New anionic cobalt(III) complexes enable enantioselective synthesis of spiro-fused oxazoline and iodoacetal derivatives
- 1SANKEN, Osaka University, Ibaraki, Japan
- 2Pharmaceutical Organic Chemistry Department, Faculty of Pharmacy, Suez Canal University, Ismailia, Egypt
Anionic salicylimine-based cobalt (III) complexes featuring chiral ligands derived from isoleucine amino acids were used as efficient bifunctional phase-transfer catalysts for electrophilic iodination of enol ethers. The Brønsted acids of these complexes enabled the enantioselective asymmetric iodocyclization of enol ethers, furnishing spiro-fused oxazoline derivatives in high yields with up to 90:10 er. In addition, chiral cobalt (III) complexes catalyze the asymmetric intermolecular iodoacetalization of enol ethers with various alcohols to afford 3-iodoacetal derivatives in high yields with up to 92:8 er.
1 Introduction
Chiral anionic phase-transfer catalysis has emerged as a distinct strategy to achieve various stereoselective transformations (Phipps et al., 2012; Kaneko et al., 2016; Larionov et al., 2021). Nelson et al. introduced this concept for the first time (Carter et al., 2003), but the mode of catalysis remained elusive until Toste et al. realized that the in situ replacement of ions solubilizes the cationic reagent in the organic layer and provides a chiral environment for the desired reaction with the substrate (Scheme 1A) (Rauniyar et al., 2011). Toste et al. chose Selectfluor™ as a model reagent to be solubilized and rendered chiral through ion-pairing with chiral phosphate anion catalysts for enantioselective fluorocyclization of enol ethers (Scheme 1B) (Rauniyar et al., 2011). This milestone was followed by various anionic phase-transfer catalysts and their application to different enantioselective transformations (Wang et al., 2012; Phipps and Toste, 2013; Yang et al., 2014; Yamamoto et al., 2016; Avila et al., 2017; Biswas et al., 2018; Liang et al., 2018; Long et al., 2021). Although most of these anionic catalysts show high efficiency in many reactions, their synthetic protocols are quite lengthy and expensive. Hence, novel chiral anionic platforms are highly desirable for opening new horizons for this promising anionic phase-transfer strategy.
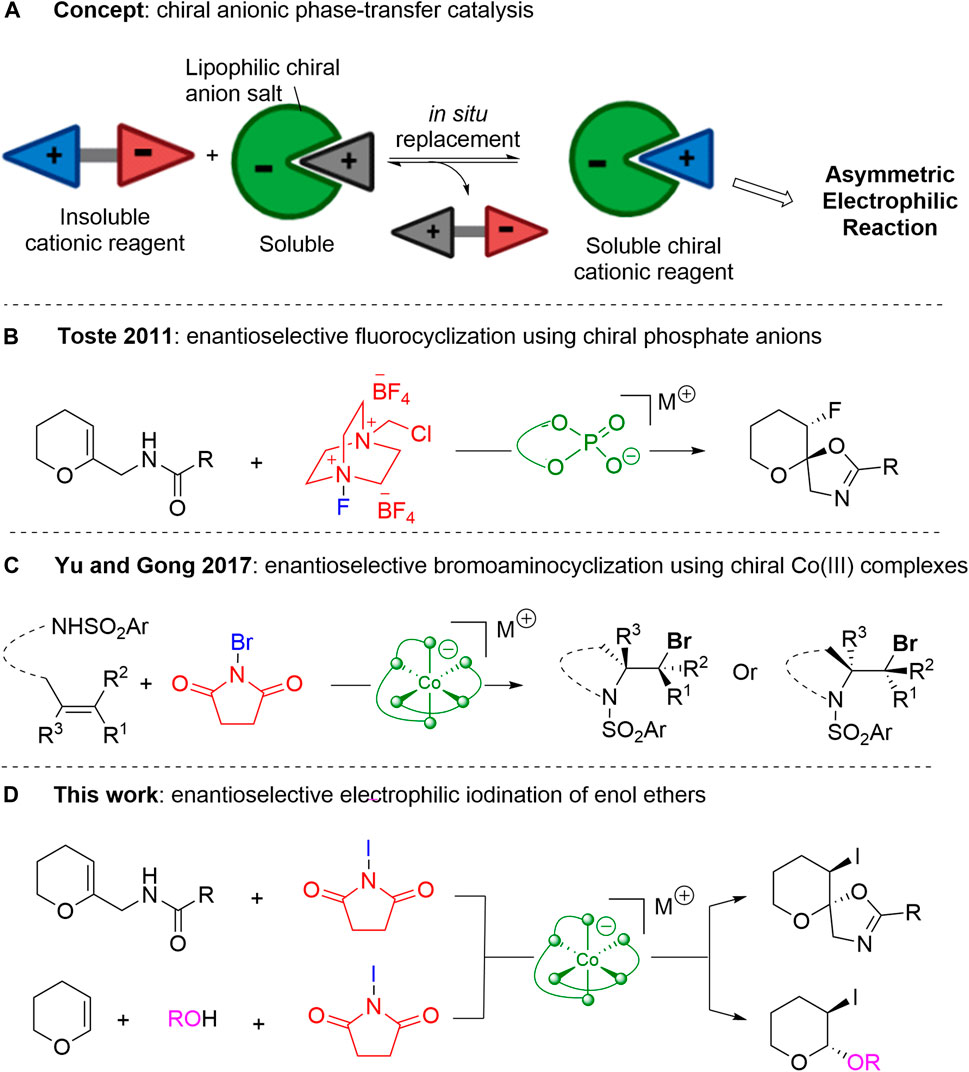
SCHEME 1. Chiral Anion-mediated Enantioselective Halocyclization. (A) Concept of anionic phase transfer catalysis. (B) Chiral phosphate anion-mediated enantioselective fluorocyclization. (C) Chiral cobalt anion-mediated enantioselective bromoaminocyclization. (D) Chiral cobalt anion-mediated enantioselective iodocyclization and iodoacetalization of enol ethers.
