Organic mass and protein extraction from secondary sewage sludge via multi-step physical alkali- and acid-based treatment
- 1Bio RE Ltd., Riga, Latvia
- 2Water Systems and Biotechnology Institute, Riga Technical University, Riga, Latvia
The perception of sewage sludge has been shifting from waste to resource, leading to various technological proposals for its management and resource recovery. This study explores a two-step sewage sludge treatment using different pathways—physical-alkali followed by physical-acid, and physical-acid followed by physical-alkali hydrolysis—to understand the efficiency of organic matter (OM) and Kjeldahl nitrogen extraction, and protein solubilization. Hydrolysis of the sewage sludge was performed with 3 M H2SO4 and 2.8 M NaOH and combined with physical treatment—thermal, ultrasonication, microwave irradiation, and cavitation. The results showed that cavitation chemical hydrolysis in an alkaline environment (CCH-alkali) extracted the highest amount of OM—up to 79.0%. When further cavitation chemical hydrolysis in an acid environment (CCH alkali–acid) was performed, OM extraction reached 90.2%. Physical-alkali treatment showed better performance in resource recovery from secondary sludge (SS) in both treatment steps. The highest protein extraction rate of 23,046 mg/L in the supernatant was obtained using SS treatment with microwave chemical hydrolysis in an alkaline environment (MCH-alkali). Although physical-acid treatment resulted in reduced protein solubilization and OM extraction, it provides a higher protein hydrolysis rate. Organic nitrogen compounds were better extracted with thermal-alkali treatment, reaching 95.3% removal. The study showed that different physical treatment methods demonstrate selective resource recovery or extraction performance.
1 Introduction
The sustainable management of sewage sludge from municipal wastewater treatment facilities remains a global issue, and, despite numerous technologies being introduced, it is not yet fully resolved. Based on published estimates and interpolation of missing data, the annual production of this sludge in EU-28 countries was estimated at 9.0 to 9.5 million tons of dry solids in 2016 (Eurostat, 2016). After a significant increase in sludge production observed in the 1990s, recent trends of sludge generation have tended to plateau in almost all European countries due to the construction of new wastewater treatment plants (WWTPs) that comply with EU Directive 91/271/EEC (Gianfredi et al., 2021). From total sludge generated in the WWTPs, biological treatment produces solid, semisolid, or slurry residual material known as wastewater secondary sludge (SS) (Hauduc et al., 2013). The dry solid content of SS can vary 4%–25%, depending on the SS treatment method used for dewatering—SS gravity thickening is the least efficient, and solid/liquid separation techniques, like centrifugal treatment and treatment in a belt, screw, or filter press, is the most effective (Wang et al., 2007). Despite the variable water content in excess sludge after treatment, dry matter content remains approximately the same and consists of pathogenic and non-pathogenic protozoa, metazoan, bacteria, filamentous fungi, and algae that are engulfed in various suspended solids (Amaral et al., 2004), inorganic substances, and persistent organic pollutants (Raheem et al., 2018). Sludge matrix is rich with organic polymers, including polysaccharides, nucleic acids, humic substances, and some lipid fractions. In addition to the organic fraction, SS inorganic fraction comprises various heavy metals (Raheem et al., 2018) which can result in secondary pollution if handled without precautions.
The estimated protein content in SS typically varies from 32%–41% of total solids (Capodaglio, 2023). Despite high variability, the extraction of proteins from SS is of high economic importance due to the amino acid profile and potential reuse. From all SS disintegration and hydrolysis techniques, thermal SS treatment in the presence of alkali or acid addition is the most often used (Devos et al., 2020). At the same time, these methods mostly focus on SS pre-treatment for further downstream anaerobic digestion and give minor attention to protein or other valuable material extraction from the sludge. Lately, an interest in the hydrolysis of SS to produce food supplements for animal feed (Lau, 1981; Xiao and Zhou, 2020), liquid fertilizers (Liu et al., 2009), or protein foaming agents (Collivignarelli et al., 2017) has arisen. The application of thermal–chemical treatment methods has resulted in the recovery of 46%–91.4% of the total available protein in SS (Xiao and Zhou, 2020; Gao et al., 2021; Hui et al., 2022a). Protein solubilization using ultrasonication produced 32–660 mg/L protein in the solution and microwave irradiation 50–1,900 mg/L in the hydrolysis mass. Combination with biological pre-treatment methods has increased the solubilized protein concentration from 50 to 200 mg/L in reaction mass. Furthermore, numerous combinations of different abovementioned techniques have resulted in 550 mg/L protein concentration with ultrasonic-acid treatment as the lowest yield and thermal-acid treatment of up to 8,100 mg/L as the highest in the reaction mass (Xiao and Zhou, 2020). Studies have also focused on OM recovery from SS in general, and applied alkaline treatment above pH 11. Up to 75% of available OM has been recovered from SS when using NaOH and stirring at room temperature (Becerra et al., 2010). It has also been concluded that using a low ultrasonic alkali treatment with a dose of 0.1 g NaOH/per 1 g of SS makes it possible to extract the OM fraction in a notable amount which can contain approximately 30.5% protein-like substances together with saccharide-type substances and humic acids (Li et al., 2016). Despite better OM extraction efficiencies being observed in alkali conditions, the exact mechanisms are still not well understood.
The assessment of organic nitrogen recovery during SS hydrolysis is estimated as total Kjeldahl nitrogen (TKN) since the major organic nitrogen amount is released during the protein degradation and Kjeldahl test (Bi et al., 2014a). Previously, a 48 h treatment of SS in an acid (pH 2 with HCl) or alkali (pH 12 with NaOH) environment and constant stirring resulted in a TKN concentration of 8.3 and 14.2 mg/g OM from SS, respectively (Tavares de Sousa et al., 2021). However, due to the availability of different analysis methods, data interpretation, and a high variability of SS samples used in past experiments, it is rather impossible to compare the results obtained by different treatment methods. Thus, a complex meta-analysis is required to compare and organize the data in a unified system for the different SS physicochemical treatment methods’ efficiency in extracting protein, organic matter, and other nitrogen compounds. This study tested all currently suggested treatment approaches in a unified system where a single standardized SS batch was subjected to a two-step physicochemical treatment using thermal–chemical, ultrasonic-chemical, cavitation–chemical, and microwave–chemical treatment in acidic and alkali environments to estimate the boundaries of material recovery. Although protein extraction has been viewed as the main process parameter, other biopolymers present in SS can mineralize or are transferred into the liquid phase during the treatment. This in turn affects protein extraction, modifies the sludge matrix, and changes the composition of the hydrolysis mass. To monitor the transfer of biopolymers and avoid decreased product concentration, it is crucial to control organic mass transfer and TKN content. Thus, a comprehensive methodological setup with the subsequent validation of extraction, protein solubilization, and OM and Kjeldahl nitrogen extraction from SS was made to compare the efficiencies of the treatments. Moreover, since, after the first physicochemical treatment step of SS, there was still much organic rich material remaining in the centrifuged sludge pellets, it is essential to understand if changes in the chemical environment increase the extraction efficiency of protein and determine the total extractable protein yield from SS.
