Facile Synthesis of Water-Soluble Rhodamine-Based Polymeric Chemosensors via Schiff Base Reaction for Fe3+ Detection and Living Cell Imaging
- 1Key Laboratory of Colloid and Interface Chemistry of the Ministry of Education, School of Chemistry and Chemical Engineering, Shandong University, Jinan, China
- 2Key Laboratory of High Efficiency and Clean Mechanical Manufacture of the Ministry of Education, Center for Advanced Jet Engineering Technologies (CaJET), School of Mechanical Engineering, Shandong University, Jinan, China
Quantitative and accurate determination of iron ions play a vital role in maintaining environment and human health, but very few polymeric chemosensors were available for the detection of Fe3+ in aqueous solutions. Herein, a water-soluble rhodamine-poly (ethylene glycol) conjugate (DRF-PEG), as a dual responsive colorimetric and fluorescent polymeric sensor for Fe3+ detection with high biocompatibility, was first synthesized through Schiff base reaction between rhodamine 6G hydrazide and benzaldehyde-functionalized polyethylene glycol. As expected, the introduction of PEG segment in DRF-PEG significantly improved the water solubility of rhodamine derivatives and resulted in a good biosensing performance. The detection limit of DRF-PEG for Fe3+ in pure water is 1.00 μM as a fluorescent sensor and 3.16 μM as a colorimetric sensor at pH 6.5. The specific sensing mechanism of DRF-PEG toward Fe3+ is proposed based on the intramolecular charge transfer (ICT) mechanism, in which the O and N atoms in rhodamine moiety, together with the benzene groups from benzaldehyde-modified PEG segment, participate in coordination with Fe3+. Furthermore, DRF-PEG was applied for the ratiometric imaging of Fe3+ in HeLa cells and showed the potential for quantitative determination of Fe3+ in fetal bovine serum samples. This work provides insights for the design of water-soluble chemosensors, which can be implemented in iron-related biological sensing and clinical diagnosis.
Introduction
Iron ion is one of the most abundant transition metal ions in the human body and plays a vital role in biological metabolism (Guo et al., 2015; Liu et al., 2017; Fan et al., 2019). The deficiency in iron will lead to the lack of iron-related proteins and cause anemia, cancer, or other health problems (Park and Lee, 2020), while excess iron can inhibit the absorption of nutrients and cause irreversible damage to neurological systems and human organs, leading to serious diseases, such as Alzheimer’s disease, hepatic fibrosis or even death (Nayab and Shkir, 2017; Lin et al., 2020). The determination of Fe3+ in natural water or human body fluid is of great importance to human health and biological environment. Among the numerous detection assays, such as atomic absorption (Frankowski et al., 2010), mass spectrometry (Jia et al., 2018), Raman scattering (Song et al., 2020), and so on, optical spectroscopy methods combined with molecular sensors have attracted much attention for the characteristics of easy operation, high sensitivity, and high throughput (Cao et al., 2014; Jin et al., 2018; Jung et al., 2019). Many chemosensors for Fe3+ have been developed, but very few can be directly applied in water or biological samples due to their intrinsic hydrophobicity and cytotoxicity (Carter et al., 2014; Hou et al., 2017). Furthermore, background interference from the testing environment, such as bacteria or proteins in biosamples, may result in a significant reduction of signal-to-noise ratio and lead to detection failure (Zhang et al., 2019). Therefore, fluorescent chemosensors with excellent water solubility and biocompatibility that can be applied in complex environmental or biological systems are highly desirable.
Due to the excellent optical properties and unique switching nature of spirocyclic structures, rhodamine derivatives are widely employed for detecting metal ions (Song Y. et al., 2019; Jiao et al., 2018; Wu et al., 2018). The sensing mechanism of rhodamine sensors is based on the structural transformation from spirolactam ring to open-loop state when combined with specific ions, releasing strong fluorescent signals. Most rhodamine-derived sensors can only work in organic solvent or organic solvent-water mixtures owing to their inherent poor water solubility, which greatly inhibits their applications in practical biological and environmental systems (Zhang Y. et al., 2020; Geng et al., 2017; Choudhury et al., 2020; Shi et al., 2016). Many efforts have been devoted to inventing fluorescent sensors with good water solubility and biocompatibility, which can be achieved by introducing water-soluble carbohydrates (Chen and Fang, 2018) or hydrophilic polymer segments (Li et al., 2015; Li et al., 2016; Geng et al., 2017; Rong et al., 2017; Wu et al., 2017) into rhodamine fluorophores.
Because of the excellent biocompatibility and hydrophilicity, poly (ethylene glycol) (PEG) is commonly used in biomedical research (Liu et al., 2015). By virtue of the antifouling properties of PEG toward proteins, it is expected that the detection performance of PEG-derived polymeric sensors would be improved and the background interference from biological contamination should be reduced (Liu et al., 2015). A few water-soluble polymeric sensors obtained by combining PEG segment and rhodamine moiety have been designed for the detection of Cu2+, Hg2+, and Al3+ (Li et al., 2016; Geng et al., 2017; Li et al., 2018). However, the rhodamine-PEG-conjugated polymeric sensors, which can be applied in detecting Fe3+ in pure aqueous solutions with high biocompatibility, have not been reported. This work aims to develop a highly water-soluble rhodamine-PEG-conjugated polymeric sensor for Fe3+ and evaluate its potential applications in biological systems.