Belokon et al. introduced a new series of octahedral anionic salicylimine-based cobaltate (III) complexes that could be readily prepared via a facile one-pot protocol (Belokon et al., 1977). The three-dimensional arrangement of two enantiopure tridentate salicylimine-based ligands around the octahedral cobalt center can render the center stereogenic, generating two types of meridional isomers: Λ and Δ mer isomers (Ghosh et al., 2017; Cruchter and Larionov, 2018). The metal center itself does not play any role in the catalytic cycle, although it offers a rigid framework to create a chiral environment.
In the last decade, the use of anionic chiral cobalt (III) complexes as phase-transfer catalysts for different asymmetric transformations has received much attention (Gong et al., 2014; Yan et al., 2017; Cruchter and Larionov, 2018; Liu K et al., 2018; Larionov et al., 2021). This can be attributed to the ease of creating a wide range of new structurally diverse chiral metal complexes with distinct features. The positive charge on the cobalt center is compensated for by ligand coordination to generate a lipophilic chiral anion that can be either in the form of a Brønsted acid or salt. Although the separation of these two mer isomers via column chromatography (Belokon et al., 2004) is essential for their application, the highly stereocontrolled preparation of octahedral cobalt complexes remains challenging (Belokon et al., 2012; Larionov et al., 2015; Larionov et al., 2016; Salem et al., 2021).
Generally, two octahedral anionic salicylimine-based cobaltate (III) complexes can be distinguished: stereogenic-(only)-at-metal complexes and stereogenic-at-metal complexes featuring chiral ligands (Cruchter and Larionov, 2018). In the first type, cobalt acts as the exclusive element of chirality; for example, the first complex reported by Belokon et al., in which the salicylimine ligand was derived from a glycine amino acid (Belokon et al., 1977). Alternatively, stereogenic-at-metal complexes featuring chiral ligands represent the main proportion of salicylimine-based cobalt (III) complexes and offer two significant advantages over the first type. First, it is easier to separate and purify the two mer isomers compared to other stereogenic-(only)-at-metal complexes that require either expensive chiral counter cations or resolution via chiral HPLC. Second, this additional element of chirality creates an optimum chiral environment that affords enantioselective transformations. Therefore, only the second type of complex was used as a chiral anionic phase-transfer catalyst.
In 2017, Yu et al. reported the enantioselective bromoaminocyclization of olefins using anionic cobalt (III) complexes as bifunctional phase-transfer catalysts to shuttle the less-soluble brominating reagent to the reaction solution and to control stereoselectivity (Scheme 1C) (Jiang et al., 2017). Similarly, Yu et al. utilized anionic cobalt (III) complexes for the intramolecular and intermolecular halogenation of olefinic bonds (Li et al., 2018; Liu W et al., 2018; Wang et al., 2019; Sun et al., 2021; Wu et al., 2021). However, the enantioselective iodocyclization of enol ethers has been studied less because of the limitations posed by the background reaction.
Thus, in this work, we conduct an anionic octahedral cobalt (III) complex-mediated enantioselective iodocyclization reaction of enol ethers and a diastereoselective synthetic study of anionic octahedral cobalt (III) complexes as bifunctional phase-transfer catalysts (Scheme 1D).
2 Results and discussion
2.1 Iodocyclization of enol ethers
2.1.1 Catalyst screening
Enol ether derivative 1a was selected as the model starting material to investigate its iodocyclization reaction, which can afford spiro-fused oxazoline scaffold 2a, the core structure of many chiral ligands, and biologically active compounds as one of the N-heterocycles (Banks et al., 2001; Heckmann and Bach, 2005; Holmes et al., 2005; Yadav et al., 2011; Bhandari Neupane et al., 2019; Bylo et al., 2020; Salem et al., 2020; Connon et al., 2021; Gardouh et al., 2021). The reaction of enol ether derivative 1a with N-iodosuccinimide (NIS) was initially examined in the presence of 10 mol% of the sodium salts of anionic chiral cobalt (III) complexes: Λ-(S,S)-3a and Λ-(S,S)-3b (Table 1). As anticipated, the desired reaction proceeded smoothly to give spiro-fused oxazoline derivative 2a in high yields but with no enantioselectivity (entries 1–2). Some previous reports have highlighted that metal-templated Brønsted acids exhibit much higher catalytic activity and stereoselectivity than their corresponding salts (Li et al., 2018). Hence, we attempted to check this possibility with Λ-(S,S)-3c; however, there was no improvement in the enantioselectivity (entry 3). Although the opposite mer isomers Δ-(S,S)-3b and Δ-(S,S)-3c were also obtained, no improvement in the enantioselectivity was observed (entries 4–5). Similarly, increasing the bulkiness of side chain R2 in the Λ-(S,S)-3d complex did not significantly enhance the enantioselectivity (entry 6). Therefore, we reasoned that a significant adjustment to the cobalt (III) complex structure would be crucial for breaking the enantioselectivity ceiling that we bumped into.
