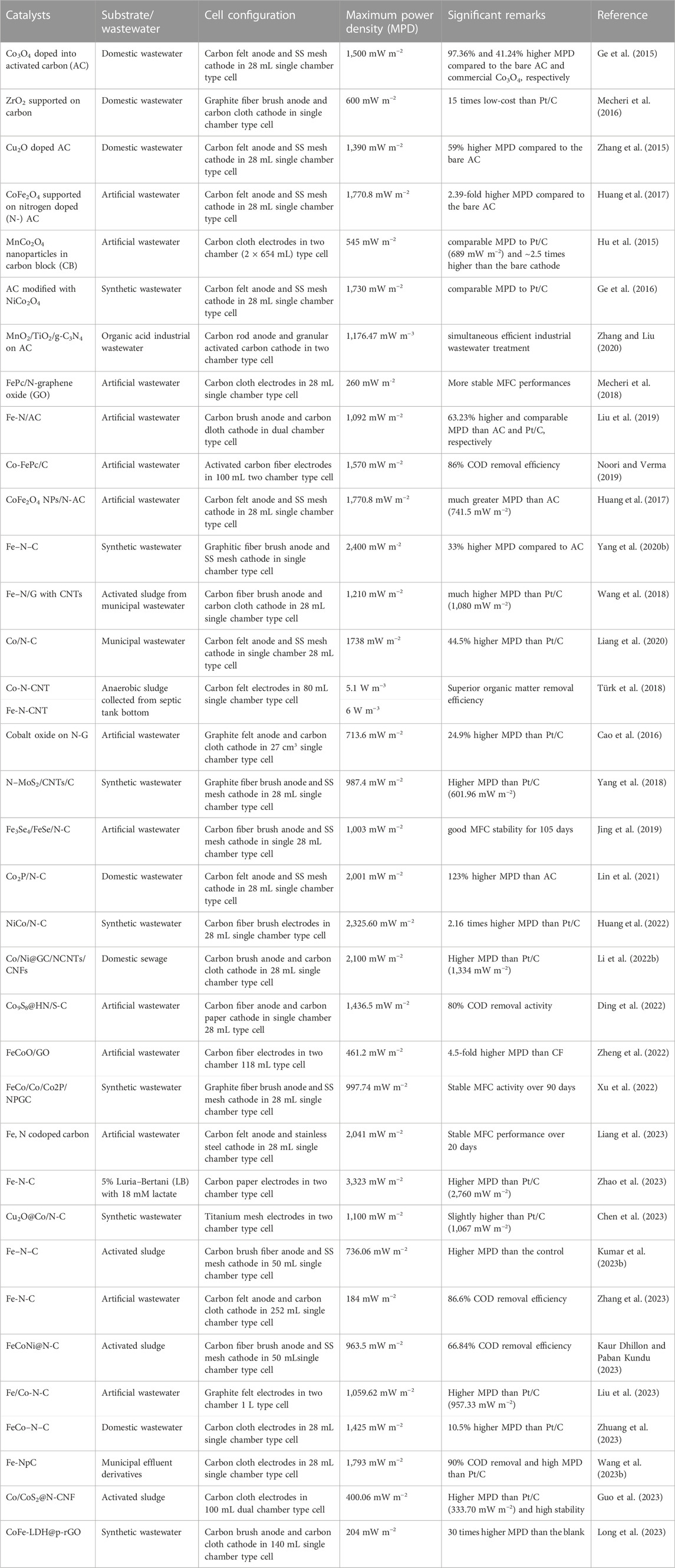
TABLE 3. Summary of the MFC configurations and performances of some significant non-precious carbon-based ORR electrocatalysts.
The metal organic framework (MOF) composites exhibited the excellent performances in MFCs (Priyadarshini et al., 2021). The iron-based MOF, Fe-t-MOF/PANI synthesized by a sustainable route using terephthalic acid monomer (t) derived from plastic waste showed a power density of 680 mW m-2 (Kaur et al., 2021). The Ni-MOF-74, the another cost-effective ORR electrocatalyst, exhibited a maximum power density of 446 mW m–2 with sulfamethoxazole degradation of 84% COD removal (Li S. et al., 2021). The Ni-catecholate-based MOF grown on NiCoAl-LDH/MWCNTs showed an enhanced maximum power density of 448.5 mW m–2 in MFC, which also attributed to the high conductivity and more active sites (Chen et al., 2021). Cheng et al. reported the Co3O4-ZIF/Zn composite showed a highly stable power generation efficiency of 656.9 mW m–2 for up to 30 days compared to the commercial Pt/C catalyst (Chang et al., 2021). The ZIF-67/CNFs showed higher power generation efficiency of 1.191 W m–2, which was higher than that of Pt and CNF due to the porous structure and lower internal resistance of the catalyst (Jiang et al., 2021). Recently, Chen et al. reported the ZIF-67@Ti3AlC2/ZnAl-LDH, which exhibited a 2-fold higher maximum power density of 587 mW m-2 than that of their components, owing to the multiple active sites, high electrical conductivity, and high surface area of the catalyst (Chen et al., 2022d). The covalent organic frameworks (COFs) based composites and their derivatives showed good efficiency in MFCs. For example, the cobalt oxides incorporated COF-derived carbon (Co/N-C-COPs) ORR electrocatalyst showed higher efficiency of 1,817 mW m−2 than the Pt/C catalyst (1,622 mW m−2) in the MFC applications (Yang et al., 2020a). Recently, Chen et al. prepared a highly stable COF-300@NiAl-LDH/GO catalyst showed the enhanced ORR activity in MFC with a maximum power density of 481.69 mW m–2. The high conductivity, catalytic activity, and high electron transfer properties of the catalyst improved the MFC performances of the catalyst(Chen et al., 2022c). Similarly, the MOF derivatives also demonstrated the excellent ORR performances in the MFC applications(Zhang Y. et al., 2018; Wang X. et al., 2022). Tang et al. reported the dual metal (Ni, Co) and N-doped carbon ORR electrocatalysts derived from MOF generated a higher maximum power density of 4,335.6 mW m−2 with outstanding durability over 755 h. This excellent performance of the catalyst resulted from the high surface area and uniform distribution of N and metal species in the graphite structure(Tang et al., 2015). The zeolitic imidazolate framework-67 (ZIF-67) derived Co-N-C composite exhibited a maximum output power density of 399.7 mW m−2 (Li J. C. et al., 2018). Xue et al. reported the Fe, Co, and N-doped carbon derived from ZIF-67 generated a higher maximum power density of 1769.95 mW m-2 than the Pt/C catalyst of 1,410.3 mW m–2. The higher performance was achieved by the optimum pyrolysis temperature of 900°C, which resulted from the higher graphitization and corrosion resistance along with the higher conductivity and active sites of the catalysts (Xue et al., 2020). The N-doped CNT-embedded Co nanoparticles derived from bimetallic MOF produced a much higher power density than Pt/C and 2.54 times higher than the pure AC. The N and metal combination with CNT enhanced the ORR activity, and the reduced total and charge transfer resistance resulted in the higher MFC performances (Zhang S. et al., 2019). The hierarchical porous Fe-N-C nanofibers developed using MOF and bacterial cellulose exhibited high power generation than the commercial Pt/C catalysts of 640.56 mW m−2 with 66.6% COD removal performance which was attributed to its more active sites and porous structure (Li H. et al., 2021). in another report, Li et al. prepared ZIF-67-derived CoNi-LDH@CNFs, which exhibited long durability in MFC and showed a higher maximum power density of 1,390.37 mW m–2 compared to the Pt/C. The higher four-electron transfer ORR was promoted by CoNi active sites and the nanoflower structure of the catalyst (Li H. et al., 2022). The MFC performance of the Fe-N-C catalyst prepared from Fe-doped ZIF-8 was optimized for cost-effective and stable activity using pyrolyzing at different temperature (Wang D. et al., 2022). Similarly, the Co0.7Fe0.3@Co-NC-1 catalyst derived from different MOF precursors showed good ORR activity with 2,486 mW m–2 maximum power density due to the optimized electronic structure and porous carbon (Zhang X. et al., 2022). Recently, Huang et al. demonstrated the 1.45 times higher maximum power generation of 1974 mW m-2 by Fe/Fe3C/NC catalyst derived from MOF than the Pt/C catalyst due to the mesoporous structure and the presence of N in the catalyst (Huang et al., 2023). Ding et al. prepared Co encapsulated in hierarchical porous N-C (Co/HNC) derived from ZIF based MOF, which showed good ORR activity and a maximum power density of 1324 mW m–2 in MFC application (Ding et al., 2023). Qin et al. reported a novel hybrid porous CoCu@N-CNFs ORR catalysts which showed a slightly higher maximum power density of 543 mW m–2 than Pt/C due to the synergistic effect of CoCu alloy (Qin et al., 2023). Table 4 summarizes the maximum power density, wastewater, and cell configuration with important remarks corresponding to some significant non-precious MOFs- and COFs-based ORR electrocatalysts for the MFC applications.
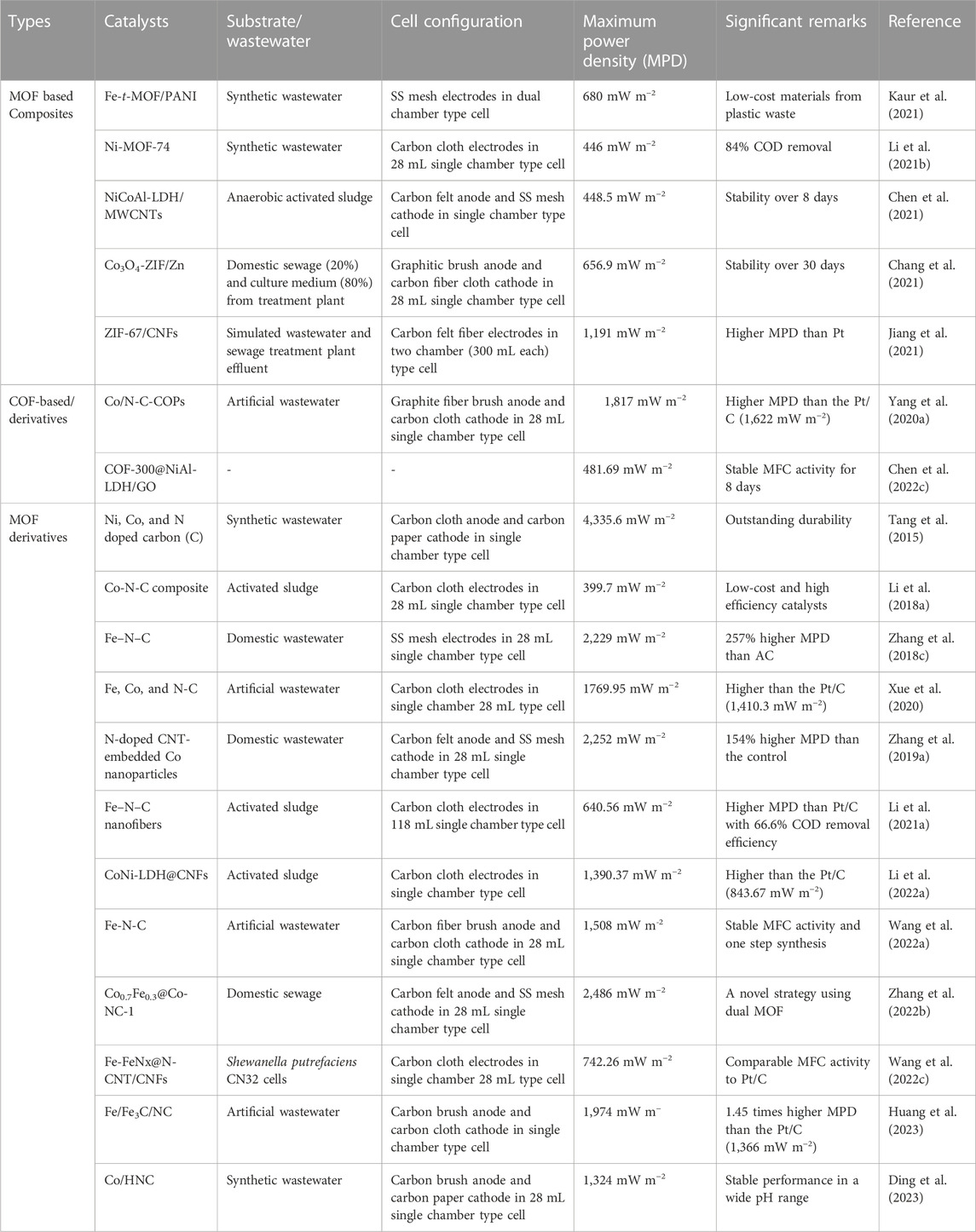
TABLE 4. The MFC configurations and performances of some significant non-precious metal-organic frameworks (MOFs) and covalent organic frameworks (COFs) based ORR electrocatalysts.
6.3 Non-metals and/or carbon-based ORR electrocatalysts
The carbon-based materials without any metals have attracted scientists to discover the high-efficiency ORR electrocatalysts due to their chemical inertness, high electrical conductivity, high stability, high surface area and porosity, good mechanical properties, and inexpensive (Pötschke et al., 2022). The chemically modified carbon block (Vulcan XC-72R) showed the comparable ORR performance in the MFC applications (Duteanu et al., 2010; Yang et al., 2014). The nitrogen doped carbon block performed 2.2 times higher than the pure carbon block in the MFC applications (Kumar et al., 2017). The AC as ORR electrocatalyst on nickel foam as a current collector showed comparable power generation efficiency as that of commercial Pt/C in air-cathode MFC application. The cathode cost was very cheaper (1/30th) when compared with the commercial Pt/C electrocatalyst (Cheng and Wu, 2013). The chemically treated P-doped or non-doped AC showed enhanced performances in MFC applications (Chen Z. et al., 2014; Liu et al., 2015; Wang et al., 2017). Similarly, the low-cost N-doped carbon materials showed 11.3% higher(Feng et al., 2012) and comparable(Shi et al., 2012) performances as the commercial Pt/C catalyst in the MFC applications. The pretreated N-doped AC showed higher maximum power density in the air-cathode MFCs when compared with the commercial Pt/C and the pure AC (Zhang et al., 2014). The surface structural modification of AC also enhanced the ORR performance in MFC applications (Li et al., 2014; Liu et al., 2016). The porous structural modification of AC generated 2.4 times higher power density than that of the unmodified one in the air-cathode MFCs (Li et al., 2014). A large-scale MFC of 85 L was constructed with an AC-based air cathode of 0.62 m2 exposed area and a graphite fiber-based anode of 5.1 cm diameter, 61 cm long. A maximum power density of 0.101 W m–2 was achieved in static flow conditions. The maximum power density was further increased by recirculating the anolyte (domestic wastewater) over the electrodes in a diagonal direction by 17% to 0.118 W m–2 at a hydraulic retention time (HRT) of 33 min (Rossi et al., 2019).