2 Materials and methods
2.1 Wastewater secondary sludge characteristics used in the study
To ensure uniform results, a single batch of SS was used in all tests performed and was stored at 4°C. Preliminary studies have shown that, although some changes in the feed material can occur, the effect is not significant (p > 0.05), especially on the extracted protein outcome. For analyses, centrifuged SS after aerobic process was collected from the municipal WWTP with a wastewater treatment capacity of 5,000 m3/day with approximately 60% of the capacity from domestic sources and 40% from industry, including chemical, pharmaceutical, and non-food production sites in the municipal area. The collected sample was well-mixed and monitored every time to remain at a constant temperature (4 C). No significant changes in the dry solid content of SS during the experiments were observed. All tests were performed over 43 days. The composition of SS used in first-step treatment was: dry matter 14.8% ± 0.3%, ash 3.8% ± 0.2% and volatile solid content 11.1% ± 0.2%, and Kjeldahl nitrogen content from OM 6.3% ± 0.2%. Centrifuged sludge material (pellet, 15%–16% solids) from the corresponding first-step treatment was taken as the feed material for the secondary treatment in a quantity proportional to the dry matter content in original SS 14.8% dry matter.
All further experiments and analyses were performed in triplicate.
2.2 Treatment setup
The following physicochemical hydrolysis methods were applied to the SS: temperature-based chemical hydrolysis (TCH), hydrodynamic-cavitation-induced chemical hydrolysis (CCH), ultrasonic-assisted chemical hydrolysis (UCH), and microwave-enhanced chemical hydrolysis (MCH). To maximize the extraction of OM from SS, hydrolysis was conducted in two distinct steps. The first involved utilizing a selected physical treatment method and chemical conditions, while the second step involved altering the chemical environment while maintaining the same physical treatment method and conditions. All technical parameters selected for the treatment conditions were estimated according to the exploitation abilities of the equipment used and method-optimization preliminary tests.
In a preliminary study, the concentrations of H2SO4 and NaOH were assessed for their suitability as extraction agents. Following this investigation, concentrations of 3 M H2SO4 and 2.8 M NaOH were chosen due to their consistent performance in hydrolysis processes. Subsequent potentiometric titration experiments revealed that the pKa values of the hydrolysis mass were similar across all experiments conducted. The pKa values for the reaction masses, as determined through potentiometric titration, exhibited ranges of 3.5–4.5 and 7.8–8.5, with a smaller maximum in titration curve of 10.9–11.2.
The hydrolysis mass obtained from the physical–chemical treatment underwent centrifugation at 4,000 rpm for 20 min to ensure effective phase separation. Subsequently, the supernatant was analyzed for protein concentration and subjected to formol titration. Meanwhile, the resulting pellets were examined for OM loss.
To adhere to the chosen treatment method, the methods were abbreviated using a consistent format where the first three capital letters denote the physical hydrolysis method, followed by the chemical environment. For example, if the treatment method is thermal treatment followed by chemical hydrolysis and it is performed in an alkali environment, it will be abbreviated as TCH-alkali, and if the produced pellets are subjected to further treatment in a second step, the abbreviation will include both chemical treatment names in the respective treatment order, such as TCH alkali–acid.
2.2.1 Thermal chemical hydrolysis (TCH-alkali/TCH-acid/TCH alkali–acid/TCH acid–alkali)
In the first hydrolysis step, 600 g of SS was inserted in a glass beaker and supplied with 300 mL of either 3 M H2SO4 or 2.8 M NaOH to provide an acid or alkali environment (Figure 1). The sample was then shaken in an orbital shaker (150 rpm, OHAUS, Germany) for 3 h at 80 C. Evaporated water was constantly added to sustain the same reaction volume. Due to mass reduction after centrifugation and the first hydrolysis, only 200 g of pellets were used in the second hydrolysis step and 100 mL of extraction agent to keep the same concentration.
2.2.2 Ultrasound chemical hydrolysis (UCH-alkali/UCH-acid/UCH alkali–acid/UCH acid–alkali)
Ultrasonication was performed in an ultrasonication bath (PS-10A, ROHS) at 79 W, 40 kHz. A measure of 300 g SS or 100 g of centrifugation pellets after primary hydrolysis were diluted with 150 mL or 50 mL of 3 M H2SO4 or 2.8 NaOH solution, respectively, and placed in the ultrasonication bath with mechanical stirring at 150 rpm for 2 h. The recorded sample temperature did not increase above 40 C.
2.2.3 Cavitation chemical hydrolysis (CCH-alkali/CCH-acid/CCH alkali–acid/CCH acid–alkali)
For cavitation treatment, 600 g of SS were first treated with 300 mL acid or alkali solution and then stirred at 22,000 rpm for 30 min with a laboratory rotor–stator homogenizer (500 W, Fluco FA25D) equipped with a straight rotary blade. The reaction mass spontaneously warmed to 70–80°С. A further 200 g of centrifugated pellets were again diluted with 100 mL of 3 M H2SO4 or 2.8 NaOH solution according to the treatment pathway and repeatedly treated with cavitation for 30 min at 22,000 rpm. During the second cavitation treatment, the reaction mass heated to the same temperature.
2.2.4 Microwave chemical hydrolysis (MCH-alkali/MCH-acid/MCH alkali–acid/MCH acid–alkali)
Samples were treated in an industrial microwave oven (EMS 200, Korber) at 650 W. We placed 600 g of SS in an oven for 15 min where the mass heated to 95°C. After the microwave exposure, 300 mL of 3 M H2SO4 or 2.8 NaOH solution was added to the hot mass and stirred mechanically for 30 min with a pedal stirrer (Fluko 200) until the mass reached room temperature. For the secondary hydrolysis, 200 g of centrifugation pellets where again exposed to the same strength microwaves for 10 min, then again mixed with 100 mL of the corresponding acid or alkali solution, and stirred till the mass reached room temperature.