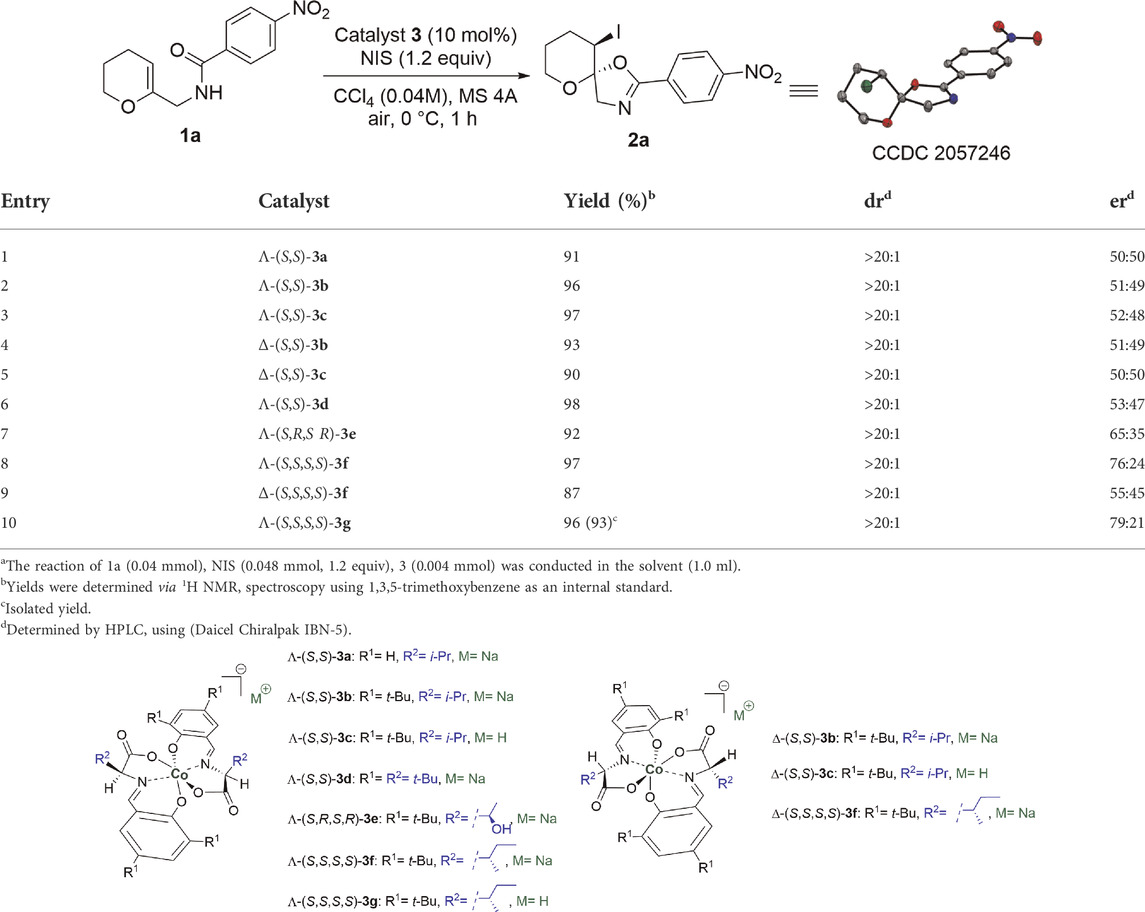
TABLE 1. Screening of Various Anionic Octahedral Salicylimine-based Cobalt (III) Complexesa.
2.1.2 Designing new catalysts
Although asymmetric induction of anionic octahedral cobalt (III) complexes has not been identified, the catalytic cavity lies near the amino acid moiety of salicylimine ligands (Cruchter and Larionov, 2018). The above analysis led us to propose that introducing an additional element of chirality in the coordinating salicylimine ligands can modify the chiral environment and improve the enantioselectivity of this iodocyclization reaction. Accordingly, we used two amino acids with chiral side chains (threonine and isoleucine) to prepare the corresponding anionic cobalt (III) complexes via a one-pot protocol. These complexes were then tested in the model reaction under the same conditions, and spiro-fused oxazoline derivative 2a was isolated in high yields and with substantially improved enantioselectivity (entries 7–8). In particular, Λ-(S,S,S,S)-3f provided 97% yield and 76:24 er. We also evaluated the opposite mer isomer Δ-(S,S,S,S)-3f, in which the enantiomeric ratio decreased to 55:45 er (entry 9). Generally, the two mer isomers (Λ and Δ) do not offer an equivalent degree of enantio-induction owing to the distortion of the chelate rings, which leads to an increase in the distance between the coordinating ligands and alters the chiral environment (Belokon et al., 2004; Belokon et al., 2009; Maleev et al., 2013). Finally, we checked the potential of the corresponding metal-templated Brønsted acid Λ-(S,S,S,S)-3g, which slightly increased the enantioselectivity to 79:21 er (entry 10). The absolute configuration of 2a was determined using X-ray crystallography (Table 1). To the best of our knowledge, this is the first report of anionic cobalt (III) complexes showing three elements of chirality (one from the stereogenic center of cobalt and two in each tridentate ligand coming from the chiral centers of amino acids). This additional element of chirality plays a critical role because of its proximity to the catalytic sites of complexes.
2.1.3 Diastereoselective complexation of octahedral cobalt complexes
As highlighted previously, the diastereoselective complexation of octahedral salicylimine-based cobalt complexes remains elusive. Although a few tridentate ligands afforded a single mer isomer exclusively upon complexation with cobalt, most of the similar ligands afforded a mixture of Λ and Δ mer isomers (Belokon et al., 2012; Larionov et al., 2015; Larionov et al., 2016; Salem et al., 2021). Yu et al. conducted a preliminary study on the effects of reaction conditions on the ratio of the two mer isomers (Jiang et al., 2017). To understand the reason for these discrepancies, we correlated our experimental observations with a preliminary computational study to further understand this phenomenon. Based on DFT calculations at the B3LYP function using the Wachters–Hay basis set for Co and D95** for other atoms in the Gaussian 16 package, we observed a variation in energy between the two mer isomers. Λ-(S,S)-3 complexes were more stable than Δ-(S,S)-3, which explains why the former were obtained as the major product in most cases. Some Λ-(S,S)-3d and Λ-(S,R,S,R)-3e complexes were obtained exclusively as pure Λ isomers because of the high energy gaps between Λ and Δ. Our computational results are also in good agreement with the experimental results obtained (Yu et al., 2015) that demonstrated that Δ-(R,R)-3 was obtained exclusively using d-amino acid-based ligands (Figure 1). Finally, our DFT calculations suggested that the bulkiness and configuration of the side chain of the amino acid (R2) are key parameters that control the yield and configuration of the major isomer (see ESI for more information).
2.1.4 Optimization of reaction conditions
After determining the optimal catalyst for this reaction, we started to optimize the conditions by screening different parameters, including catalyst loading (Table 2, entries 1–3), solvents (entries 4–11), concentrations (entries 12–15), temperature (entry 16), iodinating agents (entries 17–18), and additives (MS 3A, MS 4A) (entry 19), which showed that the reaction in CCl4 afforded a higher enantioselectivity and MS 4A was the best additive to adsorb any moisture and confirm the ultimate dryness. The higher enantioselective induction in the case of CCl4 compared to that in other solvents can be attributed to its lower dielectric constant (∼2.2); hence, CCl4 molecules do not interpose between the two ions, which is crucial for chiral anion-mediated reactions (Lacour and Moraleda, 2009). Notably, the reaction temperature and concentration exerted little effect on enantioselectivity.
