Transformation of sodium bicarbonate and CO2 into sodium formate over NiPd nanoparticle catalyst
- Department of Chemical and Biomolecular Engineering, National University of Singapore, Singapore
The present research systematically investigated, for the first time, the transformation of sodium bicarbonate and CO2 into sodium formate over a series of Ni based metal nanoparticles (NPs). Ni NPs and eight NiM (M stands for a second metal) NPs were prepared by a facile wet chemical process and then their catalytic performance were evaluated in sodium bicarbonate hydrogenation. Bimetallic NiPd NPs with a composition of 7:3 were found to be superior for this reaction, which are more active than both pure Ni and Pd NPs. Hot filtration experiment suggested the NPs to be the truly catalytic active species and kinetic analysis indicated the reaction mechanism to be different than most homogeneous catalysts. The enhanced activity of the bimetallic nanoparticles may be attributed to their smaller size and improved stability.
Introduction
Utilizing renewable resources is a prerequisite for a sustainable society. One easily available renewable carbon resource is carbon dioxide (CO2), which has the advantages of being non-toxic, abundant, and economical (Sakakura et al., 2007). Unfortunately, few running industrial process employs CO2 as a building block as CO2 is the most oxidized state of carbon and therefore difficult to be activated. However, increasing efforts have been devoted to the transformation of CO2 into value added substances including methane (Riedel et al., 2003; Sai Prasad et al., 2008; Sharma et al., 2011), methanol (Imai et al., 1995; Kilo et al., 1997; Barrault and Urresta, 1999; Natesakhawat et al., 2012; Wesselbaum et al., 2012), formaldehyde (Lee et al., 2001), formic acid (Inoue et al., 1976; Yin et al., 2001; Elek et al., 2003; Himeda et al., 2003, 2006; Laurenczy et al., 2007; Tanaka et al., 2009; Federsel et al., 2010a,b; Sanz et al., 2010; Boddien et al., 2011a,b; Hao et al., 2011; Himeda et al., 2011; Peng et al., 2011; Preti et al., 2011; Wan-Hui and Yuichiro, 2012; Ziebart et al., 2012) and organic carbonates (Tomishige et al., 2001; Li and Zhong, 2002; La et al., 2007; Dai et al., 2009; Kohno and Sakakura, 2009; Lee et al., 2011; Ghazali-Esfahani et al., 2013). Among them, formic acid, conveniently obtained by hydrogenation of CO2, is highly attractive as formic acid is an important chemical used as preservative and antibacterial agent in food as well as the tanning of leather. Moreover, formic acid has been regarded as a clean source for H2 generation (Fellay et al., 2009; Boddien et al., 2011a; Himeda et al., 2011).
In aqueous solution, the hydrogenation of CO2 into formic acid is thermodynamically unfavorable. Hence many base additives such as KOH, NEt3 are used to promote this reaction. NaHCO3 is another starting material that could be used to overcome the thermodynamic limitation. Currently, the research activity on the reduction of CO2 into formic acid is predominantly focused on homogeneous catalysts. A variety of organometallic catalysts based on Ru (Yin et al., 2001; Elek et al., 2003; Himeda et al., 2006; Laurenczy et al., 2007; Federsel et al., 2010b; Hao et al., 2011; Himeda et al., 2011; Wesselbaum et al., 2012), Ir (Tanaka et al., 2009; Schmeier et al., 2011), Rh (Himeda et al., 2003, 2011), or Fe (Sai Prasad et al., 2008; Federsel et al., 2010a; Boddien et al., 2011b; Ziebart et al., 2012) have been developed for the hydrogenation of CO2. The classical Wilkinson catalyst (RhCl(PPh3)3) and the Ru analogue (RuCl(PPh3)3) were firstly introduced by Inoue et al. in 1976 (Inoue et al., 1976). These two complexes exhibited turnover number (TON) of 22 and 87, respectively, under room temperature and 50 bar H2/CO2 (1/1) in the presence of triethylamine for 20 h, which were more active than other catalysts being tested. Following this work, various Rh and Ru catalyst bearing phosphine and nitrogen ligands have been investigated. For example, Joó et al. reported the [RuCl2(tppms)2]2 (tppms = sodium diphenylphosphinobenzene-3-sulfonate) which exhibited a turnover frequency (TOF) of 54 h−1 at 50°C and 10 bar H2. The meritorious catalytic activity of Ir complexes has only been reported recently, although the initial study on Ir catalysts could date back to 1976 (Inoue et al., 1976). Tanaka and his colleagues reported a remarkable TOF of 150000 h−1 with PNP-Ir trihydride complex as the catalyst (Tanaka et al., 2009). Catalysts based on non-precious metals have also been explored. To date, the most active iron catalyst for the hydrogenation of carbon dioxide and bicarbonate was reported recently by Carolin et al. (Ziebart et al., 2012). Employing iron(II)-fluoro-tris[(2-(diphenylphosphino)phenyl)phosphino]tetrafluoroborate as the catalyst, the hydrogenation of bicarbonates results in good yields with high catalyst productivity and activity (TON >7500, TOF >750). Interestingly, Beller et al. reported a catalyst, [RuCl2(C6H6)]2/dppm (dppm = 1,2-bis(diphenylphosphino)methane), which is formed in-situ and can catalyze both the hydrogenation of bicarbonate and the dehydrogenation of formate in H2O/THF (Boddien et al., 2011a). As such, a hydrogen storage system based on the inter-conversion of formate and bicarbonate that is controlled by one catalyst becomes possible. There are several excellent reviews providing more comprehensive and detailed information for CO2 hydrogenation over homogeneous catalysts (Jessop et al., 1995; Scott et al., 2005; Himeda, 2007; Wan-Hui and Yuichiro, 2012).
Although homogeneous catalysts proved to be very successful in terms of achieving high catalytic activity, they are intrinsically associated with a few limitations. For instance, the ligand and the catalyst are sometimes not readily available and/or the costs are high. Removing the catalyst from product is also very difficult, which makes the recycle and reuse challenging. These limitations can be easily overcome by using heterogeneous catalysts. The very first approach to CO2 hydrogenation into formic acid was carried out by Farlow and Adkins in 1935 using Raney nickel as a catalyst under 20–40 MPa and at 353–423 K (Farlow and Adkins, 1935). Afterwards, very few heterogeneous systems have been reported. Preti et al. investigated CO2 hydrogenation in the presence of neat NEt3 to form HCOOH/NEt3 adducts over gold black (Preti et al., 2011). Tsang and co-workers explored the possibility of using copper zinc oxide catalyst for the methyl formate production from CO2 (Kerry Yu and Tsang, 2011). There were several investigations on the heterogenization of homogeneous catalysis by immobilizing ruthenium complex catalysts on silica and polystyrene resin (Baiker, 2000; Zhang et al., 2004). Besides, Peng et al. have conducted density functional theory (DFT) calculations on CO2 hydrogenation to formic acid on Ni(111) (Peng et al., 2011). Nevertheless, hydrogenation of carbon dioxide (or bicarbonate) into formic acid over heterogeneous catalyst has not been systematically attempted.