2.3 Analytical methods
Measurements of absolute protein concentration in the SS were challenging due to limitations in existing standards and process inhibitors originating from the substrate itself. Even the most robust techniques require pre-treatment or purification of the samples (Redmile-Gordon et al., 2013). Alternatively, only a rough estimate of the actual protein and oligopeptide content in the solution could be made, since alternative methods are inaccurate for determining the size and shape of the proteins (Simonian and Smith, 2006). The method by Lowry et al. (1951) is no exception; However, due to its widespread use in similar research and moderate sensitivity to various matrices, a Lowry protein assay was an acceptable substitute for precise protein concentration detection in the sludge hydrolysis mass (Waterborg, 2009). The protein extraction could be tracked by total Kjeldahl nitrogen shift. While total protein is recalculated from this analysis using a coefficient, this value can vary in different protein samples, and the coefficient is usually determined according to amino acid content (Mariotti et al., 2008). Since the approximate amino acid content remains unknown for the samples, it is also not possible to select an adequate coefficient since SS contains not only proteins but other nitrogen compounds. For example, 12%–30% of the cell dry mass is nucleic acids, and the nitrogen content in the nucleotides has been estimated approximately 16%–17% from total solids (Farrell et al., 2004).
Therefore, a Lowry assay was used instead. However, Kjeldahl nitrogen is still a suitable parameter for following the nitrogen extraction rate. To obtain more information about the protein hydrolysis rate, Sorensen’s formol titration was used. It provides information about the end carboxylic group concentration present in a hydrolysis sample (Taylor, 1957): the higher the carboxylic acid concentration relative to protein concentration, the higher the extracted protein hydrolysis rate.
2.3.1 Gravimetric analyses
Gravimetric analysis was conducted on SS and hydrolysis mixture pellets following a standardized method for total dry solids and volatile solids. Volatile solids in this study are referred to as OM (APHA, 2012). In summary, to determine dry matter content, samples were placed in a drying oven (Memmert UF750) at 105 C for 12 h or until a constant mass was achieved. Ash content was determined by heating the samples to 550°C (Snoll 7000) for 5 h or until a constant mass was obtained. Volatile solids were calculated as the difference between the dry matter and ash content.
2.3.2 Protein concentration determination with a modified Lowry method
A modified Lowry method was used to determine protein concentration. The method is based on the Biuret reaction, in which protein peptide bonds react with copper under alkaline conditions to produce Cu+, which then reacts with the Folin reagent and gives the Folin–Ciocalteau reaction, in which phosphomolybdotungstate is reduced to heteropolybdenum blue by the oxidation of aromatic amino acids by copper. This technique is most effective on solutions with protein concentrations between 0.01 and 1.0 mg/mL (Waterborg, 2009). For this study, a commercial test kit (MFCD00132625, Sigma-Aldrich) was used for analysis, and 0.125–2,500 μg/mL bovine serum albumin (A3295-10g, Sigma-Aldrich) was selected as the standard reference protein. Absorption was measured at 660 nm (Halo DB-20 UV-VIS Double Beam spectrophotometer, Dynamica).
2.3.3 Formol titration
An adjusted formol titration method by Taylor (1957) was used to determine the amino acid concentration in the supernatant of the hydrolysis mass. It should be noted that, according to this method, protein is also counted as a long-chain amino acid. The amino group from the end amino acid or amino acid side chain interacts with formaldehyde at neutral or alkaline pH, releasing a proton and reducing the pKa of the amino acid–formaldehyde combination. The number of free amino groups present can be directly measured by titrating the protons with a base if the pH of the reaction mixture is lower than the pKa of the present amino acid or peptide complexes (Kala et al., 2019).
For analysis, a suitable amount of hydrolysis mass was taken and, depending on the chemical properties, diluted with water to 50 mL and further neutralized either with 1 M NaOH or 1 M HCl to pH 8. Then, 20 mL of neutralized formaldehyde (pH 8.5) were added and back-titrated with 0.05 M NaOH titrant to pH 8.5 using an electrode for pH measurements. After titration, the end carboxyl group concentration was expressed in mmol/L according to the spent 0.05 M NaOH.
2.3.4 Total nitrogen determination
Total nitrogen was determined in the SS, and pellets of both hydrolysis steps (Figure 1) and nitrogen transfer were calculated arithmetically from the initial sludge sample. Kjeldahl nitrogen was measured according to ISO 11261:1995 (ISO, 2022). Nitrate and nitrite are included in the measurement pool, but compounds with special chemical N-bonding (N-N, N-O, and heterocycles) cannot be digested entirely, and were thus omitted in these measurements.
3 Results and discussion
3.1 Organic matter hydrolysis and solubilization
Organic matter (OM) hydrolysis and solubilization refer to the process by which large biological molecules are broken down into smaller fragments, solubilized, and then transferred into a liquid phase from a solid state, making them chemically available for further reactions or utilization (Pham et al., 2021). It is known that hydrodynamic cavitation in water causes the formation of •OH radicals which are very strong and nonspecific oxidizing species (Mancuso et al., 2019). Therefore, some of the extracted OM can mineralize and become unavailable as organic material in the supernatant. At the same time, it is removed from the feed material, and extraction efficiency can be compared across the methods by analyzing the sludge and not the supernatant. To determine this transfer, the study’s OM was identified as the remaining volatile organic content in the pellets after each centrifugation. This OM was expressed as a percentage of the lost OM in the solid pellets compared to feed-material OM. This transfer of OM reflects the efficiency of hydrolysis and is expressed as a weight percentage (w/w %) representing the proportion of OM recovered from the feed sludge OM in each treatment method and at each step of sludge hydrolysis.
By comparing the overall results, the most effective organic matter transfer into a solution took place when SS was treated with a two-step treatment of physical-alkali–acid hydrolysis reaching 75.2% on average. The physical-acid–alkali pattern for all physical treatment methods resulted in 72.83% transfer (Table 1). The better dissolution of OM during alkaline hydrolysis can be explained by the amino acid composition of the sludge proteins. According to the literature data, sludge proteins contain a high content of dibasic amino acids—aspartic and glutamic (Gao et al., 2020). During hydrolysis, free acid groups of these amino acids can form salts that are readily soluble in water at low pH and provide better protein solubility at alkali pH due to soluble sodium salt formation. Superior SS solubility and degradation in alkali environment have been observed before, and it is known that an alkali environment more efficiently destroys sludge flocks and performs better cell destruction (Tavares de Sousa et al., 2021); further research is needed to better understand this process.