The graphite in the cathode activated using H3PO4 and HNO3 generated 7.9 W m-3 and 6.5 W m-3, respectively of maximum power densities which were 2.4 and 1.8 times greater than that of the bare graphite in the MFC applications (Zhang et al., 2016). The porous structure and high crystallinity of graphite were proven to have a high surface area and, thus, better performance in MFC (Xing et al., 2017). The 3D graphite particle showed as a potential cathode for the generation of H2O2 in the MFC application with 84% COD removal efficiency (Fu et al., 2010; Chen J. Y. et al., 2014). Interestingly, an ultra-low cost MFC fabricated with pencil trace demonstrated excellent performance for portable applications of MFCs (Lee et al., 2016). The three dimensional (3D) graphene nanosheets exhibited a maximum power density of 2.059 W m-2 in the MFC application which was higher than that of activated carbon (Santoro et al., 2017). Dong et al. reported the oxidized graphene in MFC application and achieved 131% more generation of H2O2 than that of pure graphene cathode which was due to the oxygen containing functional groups present in the catalyst (Dong et al., 2018).
The N is one of the most widely used heteroatoms doped with carbon to enhance the ORR activity due to its electronegativity, close atomic radius to carbon, and facilitate easy oxidant adsorption (Dhilllon et al., 2022). The N-doped carbon aerogel (CA) was developed for ORR activity, and its performance was further increased by activating the CA with KOH. The activation of KOH increased the surface area, hierarchically porous structure, reduced C-O-C and COOH, higher pyridinic N content, and decreased pyrrolic N content, thus the enhanced MFC performance than that of non-activated CA (Tian et al., 2018). In another report, Yang et al. demonstrated the improvement of active sites by N doping in the N-CA, which generated 1,048 mW m–2 of power density in the MFC application, which was comparable with the efficiency of the commercial Pt/C (1,051 mW m–2) catalyst (Yang et al., 2019). Wang et al. reported the N-doped carbon derived from isoreticular MOF-3 modified with g-C3N4, which showed a maximum output power density of 1,402.8 mW m–2 in MFC application. This performance was higher than the Pt (1,292.8 mW m–2) and attributed to the introduction of more active N and mesoporous structure when using g-C3N4 as the template for the catalyst synthesis (Wang et al., 2020).
The carbon materials derived from different organic precursors and/or biomass showed excellent performances in MFC applications. The porous AC derived from different sources such as Arhar stalks(Om Prakash et al., 2021), Bamboo(Yang et al., 2017), corncob (Li M. et al., 2018), and coconut shell (Sekhon et al., 2021) showed higher thermal stability and high surface area which results in better MFC performances. The other carbon materials derived from different biomass, such as cornstalk (Sun Y. et al., 2016), sewage sludge (Mian et al., 2019), bacterial cellulose (Wu et al., 2016), hemoglobin (Maruyama et al., 2007), etc., showed excellent ORR performance. Zhou et al. prepared the carbon nanofibers (CNFs) from spider silk exhibited 1800 mW m–2 power density in the MFC, which was higher than the commercial Pt/C electrocatalyst (704 mW m–2). The higher MFC performances were attributed to the increased surface area and more active sites by N and S doping in the catalyst (Zhou et al., 2016). The carbon derived from chitosan having high surface area by high temperature KOH activation showed an increased MFC performance of 1,435 mW m–2, which was 101% higher than the pure AC in the medium of domestic wastewater and nutrient solution (1:1) (Liu et al., 2018). Linget al. demonstrated the MFC performance of the carbon material derived from chitosan was again enhanced by five times from 322.4–1,603.6 mW m–2 by co-doping of N and P. The C-O bond, N, and P existence resulted in a large surface area, lower total resistance, more oxygen transfer, and abundant active sites in the catalyst, which enhanced the ORR and MFC performance (Liang et al., 2019). The P-doped carbon derived from cellulose showed 1,312 mW m−2 of maximum power density in the air-cathode MFC application, which was three-fold higher than the pure carbon and also higher than that of Pt/C electrocatalyst (Liu et al., 2014). The N and F co-doped carbon black obtained from the polytetrafluoroethylene and BP-2000 mixture by pyrolysis under an ammonium atmosphere showed a maximum power density of 672 mW m–2. At the same time, the commercial Pt/C achieved a lesser of 572 mW m–2 (Meng et al., 2015). Ye et al. reported the carbon electrocatalyst obtained from the lotus leaf which showed a maximum power density of 511.5 mW m–2. This hierarchically structured carbon catalyst was stable and showed four-electron transfer performance comparable to the commercial Pt/C catalyst due to the large surface area and porous structure (Ye et al., 2019). The N self-doped porous carbon derived from duckweed provided more active sites for ORR reaction in MFC, which resulted in 625.9 mW m–2 of power density and higher stability than the commercial Pt/C electrocatalyst (Gong et al., 2020). Pepè Sciarria et al. reported the biochar obtained from Olive mill waste and pistachio nutshell which showed a high surface area and O and N functionalities. The MFC performance of the biochar was 271 mWm–2 of maximum output power density, which was 15 times higher than the commercial carbon black (Pepè Sciarria et al., 2020). The N-doped carbon ORR electrocatalysts derived from pomelo peel showed MFC performance of 907.2 mW m–2 power generation, which was comparable to the Pt/C (1,022.9 mW m–2) electrocatalyst (Zhang et al., 2020). Carbon materials derived from watermelon as ORR catalysts showed the good performances in the MFC applications (Zhong et al., 2019; Jiang et al., 2022). The biochar materials derived from different sources such as microalgae, pomelo peel, and eggplant performed well as low-cost and durable materials compared to the commercial Pt/C (Chakraborty et al., 2020; Zhang et al., 2020; Zha et al., 2021; Wang K. et al., 2022; Dhanda et al., 2023). Zhu et al. developed the porous Co and N doped carbon using tea residue showed excellent MFC efficiencies, such as higher maximum power density than Pt/C with COD removal activity. The excellent activity was attributed to the pyridinic-N, and pore structure of the catalyst (Zhu H. et al., 2022). Yang et al. prepared N-doped biochar from microalgae residue by single-step pyrolysis method, which showed a maximum power density of 843.6 mW m−2 and comparable MFC performances with commercial Pt/C. The optimized pyrolysis process resulted in porous structure and improved N content in the catalyst, which promoted the better four-electron pathway ORR in MFC (Yang et al., 2023). In addition, the activated carbon from areca nut husk demonstrated a maximum power density of 590 mW m–2 with good COD removal activity. The better MFC performances was attributed to the porous structure, graphitic nature, and N content present in the catalyst (Subran et al., 2023). Several significant non-metals and/or carbon-based ORR electrocatalysts with their maximum power density, wastewater, cell configuration, and important remarks in the MFC applications are given in Table 5.
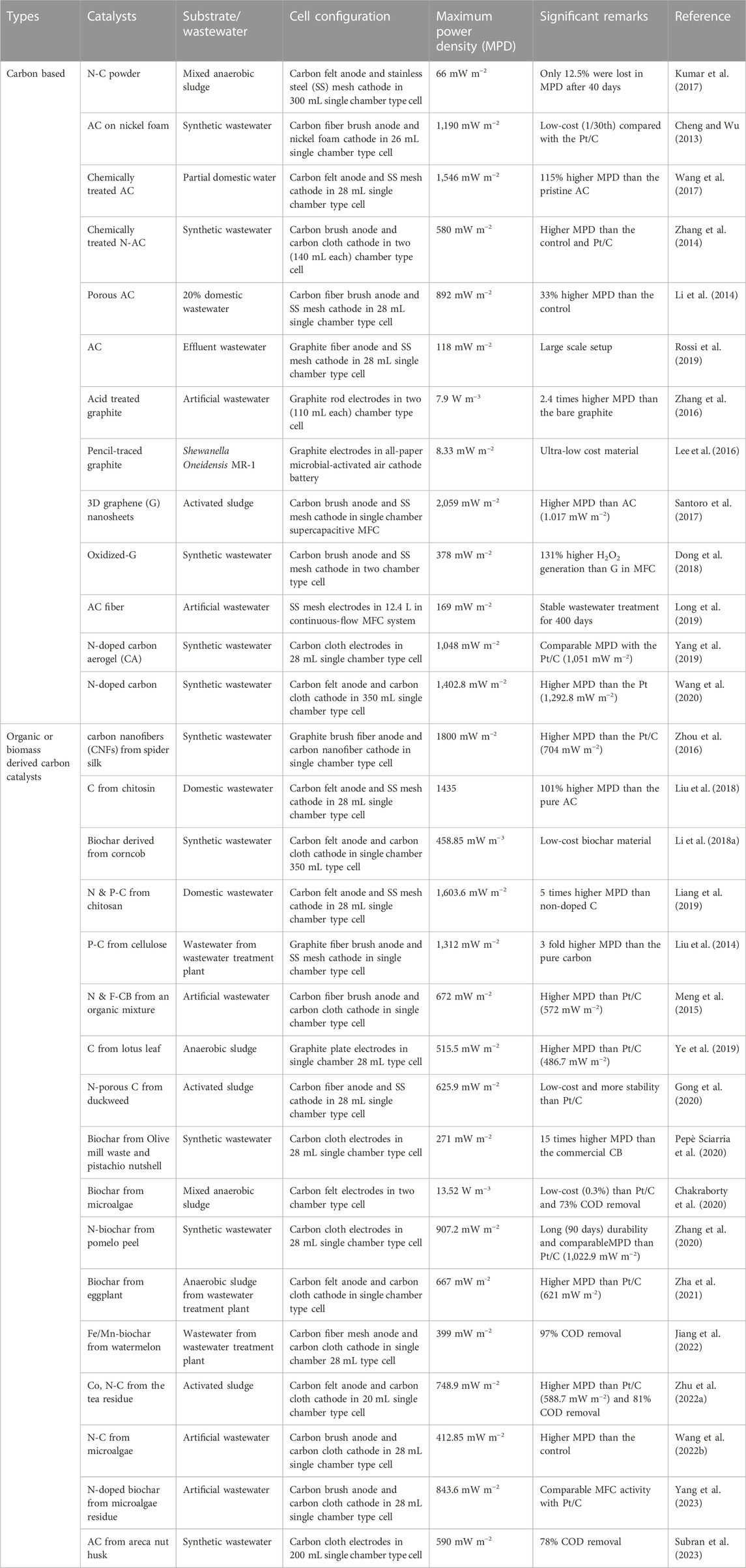
TABLE 5. Several significant non-metals and/or carbon-based ORR electrocatalysts with their maximum power density, MFC configurations, and important remarks in the MFC applications.
6.4 Biocatalysts
Besides exploring the chemically fabricated ORR electrocatalysts, several biomaterials, such as enzymes, living microbial cells, etc., are used as ORR catalysts at the cathode of MFCs (He and Angenent, 2006). The construction of the biocathode was done by growing electroactive bacteria on carbon felt materials or stainless steel mesh and demonstrated a maximum stable power generation of 12.3 μW cm−2 (De Schamphelaire et al., 2010). The laccase immobilized on graphite cathode utilized in MFC showed enhanced decolorization of the organic contaminant and generated higher power density (Savizi et al., 2012). The microorganism on the CNT-deposited stainless steel mesh cathode played an essential role in enhancing the power generation efficiency in the MFC applications (Zhang et al., 2013). The cathode choosing was a limiting factor in the MFC application while using the biomaterials as the ORR electrocatalysts. In a comparative study, the graphite felt biocathode demonstrated the better power generation efficiency in the MFC application among graphite felt, carbon paper, and stainless steel mesh biocathodes (Zhang et al., 2012). The low-cost biocathode materials such as semicoke and activated carbon also showed better performance in MFC application, costing just 2.8% and 22.7% compared to graphite and carbon felt biocathode, respectively (Wei et al., 2011). Besides, the carbon materials such as graphite granules, activated carbon granules, and activated carbon powder in the cathodic part played a significant role to enhance the MFC performances. Among the above, activated carbon granules have greatly enhanced the biocathode’s efficiency by increasing the active microbes for ORR, which resulted in higher MFC performances (Tursun et al., 2016). The biocathode developed with CNT/chitosan exhibited 130% higher electricity generation efficiency when compared with the carbon paper biocathode in MFC application due to the decreased energy loss at the electrode/bacteria interface (Liu et al., 2011). The bacterial cellulose doped with Cu and P showed the maximum output current density of 1,177.31 mW m–2, which was attributed to their more active sites of Cu and P present in the electrocatalyst(Li et al., 2019). Interestingly, Izadi et al. demonstrated highly enhanced power generation efficiency over gas diffusion biocathode consisting of iron-oxidizing bacteria (IOB) of 1.1 W m–2 maximum power density compared to Pt catalyst in MFC application. The mechanism of the MFC process involves oxidation of Fe2+ by IOB and regeneration at the cathode as shown in Figure 4 (Izadi et al., 2019). Very recently, sarma et al. reported the Chlorella sorokiniana, and Philodendron erubescens in the cathodic part of the MFCs showed significantly increased power generation efficiency. The enhanced activity was attributed to the presence of C. sorokiniana, which improved the ORR rate and dissolved oxygen concentration (Sarma et al., 2023). The summary of several significant biomaterials-based ORR electrocatalysts with their maximum power density, wastewater, cell configuration, and essential remarks in the MFC applications are given in Table 6.
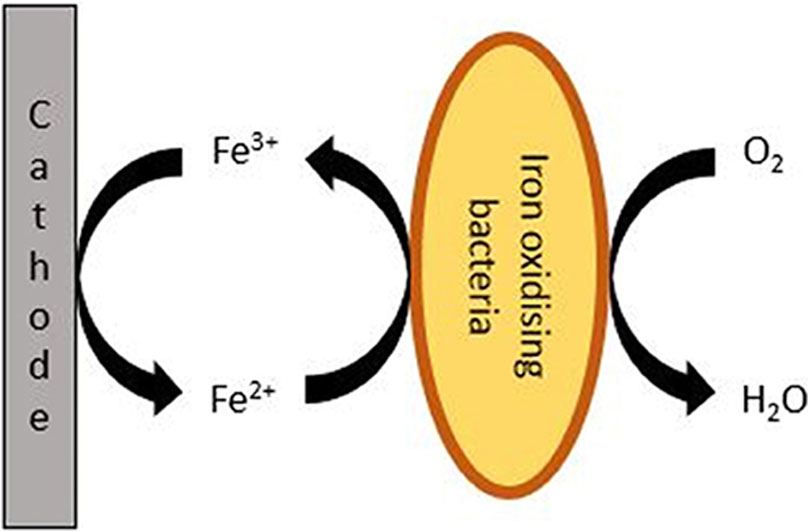
FIGURE 4. Mechanism of biocathode reactions using iron-oxidizing bacteria in microbial fuel cell (MFC) application (Izadi et al., 2019).