Herein, rhodamine 6G hydrazide (Rh) is selected as the fluorescent/colorimetric sensing receptor, and PEG segment is chosen as the hydrophilic segment. The polymeric sensor (DRF-PEG) containing both PEG chain and rhodamine moiety is synthesized through a facile Schiff base reaction between the amine group (−NH2) of Rh and the aldehyde group (−CHO) of di-benzaldehyde terminated poly (ethylene glycol) (DF-PEG). The rhodamine moiety of DRF-PEG serves as a sensing fluorophore in detecting metal ions, while the PEG segment improves the sensor’s water solubility and biocompatibility. DRF-PEG is highly sensitive and selective for Fe3+ in water with dual-responsive fluorescent and colorimetric response. MTT assay suggests that DRF-PEG possesses low cytotoxicity and good biocompatibility, which can be utilized for intracellular imaging. Furthermore, the detection performance of DRF-PEG for Fe3+ is evaluated in fetal bovine serum. This work provides a facial and typical model for designing polymeric sensors with high biocompatibility and water solubility, which can be applied in living cell imaging or metal ion detection in biological systems.
Experimental Section
Materials
Rhodamine 6G (95%), 1,3-dicyclohexylcarbodiimide (DCC, 99%), N,N-dimethylpyridin-4-amine (DMAP, 99%), and 4-carboxybenzaldehyde (98%) were obtained from Aladdin Chemistry Co. Ltd. (Shanghai, China). Polyethylene glycol (PEG, MW 4,000 Da), hydrazine monohydrate (50%), silver nitrate, chloride salts of K+, Na+, Mg2+, Ca2+, Cu2+, Hg2+, Co2+, Cd2+, Zn2+, Ni2+, Mn2+, Al3+, Cr3+, and Fe3+ were purchased from Sinopharm Chemical Reagent Co. Ltd. MTT (thiazolyl blue tetrazolium bromide) was obtained from Thermo Fisher Scientific (China). Ultrapure water (18.2 MΩ cm) was obtained from the Milli-Q system and applied throughout the experiment. Other solvents and chemicals were of analytical reagent grade and used without further treatment.
Instrumentation
1H NMR spectra were obtained on a Bruker Advance 400 spectrometer (Germany). FT-IR spectra were obtained on a TENSOR II FTIR routine spectrometer from Bruker (Germany) after pelleting samples with KBr. The UV-Vis absorption measurement was carried out on a Hitachi U-4100 UV/Vis/NIR spectrometer (Japan). Fluorescence spectra were performed on a FluoroMax-4 high efficiency integrated fluorescence spectrometer (Horiba. United States), and each measurement was repeated three times. The pH of the solution was measured by a Mettler Toledo SevenCompact pH meter S210. Confocal laser scanning microscopy (CLSM) images were recorded on a Leica TCS SP8 lighting confocal microscope (Germany).
Synthesis
Rhodamine 6G hydrazide was prepared through the reduction of rhodamine 6G by hydrazine monohydrate using the reported procedure (Yang et al., 2002).
Synthesis of Di-Benzaldehyde Terminated Poly (Ethylene Glycol)
DF-PEG was synthesized by following the previously reported method (Zhang et al., 2011; Yan et al., 2017). Under a nitrogen atmosphere, PEG4000 (8.0 g, 2.0 mmol) in dry THF was mixed with 4-formylbenzoic acid (0.90 g, 6.0 mmol) and DMAP (0.09 g). After complete dissolution of all the compounds by sonication, DCC (1.65 g) in 50 ml of THF was added. The mixture was thoroughly stirred at 25°C for 48 h and then filtered. Filtrates were dried through vacuum rotary evaporation and precipitated by ethyl ether three times. The obtained precipitate was dried under vacuum for 2 days to give DF-PEG as a white waxy solid. Yield: 6.4 g (75%). 1H NMR (400 MHz, CDCl3) δ: 3.40–3.90 (m, 360 H; −OCH2CH2–), 4.48–4.53 (m, 4 H; −COOCH2−), 7.95 (d, J = 8.4 Hz, 4 H; −ArH), 8.21 (d, J = 8.4 Hz, 4 H; −ArH), 10.10 (s, 2 H; −ArCHO).