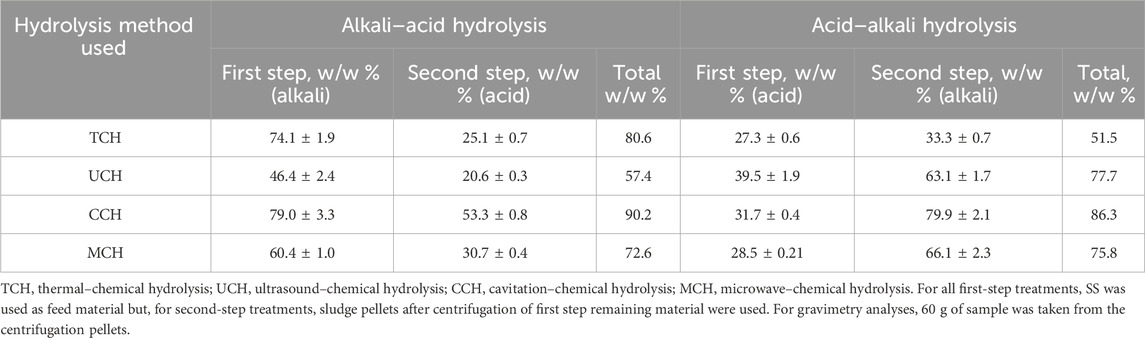
TABLE 1. Percentage of organic matter extracted into supernatant with various pre-treatment methods.
Reduced OM extraction performance for UCH-alkali treatment is probably related to its lower temperature and treatment time than other methods. At the same time, UCH-alkali treatment is only 6.9% more efficient than UCH-acid treatment. This shows that the chemical environment is not critical for ultrasound-enhanced chemical hydrolysis and that temperature is crucial for cell degradation. This may be explained by the fact that ultrasonication mainly induces cell wall lysis (Zhang et al., 2007) to such a degree that it compensates the low temperature disadvantage. Nevertheless, industrial UCH acid–alkali and UCH alkali–acid SS treatment according to the literature’s data will be too energy consuming to reach an effective extraction (Gao et al., 2021).
The most effective OM extraction can be achieved with CCH alkali–acid and CCH acid–alkali treatment that resulted in 90.2 w/w % and 86.3 w/w % extraction rates, respectively, from the loaded OM. CCH-alkali treatment also showed the highest OM extraction rate after the first step −79.0 w/w % from loaded OM. This was the highest extraction rate for all first-step extraction methods. The secondary treatment of pellets with acid in CCH alkali–acid treatment yielded 53.3 w/w % from the loaded OM, reaching 90.2% of total OM extraction. The observed trend can be explained by the successful chemical hydrolysis and a strong grinding and mass exchange during hydrodynamic cavitation that results in good material dispersion in the solution. Furthermore, due to the high friction during the cavitation, temperature increase was observed in the samples, which also had a positive influence on cell breakdown. A similar effect was observed also for CCH acid–alkali treatment, although the total OM extraction efficiency is more related to the alkali treatment step.
Despite the OM transfer rate with MCH alkali–acid treatment being slightly smaller than TCH alkali–acid or CCH alkali–acid treatment, the process will still be less time and energy intensive since MCH required a shorter exposure time—15 min in a microwave with 30 min of post treatment for both hydrolysis steps, instead of 3 h with mixing at 80 C for thermal treatment.
3.2 Shift in nitrogen compounds during hydrolysis
To gain more information about the biomolecule transfer in-between the system phases, Kjeldahl nitrogen was measured in the pellets remaining after post-hydrolysis centrifugation. Nitrogen extraction efficiency was calculated as for OM extraction, and the result was expressed as the weight percentage between the initial nitrogen content in the sludge and the nitrogen left in the pellets after the first and second centrifugation (Table 2).
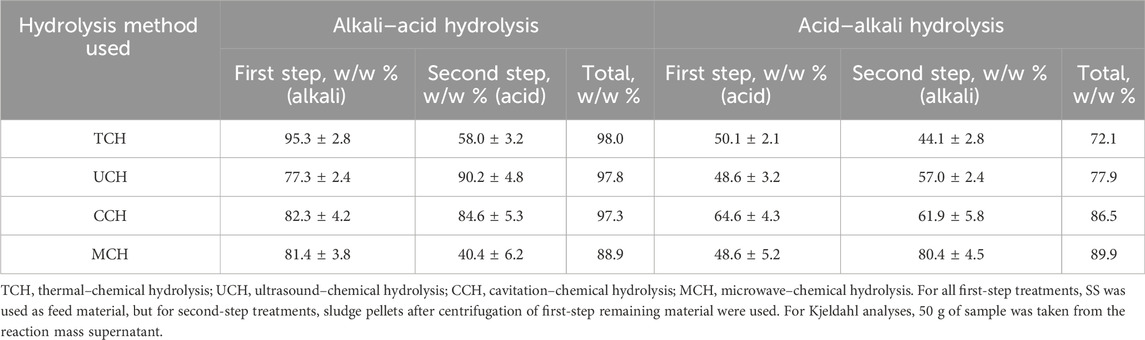
TABLE 2. Kjeldahl nitrogen recovery from the secondary sludge (SS) by different treatment methods in the supernatant after two hydrolysis steps.
During the first physical-alkali treatment, regardless of the physical treatment method used, most of the nitrogen compounds were transferred into the supernatant. The limited effect of acid in physical-acid treatment was due to the absence of cell lysis, with only sludge flock breakage being identified (Tavares de Sousa et al., 2021). The best performance was shown by the TCH-alkali treatment where, already in the first step, 95.5 w/w % of nitrogen from loaded OM was transferred into the liquid phase. Despite the process giving a high nitrogen extraction rate, it is energy and time-consuming, since the reaction mass must be heated for 3 h at 80 C. It has been stated that the most abundant nitrogen form detected by X-ray photoelectron spectroscopy in SS is protein-nitrogen and pyridine-nitrogen. SS thermal treatment favors pyridine-nitrogen release, but alkali treatment with NaOH favors protein-nitrogen extraction (Xiao et al., 2017). The combination of these two treatment conditions in this study show that both nitrogen species are hydrolyzed, thus producing the high TKN extraction rate.