Nanoparticle (NP) catalysts represent an area where traditional homogeneous catalysis and heterogeneous catalysis converge (Yan et al., 2010a). It has been known for many years that the size of metal particles immobilized on solid supports in heterogeneous catalysts are often of nanoscale dimensions, nevertheless, recent advances in nanoscience enable the precise characterization of NPs and open the way for a further enhancement of their catalytic properties (Yuan et al., 2012). One of the main branches of nanocatalysis is catalysis by “soluble” NPs in liquid phase. NP catalysis in traditional solvents originated from colloidal chemistry but is now well-advanced as an independent discipline of research (Roucoux et al., 2002). Generally these dispersed NP catalyst exhibited increased activity under mild reaction conditions. A majority of previous studies were focused on nobel metal NPs and more attention should be paid to first-row transitional metals such as nickel, as these metals are more abundant and much more affordable. Herein, we report a series PVP stabilized Ni and NiM (M stands for the second metal) NPs in aqueous solution, which can effectively transform carbon dioxide and sodium bicarbonate to formic acid under mild condition.
Materials and Methods
Materials
Sodium bicarbonate (99.99%) was purchased from AnalaR NORMAPUR; sodium formate (98.0%) was obtained from Fluka; sodium borohydride (95.0%) was from TCI; polyvinylpyrrolidone (PVP, M.W. = 40000) was from Alfa Aesar rhodium (III) chloride hydrate (Rh 38.5–45.5%) was from Alfa Aesar; Fe(II) chloride tetrahydrate (98%) was from Singma Aldrich; palladium(II) chloride (Pd 59.50%) was from Shaanxi Kaida Chemical Engineeering Co., Ltd.; auric chloride acid (Au 50%), chloroplatinic acid (Pt 47%), nickel(II) chloride hydrate (98.0%), copper(II) chloride (99%) and ruthenium(III) chloride hydrate (Ru 37%) were from Sinopharm Chemical Reagent (SCR). CO2 (99.998%) and H2 (99.995%) were purchased from was from Singapore Oxygen Air Liquide Pte Ltd. (SOXAL). All chemicals were used as received.
Catalyst Preparation
For preparation of Ni, Ni8Ru2, Ni8Rh2, Ni8Au2, Ni8Pt2, Ni8Fe2, Ni8Ag2, and Ni8Cu2 NPs, the desired amount of metal precursor (1.2 mmol, for bimetallic NPs, the Ni:M ratio was 4:1 with a total metal content of 1.2 mmol) and PVP (2.664 g, 20 molar equivalent) are dissolved in H2O (30 ml). NaBH4 (0.2269 g, 6 mmol) was dissolved in H2O (10 ml) and was added into the metal salt-PVP solution in one portion. The color of the solution turned to dark immediately indicating the reduction of the metal precursors. The final solution was transferred into a reactor (100 ml) when effervescence ceased.
For NPs containing Pd, PdCl2 (1.073 g, 6 mmol) was first dissolved in HCl solution (15 ml, 2 M) to obtain H2PdCl4. After that, the solution was dried using a rotary evaporator (IKA, RA10) to remove water and excess HCl. The remaining H2PdCl4 is dissolved in H2O (25 ml) to form a 0.24 M solution. For Ni7Pd3, Ni (II) chloride hexahydrate (0.200 g, 0.84 mmol) were dissolved together with H2PdCl4 solution (1.5 ml, 0.24 M) and PVP (2.664 g, 24 mmol) in H2O (30 ml). NaBH4 (0.2269 g, 6 mmol) were dissolved in H2O (10 ml) and added into the metal salt-PVP solution immediately. The color of the solution turned to dark immediately indicating the reduction of the metal precursors. The final solution was transferred into a reactor (100 ml) when effervescence ceased. Pd, Ni8Pd2, Ni6Pd4, Ni4Pd6, and Ni2Pd8 NPs were prepared in a similar way except the ratio of Ni and Pd was adjusted to the desired value.
Catalyst Characterization
Transmission electron microscopy (TEM) images were taken on a JEOL JEM-2010 microscope operating at 200 kV. TEM samples were prepared by diluting fresh prepared catalyst solution (0.05 ml) with methanol (1.5 ml), following which one drop of the solution was placed on a carbon film covered copper grid and dried under air.
X-ray photoelectron spectroscopy (XPS) measurements were performed on a VG ESCALAB MKII spectrometer, using mono Al Kα X-ray source (hν = 1486.71 eV, 5 mA, 15 kV) and calibrating N 1 s (N in PVP) to 399.70 eV. A thick PVP layer on the NP surface would prevent the photoelectrons from the metals to be detected. As such, NPs for XPS measurement were prepared with reduced PVP (5 instead of 20 equiv.). Fresh NP solution (4 ml) was mixed with acetone (50 ml). The mixture was centrifuged and viscous semi-solid material at the bottom of the centrifugation tube was collected, dried under vacuum, and analyzed by XPS.
X-Ray Powder Difraction (XRD) was performed on a Bruker D8 Advanced Diffractometer with Cu Kα radiation at 40 kV. The XRD sample was prepared by freeze drying the fresh NP solution (4 ml) as described in the catalyst preparation section.
Hydrogenation of Bicarbonate
Catalytic reactions were carried out in a high-pressure reactor (100 ml). NaHCO3 (1.008 g, 12 mmol) was added into the prepared nanoparticles solution. The reactor was pressurized with H2 (20 bar) and heated to 80°C for 2 h with a stirring speed of 250 rpm. After the reaction, the reactor was cooled down to room temperature and then the pressure was released. In some cases, CO2 (5–30 bar) was simultaneously purged into the reactor before the reaction.
Product Analysis
Product analysis was performed on an Agilent 1200 Series (Agilent Technologies, Germany) LC system equipped with a binary pump, an online degasser, an auto plate-sampler, and a thermostatically controlled column compartment. Chromatographic separation was carried out at 50°C on an Agilent ZorBax Hi-Plex H column (7.7 mm × 300 mm). The mobile phase used was H2SO4 aqueous solution (0.005 M). The flow rate was kept at 0.75 ml/min, run time was 15 min and the UV detector was set at 220 nm. The sample volume injection was 20 μL. A series of sodium formate was prepared (0.05 M, 0.10 M, 0.15 M, and 0.20 M, respectively). These standard solutions were used to generate a calibration curve with a R2 of 0.999.