Synthesis of Polymeric Sensor Containing Both PEG Chain and Rhodamine Moiety
The solution of R6G hydrazide (0.11 g, 0.25 mM) in 20 ml of acetone was slowly added into 30 ml of acetone with DF-PEG (0.53 g, 0.13 mM). After refluxed at 58°C for 8 h and cooled overnight, the mixture was then filtered. The obtained filtrate was concentrated under vacuum to give DRF-PEG as a purple solid. Yield: 1.0 g (80%). 1H NMR (400 MHz, DMSO-d6) δ: 1.20 (t, J = 7.2 Hz, 12 H; −CCH3), 1.85 (s, 12 H; −ArCH3), 3.06–3.17 (m, 8 H; −NCH2−), 3.40–3.80 (m, 360 H; −OCH2CH2−), 4.40–4.46 (m, 4 H; −COOCH2−), 5.01 (t, J = 5.2 Hz, 4 H; −NH−), 6.16 (s, 4 H; −ArH), 6.21 (s, 4 H; −ArH), 6.94–6.99 (m, 2 H; −ArH), 7.48–7.53 (m, 4 H; −ArH), 7.76–7.81 (m, 2 H; −ArH), 8.06 (d, J = 8.4 Hz, 4 H; −ArH), 8.16 (d, J = 8.4 Hz, 4 H; −ArH), 10.12 (s, 2 H; −NCH−).
Spectroscopic Study
Stock solutions of Fe3+ and other metal ions (1.0 × 10–3 mol/L) were prepared by dissolving the related inorganic salts in Milli-Q water for the fluorescence and absorption experiment. Stock solution of DRF-PEG was prepared in Milli-Q water with a concentration of 1.0 mg/ml. All the spectral experiments were conducted in an aqueous solution except for the absorption test related to Rh because of its poor water solubility. For the absorption and fluorescence selectivity experiment, DRF-PEG solution (0.1 mg/ml) was mixed with different metal ions (10–4 M). For the sensitivity experiment, DRF-PEG solution (0.1 mg/ml) was mixed with different concentrations of Fe3+. The fluorescence emission spectra of DRF-PEG were collected from 520–680 nm with an excitation wavelength of 500 nm. The quartz cuvette with a 1-cm path length was used for the absorption and emission studies. For the pH test, the pH of the tested solution was adjusted by using 0.1 mol/L of HCl, and 0.1 mol/L of NaOH solutions at 25°C.
Cytotoxicity Investigation
The cytotoxicity of DRF-PEG was evaluated by a standard MTT assay on HeLa cells (human cervical carcinoma cells) obtained from Shanghai EK-Bioscience Biotechnology Co., Ltd.
HeLa cells were seeded at a density of 1 × 104 cells/well and cultured in DMEM medium with 1% antibiotic and 10% FBS (fetal bovine serum). After cell attachment, the cells were incubated with different concentrations of DRF-PEG (0, 6.25, 12.5, 25, 50, 100, 125, 250, 500 μg/ml) and maintained at 37°C under 5% CO2 for 24 h. After washing with PBS buffer three times, fresh culture medium containing MTT (10 μl, 5 mg/ml) was added and incubated for another 4 h. Then the culture medium was removed and washed with PBS buffer. The samples were then dissolved in 150 μl of DMSO and shook for 10 min. Compared with the control sample, the cell viability was estimated through the optical density of the mixture at 450 nm.
Living Cell Imaging
HeLa cells (1 × 104 cells/well) were first cultured in DMEM medium at 37°C with 10% FBS for 24 h under 5% CO2. Then the culture medium was removed, and fresh medium containing 0.1 mg/ml DRF-PEG was added. Following incubation for 0.5 h, the samples were washed with PBS buffer to remove the residual DRF-PEG in the culture medium. Subsequently, cell culture media with different concentrations of Fe3+ (0, 5, 20, 100 μM) were added and cultured for another 30 min to allow effective uptake of Fe3+. Before imaging, the cells were rinsed with PBS buffer three times to remove the residual Fe3+ in the culture medium. CLSM images of the cells were captured at ambient temperature under an excitation wavelength of 488 nm, and the fluorescent signals were collected between 500 and 600 nm.
Determination of Iron in Fetal Bovine Serum
Fetal bovine serum obtained from Gibco (Germany) was used to investigate the potential application of DRF-PEG in biosystems. To reduce background interference, bovine serum lipids were removed with chloroform–methanol mixture before the test by referring to the reported procedure (Park and Lee, 2020). Stock solutions of diluted fetal bovine serum (10%) with 0.1 mg/ml DRF-PEG were prepared. After adding different concentrations of Fe3+ (100–1,000 μM), the fluorescent emission signals of serum samples were recorded.
Results and discussion
The rhodamine-derived polymeric sensor (DRF-PEG) was facilely synthesized through Schiff base reaction between DF-PEG and Rh with a reaction ratio of 1:2. DF-PEG was synthesized through esterification reaction among poly (ethylene glycol) and 4-carboxybenzaldehyde acid in the presence of DCC/DMAP at ambient temperature. PEG chain in DF-PEG contributes to improve the water solubility of DRF-PEG, while the terminal benzaldehyde group acts as a connecting bridge and will react with amine groups in rhodamine 6G hydrazide. One DF-PEG molecule is covalently bonded with two Rh molecules via Schiff base (imine, −N=CH−) linkages. The synthetic route of DRF-PEG is illustrated in Scheme 1. The successful preparation of DRF-PEG was confirmed by FT-IR and 1H NMR spectra (Figure 1 and Supplementary Figures S1–S3).