CCH-alkali and MCH-alkali first step hydrolysis demonstrated 82.3 w/w % and 81.4 w/w % nitrogen transfer, respectively, within a shorter time and potentially smaller energy consumption. Therefore, these methods could be selected as more suitable for industrial use and further development. Understanding that efficient nitrogen extraction from SS is influenced by both alkali and temperature, it can be concluded that neither microwave irradiation nor cavitation-released •OH radicals increase nitrogen extraction efficiency. The temperature factor is present in both processes. To better understand organic nitrogen hydrolysis mechanics, further research would be necessary to evaluate the reaction time and changes of the nitrogen source in the sludge.
MCH- and UCH-alkali treatment show slightly better selective nitrogen compound extraction efficiency because a smaller amount of OM was transferred into the solution but a relatively higher nitrogen fraction was extracted from the loaded material—most probably leaving the non-nitrogen organics, like polysaccharides and lignin, in the pellets. Ultrasonication affects cell walls with •OH radicals, which form during acoustic cavitation; thus, the cells are destroyed as a result of intracellular substance release (Liu et al., 2021). It has been observed that microwaves can destroy microorganisms at temperatures lower than the thermal destruction point by interacting with the cell membrane and releasing proteins (Shaw et al., 2021). UCH and MCH sludge treatment required shorter treatment time than TCH hydrolysis; therefore, less OM was extracted from the SS. At the same time, these methods more selectively targeted bacterial cell walls.
Overall nitrogen transfer with physical-alkali one-step hydrolysis is, on average, 31.1% higher than with physical-acid one step hydrolysis (Table 2). The limited effect of the acid treatment can be attributed to the lack of cell breakdown and to higher interaction with the SS flocks (Sahinkaya, 2015); however, in an alkali environment, cell lysis is more likely (Bi et al., 2014a). However, as the second treatment step, physical-acid treatment was more advantageous than the same type physical-alkali treatment second step. Only MCH alkali–acid treatment showed 40% better nitrogen extraction than MCH acid–alkali treatment. This demonstrates that microwave irradiation affects the cell walls of organisms in the SS via both temperature and microwaves. To make further estimations about nitrogen compound extraction mechanisms, the changes in different organic nitrogen sources must be examined; however, since the OM extraction during the first step physical-alkali treatment step is greater than physical-acid treatment, it could be related to the fact that organic compounds are more physically and chemically available for degradation.
Physical-alkali–acid two-step SS hydrolysis methods are slightly more effective for nitrogen extraction than physical-acid–alkali hydrolysis since the first physical-alkali treatment more efficiently extracted nitrogen from the SS. The best performing technique for nitrogen extraction was the TCH alkali–acid technique, which reached 98.0 w/w % of total nitrogen loaded while simultaneously being the most time and energy consuming method. At the same time, CCH alkali–acid and UCH alkali–acid treatment showed approximately the same nitrogen extraction efficiency of 97.3 and 97.8 w/w % of nitrogen loaded, respectively, with less energy consumption and shorter treatment time. However, these methods have been recognized as more technologically demanding.
3.3 Protein concentration in hydrolysis mass
Protein extraction from SS occurred during both treatment steps using all selected physical treatment methods; however, since physical-alkali treatment methods showed a noticeable advantage in SS protein solubilization, only physical-alkali treatment methods are discussed further here and compared to the data in the literature. It is hard to compare protein extraction or recovery efficiency between experiments and studies where different SS has been used since the initial protein content in the SS remains unknown.
Protein concentration analyses showed that the highest protein concentration in supernatant is obtained with MCH-alkali hydrolysis: 23,046 mg/L of protein. In other studies where SS with dry matter content of 13% was treated, microwave irradiation for 10 min at 25 C produced only 245.14 mg/L protein in the supernatant (X. Zhang et al., 2021). This shows that the temperature, reaction time, and high pH favor a protein extraction process which, in combination with several treatment factors, can give noticeably higher protein extraction efficiency through cell wall damage than just microwave treatment.
MCH alkali extraction is followed by TCH-alkali treatment producing 15,375 mg/L of protein in the supernatant. Other studies using one-step thermal treatment in an alkali environment at 120 C and pH 12 for 4 h produced 21,024 mg/L protein in the reaction mass but, when temperature is decreased to 100 C, the amount decreased to approximately 11,200 mg/L (Gao et al., 2020). This shows that increase in the pH could compensate for the temperature decrease. In this study, high amounts of NaOH were used at a lower temperature.
During the CCH-alkali SS treatment, it was possible to reach 15,178 mg/L of protein in the supernatant. In other studies with 60 min cavitation treatment at pH 10 and 50 C, only 2,040 mg/L protein in supernatant were produced from sludge with dry solid content of 43.5% (Mancuso et al., 2019). This again shows that a higher temperature and pH in the protein extraction process from SS permits higher protein extraction efficiency due to the ability of NaOH to dissolve membrane proteins and cause the saponification of membrane lipids (Hui et al., 2022a). Thus, the destruction of the microbial cells occurs as a combination of chemical treatment, •OH radical interaction, and temperature increase.
Of all four selected treatment methods, UCH-alkali showed the smallest protein extraction efficiency at only 13,917 mg/L of the extracted protein in the supernatant. This again highlights the importance of the temperature effect. The quantity obtained is still approximately 40% higher than other reported results where ultrasonic-alkaline treatment at 28 kHz, pH 12 for 60 min produced 7,900 mg/L of soluble protein from SS with dry solid content of 7.4% (X. Liu et al., 2008). Nevertheless, both results could be evaluated as being similar since, in the current study, SS with approximately two times higher dry solid content was used and sludge was treated for an additional 1 h. Optimizing the protein extraction efficiency adjustment of the ultrasound frequencies would be advisable (Le et al., 2015).