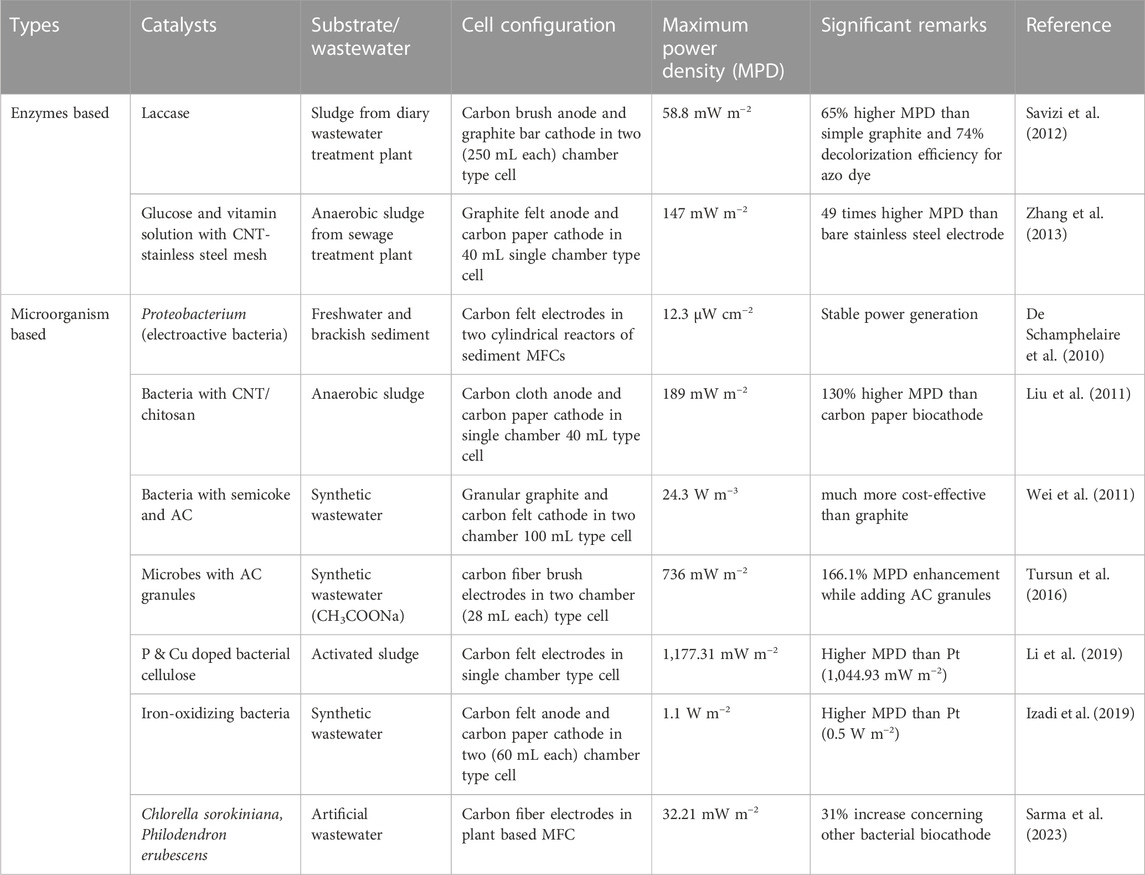
TABLE 6. The summary of several significant biomaterials-based ORR electrocatalysts with their maximum power density, MFC configurations, and essential remarks in the MFC applications.
7 Durability of the ORR electrocatalysts
The long-term stability of the ORR electrocatalysts is a major challenging factor of the MFCs for real-time applications. The researchers have intensively focused on improving the durability along with the higher activity of the ORR electrocatalysts. Several methodologies, such as optimizing structural and chemical composition (Li J. C. et al., 2018), using support materials (Mecheri et al., 2018)., obtaining from other materials (e.g.,: derivatives of MOFs, COFs, and biomaterials) (Yang et al., 2020a), introducing interface with other components (Zhang and Liu, 2020), etc., are demonstrated improved stability and durability of the ORR electrocatalysts. Here are some significant achievements in improving the durability of the ORR electrocatalysts for MFC applications. The FePc stability was increased by less than 1% loss when the introduction of N-GO, which increased the OH group density, and the interaction between FePc and N-GO (Mecheri et al., 2018). Li et al. demonstrated the chemical composition and structural optimization of Co-N-C composite derived from MOF could achieve higher and more stable MFC performance for 1,200 h (Li J. C. et al., 2018). The activated carbon fiber on the stainless-steel ring electrode was used as an air cathode for a continuous-flow MFC system to treat azo dye wastewater for 400 days. This long-term efficiency was attributed to the abundance of microorganisms during the MFC operation. However, the removal efficiency remained stable, and the power output of 169 mW m–2 decreased slowly on the 400th day (Long et al., 2019). The stability of Fe3O4@NiFe in MFC operation was enhanced by introducing LDH, which improved the rate capability, electroactive sites, and cycling stability (Jiang et al., 2020a). Yang et al. demonstrated a high stable MFC performance over 200 h when using COF-derived Co/N-C-COPs ORR electrocatalyst than the Pt/C catalyst, which was due to the high ORR activity and poison tolerance properties of the catalyst (Yang et al., 2020a). Zhang and Liu demonstrated a stable and durable MFC activity by a novel MnO2/TiO2/g-C3N4 ORR electrocatalyst when supported on granular activated carbon for more than 6 months which enhanced the ORR rate and decreased the activation energy of the reaction (Zhang and Liu, 2020). Zhang et al. demonstrated that the high degree of graphitization, good electrical conductivity, and higher specific surface area of the N-C OOR electrocatalyst derived from pomelo peel enhanced the long-term durability in MFC operation for 90 days (Zhang et al., 2020). The mesoporous structure, high N content, and abundant active sites of the Co3O4-ZIF/Zn composite catalyst played a significant role in stable performance for up to 30 days in the MFC operation compared to the commercial Pt/C catalyst (Chang et al., 2021). The improved stability of MnO2@Co3O4 composite catalyst in MFC was achieved by tuning its structural properties, such as high surface area and high ion flow efficiency (Chen et al., 2022a). The CaFe0.9Cu0.1O3 porous perovskite catalyst stability was increased due to the presence of Cu, which increased the amount of Fe3+ and resulted in the higher adsorption of oxygen (Zhang H. et al., 2022). Interestingly, N-doped partially graphitized carbon (NPGC) based composite, FeCo/Co/Co2P/NPGC showed superior stable MFC performance over 90 days with a maximum power density of 997.74 mW m−2, which was due to the different metallic active sites and N-doping of the catalyst (Xu et al., 2022). The combined action of Ti3AlC2, ZnAl-LDH, and ZIF-67 in the ZIF-67@Ti3AlC2/ZnAl-LDH ORR electrocatalyst improved the stability and durability in the MFC operation for 8 days (Chen et al., 2022d). The single-atom structure Phen-Fe MFC demonstrated highly stable and durable performance over 20 days due to the excellent ORR property of the catalyst (Liang et al., 2023). The stable performance of N-MnO2@NiAl-LDH in the MFC application over 7 days was attributed to its excellent porosity, electrochemical active sites, and the presence of N and MnO2 in the catalyst (Xu et al., 2023).The Co/HNC catalyst showed good stable ORR activity with a wide pH range owing to the strong interaction of Co and N-C, hierarchical porous structure, and presence of N in the catalyst (Ding et al., 2023). Therefore, the proper design and development of the ORR electrocatalyst for MFCs could still be explored using an appropriate methodology for efficient practical applications.
8 Challenges and prospects for the practical application
The design and development of highly efficient ORR electrocatalysts are one of the major challenging factors in achieving the practical applications of MFCs. However, Pt is a benchmark ORR electrocatalyst, and its high cost is challenging. In the case of precious metal-based ORR electrocatalysts such as precious metal-based alloys, composites, etc., again, their high cost comes into the picture of challenges. Thus, the alternative highly efficient ORR electrocatalysts contain significantly lower Pt content are should still be explored.
The most common challenge of non-precious metal-based ORR electrocatalysts is the leaching of corresponding metal ions during the reaction, which results in performance loss and environmental issues. The methods such as incorporating or forming composites with other suitable materials have been adopted to overcome the leaching issues. Still, the ORR electrocatalysts' efficiency is lower and involves complex synthetic processes.
Pure graphite or carbon as ORR electrocatalysts in MFCs are limited due to their low performance. Doping of metals or non-metals increased the above catalysts’ performances in the MFCs. However, the durability and the insight mechanism of ORR activity of the effect of doping should be further explored for a better understanding of the reaction. Besides, high N content in the graphite or carbon catalyst decreases the conductivity, which results in lowering the performance of the electrocatalyst.
The challenges involved in the biocatalysts are poor electron transfer and oxygen diffusion at the interface of the cathode and microbial metabolism, and growth utilizes organic carbon, which decreases the ORR performances. In addition to the above, the careful design and development of ORR electrocatalysts of any kind with suitable phase, crystalline, and surface morphological structure is the real challenge for the practical applications of MFCs.
Besides the performances of the air cathode MFC being quite good on the laboratory scale, the ORR electrocatalysts’ stability still needs to be achieved at the peak. Thus, more experimental and theoretical studies for the development of alternative cost-effective, highly efficient, and durable ORR electrocatalysts should be done for the real-time applications. The development of alternative efficient ORR electrocatalysts may be explored for the practical applications of MFCs by using several methodologies include, formation of potential heterojunction by integrating two or more different materials, the introduction of more multifunctional metals (e.g., Fe, Co, Ni, Cu, etc.), and or heteroatoms (e.g., C, N, P, F, etc.) active sites, porous structure, and surface defects, and preparation from inexpensive or waste materials.
9 Conclusion
In conclusion, the air-cathode MFC has been considered a potential technology due to the usage of air as a catholyte in the cathode chamber. The key hindrances to achieving better performances are the factors such as thermodynamic factors, which include activation losses, ohmic losses, and transport losses, and other factors, such as biofouling, catalyst inactivation, and excessive biofilm growth. The development of highly efficient and durable ORR electrocatalysts is one of the main challenges for their practical applications. The high cost of Pt is the main hindrance to large-scale applications. Several attempts have been made to develop alternative ORR electrocatalysts, such as the combination of Pt with other non-precious metals, carbon-based materials, and metal oxides. The non-precious metals based and non-metals and/or carbon based electrocatalysts were also extensively studied as potential ORR electrocatalysts in the MFCs. In addition to the above, the biocatalysts also showed good performances in the MFC applications. Based on the current review, metal-free ORR electrocatalysts such as carbon-based catalysts have been considered as one of the potential alternatives due to their excellent physicochemical properties. However, the stability and efficiency of the ORR electrocatalysts still need to improve for the practical applications of the MFCs. Besides, the quite good performances of the air-cathode MFCs on the lab-scale should be further extended to real-time applications by adopting several experimental and theoretical research.
Author contributions
KE: Prepare original draft of the manuscript; PS: Prepare and review the original draft of the manuscript and supervision; CC: Review of the manuscript and supervision; FS-G, MRK, SYC, SK, and RVM: Review of the manuscript. All authors contributed to the article and approved the submitted version.
Funding
The Government of Chile, Santiago through FONDECYT/ANID Post-doctoral Project No. 3220357.
Acknowledgments
The author PS grateful to the Government of Chile, Santiago for the financial assistance through FONDECYT/ANID Post-doctoral Project No. 3220357.
Conflict of interest
The author SC declared that they were an editorial board member of Frontiers, at the time of submission. This had no impact on the peer review process and the final decision.
The remaining authors declare that the research was conducted in the absence of any commercial or financial relationships that could be construed as a potential conflict of interest.
Publisher’s note
All claims expressed in this article are solely those of the authors and do not necessarily represent those of their affiliated organizations, or those of the publisher, the editors and the reviewers. Any product that may be evaluated in this article, or claim that may be made by its manufacturer, is not guaranteed or endorsed by the publisher.
References
Abubackar, H. N., Biryol, İ., and Ayol, A. (2023). Yeast industry wastewater treatment with microbial fuel cells: effect of electrode materials and reactor configurations. Int. J. Hydrogen Energy 48, 12424–12432. doi:10.1016/j.ijhydene.2022.05.277
Aelterman, P., Rabaey, K., The Pham, H., Boon, N., and Verstraete, W. (2006). Continuous electricity generation at high voltages and currents using stacked microbial fuel cells. Environ. Sci. Technol. 40, 3388–3394. doi:10.1021/es0525511
Agudelo-Escobar, L. M., Cabrera, S. E., and Avignone Rossa, C. (2022). A bioelectrochemical system for waste degradation and energy recovery from industrial coffee wastewater. Front. Chem. Eng. 4, 814987. doi:10.3389/fceng.2022.814987
Aiswaria, P., Naina Mohamed, S., Singaravelu, D. L., Brindhadevi, K., and Pugazhendhi, A. (2022). A review on graphene/graphene oxide supported electrodes for microbial fuel cell applications: challenges and prospects. Chemosphere 296, 133983. doi:10.1016/j.chemosphere.2022.133983
Alireza, A. A., Hamnabard, N., Meshkati, S. M. H., Pakan, M., and Ahn, Y. H. (2019). Effectiveness of phase- and morphology-controlled MnO 2 nanomaterials derived from flower-like δ-MnO 2 as alternative cathode catalyst in microbial fuel cells. Dalt. Trans. 48, 5429–5443. doi:10.1039/c9dt00520j
Amirul Islam, M., Wai Woon, C., Ethiraj, B., Kui Cheng, C., Yousuf, A., and Maksudur Rahman Khan, M. (2016). Ultrasound driven biofilm removal for stable power generation in microbial fuel cell. Energy & Fuels 31, 968–976. doi:10.1021/acs.energyfuels.6b02294
An, J., Kim, B., Chang, I. S., and Lee, H.-S. (2015). Shift of voltage reversal in stacked microbial fuel cells. J. Power Sources 278, 534–539. doi:10.1016/j.jpowsour.2014.12.112
Babanova, S., Jones, J., Wiseman, K., Soles, J., Garcia, J., Huerta, P., et al. (2022). Bioelectrochemical treatment technology—the new practical approach for wastewater management and GHG emissions reduction. Front. Chem. Eng. 4, 832505. doi:10.3389/fceng.2022.832505
Bagchi, S., and Behera, M. (2019). Methanogenesis suppression in microbial fuel cell by aluminium dosing. Bioelectrochemistry 129, 206–210. doi:10.1016/j.bioelechem.2019.05.019
Blanchet, E., Erable, B., De Solan, M.-L., and Bergel, A. (2016). Two-dimensional carbon cloth and three-dimensional carbon felt perform similarly to form bioanode fed with food waste. Electrochem. Commun. 66, 38–41. doi:10.1016/j.elecom.2016.02.017
Canfield, J. H., Goldner, B. H., and Lutwack, R. (1963). Utilization of human wastes as electrochemical fuels. NASA Tech. Rep. Magna Corp. Anaheim CA 63, 615–616.