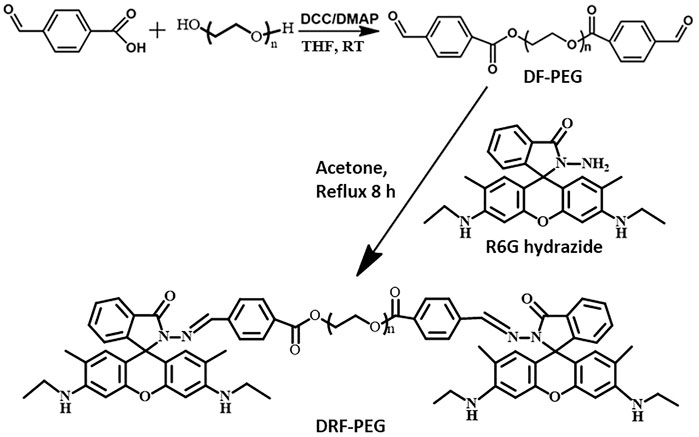
Scheme 1. Schematic illustration for the synthesis of di-benzaldehyde terminated poly (ethylene glycol) (DF-PEG) and polymeric sensor containing both PEG chain and rhodamine moiety (DRF-PEG).
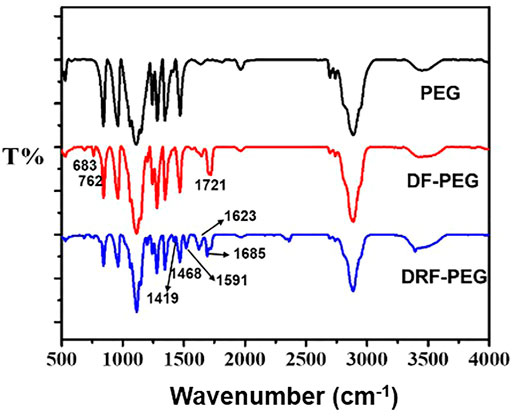
FIGURE 1. FT-IR spectra of poly (ethylene glycol (PEG), di-benzaldehyde terminated poly (ethylene glycol) (DF-PEG), and polymeric sensor containing both PEG chain and rhodamine moiety (DRF-PEG).
As shown in Figure 1 (red line), the prominent peak located at 1,721 cm−1 originated from the stretching vibration of a carbonyl (–C=O) comes from the benzaldehyde groups or the newly formed ester groups in DF-PEG. Compared with the FT-IR spectrum of PEG, newly emerged peaks below 900 cm−1 were observed in DF-PEG, which can be ascribed to the characteristic absorption of benzene rings due to the introduction of benzaldehyde groups (Zhang et al., 2011). After combination with rhodamine 6G hydrazide, DRF-PEG with two rhodamine moieties is formed, and characteristic absorption signals of rhodamine moieties (1,419, 1,468, 1,591, and 1,685 cm−1) appear on the FT-IR spectrum (blue line). The transmission band at 1,419 cm−1 is caused by the C–N deformation of rhodamine moieties, and the peak at 1,519 cm−1 can be assigned to the deformation of aromatic rings (Guo et al., 2015; Maity et al., 2018). The sharp peak at 1,623 cm−1 suggests the formation of Schiff base linkage (imine, −N=CH−) in DRF-PEG (Jia et al., 2015). The result of FT-IR and 1H NMR spectra clearly confirms the successful grafting of rhodamine moieties to DF-PEG.
Figure 2A shows the absorption spectra of Rh, DF-PEG, and DRF-PEG. Because of the poor water solubility of Rh, the absorption experiment herein was conducted in ethanol to ensure the authenticity. DRF-PEG shows two intense absorption bands at 241 and 298 nm, which can be ascribed to intramolecular π–π* and n–π* transition of anthracene moieties in rhodamine units originated from Rh. For DF-PEG, the shoulder peak around 300 nm is weaker than that of Rh and DRF-PEG because there are no anthracene structures in DF-PEG, which possess fewer benzene groups. The intense absorption of DRF-PEG from 250 to 320 nm confirms the successful grafting of rhodamine moieties to DF-PEG (Maity et al., 2018; Dewangan et al., 2019). No absorption above 500 nm is observed for all the solutions in the absence of Fe3+. However, with the addition of Fe3+, a new absorption peak centered at 532 nm is observed for Rh and DRF-PEG due to the ring opening of spirolactam structure in rhodamine moieties, resulting in the orange color of the solution (Figure 2B). This suggests that the rhodamine units from Rh are successfully conjugated onto DF-PEG, and DRF-PEG can be applied for the recognition of Fe3+.