Carboxylic acid concentration determined by formol titration (Table 3) provides more knowledge about the peptide size and protein hydrolysis level in each hydrolysis step, since the proteins can be cut into smaller oligopeptides. A modified Lowry method also determines the protein concentration by producing a complex with peptide bonds; therefore, the higher the carboxyl group concentration at the same protein level, the smaller the peptides. In the first step of physical-acid SS hydrolysis, five times less protein was extracted on average. The carboxyl group concentration at first-step treatment remained either at the same level for TCH-acid and CCH-acid or was notably higher for UCH-acid and MCH-acid hydrolysis than physical-alkali hydrolysis. Thus, it can be concluded that physical–alkali hydrolysis focuses more on cell destruction and OM extraction than on protein hydrolysis, but that physical-acidic hydrolysis in the first step results in protein cleavage rather than extraction. UCH-acid and MCH-acid hydrolysis demonstrate the highest carboxylic group concentration than other first-step acid hydrolysis methods. This could be explained by ultrasonication and microwave treatment affecting both sludge disintegration, biomolecule extraction, and protein hydrolysis as such. The application of these physical treatment methods has been validated in the food industry to treat high molecular weight protein fractions (Chen et al., 2019). Higher protein hydrolysis in an acid environment is also confirmed if we look at the second hydrolysis step, where protein concentration after the physical-acid-alkali hydrolysis is, on average, 9.5 times higher than physical-alkali–acid hydrolysis and the carboxylic group concentration is only 2.4 times higher on average. This means that proteins after physical-alkali–acid hydrolysis have a smaller molecular mass.
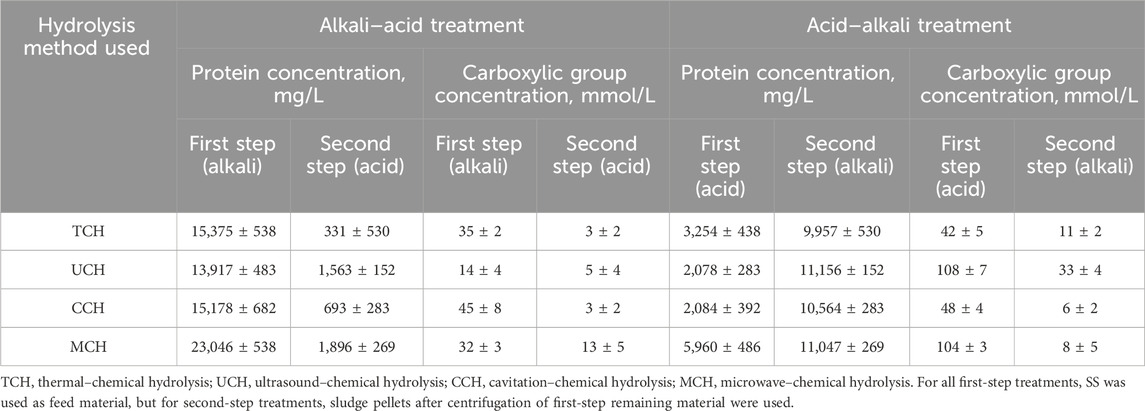
TABLE 3. Protein concentration detected by modified Lowry method and Formol titration in supernatant after first and second hydrolysis steps, depending on the hydrolysis method used.
3.4 Future perspectives
As estimated in this study, organic material extraction and protein recovery from SS were more efficient through physical-alkali treatment (Tables 1, 3). Only nitrogen compounds were better extracted from SS pellets during physical-alkali-acid secondary treatment (Table 2). Nevertheless, the purity of the obtained nitrogen compounds could be variable, and additional testing would be needed to evaluate the changes in the nitrogen-compound types. On average, physical-alkali SS treatment showed 80%–89% better protein extraction efficiency than physical-acid hydrolysis, both as first and second step hydrolysis, respectively (Table 3). This can be linked to OM extraction, where, under alkali conditions, the first step treatment yielded 33% and the second step 28% more OM than acid hydrolysis (Table 1).
Since both TCH- and MCH-alkali treatment have the temperature effect present, it is most likely that microwave interaction with cell membranes or other enclosed proteins in the SS flock enhances protein extraction. Simultaneously, less interaction with protein peptide bonds occurs at these treatment conditions, since the carboxylic group concentration is smaller even at the higher protein concentration in the MCH-alkali sludge treatment step. Thus, different physical treatment methods demonstrate selective resource recovery or extraction performance.
The proposed technologies can be introduced into WWTP technological process to obtain protein-rich solutions applicable to the production of high-value liquid fertilizer (Liu et al., 2009) and protein-based surface-active compound (Collivignarelli et al., 2017). After concentration, the solutions can be used as supplements to animal feed (Xiao and Zhou, 2020). In addition to protein extraction, the overall OM in SS is reduced, thus producing less sludge. Consequently, the costs of wastewater treatment can be reduced. Some of the physical SS treatment methods used in this study are already being used to improve SS dewatering, such as SS treatment with ultrasound (Feng et al., 2009) with rotor/stator type hydrodynamic cavitation equipment (Kim et al., 2019). Further research is needed to determine how much sludge reduction affects each of the methods used; however, there is reason to believe that the treated material after solid–liquid separation would have a higher dry matter content than a raw material. The processed sludge hydrolysis mass contains a large amount of organic matter; therefore, after protein separation, it is possible to use this solution as a carbon source in the WWTP nitrification process to compensate for the lack of carbon in wastewater denitrification.
4 Conclusion
The protein recovery rate and the OM and Kjeldahl nitrogen extraction rates decrease in second-step treatment irrespective of the physical treatment method used. SS one-step physical–alkali treatment was more efficient than one- or two-step SS physical-acid treatment. The outcomes are related to multi-factor effects of temperature, chemicals, and •OH radical formation during treatment. MCH-alkali hydrolysis produced 23,046 mg/L of protein from SS, while TCH-alkali treatment produced 95.5 w/w % of nitrogen from loaded OM into the liquid phase. These findings show that each physical SS treatment method targets different biopolymers and can selectively extract molecules of interest, such as OM, protein, or organic nitrogen compounds. This study confirms that proteins can be extracted from SS; however, due to the potential variation in the quantities, further research is needed to understand the possibilities for their use in other industries.
Data availability statement
The raw data supporting the conclusion of this article will be made available by the authors without undue reservation.
Author contributions
ES: conceptualization, supervision, and writing–review and editing. EK: conceptualization, data curation, investigation, methodology, and writing–original draft. LM: funding acquisition and writing–review and editing.
Funding
The author(s) declare that financial support was received for the research, authorship, and/or publication of this article. This research was funded by the ERDF Project “Waste to resource technology development using sewage sludge as raw material,” No. 1.1.1.1/20/A/041.
Acknowledgments
The authors acknowledge the wastewater treatment facility for providing the test material.
Conflict of interest
Authors ES and EK were employed by Bio RE Ltd.
The remaining authors declare that the research was conducted in the absence of any commercial or financial relationships that could be construed as a potential conflict of interest.