Results and Discussion
Ni is an ideal metal for the hydrogenation of CO2, as it is cheap, abundant, and in principle magnetically recoverable and recyclable. It is among the first catalysts that are known to be active for this reaction, however, there is still no systematic investigation concerning the catalytic activity of Ni containing NPs in CO2 hydrogenation. As such Ni and eight other NiM (M represents a second metal, and the mole ratio between Ni and M was fixed to be 4:1) NPs were prepared with a classical solvent reduction method by employing PVP as the stabilizer and NaBH4 as the reducing agent in water (Yan et al., 2009b, 2010b). Following that the catalytic activity of these NPs in the hydrogenation of sodium bicarbonate into sodium formate was evaluated in the presence of 20 bar hydrogen under 80°C (see Figure 1). Pure Ni NPs were active for the reaction, with a TOF of 0.87 being observed, which is significantly more active than that of Raney Ni catalysts (Farlow and Adkins, 1935). The superior catalytic activity of PVP stabilized Ni NPs is not unexpected, as metal catalysts with smaller particle sizes generally display improved catalytic performance due to the increased percentage of surface atoms. Interestingly, the activity of the catalysts dropped in most cases when the second metal is introduced. Pt, Ag, and Cu, in particular, exhibited very strong inhibition effect. On the other hand, NiPd NPs were more active than pure Ni NPs. As a control, we synthesized pure Pd NPs under identical conditions and found that they had a comparable activity with pure Ni NPs but were less active than bimetallic NiPd NPs. Hence, it is demonstrated that the NiPd bimetallic catalyst to be superior over the monometallic catalysts as well as bimetallic catalysts with other metal combinations.
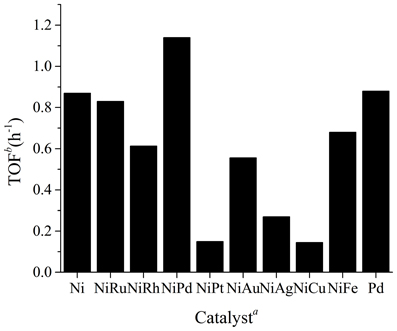
Figure 1. TOF of Ni, Pd, and NiM NPs in the hydrogenation of sodium bicarbonate to sodium formate. Reaction conditions: 0.012 mol NaHCO3, 1.2 mmol catalyst, 24 mmol PVP, 40 ml H2O, 20 bar H2, 80°C, 2h. aAll the NiM were prepared with the precursor molar ratio Ni: M = 4:1. bTOF was defined as (the mole of sodium formate)/(the mole of metal · reaction time).
In the next step, we synthesized NiPd NPs with different metal ratio, simply achieved by modifying the amount of the two metal precursors before reduction. NiPd NPs with five different compositions were prepared and subjected to catalytic test. The results are compiled in Figure 2. For easy comparison the TOF of pure Ni NPs and Pd NPs are also plotted. A volcano type of relationship between the metal ratio and the catalytic activity was observed. NiPd NPs with a Pd content of 30% (denoted as Ni7Pd3 NPs) were most active (1.20 h−1). Although the TOF of NiPd NPs is not comparable to the most active homogenous complexes, it is one of the highest values for heterogeneous catalysts reported to date.
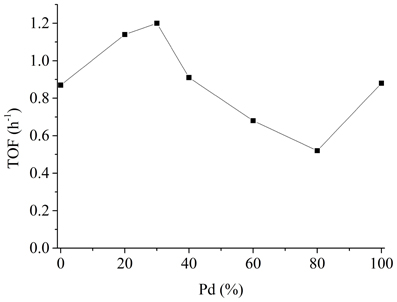
Figure 2. TOF of NiPd NPs as a function of Pd content. Reaction conditions: 0.012 mol NaHCO3, 1.2 mmol catalyst, 24 mmol PVP, 40 ml H2O, 20 bar H2, 80°C, 2h.
In some cases, metal NPs only act as the pre-catalysts for a reaction. A good example is the Pd NP catalyzed C-C coupling reaction in which the Pd(II) leached from the NP surface are believed to be the catalytic active species (Yin and Liebscher, 2006; Yan et al., 2009a). To confirm whether the reaction was truly catalyzed by heterogeneous Ni7Pd3 NPs a hot filtration experiment, initially developed by Maitlis et al. (Widegren and Finke, 2003) to distinguish homogeneous catalyst from heterogeneous ones, was carried out. The reaction was conducted as normal, quenched at 30 min, and analyzed by HPLC (45% sodium formate yield). Then the Ni7Pd3 NPs were removed by ultra-filtration and the remaining solution was used for catalysis under identical conditions. The yield of sodium formate remained unchanged (44.8%) after 1 h, indicating the reaction ceased after removal of Ni7Pd3 NPs. In addition, most homogeneous catalysts involve metal hydride as the key intermediate in the catalytic cycle. However, employing NMR to detect the presence of metal hydride in the solution was unsuccessful. These experiments provide strong evidences that the heterogeneous Ni7Pd3 NPs were responsible for the observed catalytic activity.
To rationalize the superior catalytic activity of NiPd NPs in sodium formate hydrogenation, TEM, XRD, and XPS analysis were conducted. Figures 3A–C are the representative TEM images of the Ni, Pd, and Ni7Pd3 NPs. These NPs were not well-separated from each other which makes the accurate determination of their sizes challenging. Nevertheless, it is apparent that pure Ni (20~40 nm) and Pd (~200 nm) NPs were significantly larger than Ni7Pd3 (<10 nm) NPs.
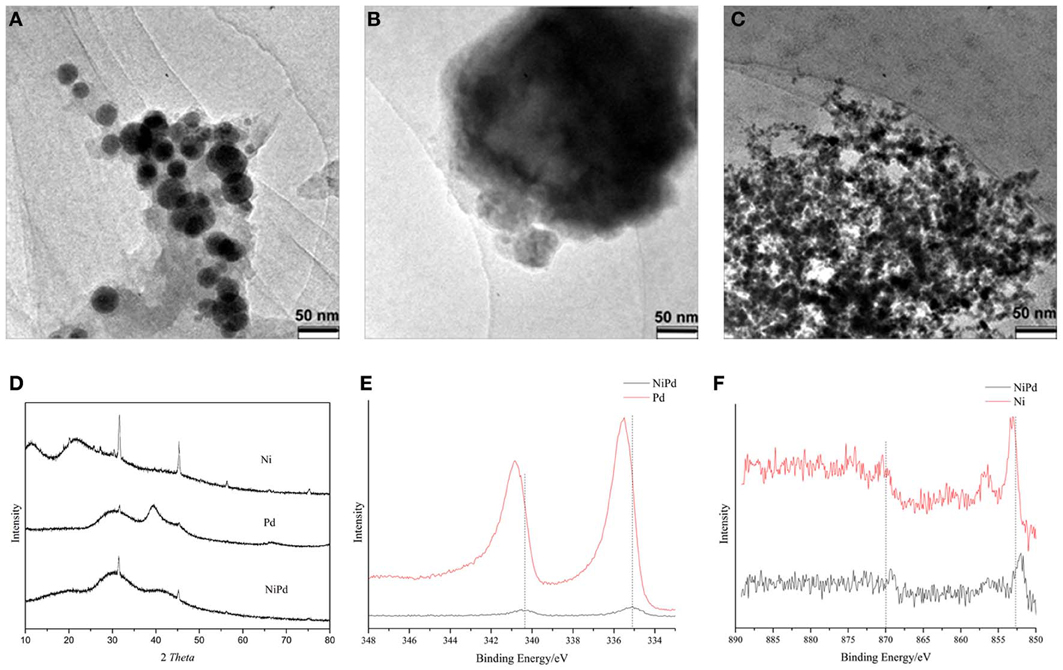
Figure 3. TEM images of (A) Ni, (B) Pd, and (C) Ni7Pd3 catalysts. (D) XRD patterns of Ni, Pd, and Ni7Pd3. (E) Pd 3d and (F) Ni 2p XPS spectra for Ni7Pd3, Ni, and Pd.