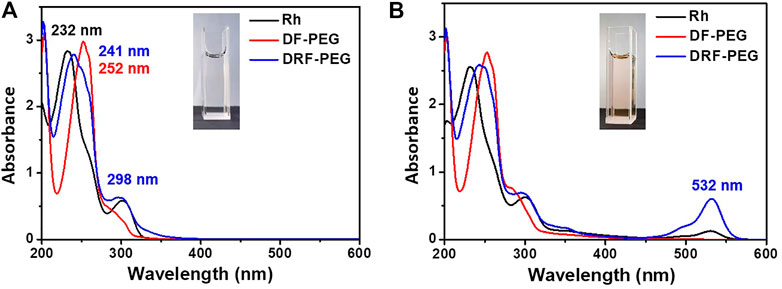
FIGURE 2. Absorption spectra of rhodamine 6G hydrazide (Rh), DF-PEG, and DRF-PEG (A) before and (B) after the addition of iron(Fe3+).
The selectivity of DRF-PEG for the detection of Fe3+ in water was investigated by both fluorescence and absorption measurements (Figure 3). After the addition of various metal ions, the spectroscopic measurements were carried out immediately, and the response process was completed instantaneously. As shown in Figures 3A,B, the fluorescence emission intensity of DRF-PEG or DRF-PEG in the presence of most monovalent and divalent metal ions (K+, Na+, Ag+, Mg2+, Ca2+, Cu2+, Co2+, Cd2+, Zn2+, Ni2+, and Mn2+) was very weak at 543 nm. The addition of Hg2+ and Cr3+, and Al3+ gave rise to a slight increase in fluorescence intensity (4.5- to 6.7-fold) at 553 nm, and a bathochromic shift was observed, whereas the presence of Fe3+ induced the most prominent fluorescence enhancement (15-fold) at 555 nm and the largest bathochromic shift (12 nm), suggesting the selective and specific recognition of DRF-PEG toward Fe3+. The affinity between Fe3+ and DRF-PEG should be the strongest, which has a relationship with the metal-ion radius and ligand configuration (Dey et al., 2017). The chelation of Fe3+ induces the structural transformation of rhodamine moiety from spirolactam ring to ring-opened form and brings about fluorescence “turn-ON” effect (Yang et al., 2002). For the selective absorption measurement (Figures 3C,D), an obvious absorption band at 532 nm emerged, and the relative intensity of 253 nm peak was enhanced for DRF-PEG in the presence of Fe3+, while for other metal ions, only the band at 253 nm and the shoulder peak at 298 nm were observed. The absorbance at 532 nm by the DRF-PEG-Fe3+ complex results in the solution color change from colorless to orange (Supplementary Figure S4). Therefore, DRF-PEG can be invoked as a sensitive colorimetric/fluorescent chemosensor for Fe3+ detection in aqueous solutions. It is worth noting that the detection of Fe3+ herein by DRF-PEG does not need the assistance of any buffer medium or organic additives, which is much desirable for practical applications.
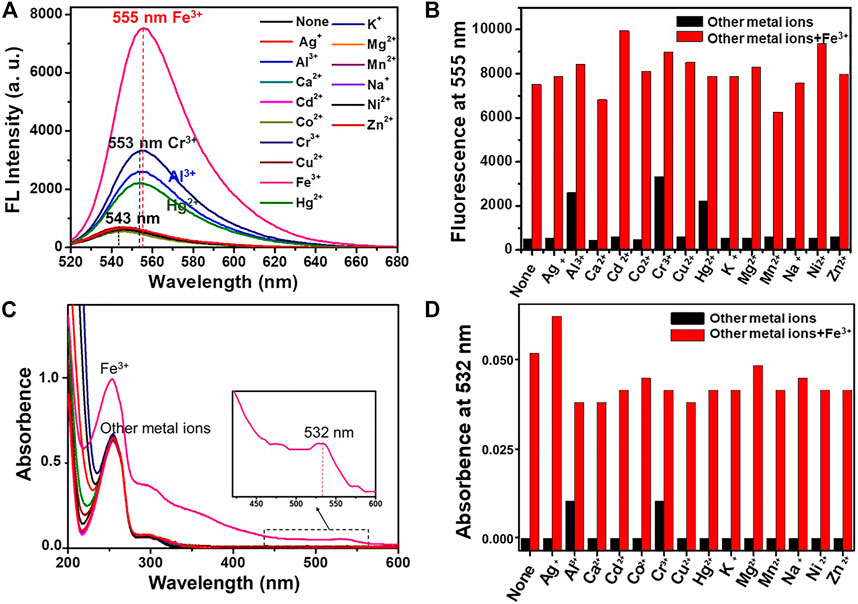
FIGURE 3. Fluorescence (A) and UV-Vis (C) spectra of DRF-PEG (0.1 mg/ml) in the presence of various metal ions (10–4 M) in water (pH 6.5). Fluorescence emission intensity at 555 nm (B) and absorption intensity at 532 nm (D) of DRF-PEG (0.1 mg/ml) with various metal ions before and after the addition of Fe3+. The black bars are the absorption/fluorescent response of DRF-PEG to various metal ions (10–4 M). The red bars are the absorption/fluorescent response after the subsequent addition of Fe3+ (10–4 M) to the above aqueous solution.