Cao, C., Wei, L., Su, M., Wang, G., and Shen, J. (2016). Enhanced power generation using nano cobalt oxide anchored nitrogen-decorated reduced graphene oxide as a high-performance air-cathode electrocatalyst in biofuel cells. RSC Adv. 6, 52556–52563. doi:10.1039/c6ra11095a
Cetinkaya, A. Y., Ozdemir, O. K., Koroglu, E. O., Hasimoglu, A., and Ozkaya, B. (2015). The development of catalytic performance by coating Pt-Ni on CMI7000 membrane as a cathode of a microbial fuel cell. Bioresour. Technol. 195, 188–193. doi:10.1016/j.biortech.2015.06.064
Chakraborty, I., Bhowmick, G. D., Ghosh, D., Dubey, B. K., Pradhan, D., and Ghangrekar, M. M. (2020). Novel low-cost activated algal biochar as a cathode catalyst for improving performance of microbial fuel cell. Sustain. Energy Technol. Assessments 42, 100808. doi:10.1016/j.seta.2020.100808
Chandrasekhar, K., and Ahn, Y. H. (2017). Effectiveness of piggery waste treatment using microbial fuel cells coupled with elutriated-phased acid fermentation. Bioresour. Technol. 244, 650–657. doi:10.1016/J.BIORTECH.2017.08.021
Chang, J., Wang, W., Wang, Y., Su, C., Pan, J., Wang, H., et al. (2021). Fabrication of bimetallic Co/Zn leaf blade structure template derived Co3O4-ZIF/Zn and its ORR catalytic performance for MFC. J. Taiwan Inst. Chem. Eng. 129, 144–153. doi:10.1016/J.JTICE.2021.09.027
Chaturvedi, A., Dhillon, S. K., and Kundu, P. P. (2022). 1-D semiconducting TiO2 nanotubes supported efficient bimetallic Co-Ni cathode catalysts for power generation in single-chambered air-breathing microbial fuel cells. Sustain. Energy Technol. Assessments 53, 102479. doi:10.1016/j.seta.2022.102479
Chaturvedi, A., and Kundu, P. P. (2022). Co-doped zeolite–GO nanocomposite as a high-performance ORR catalyst for sustainable bioelectricity generation in air-cathode single-chambered microbial fuel cells. ACS Appl. Mat. Interfaces 14, 33219–33233. doi:10.1021/acsami.2c07638
Chaturvedi, A., and Kundu, P. P. (2021). Recent advances and perspectives in platinum-free cathode catalysts in microbial fuel cells. J. Environ. Chem. Eng. 9, 105662. doi:10.1016/J.JECE.2021.105662
Chen, H., Jiang, D., Xie, H., Liu, Y., Li, S., and Wang, Y. (2023). Cu 2 O@Co/N-doped carbon as antibacterial catalysts for oxygen reduction in microbial fuel cells. Environ. Sci. Nano 10, 158–165. doi:10.1039/D2EN00980C
Chen, J., Liu, Y., Yang, J., Wang, H., Liu, H., Cao, S., et al. (2022a). The potential of Co3O4 nanoparticles attached to the surface of MnO2 nanorods as cathode catalyst for single-chamber microbial fuel cell. Bioresour. Technol. 346, 126584. doi:10.1016/j.biortech.2021.126584
Chen, J., Yang, J., Jiang, L., Wang, X., Yang, D., Wei, Q., et al. (2021). Improved electrochemical performances by Ni-catecholate-based metal organic framework grown on NiCoAl-layered double hydroxide/multi-wall carbon nanotubes as cathode catalyst in microbial fuel cells. Bioresour. Technol. 337, 125430. doi:10.1016/J.BIORTECH.2021.125430
Chen, J., Yang, J., Wang, R., Yang, Y., and Liu, Y. (2022b). Design and research progress of nano materials in cathode catalysts of microbial fuel cells: A review. Int. J. Hydrogen Energy 47, 18098–18108. doi:10.1016/j.ijhydene.2022.04.020
Chen, J., Yang, J., Wang, X., Yang, D., Yang, M., Tian, J., et al. (2022c). Cathode catalyst selection for enhancing oxygen reduction reactions of microbial fuel cells: COF-300@NiAl-LDH/GO and Ti3AlC2/NiCoAl-LDH. Int. J. Hydrogen Energy 47, 16179–16188. doi:10.1016/j.ijhydene.2022.03.107
Chen, J., Yang, J., Wu, Y., Zhao, Y., Wang, X., Wang, J., et al. (2022d). Three-dimensional ZIF-67 attached lamellar Ti3AlC2 combined with ZnAl-LDH as cathode catalyst for enhancing oxygen reduction reaction of microbial fuel cells. Int. J. Hydrogen Energy 47, 16262–16271. doi:10.1016/j.ijhydene.2022.03.133
Chen, J. Y., Li, N., and Zhao, L. (2014a). Three-dimensional electrode microbial fuel cell for hydrogen peroxide synthesis coupled to wastewater treatment. J. Power Sources 254, 316–322. doi:10.1016/J.JPOWSOUR.2013.12.114
Chen, W., Liu, Z., Li, Y., Jiang, K., Hou, J., Lou, X., et al. (2019). A novel stainless steel fiber felt/Pd nanocatalysts electrode for efficient ORR in air-cathode microbial fuel cells. Electrochim. Acta 324, 134862. doi:10.1016/J.ELECTACTA.2019.134862
Chen, Z., Li, K., and Pu, L. (2014b). The performance of phosphorus (P)-doped activated carbon as a catalyst in air-cathode microbial fuel cells. Bioresour. Technol. 170, 379–384. doi:10.1016/j.biortech.2014.07.114
Cheng, C., Hu, Y., Shao, S., Yu, J., Zhou, W., Cheng, J., et al. (2019). Simultaneous Cr(VI) reduction and electricity generation in Plant-Sediment Microbial Fuel Cells (P-SMFCs): synthesis of non-bonding Co3O4 nanowires onto cathodes. Environ. Pollut. 247, 647–657. doi:10.1016/J.ENVPOL.2019.01.084
Cheng, S., and Wu, J. (2013). Air-cathode preparation with activated carbon as catalyst, PTFE as binder and nickel foam as current collector for microbial fuel cells. Bioelectrochemistry 92, 22–26. doi:10.1016/j.bioelechem.2013.03.001
Cohen, B. (1931). The bacterial culture as an electrical half-cell. J. Bacteriol. 21, 18–19. Available at: https://www.scopus.com/inward/record.uri?eid=2-s2.0-0002906445&partnerID=40&md5=bfcdc2eb3fcc88f839a3083f277efb6f.
Daghio, M., Aulenta, F., Vaiopoulou, E., Franzetti, A., Arends, J. B. A., Sherry, A., et al. (2017). Electrobioremediation of oil spills. Water Res. 114, 351–370. doi:10.1016/j.watres.2017.02.030
Dai, H. Y., Yang, H. M., Jian, X., Liu, X., and Liang, Z. H. (2017). Performance of Ag2O/Ag electrode as cathodic electron acceptor in microbial fuel cell. Acta Metall. Sin. Engl. Lett. 30, 1243–1248. doi:10.1007/s40195-017-0616-1
De Schamphelaire, L., Boeckx, P., and Verstraete, W. (2010). Evaluation of biocathodes in freshwater and brackish sediment microbial fuel cells. Appl. Microbiol. Biotechnol. 87, 1675–1687. doi:10.1007/s00253-010-2645-9
Dey, N., Samuel, G. V., Raj, D. S., and Gajalakshmi, B. (2023). Nanomaterials as potential high performing electrode materials for microbial fuel cells. Appl. Nanosci. 13, 2625–2640. doi:10.1007/s13204-022-02371-3
Dhanda, A., Raj, R., Sathe, S. M., Dubey, B. K., and Ghangrekar, M. M. (2023). Graphene and biochar-based cathode catalysts for microbial fuel cell: performance evaluation, economic comparison, environmental and future perspectives. Environ. Res. 231, 116143. doi:10.1016/j.envres.2023.116143
Dhilllon, S. K., Kundu, P. P., and Jain, R. (2022). Catalytic advancements in carbonaceous materials for bio-energy generation in microbial fuel cells: A review. Environ. Sci. Pollut. Res. 1, 24815–24841. doi:10.1007/s11356-021-17529-9
Dhillon, S. K., and Kundu, P. P. (2023). Transition metal sulfide/oxide nanoflowers decorated on poly (aniline-2-sulfonic acid) modified polyacrylamide derived carbon cathode catalyst for bioenergy generation in microbial fuel cells. Electrochim. Acta 461, 142697. doi:10.1016/j.electacta.2023.142697
Díaz-Vázquez, D., Garibay, M. V., Fernández del Castillo, A., Orozco-Nunnelly, D. A., Senés-Guerrero, C., and Gradilla-Hernández, M. S. (2022). Yeast community composition impacts on tequila industry waste treatment for pollution control and waste-to-product synthesis. Front. Chem. Eng. 4, 1013873. doi:10.3389/fceng.2022.1013873
Ding, F., Liu, H., Jiang, X., Jiang, Y., Cheng, J., Tu, Y., et al. (2023). Bimetallic zeolite imidazolium framework derived multiphase Co/HNC as pH-universal catalysts with efficient oxygen reduction performance for microbial fuel cells. Electrochim. Acta 438, 141548. doi:10.1016/j.electacta.2022.141548
Ding, F., Liu, H., Jiang, X., Jiang, Y., Tu, Y., Xiao, W., et al. (2022). Co9S8 nanoparticles encapsulated in N,S co-doped hierarchical carbon as an efficient oxygen reduction electrocatalyst for microbial fuel cells. J. Electroanal. Chem. 909, 116130. doi:10.1016/j.jelechem.2022.116130
Dong, H., Liu, X., Xu, T., Wang, Q., Chen, X., Chen, S., et al. (2018). Hydrogen peroxide generation in microbial fuel cells using graphene-based air-cathodes. Bioresour. Technol. 247, 684–689. doi:10.1016/j.biortech.2017.09.158
Duteanu, N., Erable, B., Senthil Kumar, S. M., Ghangrekar, M. M., and Scott, K. (2010). Effect of chemically modified Vulcan XC-72R on the performance of air-breathing cathode in a single-chamber microbial fuel cell. Bioresour. Technol. 101, 5250–5255. doi:10.1016/j.biortech.2010.01.120
Dziegielowski, J., Metcalfe, B., and Di Lorenzo, M. (2021). Towards effective energy harvesting from stacks of soil microbial fuel cells. J. Power Sources 515, 230591. doi:10.1016/j.jpowsour.2021.230591
Fang, C., and Achal, V. (2019). The potential of microbial fuel cells for remediation of heavy metals from soil and water—review of application. Microorganisms 7, 697. doi:10.3390/microorganisms7120697
Feng, Y., Shi, X., Wang, X., Lee, H., Liu, J., Qu, Y., et al. (2012). Effects of sulfide on microbial fuel cells with platinum and nitrogen-doped carbon powder cathodes. Biosens. Bioelectron. 35, 413–415. doi:10.1016/j.bios.2011.08.030
Fu, L., You, S. J., Yang, F. L., Gao, M. M., Fang, X. H., and Zhang, G. Q. (2010). Synthesis of hydrogen peroxide inmicrobial fuel cell. J. Chem. Technol. Biotechnol. 85, 715–719. doi:10.1002/jctb.2367
Gao, M., Lu, J.-Y., and Li, W.-W. (2020). “Oxygen reduction reaction electrocatalysts for microbial fuel cells,” in Novel catalyst materials for bioelectrochemical systems: Fundamentals and applications. Editors L. Singh, D. M. Mahapatra, and H. Liu (ACS Publications), 73–96. doi:10.1021/bk-2020-1342.ch004
Gargalo, C. L., Rapazzo, J., Carvalho, A., and Gernaey, K. V. (2022). Optimal conversion of organic wastes to value-added products: toward a sustainable integrated biorefinery in Denmark. Front. Chem. Eng. 4, 837105. doi:10.3389/fceng.2022.837105
Gautam, R. K., and Verma, A. (2019). “Electrocatalyst materials for oxygen reduction reaction in microbial fuel cell,” in Microbial electrochemical technology sustainable platform for fuels, chemicals and remediation. Editors S. Venkata Mohan, S. Varjani, and A. Pandey (Elsevier B.V), 451–483. doi:10.1016/B978-0-444-64052-9.00018-2
Ge, B., Li, K., Fu, Z., Pu, L., Zhang, X., Liu, Z., et al. (2016). The performance of nano urchin-like NiCo2O4 modified activated carbon as air cathode for microbial fuel cell. J. Power Sources 303, 325–332. doi:10.1016/j.jpowsour.2015.11.003
Ge, B., Li, K., Fu, Z., Pu, L., and Zhang, X. (2015). The addition of ortho-hexagon nano spinel Co3O4 to improve the performance of activated carbon air cathode microbial fuel cell. Bioresour. Technol. 195, 180–187. doi:10.1016/J.BIORTECH.2015.06.054
Ghosh, D., Chakraborty, I., Ghangrekar, M. M., and Pradhan, D. (2023). Precious-metal-free solvothermally synthesized CeO 2 nanosphere-graphitic carbon nitride sheet composites for oxygen reduction reaction. ACS Appl. Energy Mat. 6, 4570–4583. doi:10.1021/acsaem.2c03959
Gong, X. B., You, S. J., Wang, X. H., Gan, Y., Zhang, R. N., and Ren, N. Q. (2013). Silver-tungsten carbide nanohybrid for efficient electrocatalysis of oxygen reduction reaction in microbial fuel cell. J. Power Sources 225, 330–337. doi:10.1016/j.jpowsour.2012.10.047
Gong, X., Peng, L., Wang, X., Wu, L., and Liu, Y. (2020). Duckweed derived nitrogen self-doped porous carbon materials as cost-effective electrocatalysts for oxygen reduction reaction in microbial fuel cells. Int. J. Hydrogen Energy 45, 15336–15345. doi:10.1016/J.IJHYDENE.2020.03.177
Gude, V. G. (2016). Wastewater treatment in microbial fuel cells – An overview. J. Clean. Prod. 122, 287–307. doi:10.1016/j.jclepro.2016.02.022
Guo, S., Liu, Y., Sun, Y., and Li, C. (2023). Heterostructure-induced enhanced oxygen catalysis behavior based on metal cobalt coupled with compound anchored on N-doped carbon nanofiber for microbial fuel cell. J. Colloid Interface Sci. 636, 305–316. doi:10.1016/j.jcis.2023.01.013
Guo, X., Jia, J., Dong, H., Wang, Q., Xu, T., Fu, B., et al. (2019). Hydrothermal synthesis of FeMn bimetallic nanocatalysts as high-efficiency cathode catalysts for microbial fuel cells. J. Power Sources 414, 444–452. doi:10.1016/J.JPOWSOUR.2019.01.024