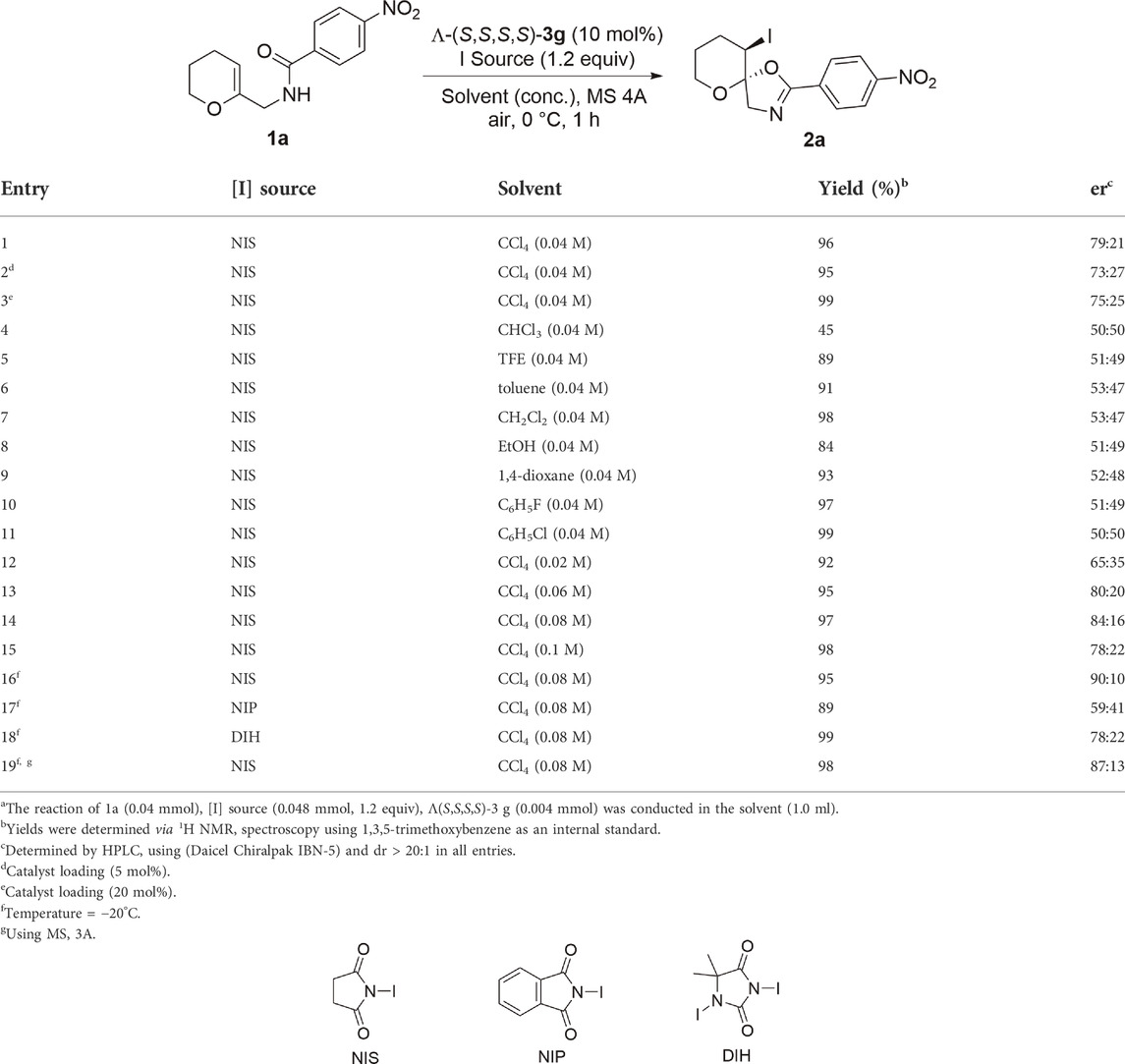
TABLE 2. Optimization of reaction conditionsa.
2.1.5 Substrate scope and limitations
With the optimized conditions in hand, we next explored the substrate scope of enantioselective iodocyclization (Table 3). Various enol ether derivatives (1) were well tolerated, affording spiro-fused oxazoline derivatives (2) in up to 99% yield and 90:10 er. Lower enantioselectivities were observed for halo-substituted spiro-fused oxazoline derivatives 2b, 2c, 2g, 2i, and 2j, because of the higher solubility of their corresponding starting materials. Highly soluble substrates are more likely to undergo fast background reactions, which, in turn, affect the enantioselectivity. This phenomenon can be further realized by comparing both the catalytic and background reactions of the two substrates that show a large variance in enantioselectivity, for example, 1a and 1c. The calculated rate constants k for the formation of 2c showed that the catalytic pathway proceeded 2.25 times faster than the background pathway (Figure 2A), while k for the formation of 2a was approximately five times faster than that of the background pathway. Hence, we can rationalize the variance in the enantioselectivities of different substrates (Figure 2B).