Anti-interference ability of DRF-PEG to Fe3+ is also assessed by fluorescence and UV-Vis absorption spectroscopy at pH 6.5 (Figures 3B, D). Competition experiments are carried out in the presence of Fe3+ mixed with equivalent amount of interfering metal ions (K+, Na+, Ag+, Mg2+, Ca2+, Cu2+, Co2+, Cd2+, Zn2+, Hg2+, Ni2+, Mn2+, Cr3+, and Al3+). Both the fluorescence emission and absorption enhancement of DRF-PEG in the presence of Fe3+ were not influenced by all the investigated interfering ions. These results reveal that DRF-PEG shows high selectivity and anti-interference capability for detection of Fe3+ over other metal ions. DRF-PEG can serve as a dual-responsive fluorescence turn-on and colorimetric chemosensor for detection of Fe3+ with high selectivity in pure aqueous solution. This application of DRF-PEG does not need the assistance of organic solvent, which makes the detection of Fe3+ convenient, environmental friendly, and demonstrating great potential in practical environmental and industrial monitoring.
The specific sensing mechanism of DRF-PEG toward Fe3+ can be explained by the strong coordination ability of rhodamine moieties toward Fe3+ based on our previous reports (Qiu et al., 2013; Qiu et al., 2014; Qiu et al., 2015). As shown in Figure 2, free DRF-PEG is colorless with almost no fluorescence emission. As the nitrogen atoms of Schiff base groups together with the O, N atoms of the five-membered spirolactam ring in DRF-PEG are rich in electrons, they are prone to share electrons with positive metal ions (Yuan et al., 2018). After the addition of Fe3+, DRF-PEG starts to coordinate with Fe3+, and the coordination will induce intramolecular charge transfer (ICT) in rhodamine moiety, resulting in the ring opening of spirolactam structure and strong fluorescence emission (Scheme 2). As mentioned in Figure 2, the absorption before 320 nm is ascribed to the intramolecular π–π* and n–π* transition in benzene groups. With the increase in Fe3+ concentration, the coordination interaction of benzene group and the molecular charge transfer in rhodamine moiety is enhanced. It is supposed the benzene ring from benzaldehyde modified PEG segment in DRF-PEG also participated in the coordination process, and a strong electrostatic interaction is formed between the benzene ring and Fe3+. Therefore, the absorption intensity of DRF-PEG before 320 nm increases with Fe3+ concentration, which is the reason why DRF-PEG can also act as a colorimetric sensor for Fe3+.
As shown in Figure 4, both the fluorescence intensity (555 nm) and UV-Vis absorbance (532 nm) exhibited a linear relationship with Fe3+ concentration for a wide range (0–400 μM). Based on the titration curves, the detection limit of DRF-PEG to Fe3+ can be calculated separately from the fluorescence and absorption data through the following equation (Li et al., 2014; Zhou et al., 2017):
where k is the signal-to-noise ratio (k = 3), SD is the standard deviation of blank DRF-PEG solution, and S is the slope of regression line of the titration curve (Zhou et al., 2017). From fluorescence titration data, the limit of detection for DRF-PEG as the fluorescent chemosensor toward Fe3+ in water is determined to be 1.00 μM at pH 6.5, which is much lower than the maximum US EPA limit for Fe3+ in drinking water (5.4 μM) (Nandre et al., 2014). Similarly, the DL value of DRF-PEG as a colorimetric sensor in water for Fe3+ at pH 6.5 is estimated to be 3.16 μM based on the absorbance titration results.
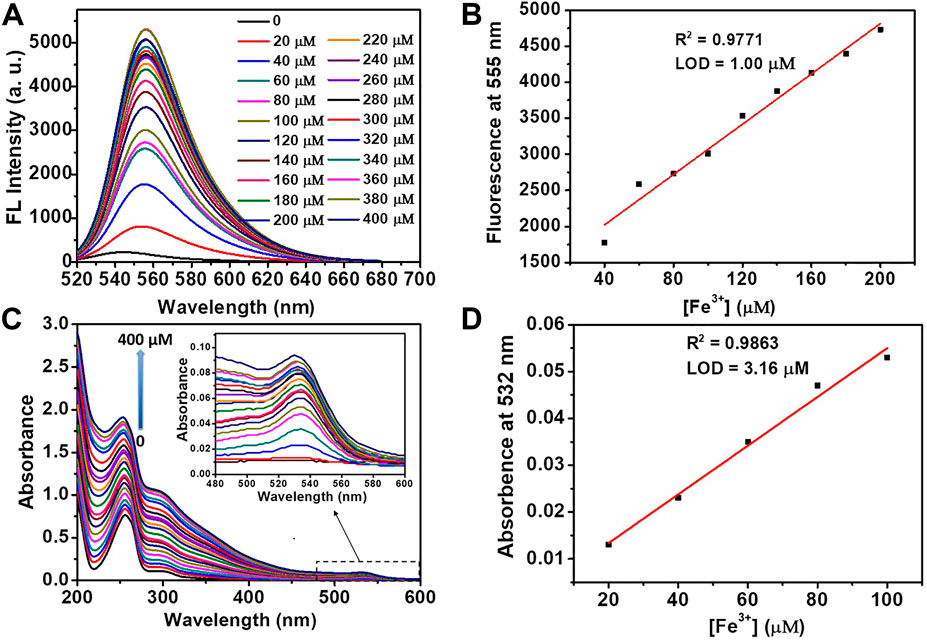
FIGURE 4. Fluorescence (A) and absorption (C) titration of DRF-PEG (0.1 mg/ml) with the addition of various concentrations of Fe3+ (0–400 μM) in water (pH 6.5). Plot of fluorescent emission intensity at 555 nm (B) and UV-Vis absorption intensity at 532 nm (D) as a function of [Fe3+].