Gupta, S., Patro, A., Mittal, Y., Dwivedi, S., Saket, P., Panja, R., et al. (2023). The race between classical microbial fuel cells, sediment-microbial fuel cells, plant-microbial fuel cells, and constructed wetlands-microbial fuel cells: applications and technology readiness level. Sci. Total Environ. 879, 162757. doi:10.1016/j.scitotenv.2023.162757
Haile, A. S., Yohannes, W., and Mekonnen, Y. S. (2020). Oxygen reduction reaction on Pt-skin Pt 3 V(111) fuel cell cathode: A density functional theory study. RSC Adv. 10, 27346–27356. doi:10.1039/D0RA02972F
Halakoo, E., Khademi, A., Ghasemi, M., Yusof, N. M., Gohari, R. J., and Ismail, A. F. (2015). Production of sustainable energy by carbon nanotube/platinum catalyst in microbial fuel cell. Procedia CIRP 26, 473–476. doi:10.1016/J.PROCIR.2014.07.034
Han, H. X., Shi, C., Yuan, L., and Sheng, G. P. (2017). Enhancement of methyl orange degradation and power generation in a photoelectrocatalytic microbial fuel cell. Appl. Energy 204, 382–389. doi:10.1016/J.APENERGY.2017.07.032
Harnisch, F., Wirth, S., and Schröder, U. (2009). Effects of substrate and metabolite crossover on the cathodic oxygen reduction reaction in microbial fuel cells: platinum vs. iron(II) phthalocyanine based electrodes. Electrochem. Commun. 11, 2253–2256. doi:10.1016/j.elecom.2009.10.002
He, Z., and Angenent, L. T. (2006). Application of bacterial biocathodes in microbial fuel cells. Electroanalysis 18, 2009–2015. doi:10.1002/elan.200603628
Hidayat, A. R. P., Widyanto, A. R., Asranudin, A., Ediati, R., Sulistiono, D. O., Putro, H. S., et al. (2022). Recent development of double chamber microbial fuel cell for hexavalent chromium waste removal. J. Environ. Chem. Eng. 10, 107505. doi:10.1016/j.jece.2022.107505
Hou, Y., Chen, J., He, Z., Yuan, H., and Abu-Reesh, I. M. (2016). Oxygen reduction reaction catalysts used in microbial fuel cells for energy-efficient wastewater treatment: A review. Mat. Horiz. 3, 382–401. doi:10.1039/c6mh00093b
Hu, D., Zhang, G., Wang, J., and Zhong, Q. (2015). Carbon-supported spinel nanoparticle MnCo2O4 as a cathode catalyst towards oxygen reduction reaction in dual-chamber microbial fuel cell. Aust. J. Chem. 68, 987. doi:10.1071/CH14516
Huang, Q., Zhou, P., Yang, H., Zhu, L., and Wu, H. (2017). In situ generation of inverse spinel CoFe 2 O 4 nanoparticles onto nitrogen-doped activated carbon for an effective cathode electrocatalyst of microbial fuel cells. Chem. Eng. J. 325, 466–473. doi:10.1016/j.cej.2017.05.079
Huang, S., Geng, Y., Chen, D., Li, N., Xu, Q., Li, H., et al. (2023). Metal-organic framework-derived Fe/Fe3C embedded in N-doped carbon as a highly efficient oxygen reduction catalyst for microbial fuel cells. Chem. Eng. Sci. 278, 118906. doi:10.1016/j.ces.2023.118906
Huang, S., Geng, Y., Xia, J., Chen, D., and Lu, J. (2022). NiCo alloy nanoparticles on a N/C dual-doped matrix as a cathode catalyst for improved microbial fuel cell performance. Small 18, 2106355. doi:10.1002/smll.202106355
Huang, X., Duan, C., Duan, W., Sun, F., Cui, H., Zhang, S., et al. (2021). Role of electrode materials on performance and microbial characteristics in the constructed wetland coupled microbial fuel cell (CW-MFC): A review. J. Clean. Prod. 301, 126951. doi:10.1016/j.jclepro.2021.126951
Izadi, P., Fontmorin, J.-M., Fernández, L. F. L., Cheng, S., Head, I., and Yu, E. H. (2019). High performing gas diffusion biocathode for microbial fuel cells using acidophilic iron oxidizing bacteria. Front. Energy Res. 7, 00093. doi:10.3389/fenrg.2019.00093
Jatoi, A. S., Akhter, F., Mazari, S. A., Sabzoi, N., Aziz, S., Soomro, S. A., et al. (2021). Advanced microbial fuel cell for waste water treatment—A review. Environ. Sci. Pollut. Res. 28, 5005–5019. doi:10.1007/s11356-020-11691-2
Jiang, B., Muddemann, T., Kunz, U., Silva e Silva, L. G., Bormann, H., Niedermeiser, M., et al. (2017). Graphite/MnO 2 and MoS 2 composites used as catalysts in the oxygen reduction cathode of microbial fuel cells. J. Electrochem. Soc. 164, E519–E524. doi:10.1149/2.0801714jes
Jiang, J., Zhang, S., Li, S., Zeng, W., Li, F., and Wang, W. (2022). Magnetized manganese-doped watermelon rind biochar as a novel low-cost catalyst for improving oxygen reduction reaction in microbial fuel cells. Sci. Total Environ. 802, 149989. doi:10.1016/j.scitotenv.2021.149989
Jiang, L., Chen, J., An, Y., Han, D., Chang, S., Liu, Y., et al. (2020a). Enhanced electrochemical performance by nickel-iron layered double hydroxides (LDH) coated on Fe3O4 as a cathode catalyst for single-chamber microbial fuel cells. Sci. Total Environ. 745, 141163. doi:10.1016/j.scitotenv.2020.141163
Jiang, L., Chen, J., Han, D., Chang, S., Yang, R., An, Y., et al. (2020b). Potential of core-shell NiFe layered double hydroxide@Co3O4 nanostructures as cathode catalysts for oxygen reduction reaction in microbial fuel cells. J. Power Sources 453, 227877. doi:10.1016/J.JPOWSOUR.2020.227877
Jiang, N., Huang, M., Li, J., Song, J., Zheng, S., Gao, Y., et al. (2021). Enhanced bioelectricity output of microbial fuel cells via electrospinning zeolitic imidazolate framework-67/polyacrylonitrile carbon nanofiber cathode. Bioresour. Technol. 337, 125358. doi:10.1016/J.BIORTECH.2021.125358
Jing, B., You, S., Ma, Y., Xing, Z., Chen, H., Dai, Y., et al. (2019). Fe3Se4/FeSe heterojunctions in cornstalk-derived N-doped carbon framework enhance charge transfer and cathodic oxygen reduction reaction to boost bio-electricity generation. Appl. Catal. B Environ. 244, 465–474. doi:10.1016/J.APCATB.2018.11.074
Kamali, M., Aminabhavi, T. M., Abbassi, R., Dewil, R., and Appels, L. (2022). Engineered nanomaterials in microbial fuel cells – recent developments, sustainability aspects, and future outlook. Fuel 310, 122347. doi:10.1016/j.fuel.2021.122347
Kamali, M., Guo, Y., Aminabhavi, T. M., Abbassi, R., Dewil, R., and Appels, L. (2023). Pathway towards the commercialization of sustainable microbial fuel cell-based wastewater treatment technologies. Renew. Sustain. Energy Rev. 173, 113095. doi:10.1016/j.rser.2022.113095
Kaur Dhillon, S., and Paban Kundu, P. (2023). Transitional trimetallic alloy embedded polyacrylamide hydrogel derived nitrogen-doped carbon air-cathode for bioenergy generation in microbial fuel cell. Sustain. Energy Technol. Assessments 55, 103001. doi:10.1016/j.seta.2022.103001
Kaur, R., Marwaha, A., Chhabra, V. A., Kim, K.-H., and Tripathi, S. K. (2020). Recent developments on functional nanomaterial-based electrodes for microbial fuel cells. Renew. Sustain. Energy Rev. 119, 109551. doi:10.1016/j.rser.2019.109551
Kaur, R., Singh, S., Chhabra, V. A., Marwaha, A., Kim, K. H., and Tripathi, S. K. (2021). A sustainable approach towards utilization of plastic waste for an efficient electrode in microbial fuel cell applications. J. Hazard. Mat. 417, 125992. doi:10.1016/J.JHAZMAT.2021.125992
Kausar, A., Ahmad, I., Zhao, T., Maaza, M., and Bocchetta, P. (2023). Green nanocomposite electrodes/electrolytes for microbial fuel cells—cutting-edge technology. J. Compos. Sci. 7, 166. doi:10.3390/jcs7040166
Kumar, A., Siddiqui, T., Pandit, S., Roy, A., Gacem, A., Souwaileh, A. A, et al. (2023a). Application of biogenic TiO2 nanoparticles as ORR catalysts on cathode for enhanced performance of microbial fuel cell. Catalysts 13, 937. doi:10.3390/catal13060937
Kumar, D., Geetanjali,, , and Kundu, P. P. (2023b). Poly (acrylamide-co-acrylonitrile) hydrogel-derived iron-doped carbon foam electrocatalyst for enhancing oxygen reduction reaction in microbial fuel cell. Int. J. Hydrogen Energy 48, 7884–7895. doi:10.1016/j.ijhydene.2022.11.109
Kumar, P., Chatterjee, P., and Ghangrekar, M. M. (2017). Fouling resistant nitrogen doped carbon powder with amino-trimethylene-phosphate cathode for microbial fuel cell. Mat. Renew. sustain. Energy 6, 9. doi:10.1007/s40243-017-0093-5
Kun, G., Hassett, D., Gu, T., and Arora, R. (2012). Microbial fuel cells: electricity generation from organic wastes by microbes. CABI International.
Lai, B., Xiao, Z., Jiang, P., Xie, Y., Li, N., and Liu, Z. (2022). Two-dimensional Ag−Fe−N/C nanosheets as efficient cathode catalyst to improve power-generation performance of microbial fuel cells. ChemElectroChem 9, e202101699. doi:10.1002/celc.202101699
Lee, S. H., Ban, J. Y., Oh, C. H., Park, H. K., and Choi, S. (2016). A solvent-free microbial-activated air cathode battery paper platform made with pencil-traced graphite electrodes. Sci. Rep. 6, 28588. doi:10.1038/srep28588
Li, B., He, Z., Wang, M., and Wang, X. (2017). PtSnP/C and PtSn/C as efficient cathode catalysts for oxygen reduction reaction in microbial fuel cells. Int. J. Hydrogen Energy 42, 5261–5271. doi:10.1016/j.ijhydene.2017.01.087
Li, C., Yi, K., Hu, S., and Yang, W. (2023). Cathodic biofouling control by microbial separators in air-breathing microbial fuel cells. Environ. Sci. Ecotechnology 15, 100251. doi:10.1016/j.ese.2023.100251
Li, D., Qu, Y., Liu, J., He, W., Wang, H., and Feng, Y. (2014). Using ammonium bicarbonate as pore former in activated carbon catalyst layer to enhance performance of air cathode microbial fuel cell. J. Power Sources 272, 909–914. doi:10.1016/j.jpowsour.2014.09.053
Li, H., Ma, H., Liu, T., Ni, J., and Wang, Q. (2019). An excellent alternative composite modifier for cathode catalysts prepared from bacterial cellulose doped with Cu and P and its utilization in microbial fuel cell. Bioresour. Technol. 289, 121661. doi:10.1016/J.BIORTECH.2019.121661
Li, H., Sun, Y., Wang, J., Liu, Y., and Li, C. (2022a). Nanoflower-branch LDHs and CoNi alloy derived from electrospun carbon nanofibers for efficient oxygen electrocatalysis in microbial fuel cells. Appl. Catal. B Environ. 307, 121136. doi:10.1016/j.apcatb.2022.121136
Li, H., Zhang, X., Qin, Y., Liu, Y., Wang, J., Peng, L., et al. (2021a). Crafting controllable Fe-based hierarchically organic-frameworks from bacterial cellulose nanofibers for efficient electrocatalysts in microbial fuel cells. J. Power Sources 512, 230522. doi:10.1016/J.JPOWSOUR.2021.230522
Li, J. C., Wu, X. T., Chen, L. J., Li, N., and Liu, Z. Q. (2018a). Bifunctional MOF-derived Co-N-doped carbon electrocatalysts for high-performance zinc-air batteries and MFCs. Energy 156, 95–102. doi:10.1016/J.ENERGY.2018.05.096
Li, J., Qian, J., Chen, X., Zeng, X., Li, L., Ouyang, B., et al. (2022b). Three-dimensional hierarchical graphitic carbon encapsulated CoNi alloy/N-doped CNTs/carbon nanofibers as an efficient multifunctional electrocatalyst for high-performance microbial fuel cells. Compos. Part B Eng. 231, 109573. doi:10.1016/j.compositesb.2021.109573
Li, M., Zhang, H., Xiao, T., Wang, S., Zhang, B., Chen, D., et al. (2018b). Low-cost biochar derived from corncob as oxygen reduction catalyst in air cathode microbial fuel cells. Electrochim. Acta 283, 780–788. doi:10.1016/j.electacta.2018.07.010
Li, S., Zhu, X., Yu, H., Wang, X., Liu, X., Yang, H., et al. (2021b). Simultaneous sulfamethoxazole degradation with electricity generation by microbial fuel cells using Ni-MOF-74 as cathode catalysts and quantification of antibiotic resistance genes. Environ. Res. 197, 111054. doi:10.1016/J.ENVRES.2021.111054
Liang, B., Li, K., Liu, Y., and Kang, X. (2019). Nitrogen and phosphorus dual-doped carbon derived from chitosan: an excellent cathode catalyst in microbial fuel cell. Chem. Eng. J. 358, 1002–1011. doi:10.1016/J.CEJ.2018.09.217
Liang, B., Su, M., Zhao, Z., He, H., Lin, S., and Liang, S. (2023). Fe, N-codoped carbon derived from different ligands for oxygen reduction reaction in air-cathode microbial fuel cells: performance comparison and the associated mechanism. Electrochim. Acta 462, 142779. doi:10.1016/j.electacta.2023.142779
Liang, B., Zhao, Y., Li, K., and Lv, C. (2020). Porous carbon codoped with inherent nitrogen and externally embedded cobalt nanoparticles as a high-performance cathode catalyst for microbial fuel cells. Appl. Surf. Sci. 505, 144547. doi:10.1016/J.APSUSC.2019.144547