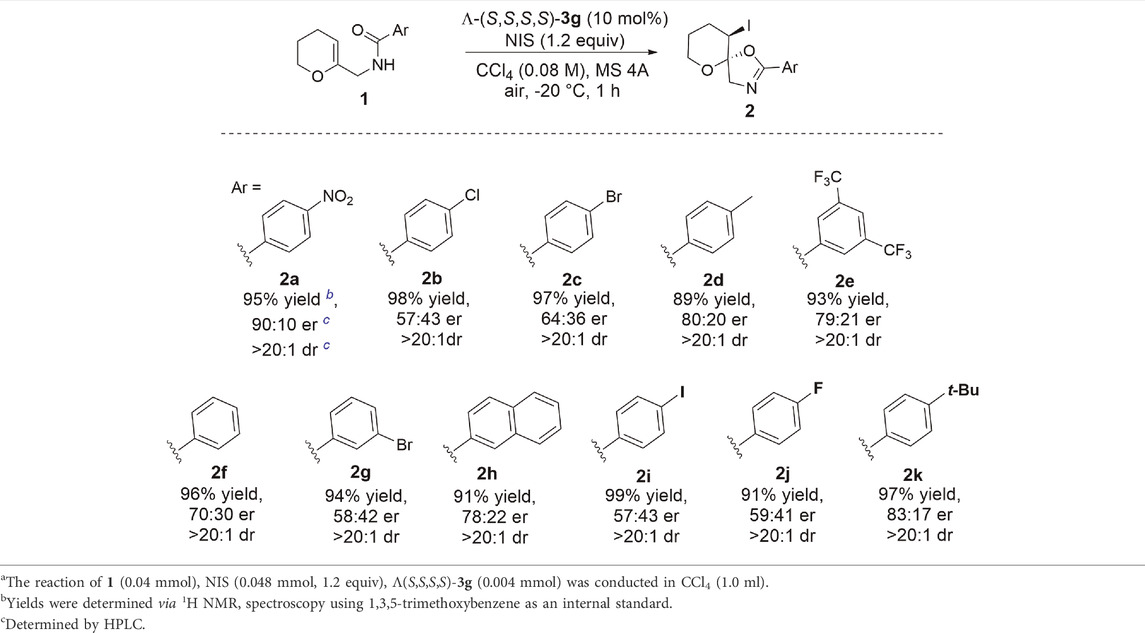
TABLE 3. Substrate scope and limitationsa.
2.1.6 Plausible reaction mechanism
Based on the kinetic study (Figure 2) and previous reports on halocyclization reactions (Marsden et al., 1994; Jiang et al., 2017; Liu W et al., 2018), a plausible mechanism was proposed (Scheme 2). The Brønsted acid [Co*]−H+ undergoes a fast exchange with the NIS to generate a lipophilic chiral iodinating agent [Co*]−I+. The generated chiral [Co*]−I+ species are highly soluble in CCl4 and thereby undergo an asymmetric iodocyclization reaction with enol ether derivative 1 to generate product 2 and regenerate the Brønsted acid [Co*]−H+ via transition states TS-II and TS-III. As shown in (Scheme 2), iodocyclization could favorably occur on the Re face in TS-II, as the Si face in TS-I might be disfavored because of the steric hindrance between the phenyl ring of substrate 1 and the sec-butyl side chain of the cobalt complex itself.
2.2 Iodoacetalization of enol ethers
To demonstrate the capacity of our new Co(III)-complex Λ-(S,S,S,S)-3g, featuring chiral ligands with two elements of chirality in each tridentate ligand, we studied another electrophilic iodination reaction of olefins, the iodoacetalization of enol ethers 4 with alcohols 5, affording 3-iodoacetal derivatives 6, which have high biological and synthetic values (Ollivier and Renaud, 2001; Haidzinskaya et al., 2015; Martinez et al., 2016). In 2018, Yu et al. conducted a primary study on this reaction using chiral Co(III)-complex-templated Brønsted acids to afford enantioenriched 3-iodoacetals in high yields and up to 83:17 er (Li et al., 2018) using only primary alcohols as substrates. Under our optimal conditions (see ESI, Funding), the scope of asymmetric intermolecular iodoacetalization with various alcohols 5 (Table 4) was explored. When primary alcohols such as substituted benzyl or naphthylmethyl were examined, 3-iodoacetal derivatives 6a–6i were isolated in up to 99% yield with 85:15 er. Electron-withdrawing substituents such as nitro groups (6c and 6e) or bromo groups (6 g–6i) on the benzyl ring improved the enantioselectivity, and the highest enantiomeric ratio of 85:15 was obtained with 4-NO2-substituted benzyl alcohol 6c. In contrast, electron-donating substituents such as methoxy groups did not have any positive impact on the enantioselectivity of product 6f. The number of electron-withdrawing substituents (e.g., two nitro groups) or their position did not show any improvement in terms of yield and enantioselectivity for derivatives 6e and 6 g–6i. Changing enol ether 4 from DHP to DHF afforded 3-iodoacetal derivative 6d in a good yield (84%); however, the enantiomeric ratio dropped to 66:34. Secondary alcohols also proved to be the optimum nucleophiles to give 3-iodoacetal derivatives 6j–6n in up to 97% yield with 90:10 er. Similarly, electron-withdrawing substituents improved the enantiomeric ratio, as shown by a comparison of derivative 6l (90:10) with 6j (83:17). Meanwhile, using rac. methyl mandelate provided two easily separable diastereomers 6n with a high enantiomeric ratio. When we attempted the reaction using both optically pure (R) and (S)-1-phenylethanol, we obtained moderate enantiomeric ratios for both, with a slight improvement in the case of (R)-6m (80:20) over (S)-6m (71:29), because its configuration is more suitable for the catalytic pocket of Λ-(S,S,S,S)-3g. Screening tertiary alcohols also afforded high yields of 3-iodoacetals 6o–6s with a high enantiomeric ratio of 92:8 er. Many functional groups were found to be compatible with our reaction system, including nitro groups (6c–6e), bromides (6g–6i), chlorides (6l), esters (6n), and dienones (6q–6s).
3 Conclusion
We introduced new anionic salicylimine-based cobalt (III) complexes with an additional element of chirality, which improved their catalytic activities toward the electrophilic iodination reactions of enol ethers. We attempted to rationalize the reasons for the discrepancies between the ratios of the mer isomers during the preparation of analogous octahedral cobalt (III) complexes. In addition, two iodination reactions of enol ethers have been studied. Iodocyclization of enol ethers 1 furnished valuable spiro-fused oxazoline derivatives 2 in high yields and good enantioselectivities up to 90:10 er. A plausible mechanism of this reaction was proposed. Asymmetric iodoacetalization of enol ethers 4 was also promoted to afford various 3-iodoacetals 6, derived from primary, secondary, and tertiary alcohols, in high yields and enantiomeric ratios of up to 92:8 er. More applications of these new anionic salicylimine-based cobalt (III) complexes 3 are currently under investigation.