For the efficient detection of metal ions in practical applications, sensors should possess the ability to be operated in a broad pH range. Namely, the fluorescent/absorption response of the sensor should take place and not be affected by the solution pH (Li et al., 2014; Li et al., 2015). The effect of pH on the absorption and fluorescent emission of DRF-PEG to Fe3+ is checked in the pH range of 2.0–13.3 (Figure 5). Hydrogen ions can also induce ring opening of the spirolactam structure in rhodamine moiety (Song Y. et al., 2019; Jiao et al., 2018). At pH 3–5, free DRF-PEG exhibits strong fluorescence/absorption due to the existence of abundant hydrogen ions in the solution. However, the marked increase in the fluorescence/absorption intensity after the addition of Fe3+ suggests that DRF-PEG can be applied for the recognition of Fe3+ in this pH range. It is proposed that the existence of H+ will restrain the coordination of Fe3+ with DRF-PEG. The fluorescence/absorption intensity first decreased with the increase in pH (5 < pH < 7), then increased when the amount of H+ is very few because the interference from hydrogen ions disappeared (7 < pH < 10). At pH >10, a large amount of Fe3+ would be precipitated by OH− according to the solubility product rule, so the fluorescence/absorption intensity of DRF-PEG decreases dramatically since pH 10. As shown in Figure 5, DRF-PEG can be used as a fluorescent chemosensor for Fe3+ in the pH range of 3.0–10.0 and served as a colorimetric sensor for Fe3+ in the pH range of 3.2–13.3. Therefore, DRF-PEG has great tolerance to the solution pH, suggesting the diverse applications of DRF-PEG in metal ion detection.
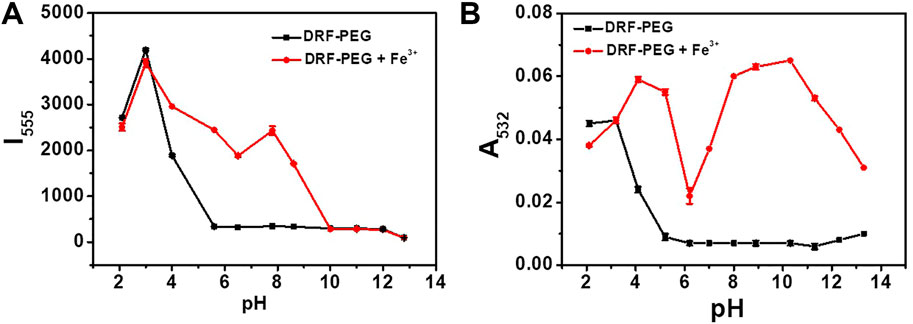
FIGURE 5. (A) Influence of pH on the fluorescent emission intensity of DRF-PEG (0.1 mg/ml) at 555 nm before and after addition of Fe3+ in water. (B) Influence of pH on the absorption intensity of DRF (0.1 mg/ml) at 532 nm before and after addition of Fe3+ ions in water.
Biocompatibility and cytotoxicity are important criteria to assess the potential application of chemical sensors in biological and medical studies (Shi et al., 2016; Dong et al., 2017). The cytotoxicity of DRF-PEG (0–500 μg/ml) to HeLa cells was evaluated through MTT assays. As shown in Figure 6, the cell viability for all the tested samples is higher than 95% even under a high concentration of DRF-PEG at 500 μg/ml after incubation for 24 h. The high cell viability of HeLa cell indicates the high biocompatibility and low cytotoxicity of DRF-PEG, suggesting high potential in living cell imaging or in vitro tests.
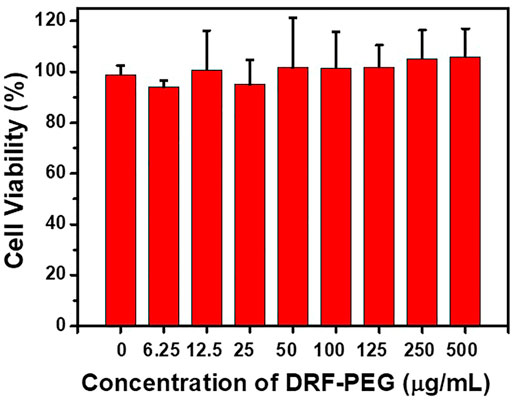
FIGURE 6. Cell viability values of human cervical carcinoma cells (HeLa) vs. the concentration of DRF-PEG. HeLa cells were incubated with different concentrations of DRF-PEG (0–500 μg/ml) for 24 h.