Figure 3D shows the XRD pattern of PVP stabilized Ni, Pd, and Ni7Pd3 NPs. For Ni NPs, the broad bands centered at around 2θ = 11 and 20° are characteristic of PVP polymer (Yuan et al., 2011). In the other two patterns, the broad peak at around 2θ = 30° is due to the presence of NaBO2—a byproduct in the preparation of catalyst. In all the three patterns, the peaks at 2θ = 31.7, 45.5, 56.5, 66.2 and 75.3° were observed and are due to the presence of NaCl. Pd metal exhibit XRD peaks at 2θ = 40.1 and 46.6° respectively, while for the Pd NPs the peaks slightly shifted to higher angle, in agreement with previous observation for PVP stabilized Pd NPs (Drelinkiewicz and Hasik, 2001). For both Ni and Ni7Pd3 NPs, no peak that can be ascribed to metal or metal oxide crystallite was observed, indicating they are amorphous materials, which is not uncommon for metal NPs reduced by NaBH4(Fan et al., 2009).
The XPS spectra of the Ni, Au, and Ni7Pd3 NPs are shown in Figures 3E,F (Ni 2p and Pd 3d regions). The binding energy (BE) of Pd 3d5/2 in Pd NPs was 335.5 eV, and it was 335.1 eV in Ni7Pd3 NPs. Both values are close to that of Pd(0) NPs prepared in other systems (335.3 eV), (Yuan et al., 2013) suggesting the Pd atoms are in the zero valent state. Nevertheless, the electron density in Ni7Pd3 NPs is slightly higher than that in pure Pd NPs. The BE of Ni 2p3/2 was 853.1 eV in Ni NPs and 852.0 eV in Ni7Pd3 NPs. Note that the BE of Ni 2p3/2 of Ni metal is 852.7 eV, the valent state of Ni in both NPs is metallic. Similar to Pd, the electron density of Ni in Ni7Pd3 NPs is higher than that in Ni NPs. Both Ni and Pd metal atoms are more electron-enriched in bimetallic NPs, which cannot be explained by mutual electron transfer between them, but instead indicating the Ni7Pd3 NPs are more stable against oxidation upon storage. Based on the peak intensity the ratio between Ni and Pd in the Ni7Pd3 NPs was calculated to be 3:5, dramatically lower than the stoichiometric ratio of 7:3. As XPS is a surface sensitive technique, it is plausible that the Ni7Pd3 NPs adopt a Pd enriched outer layer, core-shell structure. This structure can be rationalized by the fact that Pd has a lower surface energy and is in consistent with previous reports on the NiPd NPs. For example, Bertolini (Michel et al., 1998) and Creemers (Helfensteyn et al., 2003) discovered that the Pd surface composition of the PdNi alloy equilibrated at 600°C is higher than 80%. And the Ni79Pd21 and Ni29Pd71 NPs (with diameter 2–3 nm) exposed 53 and 91% of Pd atoms on the surface, respectively (Faudon et al., 1993; Renouprez et al., 1997).
It is widely accepted that the catalytic synergetic effect of two transitional metals in contact originates either from the so-called “ensemble effect” or the “ligand effect,” which describe the geometric and electronic modifications induced by the system constituents, respectively (Sinfelt et al., 1972). Ensemble requirement is known as the number of metal atoms required per adsorbed molecule for the most favorable path of a catalytic reaction (Sachtler, 2008). In bimetallic systems, the second metal introduced could effectively block certain ensembles but leave others intact, thus the catalytic activity would be changed due to the modifications of these ensemble. Alternatively, the second metal (termed as B) can be regarded as a “ligand” adjoining to the main surface atoms (termed as A). The bond between the surface atom A and its adsorbed molecules will be affected by the electronic interactions of B alloying with A, which is called ligand effect. NiPd bimetallic catalyst has been successfully employed in selective hydrogenation of buta-1,3-diene, (Massard et al., 2007) nitro-substituted aromatics, (Raja et al., 2005) CO2-methane dry reforming, (Steinhauer et al., 2009) formic acid electrooxidation (Li et al., 2011) and C-H bond cleavage reaction (Li et al., 2013). The enhanced catalytic performance of NiPd NPs were ascribed to the electron donation of Ni to Pd, (Ruban et al., 1997) the compressive strain of Pd atoms to adapt the Ni bulk lattice, (Filhol et al., 2002) and the unusual surface structure caused by surface relaxation (Filhol et al., 2001). In our system, however, these factors are unlikely to be the major reason for the superior activity of Ni7Pd3 NPs. XPS analysis indicates there is no clear evidence for the electron donation from Ni to Pd. XRD reveals the Ni7Pd3 NPs are amorphous, suggesting a majority of Pd and Ni atoms do not adapt a bulk lattice, and therefore should not suffer a significant compressive strain.
Based on TEM, XPS, and XRD analysis, we attribute the improved catalytic performance of Ni7Pd3 NPs to two main factors. First of all, the Ni7Pd3 NPs are smaller in size compared to pure Ni and Pd NPs, resulting in a higher exposer of surface atoms for catalysis. The second reason is that zero valent atoms on the NP surface are responsible for the catalytic activity. Ni7Pd3 NPs are more stable against oxidation so that they have a higher percentage of zero valent sites for the hydrogenation of sodium bicarbonate. It has been demonstrated previously that in the synthesis of bimetallic NPs from two metal salts, the presence of a noble metal salt could significantly promote the reduction of the 3-d metal such as Ni (Wang and Li, 2010). In our study, it appears that the incorporation of Pd not only promote the reduction of Ni salts during synthesis, but also stabilize the zero valent Ni atoms during storage and reaction. Unfortunately, we are not able to find a proper explanation for this effect and have to study in more detail in the future.
For CO2 hydrogenation into formic acid over homogeneous catalysts, the reaction rate is usually a function of CO2 and H2 gas pressure. In most cases, the reaction is of first order with respect to H2 pressure, (Tsai and Nicholas, 1992; Hutschka et al., 1997) although there is a report indicating at higher pressures (>60 bar) saturation kinetics was observed (lower than first order) (Thomas et al., 2001). We analyzed the catalytic activities of Ni7Pd3 NPs with varying H2 pressure (see Figure 4A). At the range of 5–40 bar, the TOF of the NPs were essentially irrelevant to the H2 concentration, suggesting the mechanism of the reaction over Ni7Pd3 NPs are fundamentally different from those over homogeneous catalysts. For homogeneous catalysts, the key step(s) in the entire catalytic cycle is generally the formation of the metal hydride, (Ziebart et al., 2012) which necessitates a high hydrogen pressure. Unlike the homogeneous catalysts, Ni (Yu and Hou, 2013) and Pd (Tungler and Fogassy, 2001) metals are well known for their ability to absorb and dissociate H2 molecules on the surface under very mild conditions. Thus, H2 activation is not the rate determine step and the concentration of H2 is not a major factor to influence the catalytic activity.