Data availability statement
Additional data supporting the findings described in this manuscript are available in the Supplementary Material. The X-ray crystallographic coordinate for structures reported in this study has been deposited at the Cambridge Crystallographic Data Centre (CCDC) under deposition number CCDC-2057246 (2a). These data can be obtained free of charge from The Cambridge Crystallographic Data Centre via www.ccdc.cam.ac.uk/data_request/cif. The authors declare that all other data supporting the findings of this study are available within the article and Supplementary Material and are available from the corresponding authors upon reasonable request.
Author contributions
MSHS: Performed the experiments and DFT calculations, analyzed the data, and wrote the manuscript; ST: Supervised the project and reviewed the manuscript. All authors have read and agreed to the published version of the manuscript.
Funding
This study was funded by grants from JSPS KAKENHI Grant-in-Aid for Scientific Research (C) (No. 22K06502), Transformative Research Areas (A) 21A204 Digitalization-driven Transformative Organic Synthesis (Digi-TOS) (No. 21H05207 and 21H05217) from the Ministry of Education, Culture, Sports, Science, and Technology (MEXT), and the Japan Society for the Promotion of Science (JSPS), JST CREST (No. JPMJCR20R1), and Hoansha Foundation (Japan).
Acknowledgments
The DFT calculations were performed at the Research Center for Computational Science, Okazaki (22-IMS-C271), we acknowledge the technical staff of the Comprehensive Analysis Center of SANKEN, Osaka University (Japan).
Conflict of interest
The authors declare that the research was conducted in the absence of any commercial or financial relationships that could be construed as a potential conflict of interest.
Publisher’s note
All claims expressed in this article are solely those of the authors and do not necessarily represent those of their affiliated organizations, or those of the publisher, the editors and the reviewers. Any product that may be evaluated in this article, or claim that may be made by its manufacturer, is not guaranteed or endorsed by the publisher.
Supplementary material
The Supplementary Material for this article can be found online at: https://www.frontiersin.org/articles/10.3389/fchem.2022.1034291/full#supplementary-material
References
Avila, C. M., Patel, J. S., Reddi, Y., Saito, M., Nelson, H. M., Shunatona, H. P., et al. (2017). Enantioselective Heck–Matsuda arylations through chiral anion phase–transfer of aryl diazonium salts. Angew. Chem. Int. Ed. Engl. 56 (21), 5900–5905. doi:10.1002/ange.201702107
Banks, J. T., Button, K. M., Gossage, R. A., D. Hamilton, T., and E. Kershaw, K. (2001). Synthesis of novel oxazoline ligands designed for attachment to gold nanoparticles. Heterocycles 55 (12), 2251–2256. doi:10.3987/com-01-9335
Belokon, Y. N., Belikov, V., Vitt, S., Savel'eva, T., Burbelo, V., Bakhmutov, V. I., et al. (1977). A simple stereochemical model of pyridoxal-dependent aldolase: Asymmetric conversions of the amino acid fragment in chiral complexes of potassium a and Δ bis-/n-salicylideneaminoacidato/cobaltate (III). Tetrahedron 33 (19), 2551–2564. doi:10.1016/0040-4020(77)80079-3
Belokon, Y. N., Bulychev, A. G., Maleev, V. I., North, M., Malfanov, I. L., and Ikonnikov, N. S. (2004). Asymmetric synthesis of cyanohydrins catalysed by a potassium Δ-bis [N-salicylidene-(R)-tryptophanato] cobaltate complex. Mendeleev Commun. 14 (6), 249–250. doi:10.1070/mc2004v014n06abeh002029
Belokon, Y. N., Larionov, V., Mkrtchyan, A., Khrustalev, V., Nijland, A., Saghyan, A., et al. (2012). A novel type of catalysts for the asymmetric CC bond formation based on chiral stereochemically inert cationic Co iii complexes. Russ. Chem. Bull. 61 (12), 2252–2260. doi:10.1007/s11172-012-0320-2
Belokon, Y. N., Maleev, V. I., Kataev, D. A., Saveleva, T. F., Skrupskaya, T. V., Nelyubina, Y. V., et al. (2009). Chiral ion pairs in catalysis: Lithium salts of chiral metallocomplex anions as catalysts for asymmetric C–C bond formation. Tetrahedron Asymmetry 20 (15), 1746–1752. doi:10.1016/j.tetasy.2009.06.006
Bhandari Neupane, J., Neupane, R. P., Luo, Y., Yoshida, W. Y., Sun, R., and Williams, P. G. (2019). Characterization of leptazolines A–D, polar oxazolines from the cyanobacterium Leptolyngbya sp., reveals a glitch with the “Willoughby–Hoye” scripts for calculating NMR chemical shifts. Org. Lett. 21 (20), 8449–8453. doi:10.1021/acs.orglett.9b03216
Biswas, S., Kubota, K., Orlandi, M., Turberg, M., Miles, D. H., Sigman, M. S., et al. (2018). Enantioselective synthesis of N, S‐acetals by an oxidative pummerer‐type transformation using phase‐transfer catalysis. Angew. Chem. Int. Ed. Engl. 130 (2), 598–602. doi:10.1002/ange.201711277
Bylo, M., Farewell, R., Coppenrath, V. A., and Yogaratnam, D. (2020). A review of deflazacort for patients with Duchenne muscular dystrophy. Ann. Pharmacother. 54 (8), 788–794. doi:10.1177/1060028019900500