Publisher’s note
All claims expressed in this article are solely those of the authors and do not necessarily represent those of their affiliated organizations or those of the publisher, editors, and reviewers. Any product that may be evaluated in this article or claim that may be made by its manufacturer is not guaranteed or endorsed by the publisher.
References
Amaral, A. L., Da Motta, M., Pons, M. N., Vivier, H., Roche, N., Mota, M., et al. (2004). Survey of Protozoa and metazoa populations in wastewater treatment plants by image analysis and discriminant analysis. Environmetrics 15 (4), 381–390. doi:10.1002/env.652
APHA (2012). “Standard methods for the examination of water and wastewater,” in American public health association (APHA), American water works association (AWWA) and water environment federation (WEF). Editors Rice, E. W., Baird, R. B., Eaton, A. D., and Clesceri, L. S. 22nd Edition (Washington, D.C., USA: WEF). http://www.sciepub.com/reference/226577 (Accessed September 30, 2022).
Becerra, G., Flor, Y., Acosta, E. J., and Grant Allen, D. (2010). Alkaline extraction of wastewater activated sludge biosolids. Bioresour. Technol. 101 (18), 6972–6980. doi:10.1016/j.biortech.2010.04.021
Bi, W., Yiyong, L., and Yongyou, H. (2014a). Recovery of phosphorus and nitrogen from alkaline hydrolysis supernatant of excess sludge by magnesium ammonium phosphate. Bioresour. Technol. 166, 1–8. doi:10.1016/j.biortech.2014.04.092
Capodaglio, A. G. (2023). Biorefinery of sewage sludge: overview of possible value-added products and applicable process technologies.” water (Switzerland). Switzerland: MDPI. doi:10.3390/w15061195
Chen, S., Dong, B., Dai, X., Wang, H., Li, N., and Yang, D. (2019). Effects of thermal hydrolysis on the metabolism of amino acids in sewage sludge in anaerobic digestion. Waste Manag. 88 (April), 309–318. doi:10.1016/J.WASMAN.2019.03.060
Collivignarelli, M. C., Castagnola, F., Sordi, M., and Bertanza, G. (2017). Sewage sludge treatment in a thermophilic membrane reactor (TMR): factors affecting foam formation. J. Environ. Sci. Pollut. Res. 24 (3), 2316–2325. doi:10.1007/s11356-016-7983-4
Devos, P., Haddad, M., and Carrère, H. (2020). Thermal hydrolysis of municipal sludge: finding the temperature sweet spot: a review. Waste Biomass Valorization 2020 (5), 2187–2205. doi:10.1007/S12649-020-01130-1
Eurostat (2016). Sewage sludge production and disposal from urban wastewater (in dry substance (d.s)) - products datasets - eurostat. Available at: https://ec.europa.eu/eurostat/web/products-datasets/-/ten00030.
Farrell, H. M., Jimenez-Flores, R., Bleck, G. T., Brown, E. M., Butler, J. E., Creamer, L. K., et al. (2004). Nomenclature of the proteins of cows’ milk—sixth revision. J. Dairy Sci. 87 (6), 1641–1674. doi:10.3168/JDS.S0022-0302(04)73319-6
Feng, X., Deng, J., Lei, H., Bai, T., Fan, Q., and Li, Z. (2009). Dewaterability of waste activated sludge with ultrasound conditioning. Bioresour. Technol. 100 (3), 1074–1081. doi:10.1016/j.biortech.2008.07.055
Gao, J., Wang, Q., Yan, X., Li, Z., and Weng, W. (2021). Protein extraction from excess sludge by thermal-acid pretreatment. Desalination Water Treat. 209 (January), 48–57. doi:10.5004/dwt.2021.26486
Gao, J., Wang, Y., Yan, Y., Li, Z., and Chen, M. (2020). Protein extraction from excess sludge by alkali-thermal hydrolysis. Environ. Sci. Pollut. Res. 27 (8), 8628–8637. doi:10.1007/s11356-019-07188-2
Gao, J., Weng, W., Yan, Y., Wang, Y., and Wang, Q. (2020). Comparison of protein extraction methods from excess activated sludge. Chemosphere 249 (June), 126107. doi:10.1016/J.CHEMOSPHERE.2020.126107
Gianfredi, V., Buffoli, M., Rebecchi, A., Croci, R., Oradini-Alacreu, A., Stirparo, G., et al. (2021). Association between urban greenspace and health: a systematic review of literature. Int. J. Environ. Res. Public Health 18, 5137. doi:10.3390/IJERPH18105137
Hauduc, H., Rieger, L., Oehmen, A., van Loosdrecht, M. C. M., Comeau, Y., Héduit, A., et al. (2013). Critical review of activated sludge modeling: state of process knowledge, modeling concepts, and limitations. Biotechnol. Bioeng. 110 (1), 24–46. doi:10.1002/BIT.24624
Hui, W., Zhou, J., and Jin, R. 2022a. “Kinetics of protein extraction from excess sludge by thermal alkaline treatment. doi:10.21203/rs.3.rs-1974348/v1
ISO (2022). 11261:1995 - soil quality — determination of total nitrogen — modified Kjeldahl method. Available at: https://www.iso.org/standard/19239.html (Accessed July 15, 2022).