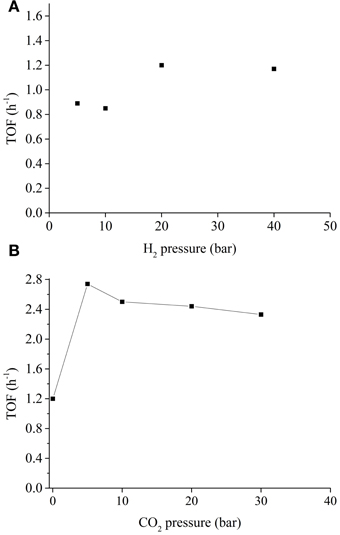
Figure 4. (A) TOF as a function of H2 pressure. Reaction condition: 0.012 mol NaHCO3, 1.2 mmol Ni7Pd3, 0.024 mol PVP, 40 ml H2O, 80°C, 2 h; (B) TOF as a function of CO2 pressure. Reaction condition: 0.012 mol NaHCO3, 1.2 mmol Ni7Pd3, 0.024 mol PVP, 40 ml H2O, 40 bar H2, 80°C, 2 h.
The relationship between catalytic activity and CO2 pressure was also explored (see Figure 4B). Introducing CO2 into the system has a significant promotion effect on the reaction rate. In the presence of 5 bar CO2, the TOF of the Ni7Pd3 NPs increased sharply to 2.8, as compared to 1.2 under CO2 free conditions. This is in agreement with previous reports using homogeneous catalysts where the system became more active when a mixture of CO2 and H2 gas was used instead of pure H2 (Himeda et al., 2004, 2007; Federsel et al., 2010b). A further increase in CO2 concentration has negligible effect on the reaction rate. The enhancement of TOF implies that the reaction mechanism might be modified in the presence of CO2. Probably, CO2 is more active than bicarbonate for the hydrogenation over Ni7Pd3 NPs. When CO2 is present in the system, it is CO2 instead of bicarbonate anion that inserts into the metal-hydrogen bond to form formate. The major function of sodium bicarbonate is to react with the formic acid generated to make the transformation thermodynamically favorable (see Figure 5).
Conclusion
For the first time, transformation of sodium bicarbonate and CO2 into sodium formate over eight Ni based metal NPs were systematically investigated. NiPd NPs with a Ni:Pd ratio of 7:3 was identified to be the most active catalyst for this reaction. Under low temperature and base free conditions, the optimized TOF of the Ni7Pd3 NPs in sodium bicarbonate hydrogenation reaches 2.8 h−1, which is among the highest for heterogeneous catalysts. The superior catalytic activity of the Ni7Pd3 NPs over pure Ni and Pd NPs were ascribed to the smaller size of the bimetallic NPs and the stabilization effect of Pd to Ni atoms. Kinetic study indicates that H2 is easily activated over the Ni7Pd3 NPs which is fundamentally different from homogeneous catalysts. Although many homogeneous complexes with much higher catalytic activity are known, the robustness of this system, the use of inexpensive metals and the heterogeneous nature (which means facile recycling) make the Ni7Pd3 NPs attractive, and worth further investigation, in CO2 hydrogenation.
Conflict of Interest Statement
The authors declare that the research was conducted in the absence of any commercial or financial relationships that could be construed as a potential conflict of interest.
Acknowledgments
We thank the AcRF Tier 1 project from MOE (R-279-000-387-112) for financial support.
References
Baiker, A. (2000). Utilization of carbon dioxide in heterogeneous catalytic synthesis. Appl. Organomet. Chem. 14, 751–762. doi: 10.1002/1099-0739(200012)14:12<751::AID-AOC85>3.0.CO;2-J
Barrault, J., and Urresta, J. (1999). Hydrogénation du dioxyde de carbone en méthanol en présence de catalyseurs à base de molybdène. C. R. Acad. Sci. IIC Chem. 2, 167–174. doi: 10.1016/S1387-1609(99)80060-7
Boddien, A., Gärtner, F., Federsel, C., Sponholz, P., Mellmann, D., Jackstell, R., et al. (2011a). CO2-“neutral” hydrogen storage based on bicarbonates and formates. Angew. Chem. Int. Ed. Engl. 50, 6411–6414. doi: 10.1002/anie.201101995
Boddien, A., Mellmann, D., Gärtner, F., Jackstell, R., Junge, H., Dyson, P. J., et al. (2011b). Efficient dehydrogenation of formic acid using an iron catalyst. Science 333, 1733–1736. doi: 10.1126/science.1206613
Dai, W.-L., Luo, S.-L., Yin, S.-F., and Au, C.-T. (2009). The direct transformation of carbon dioxide to organic carbonates over heterogeneous catalysts. Appl. Catal. A Gen. 366, 2–12. doi: 10.1016/j.apcata.2009.06.045
Drelinkiewicz, A., and Hasik, M. (2001). 2-ethyl-9,10-anthraquinone hydrogenation over Pd/polymers: effect of polymers–Pd(II) chlorocomplexes interactions. J. Mol. Catal. A Chem. 177, 149–164. doi: 10.1016/S1381-1169(01)00315-6
Elek, J., Nádasdi, L., Papp, G., Laurenczy, G., and Joó, F. (2003). Homogeneous hydrogenation of carbon dioxide and bicarbonate in aqueous solution catalyzed by water-soluble ruthenium(II) phosphine complexes. Appl. Catal. A Gen. 255, 59–67. doi: 10.1016/S0926-860X(03)00644-6
Fan, X.-B., Yan, N., Tao, Z.-Y., Evans, D., Xiao, C.-X., and Kou, Y. (2009). One-step synthesis of 2-alkyl-dioxolanes from ethylene glycol and syngas. ChemSusChem 2, 941–943. doi: 10.1002/cssc.200900163
Farlow, M. W., and Adkins, H. (1935). The hydrogenation of carbon dioxide and a correction of the reported synthesis of urethans. J. Am. Chem. Soc. 57, 2222–2223. doi: 10.1021/ja01314a054
Faudon, J. F., Senocq, F., Bergeret, G., Moraweck, B., Clugnet, G., Nicot, C., et al. (1993). Properties of supported Pd-Ni catalysts prepared by coexchange and by organometallic chemistry: I. Preparation and characterization by x-ray-diffraction, analytical microscopy, and exafs. J. Catal. 144, 460–471. doi: 10.1006/jcat.1993.1346
Federsel, C., Boddien, A., Jackstell, R., Jennerjahn, R., Dyson, P. J., Scopelliti, R., et al. (2010a). A well-defined iron catalyst for the reduction of bicarbonates and carbon dioxide to formates, alkyl formates, and formamides. Angew. Chem. Int. Ed. Engl. 49, 9777–9780. doi: 10.1002/anie.201004263
Federsel, C., Jackstell, R., Boddien, A., Laurenczy, G., and Beller, M. (2010b). Ruthenium-catalyzed hydrogenation of bicarbonate in water. ChemSusChem 3, 1048–1050. doi: 10.1002/cssc.201000151
Fellay, C., Yan, N., Dyson, P. J., and Laurenczy, G. (2009). Selective formic acid decomposition for high-pressure hydrogen generation: a mechanistic study. Chem. Eur. J. 15, 3752–3760. doi: 10.1002/chem.200801824