Carter, C., Fletcher, S., and Nelson, A. (2003). Towards phase-transfer catalysts with a chiral anion: Inducing asymmetry in the reactions of cations. Tetrahedron Asymmetry 14 (14), 1995–2004. doi:10.1016/s0957-4166(03)00367-7
Connon, R., Roche, B., Rokade, B. V., and Guiry, P. J. (2021). Further developments and applications of oxazoline-containing ligands in asymmetric catalysis. Chem. Rev. 121 (11), 6373–6521. doi:10.1021/acs.chemrev.0c00844
Cruchter, T., and Larionov, V. A. (2018). Asymmetric catalysis with octahedral stereogenic-at-metal complexes featuring chiral ligands. Coord. Chem. Rev. 376, 95–113. doi:10.1016/j.ccr.2018.08.002
Gardouh, A. R., El-Din, A. S. S., Salem, M. S. H., Moustafa, Y., and Gad, S. (2021). Starch nanoparticles for enhancement of oral bioavailability of a newly synthesized thienopyrimidine derivative with anti-proliferative activity against pancreatic cancer. Drug Des. devel. Ther. 15, 3071–3093. doi:10.2147/dddt.s321962
Ghosh, S. K., Ehnbom, A., Lewis, K. G., and Gladysz, J. A. (2017). Hydrogen bonding motifs in structurally characterized salts of the tris (ethylenediamine) cobalt trication, [Co (en) 3] 3+; an interpretive review, including implications for catalysis. Coord. Chem. Rev. 350, 30–48. doi:10.1016/j.ccr.2017.04.002
Gong, L., Chen, L. A., and Meggers, E. (2014). Asymmetric catalysis mediated by the ligand sphere of octahedral chiral‐at‐metal complexes. Angew. Chem. Int. Ed. 53 (41), 10868–10874. doi:10.1002/anie.201404305
Haidzinskaya, T., Kerchner, H. A., Liu, J., and Watson, M. P. (2015). Diastereoselective, zinc-catalyzed alkynylation of α-bromo oxocarbenium ions. Org. Lett. 17 (15), 3857–3859. doi:10.1021/acs.orglett.5b01838
Heckmann, G., and Bach, T. (2005). Synthesis of the heterocyclic core of the GE 2270 antibiotics and structure elucidation of a major degradation product. Angew. Chem. Int. Ed. 44 (8), 1199–1201. doi:10.1002/anie.200461715
Holmes, M. A., Paulsene, W., Jide, X., Ratledge, C., and Strong, R. K. (2005). Siderocalin (Lcn 2) also binds carboxymycobactins, potentially defending against mycobacterial infections through iron sequestration. Structure 13 (1), 29–41. doi:10.1016/j.str.2004.10.009
Jiang, H. J., Liu, K., Yu, J., Zhang, L., and Gong, L. Z. (2017). Switchable stereoselectivity in bromoaminocyclization of olefins: Using brønsted acids of anionic chiral cobalt (III) complexes. Angew. Chem. Int. Ed. Engl. 129 (39), 12093–12097. doi:10.1002/ange.201705066
Kaneko, S., Kumatabara, Y., and Shirakawa, S. (2016). A new generation of chiral phase-transfer catalysts. Org. Biomol. Chem. 14 (24), 5367–5376. doi:10.1039/c5ob02446c
Lacour, J., and Moraleda, D. (2009). Chiral anion-mediated asymmetric ion pairing chemistry. Chem. Commun. 2009 (46), 7073–7089. doi:10.1039/b912530b
Larionov, V. A., Feringa, B. L., and Belokon, Y. N. (2021). Enantioselective “organocatalysis in disguise” by the ligand sphere of chiral metal-templated complexes. Chem. Soc. Rev. 50, 9715–9740. doi:10.1039/d0cs00806k
Larionov, V. A., Markelova, E. P., Smol'yakov, A. F., Tat'yana, F., Maleev, V. I., and Belokon, Y. N. (2015). Chiral octahedral complexes of Co (III) as catalysts for asymmetric epoxidation of chalcones under phase transfer conditions. RSC Adv. 5 (89), 72764–72771. doi:10.1039/c5ra11760g
Larionov, V., Peregudova, S., Maleev, V., and Belokon, Y. N. (2016). A novel type of catalysts for asymmetric oxidative coupling of 2-naphthol. Russ. Chem. Bull. 65 (3), 685–688. doi:10.1007/s11172-016-1356-5
Li, N., Yu, H., Wang, R., Shen, J., Wu, W.-Q., Liu, K., et al. (2018). Enantioselective intermolecular iodoacetalization of enol ethers catalyzed by chiral Co (III)-complex-templated Brønsted acids. Tetrahedron Lett. 59 (40), 3605–3608. doi:10.1016/j.tetlet.2018.08.047
Liang, X. W., Cai, Y., and You, S. L. (2018). Asymmetric fluorinative dearomatization of tryptophol derivatives by chiral anion phase‐transfer catalysis. Chin. J. Chem. 36 (10), 925–928. doi:10.1002/cjoc.201800319
Liu, K., Jiang, H.-J., Li, N., Li, H., Wang, J., Zhang, Z.-Z., et al. (2018). Enantioselective bromocyclization of tryptamines induced by chiral Co (III)-complex-templated Brønsted acids under an air atmosphere. J. Org. Chem. 83 (12), 6815–6823. doi:10.1021/acs.joc.8b01196
Liu, W., Sahoo, B., Junge, K., and Beller, M. (2018). Cobalt complexes as an emerging class of catalysts for homogeneous hydrogenations. Acc. Chem. Res. 51 (8), 1858–1869. doi:10.1021/acs.accounts.8b00262
Long, H.-J., Li, Y.-L., Zhang, B.-Q., Xiao, W.-Y., Zhang, X.-Y., He, L., et al. (2021). Asymmetric bromoaminocyclization and desymmetrization of cyclohexa-1, 4-dienes through anion phase-transfer catalysis. Org. Lett. 23 (21), 8153–8157. doi:10.1021/acs.orglett.1c02817
Maleev, V. I., Tat’yana, V. S., Yashkina, L. V., Mkrtchyan, A. F., Saghyan, A. S., Il’in, M. M., et al. (2013). Aza-Diels–Alder reaction catalyzed by novel chiral metalocomplex Brønsted acids. Tetrahedron Asymmetry 24 (4), 178–183. doi:10.1016/j.tetasy.2013.01.011