Liew, K. B., Wan Daud, W. R., Ghasemi, M., Loh, K. S., Ismail, M., Lim, S. S., et al. (2015). Manganese oxide/functionalised carbon nanotubes nanocomposite as catalyst for oxygen reduction reaction in microbial fuel cell. Int. J. Hydrogen Energy 40, 11625–11632. doi:10.1016/j.ijhydene.2015.04.030
Lin, Z., Su, W., Zhang, S., Zhang, M., Li, K., and Liu, J. (2021). Co2P embedded in nitrogen-doped carbon nanoframework derived from Co-based metal-organic framework as efficient oxygen reduction reaction electrocatalyst for enhanced performance of activated carbon air-cathode microbial fuel cell. J. Electroanal. Chem. 895, 115355. doi:10.1016/J.JELECHEM.2021.115355
Lin, Z., Yang, A., Zhang, B., Liu, B., Zhu, J., Tang, Y., et al. (2022). Coupling the atomically dispersed Fe-N 3 sites with sub-5 nm Pd nanocrystals confined in N-doped carbon nanobelts to boost the oxygen reduction for microbial fuel cells. Adv. Funct. Mat. 32, 2107683. doi:10.1002/adfm.202107683
Liu, H., and Logan, E. B. (2004). Electricity generation using an air-cathode single chamber microbial fuel cell in the presence and absence of a proton exchange membrane. Environ. Sci. Technol. 38, 4040–4046. doi:10.1021/es0499344
Liu, J., Chu, C., Wei, L., Feng, J., and Shen, J. (2023). Iron/cobalt-decorated nitrogen-rich 3D layer-stacked porous biochar as high-performance oxygen reduction air-cathode catalyst in microbial fuel cell. Biosens. Bioelectron. 222, 114926. doi:10.1016/j.bios.2022.114926
Liu, Q., Chen, S., Zhou, Y., Zheng, S., Hou, H., and Zhao, F. (2014). Phosphorus-doped carbon derived from cellulose phosphate as efficient catalyst for air-cathode in microbial fuel cells. J. Power Sources 261, 245–248. doi:10.1016/j.jpowsour.2014.03.060
Liu, X. W., Sun, X. F., Huang, Y. X., Sheng, G. P., Wang, S. G., and Yu, H. Q. (2011). Carbon nanotube/chitosan nanocomposite as a biocompatible biocathode material to enhance the electricity generation of a microbial fuel cell. Energy Environ. Sci. 4, 1422–1427. doi:10.1039/c0ee00447b
Liu, Y., Fan, Y. S., and Liu, Z. M. (2019). Pyrolysis of iron phthalocyanine on activated carbon as highly efficient non-noble metal oxygen reduction catalyst in microbial fuel cells. Chem. Eng. J. 361, 416–427. doi:10.1016/J.CEJ.2018.12.105
Liu, Y., Guo, S., Wang, J., and Li, C. (2022). Fundamental development and research of cathodic compartment in microbial fuel cells: A review. J. Environ. Chem. Eng. 10, 107918. doi:10.1016/j.jece.2022.107918
Liu, Y., Li, K., Ge, B., Pu, L., and Liu, Z. (2016). Influence of micropore and mesoporous in activated carbon air-cathode catalysts on oxygen reduction reaction in microbial fuel cells. Electrochim. Acta 214, 110–118. doi:10.1016/j.electacta.2016.08.034
Liu, Y., Li, K., Liu, Y., Pu, L., Chen, Z., and Deng, S. (2015). The high-performance and mechanism of P-doped activated carbon as a catalyst for air-cathode microbial fuel cells. J. Mat. Chem. A 3, 21149–21158. doi:10.1039/c5ta04595a
Liu, Y., Zhao, Y., Li, K., Wang, Z., Tian, P., Liu, D., et al. (2018). Activated carbon derived from chitosan as air cathode catalyst for high performance in microbial fuel cells. J. Power Sources 378, 1–9. doi:10.1016/J.JPOWSOUR.2017.12.019
Liu, Z., Liu, J., Zhang, S., and Su, Z. (2008). A novel configuration of microbial fuel cell stack bridged internally through an extra cation exchange membrane. Biotechnol. Lett. 30, 1017–1023. doi:10.1007/s10529-008-9658-9
Logan, B., Cheng, S., Watson, V., and Estadt, G. (2007). Graphite fiber brush anodes for increased power production in air-cathode microbial fuel cells. Environ. Sci. Technol. 41, 3341–3346. doi:10.1021/es062644y
Logan, B. E., and Regan, J. M. (2006). Microbial fuel cells - challenges and applications. Environ. Sci. Technol. 40, 5172–5180. doi:10.1021/es0627592
Logan, E. B., Hamelers, B., Rozendal, R., Schröder, U., Keller, J., Freguia, S., et al. (2006). Microbial fuel cells: methodology and technology. Environ. Sci. Technol. 40, 5181–5192. doi:10.1021/es0605016
Long, P., Qin, M., Zhang, B., Liu, Q., Zhao, F., Wu, Z., et al. (2023). Nano-flower like CoFe-layered double hydroxide@reduced graphene oxide with efficient oxygen reduction reaction for high-power air-cathode microbial fuel cells. Carbon N. Y. 212, 118088. doi:10.1016/j.carbon.2023.118088
Long, X., Cao, X., Song, H., Nishimura, O., and Li, X. (2019). Characterization of electricity generation and microbial community structure over long-term operation of a microbial fuel cell. Bioresour. Technol. 285, 121395. doi:10.1016/J.BIORTECH.2019.121395
Lu, J., Ren, L., Li, C., and Liu, H. (2023). Three-dimensional hierarchical flower-like bimetallic–organic materials in situ grown on carbon cloth and doped with sulfur as an air cathode in a microbial fuel cell. New J. Chem. 47, 2068–2078. doi:10.1039/D2NJ05476K
Ma, J., Zhang, J., Zhang, Y., Guo, Q., Hu, T., Xiao, H., et al. (2023). Progress on anodic modification materials and future development directions in microbial fuel cells. J. Power Sources 556, 232486. doi:10.1016/j.jpowsour.2022.232486
Ma, Z., Cano, Z. P., Yu, A., Chen, Z., Jiang, G., Fu, X., et al. (2020). Enhancing oxygen reduction activity of Pt-based electrocatalysts: from theoretical mechanisms to practical methods. Angew. Chem. Int. Ed. 59, 18334–18348. doi:10.1002/anie.202003654
Majidi, M. R., Shahbazi Farahani, F., Hosseini, M., and Ahadzadeh, I. (2019). Low-cost nanowired α-MnO2/C as an ORR catalyst in air-cathode microbial fuel cell. Bioelectrochemistry 125, 38–45. doi:10.1016/j.bioelechem.2018.09.004
Maruyama, J., Okamura, J., Miyazaki, K., and Abe, I. (2007). Two-step carbonization as a method of enhancing catalytic properties of hemoglobin at the fuel cell cathode. J. Phys. Chem. C 111, 6597–6600. doi:10.1021/jp071451+
Mecheri, B., Ficca, V. C. A., Costa de Oliveira, M. A., D’Epifanio, A., Placidi, E., Arciprete, F., et al. (2018). Facile synthesis of graphene-phthalocyanine composites as oxygen reduction electrocatalysts in microbial fuel cells. Appl. Catal. B Environ. 237, 699–707. doi:10.1016/J.APCATB.2018.06.031
Mecheri, B., Iannaci, A., D’Epifanio, A., Mauri, A., and Licoccia, S. (2016). Carbon-supported zirconium oxide as a cathode for microbial fuel cell applications. Chempluschem 81, 80–85. doi:10.1002/cplu.201500347
Meng, K., Liu, Q., Huang, Y., and Wang, Y. (2015). Facile synthesis of nitrogen and fluorine co-doped carbon materials as efficient electrocatalysts for oxygen reduction reactions in air-cathode microbial fuel cells. J. Mat. Chem. A 3, 6873–6877. doi:10.1039/c4ta06500j
Merino-Jimenez, I., Gonzalez-Juarez, F., Greenman, J., and Ieropoulos, I. (2019). Effect of the ceramic membrane properties on the microbial fuel cell power output and catholyte generation. J. Power Sources 429, 30–37. doi:10.1016/J.JPOWSOUR.2019.04.043
Mian, M. M., Liu, G., and Fu, B. (2019). Conversion of sewage sludge into environmental catalyst and microbial fuel cell electrode material: a review. Sci. Total Environ. 666, 525–539. doi:10.1016/j.scitotenv.2019.02.200
Mier, A. A., Olvera-Vargas, H., Mejía-López, M., Longoria, A., Verea, L., Sebastian, P. J., et al. (2021). A review of recent advances in electrode materials for emerging bioelectrochemical systems: from biofilm-bearing anodes to specialized cathodes. Chemosphere 283, 131138. doi:10.1016/j.chemosphere.2021.131138
Mukherjee, A., Patel, V., Shah, M. T., Jadhav, D. A., Munshi, N. S., Chendake, A. D., et al. (2022). Effective power management system in stacked microbial fuel cells for onsite applications. J. Power Sources 517, 230684. doi:10.1016/j.jpowsour.2021.230684
Munoz-Cupa, C., Hu, Y., Xu, C., and Bassi, A. (2021). An overview of microbial fuel cell usage in wastewater treatment, resource recovery and energy production. Sci. Total Environ. 754, 142429. doi:10.1016/j.scitotenv.2020.142429
Nancharaiah, Y. V., Venkata Mohan, S., and Lens, P. N. L. (2015). Metals removal and recovery in bioelectrochemical systems: A review. Bioresour. Technol. 195, 102–114. doi:10.1016/j.biortech.2015.06.058
Noori, M. T., Mukherjee, C. K., and Ghangrekar, M. M. (2017). Enhancing performance of microbial fuel cell by using graphene supported V2O5-nanorod catalytic cathode. Electrochim. Acta 228, 513–521. doi:10.1016/J.ELECTACTA.2017.01.016
Noori, M. T., and Verma, N. (2019). Cobalt - iron phthalocyanine supported on carbide - derived carbon as an excellent oxygen reduction reaction catalyst for microbial fuel cells. Electrochim. Acta 298, 70–79. doi:10.1016/J.ELECTACTA.2018.12.056
Obileke, K., Onyeaka, H., Meyer, E. L., and Nwokolo, N. (2021). Microbial fuel cells, a renewable energy technology for bio-electricity generation: a mini-review. Electrochem. Commun. 125, 107003. doi:10.1016/j.elecom.2021.107003
Oguz Koroglu, E., Civelek Yoruklu, H., Demir, A., and Ozkaya, B. (2019). “Chapter 3.9 - scale-up and commercialization issues of the MFCs: challenges and implications,” in Microbial electrochemical technology biomass, biofuels and biochemicals. Editors S. V. Mohan, S. Varjani, and A. Pandey (Elsevier), 565–583. doi:10.1016/B978-0-444-64052-9.00023-6
Oh, S.-E., and Logan, B. E. (2007). Voltage reversal during microbial fuel cell stack operation. J. Power Sources 167, 11–17. doi:10.1016/j.jpowsour.2007.02.016
Om Prakash, M., Raghavendra, G., Ojha, S., and Panchal, M. (2021). Characterization of porous activated carbon prepared from arhar stalks by single step chemical activation method. Mat. Today Proc. 39, 1476–1481. doi:10.1016/J.MATPR.2020.05.370
Park, H. I, Mushtaq, U., Perello, D., Lee, I., Cho, S. K., Star, A., et al. (2007). Effective and low-cost platinum electrodes for microbial fuel cells deposited by electron beam evaporation. Energy Fuels 21, 2984–2990. doi:10.1021/ef070160x
Peera, S. G., Maiyalagan, T., Liu, C., Ashmath, S., Lee, T. G., Jiang, Z., et al. (2021). A review on carbon and non-precious metal based cathode catalysts in microbial fuel cells. Int. J. Hydrogen Energy 46, 3056–3089. doi:10.1016/J.IJHYDENE.2020.07.252
Pema, T., Kumar, A., Tripathi, B., Pandit, S., Chauhan, S., Singh, S., et al. (2023). Investigating the performance of lithium-doped bismuth ferrite [BiFe1−xLixO3]-graphene nanocomposites as cathode catalyst for the improved power output in microbial fuel cells. Catalysts 13, 618. doi:10.3390/catal13030618
Pepè Sciarria, T., de Oliveira, M. A. C., Mecheri, B., D’Epifanio, A., Goldfarb, J. L., and Adani, F. (2020). Metal-free activated biochar as an oxygen reduction reaction catalyst in single chamber microbial fuel cells. J. Power Sources 462, 228183. doi:10.1016/J.JPOWSOUR.2020.228183
Pötschke, L., Huber, P., Stegschuster, G., Schriever, S., Kroppen, N., Schmatz, J., et al. (2022). Customized woven carbon fiber electrodes for bioelectrochemical systems—a study of structural parameters. Front. Chem. Eng. 4, 765682. doi:10.3389/fceng.2022.765682
Potter, M. C. (1911). Electrical effects accompanying the decomposition of organic compounds. Proc. R. Soc. Lond. Ser. B, Contain. Pap. a Biol. Character 84, 260–276. doi:10.1098/rspb.1911.0073
Prasad, J., and Tripathi, R. K. (2021). Effect of sediment microbial fuel cell stacks on 9 V/12 V DC power supply. Int. J. Hydrogen Energy 46, 14628–14638. doi:10.1016/j.ijhydene.2020.07.187
Priya, A. K., Subha, C., Kumar, P. S., Suresh, R., Rajendran, S., Vasseghian, Y., et al. (2022). Advancements on sustainable microbial fuel cells and their future prospects: A review. Environ. Res. 210, 112930. doi:10.1016/j.envres.2022.112930
Priyadarshini, M., Ahmad, A., Das, S., and Ghangrekar, M. M. (2021). Metal organic frameworks as emergent oxygen-reducing cathode catalysts for microbial fuel cells: A review. Int. J. Environ. Sci. Technol. 19, 11539–11560. doi:10.1007/s13762-021-03499-5
Qin, Y., Li, H., Sun, Y., Guo, S., Liu, Y., Zhai, Z., et al. (2023). Directional assembly of multi-catalytic sites CoCu-MOFs with porous carbon nanofiber templates as efficient catalyst for microbial fuel cells. J. Environ. Chem. Eng. 11, 109662. doi:10.1016/j.jece.2023.109662
Quan, X., Mei, Y., Xu, H., Sun, B., and Zhang, X. (2015). Optimization of Pt-Pd alloy catalyst and supporting materials for oxygen reduction in air-cathode microbial fuel cells. Electrochim. Acta 165, 72–77. doi:10.1016/j.electacta.2015.02.235