Filhol, J. S., Saint-Lager, M. C., De Santis, M., Dolle, P., Simon, D., Baudoing-Savois, R., et al. (2002). Highly strained structure of a four-layer deposit of Pd on Ni(110): a coupled theoretical and experimental study. Phys. Rev. Lett. 89:146106. doi: 10.1103/PhysRevLett.89.146106
Filhol, J. S., Simon, D., and Sautet, P. (2001). Stress induced nanostructure in a Pd monolayer on Ni(110): a first principles theoretical study. Surf. Sci. 472, L139–L144. doi: 10.1016/S0039-6028(00)00952-3
Ghazali-Esfahani, S., Song, H., Paunescu, E., Bobbink, F. D., Liu, H., Fei, Z., et al. (2013). Cycloaddition of CO2 to epoxides catalyzed by imidazolium-based polymeric ionic liquids. Green Chem. 15, 1584–1589. doi: 10.1039/c3gc37085b
Hao, C., Wang, S., Li, M., Kang, L., and Ma, X. (2011). Hydrogenation of CO2 to formic acid on supported ruthenium catalysts. Catal. Today 160, 184–190. doi: 10.1016/j.cattod.2010.05.034
Helfensteyn, S., Luyten, J., Feyaerts, L., and Creemers, C. (2003). Modelling surface phenomena in Pd·Ni alloys. Appl. Surf. Sci. 212–213, 844–849. doi: 10.1016/S0169-4332(03)00088-6
Himeda, Y. (2007). Conversion of CO2 into formate by homogeneously catalyzed hydrogenation in water: tuning catalytic activity and water solubility through the acid-base equilibrium of the ligand. Eur. J. Inorg. Chem. 3927–3941. doi: 10.1002/ejic.200700494
Himeda, Y., Miyazawa, S., and Hirose, T. (2011). Interconversion between formic acid and H2/CO2 using rhodium and ruthenium catalysts for CO2 fixation and H2 storage. ChemSusChem 4, 487–493. doi: 10.1002/cssc.201000327
Himeda, Y., Onozawa-Komatsuzaki, N., Sugihara, H., Arakawa, H., and Kasuga, K. (2003). Transfer hydrogenation of a variety of ketones catalyzed by rhodium complexes in aqueous solution and their application to asymmetric reduction using chiral schiff base ligands. J. Mol. Catal. A Chem. 195, 95–100. doi: 10.1016/S1381-1169(02)00576-9
Himeda, Y., Onozawa-Komatsuzaki, N., Sugihara, H., Arakawa, H., and Kasuga, K. (2004). Half-sandwich complexes with 4,7-dihydroxy-1,10-phenanthroline: water-soluble, highly efficient catalysts for hydrogenation of bicarbonate attributable to the generation of an oxyanion on the catalyst ligand. Organometallics 23, 1480–1483. doi: 10.1021/om030382s
Himeda, Y., Onozawa-Komatsuzaki, N., Sugihara, H., and Kasuga, K. (2006). Highly efficient conversion of carbon dioxide catalyzed by half-sandwich complexes with pyridinol ligand: the electronic effect of oxyanion. J. Photochem. Photobiol. A Chem. 182, 306–309. doi: 10.1016/j.jphotochem.2006.04.025
Himeda, Y., Onozawa-Komatsuzaki, N., Sugihara, H., and Kasuga, K. (2007). Simultaneous tuning of activity and water solubility of complex catalysts by acid-base equilibrium of ligands for conversion of carbon dioxide. Organometallics 26, 702–712. doi: 10.1021/om060899e
Hutschka, F., Dedieu, A., Eichberger, M., Fornika, R., and Leitner, W. (1997). Mechanistic aspects of the rhodium-catalyzed hydrogenation of CO2 to formic acida theoretical and kinetic study. J. Am. Chem. Soc. 119, 4432–4443. doi: 10.1021/ja961579x
Imai, T., Kuroda, K., Hirano, M., and Akano, T. (1995). Methanol synthesis by catalytic hydrogenation of carbon dioxide on CuO-ZnO-Al2O3 catalysts. Kagaku kōgaku ronbunshū 21, 444–450. doi: 10.1252/kōkoronbunshō.21.444
Inoue, Y. I. H., Sasaki, Y., and Hashimoto, H. (1976). Catalytic fixation of carbon dioxide to formic acid by transition-metal complexes under mild conditions. Chem. Lett. 863–864. doi: 10.1246/cl.1976.863
Jessop, P. G., Ikariya, T., and Noyori, R. (1995). Homogeneous hydrogenation of carbon dioxide. Chem. Rev. 95, 259–272. doi: 10.1021/cr00034a001
Kerry Yu, K. M., and Tsang, S. (2011). A study of methyl formate production from carbon dioxide hydrogenation in methanol over a copper zinc oxide catalyst. Catal. Lett. 141, 259–265. doi: 10.1007/s10562-010-0500-3
Kilo, M., Weigel, J., Wokaun, A., Koeppel, R. A., Stoeckli, A., and Baiker, A. (1997). Effect of the addition of chromium- and manganese oxides on structural and catalytic properties of copper/zirconia catalysts for the synthesis of methanol from carbon dioxide. J. Mol. Catal. A Chem. 126, 169–184. doi: 10.1016/S1381-1169(97)00109-X
Kohno, K., and Sakakura, T. (2009). Catalytic transformation of carbon dioxide to organic carbonates. Yūki gōsei kagaku kyōkaishi 67, 921–933. doi: 10.5059/yukigoseikyokaishi.67.921
La, K. W., Jung, J. C., Kim, H., Baeck, S.-H., and Song, I. K. (2007). Effect of acid–base properties of H3PW12O40/CexTi1-xO2 catalysts on the direct synthesis of dimethyl carbonate from methanol and carbon dioxide: a TPD study of H3PW12O40/CexTi1-xO2 catalysts. J. Mol. Catal. A Chem. 269, 41–45. doi: 10.1016/j.molcata.2007.01.006
Laurenczy, G., Jedner, S., Alessio, E., and Dyson, P. J. (2007). In situ NMR characterisation of an intermediate in the catalytic hydrogenation of CO2 and HCO3– in aqueous solution. Inorg. Chem. Commun. 10, 558–562. doi: 10.1016/j.inoche.2007.01.020
Lee, D.-K., Kim, D.-S., and Kim, S.-W. (2001). Selective formation of formaldehyde from carbon dioxide and hydrogen over PtCu/SiO2. Appl. Organomet. Chem. 15, 148–150. doi: 10.1002/1099-0739(200102)15:2<148::AID-AOC104>3.0.CO;2-N
Lee, H., Park, S., Song, I., and Jung, J. (2011). Direct synthesis of dimethyl carbonate from methanol and carbon dioxide over Ga2O3/Ce0.6Zr0.4O2 catalysts: effect of acidity and basicity of the catalysts. Catal. Lett. 141, 531–537. doi: 10.1007/s10562-010-0544-4
Li, H. S., and Zhong, S. H. (2002). Dimethyl carbonate synthesis from carbon dioxide and methanol. Process. Chem. 14, 368–373.