Marsden, S. P., Depew, K. M., and Danishefsky, S. J. (1994). Stereoselective total syntheses of amauromine and 5-N-acetylardeemin. A concise route to the family of "Reverse-Prenylated" hexahydropyrroloindole alkaloids. J. Am. Chem. Soc. 116 (24), 11143–11144. doi:10.1021/ja00103a034
Martinez, L. P., Umemiya, S., Wengryniuk, S. E., and Baran, P. S. (2016). 11-Step total synthesis of pallambins C and D. J. Am. Chem. Soc. 138 (24), 7536–7539. doi:10.1021/jacs.6b04816
Ollivier, C., and Renaud, P. (2001). A novel approach for the formation of carbon-nitrogen bonds: Azidation of alkyl radicals with sulfonyl azides. J. Am. Chem. Soc. 123 (20), 4717–4727. doi:10.1021/ja004129k
Phipps, R. J., Hamilton, G. L., and Toste, F. D. (2012). The progression of chiral anions from concepts to applications in asymmetric catalysis. Nat. Chem. 4 (8), 603–614. doi:10.1038/nchem.1405
Phipps, R. J., and Toste, F. D. (2013). Chiral anion phase-transfer catalysis applied to the direct enantioselective fluorinative dearomatization of phenols. J. Am. Chem. Soc. 135 (4), 1268–1271. doi:10.1021/ja311798q
Rauniyar, V., Lackner, A. D., Hamilton, G. L., and Toste, F. D. (2011). Asymmetric electrophilic fluorination using an anionic chiral phase-transfer catalyst. Science 334 (6063), 1681–1684. doi:10.1126/science.1213918
Salem, M. S. H., Aziz, Y. M. A., Elgawish, M. S., Said, M. M., and Abouzid, K. A. (2020). Design, synthesis, biological evaluation and molecular modeling study of new thieno [2, 3-d] pyrimidines with anti-proliferative activity on pancreatic cancer cell lines. Bioorg. Chem. 94, 103472. doi:10.1016/j.bioorg.2019.103472
Salem, M. S. H., Kumar, A., Sako, M., Abe, T., Takizawa, S., and Sasai, H. (2021). Preparation of optically pure dinuclear cobalt(III) complex with Λ-configuration as a dianionic chiral catalyst. Heterocycles 103 (1), 225–230. doi:10.3987/com-20-s(k)41
Sun, T.-T., Liu, K., Zhang, S.-X., Wang, C.-R., Yao, C.-Z., and Yu, J. (2021). Enantioselective halocyclization of indole derivatives: Using 1, 3-dihalohydantoins with anionic chiral Co (III) complexes. Synlett 32 (07), 701–707. doi:10.1055/a-1310-5213
Wang, R., Wu, W.-Q., Li, N., Shen, J., Liu, K., and Yu, J. (2019). Brønsted acids of anionic chiral cobalt (III) complexes as catalysts for the iodoglycosylation or iodocarboxylation of glycals. Synlett 30 (09), 1077–1084. doi:10.1055/s-0037-1611810
Wang, Y.-M., Wu, J., Hoong, C., Rauniyar, V., and Toste, F. D. (2012). Enantioselective halocyclization using reagents tailored for chiral anion phase-transfer catalysis. J. Am. Chem. Soc. 134 (31), 12928–12931. doi:10.1021/ja305795x
Wu, X.-B., Gao, Q., Fan, J.-J., Zhao, Z.-Y., Tu, X.-Q., Cao, H.-Q., et al. (2021). Anionic chiral Co (III) complexes mediated asymmetric halocyclization-synthesis of 5-halomethyl pyrazolines and isoxazolines. Org. Lett. 23 (23), 9134–9139. doi:10.1021/acs.orglett.1c03456
Yadav, P. N., Beveridge, R. E., Blay, J., Boyd, A. R., Chojnacka, M. W., Decken, A., et al. (2011). Platinum-oxazoline complexes as anti-cancer agents: Syntheses, characterisation and initial biological studies. MedChemComm 2 (4), 274–277. doi:10.1039/c0md00211a
Yamamoto, E., Hilton, M. J., Orlandi, M., Saini, V., Toste, F. D., and Sigman, M. S. (2016). Development and analysis of a Pd (0)-catalyzed enantioselective 1, 1-diarylation of acrylates enabled by chiral anion phase transfer. J. Am. Chem. Soc. 138 (49), 15877–15880. doi:10.1021/jacs.6b11367
Yan, Z.-H., Li, D., and Yin, X.-B. (2017). Review for chiral-at-metal complexes and metal-organic framework enantiomorphs. Sci. Bull. 62 (19), 1344–1354. doi:10.1016/j.scib.2017.09.013
Yang, X., Phipps, R. J., and Toste, F. D. (2014). Asymmetric fluorination of α-Branched cyclohexanones enabled by a combination of chiral anion phase-transfer catalysis and enamine catalysis using protected amino acids. J. Am. Chem. Soc. 136 (14), 5225–5228. doi:10.1021/ja500882x
Keywords: cobalt(III) complexes, phase-transfer catalyst, iodoacetalization, iodocyclization, enantioselective synthesis, chiral-at-metal
Citation: Salem MSH and Takizawa S (2022) New anionic cobalt(III) complexes enable enantioselective synthesis of spiro-fused oxazoline and iodoacetal derivatives. Front. Chem. 10:1034291. doi: 10.3389/fchem.2022.1034291
Received: 01 September 2022; Accepted: 20 September 2022;
Published: 13 October 2022.
Edited by:
Gan B. Bajracharya, Nepal Academy of Science and Technology (NAST), NepalReviewed by:
Liangchun Li, Southwest University of Science and Technology, ChinaManabu Hatano, Kobe Pharmaceutical University, Japan
Copyright © 2022 Salem and Takizawa. This is an open-access article distributed under the terms of the Creative Commons Attribution License (CC BY). The use, distribution or reproduction in other forums is permitted, provided the original author(s) and the copyright owner(s) are credited and that the original publication in this journal is cited, in accordance with accepted academic practice. No use, distribution or reproduction is permitted which does not comply with these terms.
*Correspondence: Mohamed S. H. Salem, mohamedsalem43@sanken.osaka-u.ac.jp; Shinobu Takizawa, taki@sanken.osaka-u.ac.jp