Rabaey, K., and Verstraete, W. (2005). Microbial fuel cells: novel biotechnology for energy generation. Trends Biotechnol. 23, 291–298. doi:10.1016/j.tibtech.2005.04.008
Rezaei, A., Karami, Z., Feli, F., and Aber, S. (2023). Oxygen reduction reaction enhancement in microbial fuel cell cathode using cesium phosphomolybdate electrocatalyst. Fuel 352, 129040. doi:10.1016/j.fuel.2023.129040
Rismani-Yazdi, H., Carver, S. M., Christy, A. D., and Tuovinen, O. H. (2008). Cathodic limitations in microbial fuel cells: an overview. J. Power Sources 180, 683–694. doi:10.1016/j.jpowsour.2008.02.074
Rossi, R., Evans, P. J., and Logan, B. E. (2019). Impact of flow recirculation and anode dimensions on performance of a large scale microbial fuel cell. J. Power Sources 412, 294–300. doi:10.1016/J.JPOWSOUR.2018.11.054
Rout, S., Nayak, A. K., Varanasi, J. L., Pradhan, D., and Das, D. (2018). Enhanced energy recovery by manganese oxide/reduced graphene oxide nanocomposite as an air-cathode electrode in the single-chambered microbial fuel cell. J. Electroanal. Chem. 815, 1–7. doi:10.1016/J.JELECHEM.2018.03.002
Rozendal, R. A., Hamelers, H. V. M., Euverink, G. J. W., Metz, S. J., and Buisman, C. J. N. (2006). Principle and perspectives of hydrogen production through biocatalyzed electrolysis. Int. J. Hydrogen Energy 31, 1632–1640. doi:10.1016/j.ijhydene.2005.12.006
Santoro, C., Kodali, M., Kabir, S., Soavi, F., Serov, A., and Atanassov, P. (2017). Three-dimensional graphene nanosheets as cathode catalysts in standard and supercapacitive microbial fuel cell. J. Power Sources 356, 371–380. doi:10.1016/J.JPOWSOUR.2017.03.135
Santoro, C., Kodali, M., Shamoon, N., Serov, A., Soavi, F., Merino-Jimenez, I., et al. (2019). Increased power generation in supercapacitive microbial fuel cell stack using FeNC cathode catalyst. J. Power Sources 412, 416–424. doi:10.1016/j.jpowsour.2018.11.069
Sarma, P. J., Malakar, B., and Mohanty, K. (2023). Self-sustaining bioelectricity generation in plant-based microbial fuel cells (PMFCs) with microalgae-assisted oxygen-reducing biocathode. Biomass Convers. Biorefinery. doi:10.1007/s13399-023-03848-z
Savizi, I. S. P., Kariminia, H.-R., and Bakhshian, S. (2012). Simultaneous decolorization and bioelectricity generation in a dual chamber microbial fuel cell using electropolymerized-enzymatic cathode. Environ. Sci. Technol. 46, 6584–6593. doi:10.1021/es300367h
Sawant, S. Y., Han, T. H., and Cho, M. H. (2017). Metal-free carbon-based materials: promising electrocatalysts for oxygen reduction reaction in microbial fuel cells. Int. J. Mol. Sci. 18, 25. doi:10.3390/ijms18010025
Sekhon, S. S., Kaur, P., and Park, J. S. (2021). From coconut shell biomass to oxygen reduction reaction catalyst: tuning porosity and nitrogen doping. Renew. Sustain. Energy Rev. 147, 111173. doi:10.1016/J.RSER.2021.111173
Shi, X., Feng, Y., Wang, X., Lee, H., Liu, J., Qu, Y., et al. (2012). Application of nitrogen-doped carbon powders as low-cost and durable cathodic catalyst to air-cathode microbial fuel cells. Bioresour. Technol. 108, 89–93. doi:10.1016/j.biortech.2011.12.078
Shin, S. H., Choi, Y., Na, S. H., Jung, S., and Kim, S. (2006). Development of bipolar plate stack type microbial fuel cells. Bull. Korean Chem. Soc. 27, 281–285. doi:10.5012/bkcs.2006.27.2.281
Shixuan, Z., Donghao, L., Jiwei, J., Li, F., and Hua, T. (2023). Oxygen reduction activity of a Pt-N4 single-atom catalyst prepared by electrochemical deposition and its bioelectrochemical application. Electrochim. Acta 437, 141543. doi:10.1016/j.electacta.2022.141543
Si, F., Zhang, Y., Yan, L., Zhu, J., Xiao, M., Liu, C., et al. (2014). “4 - electrochemical oxygen reduction reaction,” in Rotating electrode methods and oxygen reduction electrocatalysts. Editors W. Xing, G. Yin, and J. Zhang (Amsterdam: Elsevier), 133–170. doi:10.1016/B978-0-444-63278-4.00004-5
Slate, A. J., Whitehead, K. A., Brownson, D. A. C., and Banks, C. E. (2019). Microbial fuel cells: an overview of current technology. Renew. Sustain. Energy Rev. 101, 60–81. doi:10.1016/j.rser.2018.09.044
Subran, N., Ajit, K., Krishnan, H., Pachiyappan, S., and Ramaswamy, P. (2023). Synthesis and performance of a cathode catalyst derived from areca nut husk in microbial fuel cell. Chemosphere 312, 137303. doi:10.1016/j.chemosphere.2022.137303
Sui, S., Wang, X., Zhou, X., Su, Y., Riffat, S., and Liujun, C. (2017). A comprehensive review of Pt electrocatalysts for the oxygen reduction reaction: nanostructure, activity, mechanism and carbon support in PEM fuel cells. J. Mat. Chem. A 5, 1808–1825. doi:10.1039/C6TA08580F
Sun, M., Zhai, L.-F., Li, W.-W., and Yu, H.-Q. (2016a). Harvest and utilization of chemical energy in wastes by microbial fuel cells. Chem. Soc. Rev. 45, 2847–2870. doi:10.1039/C5CS00903K
Sun, Y., Duan, Y., Hao, L., Xing, Z., Dai, Y., Li, R., et al. (2016b). Cornstalk-derived nitrogen-doped partly graphitized carbon as efficient metal-free catalyst for oxygen reduction reaction in microbial fuel cells. ACS Appl. Mat. Interfaces 8, 25923–25932. doi:10.1021/acsami.6b06895
Sun, Y., Li, H., Wang, J., Liu, Y., Guo, S., Xie, H., et al. (2023). Enhanced oxygen reduction upon Ag-Fe-doped polyacrylonitrile@UiO-66-NH2 nanofibers to improve power-generation performance of microbial fuel cells. J. Colloid Interface Sci. 648, 654–663. doi:10.1016/j.jcis.2023.05.166
Tajdid Khajeh, R., Aber, S., and Zarei, M. (2020). Comparison of NiCo2O4, CoNiAl-LDH, and CoNiAl-LDH@NiCo2O4 performances as ORR catalysts in MFC cathode. Renew. Energy 154, 1263–1271. doi:10.1016/J.RENENE.2020.03.091
Tang, H., Cai, S., Xie, S., Wang, Z., Tong, Y., Pan, M., et al. (2015). Metal-organic-framework-derived dual metal-and nitrogen-doped carbon as efficient and robust oxygen reduction reaction catalysts for microbial fuel cells. Adv. Sci. 3, 1500265. doi:10.1002/advs.201500265
Tian, X., Zhou, M., Tan, C., Li, M., Liang, L., Li, K., et al. (2018). KOH activated N-doped novel carbon aerogel as efficient metal-free oxygen reduction catalyst for microbial fuel cells. Chem. Eng. J. 348, 775–785. doi:10.1016/J.CEJ.2018.05.007
Türk, K. K., Kruusenberg, I., Kibena-Põldsepp, E., Bhowmick, G. D., Kook, M., Tammeveski, K., et al. (2018). Novel multi walled carbon nanotube based nitrogen impregnated Co and Fe cathode catalysts for improved microbial fuel cell performance. Int. J. Hydrogen Energy 43, 23027–23035. doi:10.1016/J.IJHYDENE.2018.10.143
Tursun, H., Liu, R., Li, J., Abro, R., Wang, X., Gao, Y., et al. (2016). Carbon material optimized biocathode for improving microbial fuel cell performance. Front. Microbiol. 7, 6. doi:10.3389/fmicb.2016.00006
Vilas Boas, J., Oliveira, V. B., Marcon, L. R. C., Simões, M., and Pinto, A. M. F. R. (2019). Optimization of a single chamber microbial fuel cell using lactobacillus pentosus: influence of design and operating parameters. Sci. Total Environ. 648, 263–270. doi:10.1016/j.scitotenv.2018.08.061
Wang, D., Liu, H., Cao, Z., Cai, T., Han, P., Song, J., et al. (2022a). Ordered porous nitrogen-doped carbon with atomically dispersed FeN4 for efficient oxygen reduction reaction in microbial fuel cell. Sci. Total Environ. 838, 156186. doi:10.1016/j.scitotenv.2022.156186
Wang, D., Ma, Z., Xie, Y., Zhang, M., Zhao, N., and Song, H. (2018). Fe/N-doped graphene with rod-like CNTs as an air-cathode catalyst in microbial fuel cells. RSC Adv. 8, 1203–1209. doi:10.1039/c7ra11613f
Wang, H., and Ren, Z. J. (2013). A comprehensive review of microbial electrochemical systems as a platform technology. Biotechnol. Adv. 31, 1796–1807. doi:10.1016/j.biotechadv.2013.10.001
Wang, J., Mu, K., Zhao, X., Luo, D., Yu, X., Li, W., et al. (2021). Uniform distribution of Pd on GO-C catalysts for enhancing the performance of air cathode microbial fuel cell. Catalysts 11, 888. doi:10.3390/catal11080888
Wang, J., Tian, P., Li, K., Ge, B., Liu, D., Liu, Y., et al. (2016). The excellent performance of nest-like oxygen-deficient Cu1.5Mn1.5O4 applied in activated carbon air-cathode microbial fuel cell. Bioresour. Technol. 222, 107–113. doi:10.1016/J.BIORTECH.2016.09.126
Wang, K., Yang, J., Liu, W., Yang, H., Yi, W., Sun, Y., et al. (2022b). Self-nitrogen-doped carbon materials derived from microalgae by lipid extraction pretreatment: highly efficient catalyst for the oxygen reduction reaction. Sci. Total Environ. 821, 153155. doi:10.1016/j.scitotenv.2022.153155
Wang, X., Xu, H., Huang, S., Zeng, X., Li, L., Zhao, X., et al. (2023a). CoFe alloy nanoparticles embedded in vertically grown nanosheets on N-doped carbon nanofibers as a trifunctional electrocatalyst for high-performance microbial fuel cells. Appl. Surf. Sci. 609, 155452. doi:10.1016/j.apsusc.2022.155452
Wang, X., Yuan, T., Liang, J., Yang, J., Xie, Y., Li, L., et al. (2022c). FeNx nanoparticles embedded in three-dimensional N-doped carbon nanofibers as efficient oxygen reduction reaction electrocatalyst for microbial fuel cells in alkaline and neutral media. Int. J. Hydrogen Energy 47, 23608–23617. doi:10.1016/j.ijhydene.2022.05.176
Wang, X., Zhang, H., Ye, J., and Li, B. (2023b). Atomically dispersed Fe–N4 moieties in porous carbon as efficient cathode catalyst for enhancing the performance in microbial fuel cells. J. Power Sources 556, 232434. doi:10.1016/j.jpowsour.2022.232434
Wang, Y., Zhong, K., Huang, Z., Chen, L., Dai, Y., Zhang, H., et al. (2020). Novel g-C3N4 assisted metal organic frameworks derived high efficiency oxygen reduction catalyst in microbial fuel cells. J. Power Sources 450, 227681. doi:10.1016/J.JPOWSOUR.2019.227681
Wang, Z., Liu, Y., Li, K., Liu, D., Yang, T., Wang, J., et al. (2017). The influence and mechanism of different acid treatment to activated carbon used as air-breathing cathode catalyst of microbial fuel cell. Electrochim. Acta 246, 830–840. doi:10.1016/j.electacta.2017.05.086
Wei, J., Liang, P., Cao, X., and Huang, X. (2011). Use of inexpensive semicoke and activated carbon as biocathode in microbial fuel cells. Bioresour. Technol. 102, 10431–10435. doi:10.1016/J.BIORTECH.2011.08.088
Winfield, J., Ieropoulos, I., Rossiter, J., Greenman, J., and Patton, D. (2013). Biodegradation and proton exchange using natural rubber in microbial fuel cells. Biodegradation 24, 733–739. doi:10.1007/s10532-013-9621-x
Woodward, L., Perrier, M., Srinivasan, B., Pinto, R. P., and Tartakovsky, B. (2010). Comparison of real-time methods for maximizing power output in microbial fuel cells. AIChE J. 56, 2742–2750. doi:10.1002/aic.12157
Wu, Z. Y., Liang, H. W., Chen, L. F., Hu, B. C., and Yu, S. H. (2016). Bacterial cellulose: a robust platform for design of three dimensional carbon-based functional nanomaterials. Acc. Chem. Res. 49, 96–105. doi:10.1021/acs.accounts.5b00380
Xie, H., Jiang, D., Chen, H., Ma, X., Liu, X., Qi, Q., et al. (2023). Electron transfer and surface activity of NiCoP-wrapped MXene: cathodic catalysts for the oxygen reduction reaction. Nanoscale 15, 7430–7437. doi:10.1039/D3NR00192J
Xing, Z., Gao, N., Qi, Y., Ji, X., and Liu, H. (2017). Influence of enhanced carbon crystallinity of nanoporous graphite on the cathode performance of microbial fuel cells. Carbon N. Y. 115, 271–278. doi:10.1016/j.carbon.2017.01.014
Xu, X., Xie, J., Dai, Y., Yang, L., Cai, Z., Jing, B., et al. (2022). FeCo alloys in-situ formed in Co/Co2P/N-doped carbon as a durable catalyst for boosting bio-electrons-driven oxygen reduction in microbial fuel cells. Int. J. Hydrogen Energy 47, 3063–3074. doi:10.1016/j.ijhydene.2021.10.199
Xu, Y., Zhang, X., Liu, Y., Wei, Y., Lan, F., Wang, R., et al. (2023). Trace N-doped manganese dioxide cooperated with Ping-pong chrysanthemum-like NiAl-layered double hydroxide on cathode for improving bioelectrochemical performance of microbial fuel cell. Bioresour. Technol. 381, 129139. doi:10.1016/j.biortech.2023.129139
Xue, W., Zhou, Q., and Li, F. (2020). The feasibility of typical metal–organic framework derived Fe, Co, N co-doped carbon as a robust electrocatalyst for oxygen reduction reaction in microbial fuel cell. Electrochim. Acta 355, 136775. doi:10.1016/J.ELECTACTA.2020.136775