Li, K., Zhou, Z., Wang, Y., and Wu, Z. (2013). A theoretical study of CH4 dissociation on NiPd(111) surface. Surf. Sci. 612, 63–68. doi: 10.1016/j.susc.2013.02.012
Li, R., Wei, Z., Huang, T., and Yu, A. (2011). Ultrasonic-assisted synthesis of Pd–Ni alloy catalysts supported on multi-walled carbon nanotubes for formic acid electrooxidation. Electrochim. Acta 56, 6860–6865. doi: 10.1016/j.electacta.2011.05.097
Massard, R., Uzio, D., Thomazeau, C., Pichon, C., Rousset, J. L., and Bertolini, J. C. (2007). Strained Pd overlayers on Ni nanoparticles supported on alumina and catalytic activity for buta-1,3-diene selective hydrogenation. J. Catal. 245, 133–143. doi: 10.1016/j.jcat.2006.09.014
Michel, A. C., Lianos, L., Rousset, J. L., Delichère, P., Prakash, N. S., Massardier, J., et al. (1998). Surface characterization and reactivity of Pd8Ni92 (111) and (110) alloys. Surf. Sci. 416, 288–294. doi: 10.1016/S0039-6028(98)00614-1
Natesakhawat, S., Lekse, J. W., Baltrus, J. P., Ohodnicki, P. R., Howard, B. H., Deng, X., et al. (2012). Active sites and structure–activity relationships of copper-based catalysts for carbon dioxide hydrogenation to methanol. ACS Catal. 2, 1667–1676. doi: 10.1021/cs300008g
Peng, G., Sibener, S. J., Schatz, G. C., Ceyer, S. T., and Mavrikakis, M. (2011). CO2 hydrogenation to formic acid on Ni(111). J. Phys. Chem. C 116, 3001–3006. doi: 10.1021/jp210408x
Preti, D., Resta, C., Squarcialupi, S., and Fachinetti, G. (2011). Carbon dioxide hydrogenation to formic acid by using a heterogeneous gold catalyst. Angew. Chem. Int. Ed. Engl. 50, 12551–12554. doi: 10.1002/anie.201105481
Raja, R., Golovko, V. B., Thomas, J. M., Berenguer-Murcia, A., Zhou, W., Xie, S., et al. (2005). Highly efficient catalysts for the hydrogenation of nitro-substituted aromatics. Chem. Commun. 2026–2028. doi: 10.1039/b418273a
Renouprez, A., Faudon, J. F., Massardier, J., Rousset, J. L., Delichère, P., and Bergeret, G. (1997). Properties of supported Pd–Ni catalysts prepared by coexchange and organometallic chemistry. J. Catal. 170, 181–190. doi: 10.1006/jcat.1997.1729
Riedel, T., Schulz, H., Schaub, G., Jun, K.-W., Hwang, J.-S., and Lee, K.-W. (2003). Fischer–Tropsch on iron with H2/CO and H2/CO2 as synthesis gases: the episodes of formation of the Fischer–Tropsch regime and construction of the catalyst. Top. Catal. 26, 41–54. doi: 10.1023/B:TOCA.0000012986.46680.28
Roucoux, A., Schulz, J., and Patin, H. (2002). Reduced transition metal colloids: a novel family of reusable catalysts? Chem. Rev. 102, 3757–3778. doi: 10.1021/cr010350j
Ruban, A., Hammer, B., Stoltze, P., Skriver, H. L., and Nørskov, J. K. (1997). Surface electronic structure and reactivity of transition and noble metals. J. Mol. Catal. A Chem. 115, 421–429. doi: 10.1016/S1381-1169(96)00348-2
Sachtler, W. M. H. (2008). “Ensemble and ligand effects in metal catalysis,” in Handbook of Heterogeneous Catalysis, eds G. Ertl, H. Knözinger, and J. Weitkamp (Weinheim: Wiley-VCH Verlag GmbH and Co. KGaA), 1585–1593. doi: 10.1002/9783527610044.hetcat0086
Sai Prasad, P. S., Bae, J., Jun, K.-W., and Lee, K.-W. (2008). Fischer–Tropsch synthesis by carbon dioxide hydrogenation on Fe-based catalysts. Cata. Surv. Asia. 12, 170–183. doi: 10.1007/s10563-008-9049-1
Sakakura, T., Choi, J.-C., and Yasuda, H. (2007). Transformation of carbon dioxide. Chem. Rev. 107, 2365–2387. doi: 10.1021/cr068357u
Sanz, S., Azua, A., and Peris, E. (2010). ‘(η6-arene)Ru(bis-NHC)’ complexes for the reduction of CO2 to formate with hydrogen and by transfer hydrogenation with iproh. Dalton Trans. 39, 6339–6343. doi: 10.1039/c003220d
Schmeier, T. J., Dobereiner, G. E., Crabtree, R. H., and Hazari, N. (2011). Secondary coordination sphere interactions facilitate the insertion step in an iridium(III) CO2 reduction catalyst. J. Am. Chem. Soc. 133, 9274–9277. doi: 10.1021/ja2035514
Scott, R. W. J., Wilson, O. M., and Crooks, R. M. (2005). Synthesis, characterization, and applications of dendrimer-encapsulated nanoparticles. J. Phys. Chem. B 109, 692–704. doi: 10.1021/jp0469665
Sharma, S., Hu, Z., Zhang, P., Mcfarland, E. W., and Metiu, H. (2011). CO2 methanation on Ru-doped ceria. J. Catal. 278, 297–309. doi: 10.1016/j.jcat.2010.12.015
Sinfelt, J. H., Carter, J. L., and Yates, D. J. C. (1972). Catalytic hydrogenolysis and dehydrogenation over copper-nickel alloys. J. Catal. 24, 283–296. doi: 10.1016/0021-9517(72)90072-3
Steinhauer, B., Kasireddy, M. R., Radnik, J., and Martin, A. (2009). Development of Ni-Pd bimetallic catalysts for the utilization of carbon dioxide and methane by dry reforming. Appl. Catal. A 366, 333–341. doi: 10.1016/j.apcata.2009.07.021
Tanaka, R., Yamashita, M., and Nozaki, K. (2009). Catalytic hydrogenation of carbon dioxide using Ir(III)-pincer complexes. J. Am. Chem. Soc. 131, 14168–14169. doi: 10.1021/ja903574e
Thomas, C. A., Bonilla, R. J., Huang, Y., and Jessop, P. G. (2001). Hydrogenation of carbon dioxide catalyzed by ruthenium trimethylphosphine complexes—effect of gas pressure and additives on rate in the liquid phase. Can. J. Chem. 79, 719–724. doi: 10.1139/cjc-79-5-6-719
Tomishige, K., Furusawa, Y., Ikeda, Y., Asadullah, M., and Fujimoto, K. (2001). CeO2–ZrO2 solid solution catalyst for selective synthesis of dimethyl carbonate from methanol and carbon dioxide. Catal. Lett. 76, 71–74. doi: 10.1023/A:1016711722721
Tsai, J. C., and Nicholas, K. M. (1992). Rhodium-catalyzed hydrogenation of carbon dioxide to formic acid. J. Am. Chem. Soc. 114, 5117–5124. doi: 10.1021/ja00039a024
Tungler, A., and Fogassy, G. (2001). Catalysis with supported palladium metal, selectivity in the hydrogenation of C:C, C:O and C:N bonds, from chemo- to enantioselectivity. J. Mol. Catal. A Chem. 173, 231–247. doi: 10.1016/S1381-1169(01)00152-2
Wang, D., and Li, Y. (2010). One-pot protocol for Au-based hybrid magnetic nanostructures via a noble-metal-induced reduction process. J. Am. Chem. Soc. 132, 6280–6281. doi: 10.1021/ja100845v
Wan-Hui, W., and Yuichiro, H. (2012). “Recent advances in transition metal-catalysed homogeneous hydrogenation of carbon dioxide in aqueous media,” in Hydrogenation, ed I. Karamé (Rijeka: InTech), 249–268.