Kala, R., Samková, E., Hanuš, O., Pecová, L., Sekmokas, K., and Riaukienė, D. 2019. Milk protein analysis: an overview of the methods – development and application. Acta universitatis Agriculturae et silviculturae mendelianae brunensis. Mendel university of agriculture and forestry brno. doi:10.11118/actaun201967010345
Kim, H., Sun, X., Koo, B., and Yoon, J. Y. (2019). Experimental investigation of sludge treatment using a rotor-stator type hydrodynamic cavitation reactor and an ultrasonic bath. Processes 7 (11), 790. doi:10.3390/pr7110790
Lau, D. C. W. (1981). Utilization of sewage sludge as a resource for protein extraction and recovery. Conservation Recycl. 4 (3), 193–200. doi:10.1016/0361-3658(81)90024-2
Le, N. T., Julcour-Lebigue, C., and Delmas, H. (2015). An executive review of sludge pretreatment by sonication. J. Environ. Sci. 37, 139–153. doi:10.1016/j.jes.2015.05.031
Li, D., Zhou, Y., Tan, Y., Pathak, S., Bin Abdul Majid, M., and Jern Ng, W. (2016). Alkali-solubilized organic matter from sludge and its degradability in the anaerobic process. Bioresour. Technol. 200 (January), 579–586. doi:10.1016/j.biortech.2015.10.083
Liu, H., Wang, X., Qin, S., Lai, W., Yang, X., Xu, S., et al. (2021). Comprehensive role of thermal combined ultrasonic pre-treatment in sewage sludge disposal. Sci. Total Environ. 789, 147862. doi:10.1016/j.scitotenv.2021.147862
Liu, X., Liu, H., Chen, J., Du, G., and Chen, J. (2008). Enhancement of solubilization and acidification of waste activated sludge by pretreatment. Waste Manag. 28 (12), 2614–2622. doi:10.1016/j.wasman.2008.02.001
Liu, Y., Kong, S., Li, Y., and Zeng, H. (2009). Novel technology for sewage sludge utilization: preparation of amino acids chelated trace elements (AACTE) fertilizer. J. Hazard Mater 171, 1159–1167. doi:10.1016/j.jhazmat.2009.06.123
Lowry, O. H., Rosebrough, N. J., Lewis Farr, A., and Randall, R. J. (1951). Protein measurement with the Folin phenol reagent. J. Biol. Chem. 193 (1), 265–275. doi:10.1016/s0021-9258(19)52451-6
Mancuso, G., Langone, M., Andreottola, G., and Bruni, L. (2019). Effects of hydrodynamic cavitation, low-level thermal and low-level alkaline pre-treatments on sludge solubilisation. Ultrason. Sonochemistry 59 (December), 104750. doi:10.1016/j.ultsonch.2019.104750
Mariotti, F., Tomé, D., and Patureau Mirand, P. 2008. Converting nitrogen into protein—beyond 6.25 and jones’ factors. doi:10.1080/1040839070127974948
Pham, V. T., Yu Guan, C., Han, P. C., Matsagar, B. M., Wu, K. C. W., Ahamad, T., et al. (2021). Acid-catalyzed hydrothermal treatment of sewage sludge: effects of reaction temperature and acid concentration on the production of hydrolysis by-products. Biomass Convers. Biorefinery 2021, 7533–7546. doi:10.1007/S13399-021-01495-W
Raheem, A., Sikarwar, V. S., He, J., Dastyar, W., Dionysiou, D. D., Wang, W., et al. (2018). Opportunities and challenges in sustainable treatment and resource reuse of sewage sludge: a review. Chem. Eng. J. 337 (April), 616–641. doi:10.1016/J.CEJ.2017.12.149
Redmile-Gordon, M. A., Armenise, E., White, R. P., Hirsch, P. R., and Goulding, K. W. T. (2013). A comparison of two colorimetric assays, based upon Lowry and bradford techniques, to estimate total protein in soil extracts. Soil Biol. Biochem. 67 (December), 166–173. doi:10.1016/J.SOILBIO.2013.08.017
Sahinkaya, S. (2015). Disintegration of municipal waste activated sludge by simultaneous combination of acid and ultrasonic pretreatment. Process Saf. Environ. Prot. 93, 201–205. doi:10.1016/j.psep.2014.04.002
Shaw, P., Kumar, N., Mumtaz, S., Lim, J. S., Jang, J. H., Kim, D., et al. (2021). Evaluation of non-thermal effect of microwave radiation and its mode of action in bacterial cell inactivation. Sci. Rep. 11 (1), 14003. doi:10.1038/s41598-021-93274-w
Simonian, M. H., and Smith, J. A. (2006). “Spectrophotometric and colorimetric determination of protein concentration,” in Current protocols in molecular biology. 2006. 1. doi:10.1002/0471142727.mb1001as76
Tavares de Sousa, T. A., do Monte, F. P., do Nascimento Silva, J. V., Silva Lopes, W., Leite, V. D., van Lier, J. B., et al. (2021). Alkaline and acid solubilisation of waste activated sludge. Water Sci. Technol. 83 (12), 2980–2996. doi:10.2166/wst.2021.179
Taylor, W. H. (1957). Formol titration: an evaluation of its various modifications. Analyst 82 (976), 488–498. doi:10.1039/AN9578200488
Wang, L. K., Chang, S. Y., Hung, Y. T., Muralidhara, H. S., and Chauhan, S. P. (2007). ““Centrifugation clarification and thickening” in,” in Biosolids treatment processes. Handbook of environmental engineering. Editors L. K. Wang, N. K. Shammas, and Y. T. Hung (USA: Humana Press), Vol. 6. doi:10.1007/978-1-59259-996-7_4
Waterborg, J. H. (2009). Lowry Method Protein Quantitation 7, 10. doi:10.1007/978-1-59745-198-7_2/COVER/
Xiao, K., Chen, Y., Jiang, X., Seow, W. Y., He, C., Yin, Y., et al. (2017). Comparison of different treatment methods for protein solubilisation from waste activated sludge. Water Res. 122 (October), 492–502. doi:10.1016/J.WATRES.2017.06.024
Xiao, K., and Zhou, Y. (2020). Protein recovery from sludge: a review. J. Clean. Prod. 249, 119373. doi:10.1016/j.jclepro.2019.119373
Zhang, P., Zhang, G., and Wang, W. (2007). Ultrasonic treatment of biological sludge: floc disintegration, cell lysis and inactivation. Bioresour. Technol. 98 (1), 207–210. doi:10.1016/J.BIORTECH.2005.12.002
Keywords: sludge hydrolysis, protein extraction, sludge physical–chemical treatment, sewage sludge, sewage sludge recycling
Citation: Skripsts E, Klaucans E and Mezule L (2024) Organic mass and protein extraction from secondary sewage sludge via multi-step physical alkali- and acid-based treatment. Front. Chem. Eng. 6:1346736. doi: 10.3389/fceng.2024.1346736
Received: 29 November 2023; Accepted: 20 February 2024;
Published: 08 March 2024.
Edited by:
Prithvi Simha, Swedish University of Agricultural Sciences, SwedenReviewed by:
Luis Fernando Perez-Mercado, University of San Simón, BoliviaThierry Ribeiro, UniLaSalle, France
Copyright © 2024 Skripsts, Klaucans and Mezule. This is an open-access article distributed under the terms of the Creative Commons Attribution License (CC BY). The use, distribution or reproduction in other forums is permitted, provided the original author(s) and the copyright owner(s) are credited and that the original publication in this journal is cited, in accordance with accepted academic practice. No use, distribution or reproduction is permitted which does not comply with these terms.
*Correspondence: Elvis Klaucans, elvis.klaucans@biore.lv; Linda Mezule, linda.mezule@rtu.lv