Yahia, S. A. A., Hamadou, L., Salar-García, M. J., Kadri, A., Ortiz-Martínez, V. M., Hernández-Fernández, F. J., et al. (2016). TiO 2 nanotubes as alternative cathode in microbial fuel cells: effect of annealing treatment on its performance. Appl. Surf. Sci. 387, 1037–1045. doi:10.1016/j.apsusc.2016.07.018
Yan, Z., Wang, M., Liu, J., Liu, R., and Zhao, J. (2014a). Glycerol-stabilized NaBH4 reduction at room-temperature for the synthesis of a carbon-supported PtxFe alloy with superior oxygen reduction activity for a microbial fuel cell. Electrochim. Acta 141, 331–339. doi:10.1016/j.electacta.2014.06.137
Yan, Z., Wang, M., Lu, Y., Liu, R., and Zhao, J. (2014b). Ethylene glycol stabilized NaBH4 reduction for preparation carbon-supported Pt-Co alloy nanoparticles used as oxygen reduction electrocatalysts for microbial fuel cells. J. Solid State Electrochem. 18, 1087–1097. doi:10.1007/s10008-013-2361-3
Yang, G., Sun, Y., Yuan, Z., Lü, P., Kong, X., Li, L., et al. (2014). Application of surface-modified carbon powder in microbial fuel cells. Chin. J. Catal. 35, 770–775. doi:10.1016/s1872-2067(14)60023-1
Yang, J., Yang, H., Wang, S., Wang, K., Sun, Y., Yi, W., et al. (2023). Importance of pyrolysis programs in enhancing the application of microalgae-derived biochar in microbial fuel cells. Fuel 333, 126244. doi:10.1016/j.fuel.2022.126244
Yang, L., Cai, Z., Hao, L., Ran, L., Xu, X., Dai, Y., et al. (2018). Increase of structural defects by N doping in MoS2 cross-linked with N-doped CNTs/carbon for enhancing charge transfer in oxygen reduction. Electrochim. Acta 283, 448–458. doi:10.1016/J.ELECTACTA.2018.06.152
Yang, W., Li, J., Lan, L., Zhang, Y., and Liu, H. (2020a). Covalent organic polymers derived carbon incorporated with cobalt oxides as a robust oxygen reduction reaction catalyst for fuel cells. Chem. Eng. J. 390, 124581. doi:10.1016/J.CEJ.2020.124581
Yang, W., Li, J., Ye, D., Zhu, X., and Liao, Q. (2017). Bamboo charcoal as a cost-effective catalyst for an air-cathode of microbial fuel cells. Electrochim. Acta 224, 585–592. doi:10.1016/J.ELECTACTA.2016.12.046
Yang, W., Peng, Y., Zhang, Y., Lu, J. E., Li, J., and Chen, S. (2019). Air cathode catalysts of microbial fuel cell by nitrogen-doped carbon aerogels. ACS Sustain. Chem. Eng. 7, 3917–3924. doi:10.1021/acssuschemeng.8b05000
Yang, W., Wang, X., Rossi, R., and Logan, B. E. (2020b). Low-cost Fe–N–C catalyst derived from Fe (III)-chitosan hydrogel to enhance power production in microbial fuel cells. Chem. Eng. J. 380, 122522. doi:10.1016/J.CEJ.2019.122522
Yap, K.-L., Ho, L.-N., Guo, K., Liew, Y.-M., Lutpi, N. A., Azhari, A. W., et al. (2023). Role of tin (IV) oxide as cathodic catalyst on wastewater treatment and bioelectricity generation in a baffled microbial fuel cell. IOP Conf. Ser. Earth Environ. Sci. 1135, 012007. doi:10.1088/1755-1315/1135/1/012007
Ye, W., Tang, J., Wang, Y., Cai, X., Liu, H., Lin, J., et al. (2019). Hierarchically structured carbon materials derived from lotus leaves as efficient electrocatalyst for microbial energy harvesting. Sci. Total Environ. 666, 865–874. doi:10.1016/J.SCITOTENV.2019.02.300
Yen, S. J., Tsai, M. C., Wang, Z. C., Peng, H. L., Tsai, C. H., and Yew, T. R. (2013). The improvement of catalytic efficiency by optimizing Pt on carbon cloth as a cathode of a microbial fuel cell. Electrochim. Acta 108, 241–247. doi:10.1016/j.electacta.2013.06.019
You, S., Zhao, Q., Zhang, J., Jiang, J., Wan, C., Du, M., et al. (2007). A graphite-granule membrane-less tubular air-cathode microbial fuel cell for power generation under continuously operational conditions. J. Power Sources 173, 172–177. doi:10.1016/j.jpowsour.2007.07.063
Yu, D., Bai, L., Zhai, J., Wang, Y., and Dong, S. (2017). Toxicity detection in water containing heavy metal ions with a self-powered microbial fuel cell-based biosensor. Talanta 168, 210–216. doi:10.1016/j.talanta.2017.03.048
Zerrouki, A., Kameche, M., Ait Amer, A., Tayeb, A., Moussaoui, D., and Innocent, C. (2022). Platinum nanoparticles embedded into polyaniline on carbon cloth: improvement of oxygen reduction at cathode of microbial fuel cell used for conversion of medicinal plant wastes into bio-energy. Environ. Technol. 43, 1359–1369. doi:10.1080/09593330.2020.1829088
Zha, Z., Zhang, Z., Xiang, P., Zhu, H., Zhou, B., Sun, Z., et al. (2021). One-step preparation of eggplant-derived hierarchical porous graphitic biochar as efficient oxygen reduction catalyst in microbial fuel cells. RSC Adv. 11, 1077–1085. doi:10.1039/D0RA09976G
Zhang, B., Wen, Z., Ci, S., Mao, S., Chen, J., and He, Z. (2014). Synthesizing nitrogen-doped activated carbon and probing its active sites for oxygen reduction reaction in microbial fuel cells. ACS Appl. Mat. Interfaces 6, 7464–7470. doi:10.1021/am5008547
Zhang, H., Shi, H., You, H., Su, M., Huang, L., Zhou, Z., et al. (2022a). Cu-doped CaFeO3 perovskite oxide as oxygen reduction catalyst in air cathode microbial fuel cells. Environ. Res. 214, 113968. doi:10.1016/j.envres.2022.113968
Zhang, L., Lu, Z., Li, D., Ma, J., Song, P., Huang, G., et al. (2016). Chemically activated graphite enhanced oxygen reduction and power output in catalyst-free microbial fuel cells. J. Clean. Prod. 115, 332–336. doi:10.1016/j.jclepro.2015.12.067
Zhang, Q., and Liu, L. (2020). A microbial fuel cell system with manganese dioxide/titanium dioxide/graphitic carbon nitride coated granular activated carbon cathode successfully treated organic acids industrial wastewater with residual nitric acid. Bioresour. Technol. 304, 122992. doi:10.1016/J.BIORTECH.2020.122992
Zhang, S., Su, W., Li, K., Liu, D., Wang, J., and Tian, P. (2018a). Metal organic framework-derived Co3O4/NiCo2O4 double-shelled nanocage modified activated carbon air-cathode for improving power generation in microbial fuel cell. J. Power Sources 396, 355–362. doi:10.1016/J.JPOWSOUR.2018.06.057
Zhang, S., Su, W., Wang, X., Li, K., and Li, Y. (2019a). Bimetallic metal-organic frameworks derived cobalt nanoparticles embedded in nitrogen-doped carbon nanotube nanopolyhedra as advanced electrocatalyst for high-performance of activated carbon air-cathode microbial fuel cell. Biosens. Bioelectron. 127, 181–187. doi:10.1016/J.BIOS.2018.12.028
Zhang, S., Su, W., Wei, Y., Liu, J., and Li, K. (2018b). Mesoporous MnO2 structured by ultrathin nanosheet as electrocatalyst for oxygen reduction reaction in air-cathode microbial fuel cell. J. Power Sources 401, 158–164. doi:10.1016/J.JPOWSOUR.2018.08.102
Zhang, S., Zhang, S., Liu, H., Li, L., and Guo, R. (2023). Fe-N-C-based cathode catalyst enhances redox reaction performance of microbial fuel cells: azo dyes degradation accompanied by electricity generation. J. Environ. Chem. Eng. 11, 109264. doi:10.1016/j.jece.2023.109264
Zhang, X., Li, K., Yan, P., Liu, Z., and Pu, L. (2015). N-type Cu2O doped activated carbon as catalyst for improving power generation of air cathode microbial fuel cells. Bioresour. Technol. 187, 299–304. doi:10.1016/j.biortech.2015.03.131
Zhang, X., Liang, B., Lin, Z., Zhong, M., Li, K., Wang, H., et al. (2022b). Engineering heterostructured Co0.7Fe0.3@Co doped leaf-like carbon nanoplates from dual metal-organic frameworks for high-efficiency oxygen reduction reaction in microbial fuel cell. J. Power Sources 520, 230799. doi:10.1016/j.jpowsour.2021.230799
Zhang, Y., Deng, L., Hu, H., Qiao, Y., Yuan, H., Chen, D., et al. (2020). Pomelo peel-derived, N-doped biochar microspheres as an efficient and durable metal-free ORR catalyst in microbial fuel cells. Sustain. Energy Fuels 4, 1642–1653. doi:10.1039/c9se00834a
Zhang, Y., Sun, J., Hu, Y., Li, S., and Xu, Q. (2012). Bio-cathode materials evaluation in microbial fuel cells: A comparison of graphite felt, carbon paper and stainless steel mesh materials. Int. J. Hydrogen Energy 37, 16935–16942. doi:10.1016/j.ijhydene.2012.08.064
Zhang, Y., Sun, J., Hu, Y., Li, S., and Xu, Q. (2013). Carbon nanotube-coated stainless steel mesh for enhanced oxygen reduction in biocathode microbial fuel cells. J. Power Sources 239, 169–174. doi:10.1016/j.jpowsour.2013.03.115
Zhang, Y., Tian, P., Li, K., Liu, Y., and Zhang, Z. (2018c). C3N4 coordinated metal-organic-framework-derived network as air-cathode for high performance of microbial fuel cell. J. Power Sources 408, 74–81. doi:10.1016/j.jpowsour.2018.10.036
Zhang, Y., Zhao, Y., and Zhou, M. (2019b). A photosynthetic algal microbial fuel cell for treating swine wastewater. Environ. Sci. Pollut. Res. 26, 6182–6190. doi:10.1007/s11356-018-3960-4
Zhao, C., Luo, W., Tian, H., Lu, T., Yi, L., Zhang, Y., et al. (2023). Single-atomic Fe sites decorated N-doped carbon toward oxygen reduction in MFCs. Chem. Commun. 59, 6749–6752. doi:10.1039/D3CC01415K
Zheng, L., Lin, X., Liu, Y., Li, H., Sun, Y., and Li, C. (2022). Synergistically enhanced oxygen reduction reaction and oxytetracycline mineralization by FeCoO/GO modified cathode in microbial fuel cell. Sci. Total Environ. 808, 151873. doi:10.1016/j.scitotenv.2021.151873
Zheng, T., Li, J., Ji, Y., Zhang, W., Fang, Y., Xin, F., et al. (2020). Progress and prospects of bioelectrochemical systems: electron transfer and its applications in the microbial metabolism. Front. Bioeng. Biotechnol. 8, 10. doi:10.3389/fbioe.2020.00010
Zhong, K., Li, M., Yang, Y., Zhang, H., Zhang, B., Tang, J., et al. (2019). Nitrogen-doped biochar derived from watermelon rind as oxygen reduction catalyst in air cathode microbial fuel cells. Appl. Energy 242, 516–525. doi:10.1016/j.apenergy.2019.03.050
Zhou, L., Fu, P., Cai, X., Zhou, S., and Yuan, Y. (2016). Naturally derived carbon nanofibers as sustainable electrocatalysts for microbial energy harvesting: A new application of spider silk. Appl. Catal. B Environ. 188, 31–38. doi:10.1016/J.APCATB.2016.01.063
Zhu, H., Zhang, Z., Zhou, Y., Jiang, X., Cai, F., Bai, Y., et al. (2022a). Porous Co, N co-doped carbon derived from tea residue as efficient cathode catalyst in microbial fuel cells for swine wastewater treatment and the microbial community analysis. J. Water Process Eng. 45, 102471. doi:10.1016/j.jwpe.2021.102471
Zhu, Q., Hu, J., Liu, B., Hu, S., Liang, S., Xiao, K., et al. (2022b). Recent advances on the development of functional materials in microbial fuel cells: from fundamentals to challenges and outlooks. ENERGY Environ. Mat. 5, 401–426. doi:10.1002/eem2.12173
Zhuang, S., Li, B., and Wang, X. (2023). Engineering the electronic structure of high performance FeCo bimetallic cathode catalysts for microbial fuel cell application in treating wastewater. Environ. Res. 216, 114542. doi:10.1016/j.envres.2022.114542
Keywords: microbial fuel cells (MFCs), oxygen reduction reaction (ORR), electrocatalysts, electricity production, wastewater treatment
Citation: Elangovan K, Saravanan P, Campos CH, Sanhueza-Gómez F, Khan MMR, Chin SY, Krishnan S and Viswanathan Mangalaraja R (2023) Outline of microbial fuel cells technology and their significant developments, challenges, and prospects of oxygen reduction electrocatalysts. Front. Chem. Eng. 5:1228510. doi: 10.3389/fceng.2023.1228510
Received: 24 May 2023; Accepted: 22 August 2023;
Published: 07 September 2023.
Edited by:
Oisik Das, Luleå University of Technology, SwedenReviewed by:
Hugo Olvera-Vargas, National Autonomous University of Mexico, MexicoArvind Kumar Mungray, Sardar Vallabhbhai National Institute of Technology, India
Rhoda Afriyie Mensah, Luleå University of Technology, Sweden
Copyright © 2023 Elangovan, Saravanan, Campos, Sanhueza-Gómez, Khan, Chin, Krishnan and Viswanathan Mangalaraja. This is an open-access article distributed under the terms of the Creative Commons Attribution License (CC BY). The use, distribution or reproduction in other forums is permitted, provided the original author(s) and the copyright owner(s) are credited and that the original publication in this journal is cited, in accordance with accepted academic practice. No use, distribution or reproduction is permitted which does not comply with these terms.
*Correspondence: Prabhu Saravanan, psaravanan@udec.cl; Cristian H. Campos, ccampos@udec.cl