Wesselbaum, S., Vom Stein, T., Klankermayer, J., and Leitner, W. (2012). Hydrogenation of carbon dioxide to methanol by using a homogeneous ruthenium–phosphine catalyst. Angew. Chem. Int. Ed. 51, 7499–7502. doi: 10.1002/anie.201202320
Widegren, J. A., and Finke, R. G. (2003). A review of the problem of distinguishing true homogeneous catalysis from soluble or other metal-particle heterogeneous catalysis under reducing conditions. J. Mol. Catal. A Chem. 198, 317–341. doi: 10.1016/S1381-1169(02)00728-8
Yan, N., Xiao, C., and Kou, Y. (2010a). Transition metal nanoparticle catalysis in green solvents. Coord. Chem. Rev. 254, 1179–1218. doi: 10.1016/j.ccr.2010.02.015
Yan, N., Zhang, J., Yuan, Y., Chen, G.-T., Dyson, P. J., Li, Z.-C., et al. (2010b). Thermoresponsive polymers based on poly-vinylpyrrolidone: applications in nanoparticle catalysis. Chem. Commun. 46, 1631–1633. doi: 10.1039/b923290g
Yan, N., Yang, X., Fei, Z., Li, Y., Kou, Y., and Dyson, P. J. (2009a). Solvent-enhanced coupling of sterically hindered reagents and aryl chlorides using functionalized ionic liquids. Organometallics 28, 937–939. doi: 10.1021/om801083c
Yan, N., Zhang, J.-G., Tong, Y., Yao, S., Xiao, C., Li, Z., et al. (2009b). Solubility adjustable nanoparticles stabilized by a novel PVP based family: synthesis, characterization and catalytic properties. Chem. Commun. 0, 4423–4425. doi: 10.1039/b905625d
Yin, and Liebscher, J. (2006). Carbon-carbon coupling reactions catalyzed by heterogeneous palladium catalysts. Chem. Rev. 107, 133–173. doi: 10.1021/cr0505674
Yin, C., Xu, Z., Yang, S.-Y., Ng, S. M., Wong, K. Y., Lin, Z., et al. (2001). Promoting effect of water in ruthenium-catalyzed hydrogenation of carbon dioxide to formic acid. Organometallics 20, 1216–1222. doi: 10.1021/om000944x
Yuan, X., Sun, G., Asakura, H., Tanaka, T., Chen, X., Yuan, Y., et al. (2013). Development of palladium surface-enriched heteronuclear Au–Pd nanoparticle dehalogenation catalysts in an ionic liquid. Chem. Eur. J. 19, 1227–1234. doi: 10.1002/chem.201203605
Yu, Y., and Hou, Z. (2013). Soluble nickel nanoparticles for catalytic hydrogenation. Curr. Org. Chem. 17, 336–347. doi: 10.2174/1385272811317040004
Yuan, Y., Yan, N., and Dyson, P. J. (2011). pH-sensitive gold nanoparticle catalysts for the aerobic oxidation of alcohols. Inorg. Chem. 50, 11069–11074. doi: 10.1021/ic201608j
Yuan, Y., Yan, N., and Dyson, P. J. (2012). Advances in the rational design of rhodium nanoparticle catalysts: control via manipulation of the nanoparticle core and stabilizer. ACS Catal. 2, 1057–1069. doi: 10.1021/cs300142u
Zhang, Y., Fei, J., Yu, Y., and Zheng, X. (2004). Silica immobilized ruthenium catalyst used for carbon dioxide hydrogenation to formic acid (I): the effect of functionalizing group and additive on the catalyst performance. Catal. Commun. 5, 643–646. doi: 10.1016/j.catcom.2004.08.001
Keywords: hydrogenation, carbon dioxide, sodium bicarbonate, sodium formate, nickel, palladium, nanoparticles, heterogeneous
Citation: Wang M, Zhang J and Yan N (2013) Transformation of sodium bicarbonate and CO2 into sodium formate over NiPd nanoparticle catalyst. Front. Chem. 1:17. doi: 10.3389/fchem.2013.00017
Received: 16 May 2013; Paper pending published: 20 June 2013;
Accepted: 29 August 2013; Published online: 18 September 2013.
Edited by:
Feng Luo, East China Institute of Technology, ChinaReviewed by:
Francisco Martin-Martinez, Vrije Universiteit Brussel, BelgiumChen Zhao, Technische Universitaet Muenchen, Germany
Copyright © 2013 Wang, Zhang and Yan. This is an open-access article distributed under the terms of the Creative Commons Attribution License (CC BY). The use, distribution or reproduction in other forums is permitted, provided the original author(s) or licensor are credited and that the original publication in this journal is cited, in accordance with accepted academic practice. No use, distribution or reproduction is permitted which does not comply with these terms.
*Correspondence: Ning Yan, Department of Chemical and Biomolecular Engineering, National University of Singapore, 4 Engineering Drive 4, 117576, Singapore e-mail: ning.yan@nus.edu.sg