Encouraged by the biocompatibility and high sensing performance of DRF-PEG, intracellular detection of Fe3+ and bio-imaging capability of DRF-PEG are tested in living HeLa cells by CLSM. HeLa cells were first incubated with 0.1 mg/ml DRF-PEG for 30 min, washed by PBS buffer, and then cultured with different concentrations of Fe3+ for another 30 min. As shown in Figure 7, controlled cells without additional supplement of Fe3+ display faint fluorescence emission because of a trace amount of free iron or non-transferrin-bound ions in the culture medium, while bright and strong green fluorescence is observed for HeLa cells incubated with extra Fe3+ from 5 to 100 μM. The fluorescent signals are specifically located in the intracellular areas suggesting that DRF-PEG possesses good cell membrane permeability and can be used for the monitoring of Fe3+ in living cells without any transfection agents. Intracellular fluorescence emission intensity of HeLa cells is enhanced with the amount of Fe3+ added (Figure 7 and Supplementary Figure S5). When the concentration of Fe3+ is low (5 μM), the fluorescence is mainly concentrated in the cytoplasm area of HeLa cells. With the increase in Fe3+, intracellular uptake of Fe3+ increases, and fluorescent signals start to spread over the whole cell area. Obviously, HeLa cells incubated with 100 μM of Fe3+ exhibit the strongest fluorescent emission after the same incubation period (30 min) with bright green fluorescence in the nucleus. According to the distribution of fluorescence signals, DRF-PEG can be implemented to monitor the intracellular absorption path of Fe3+, showing enormous potential in intracellular imaging and visually monitor Fe3+ in living systems.
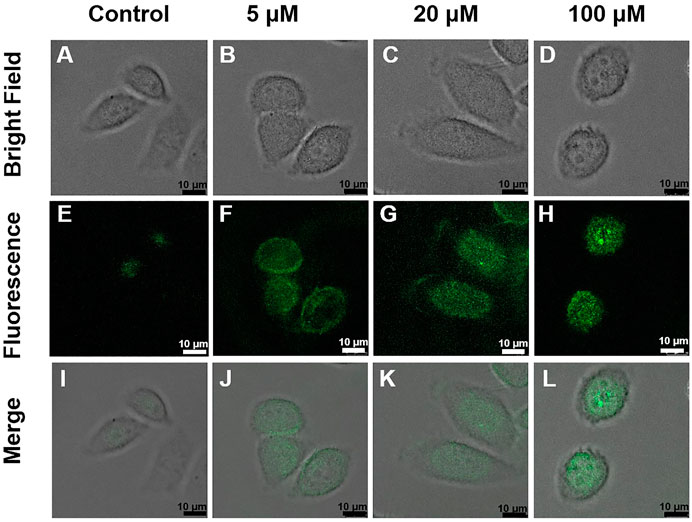
FIGURE 7. Confocal laser scanning microscopy (CLSM) images of HeLa cells treated with DRF-PEG (0.1 mg/ml) and different concentrations of Fe3+ (0, 5, 20, and 100 μM). The upper panel: bright field images, the middle: dark field, and the lower: overlays of the bright and dark field images. HeLa cells were first incubated with DRF-PEG (0.1 mg/ml) for 30 min and then with different concentrations of Fe3+ as 0 μM (A, E, and I), 5 μM (B, F, and J), 20 μM (C, G, and K), and 100 μM (D, H, and L) for another 30 min at 37°C.
As a proof of concept, the detection performance of DRF-PEG toward Fe3+ in fetal bovine serum is examined. As shown in Figure 8, the fluorescence emission intensity of DRF-PEG in 10% bovine serum shows an increasing trend with the concentration of additive Fe3+ in the range of 100–1,000 μM, and the minimum responsive concentration of Fe3+ is 100 μM. The biosensing performance of DRF-PEG toward Fe3+ can be ascribed to the anti-fouling properties of PEG chains from benzaldehyde-modified PEG segments. Results showed that the DRF-PEG possesses sensing capability toward Fe3+ in biological samples, implying its potential applications to serve as a bio-sensor in biomedical fields. In comparison with the recent rhodamine-based chemosensors for Fe3+ (Table 1), DRF-PEG shows excellent detection performance in pure aqueous solution with potential biological applications.
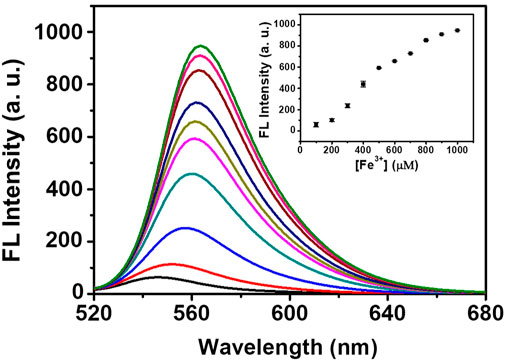
FIGURE 8. Fluorescence spectra of DRF-PEG in diluted serum (10%) with different concentrations of Fe3+ (100–1,000 μM). The inset is the fluorescence intensity as a function of [Fe3+].
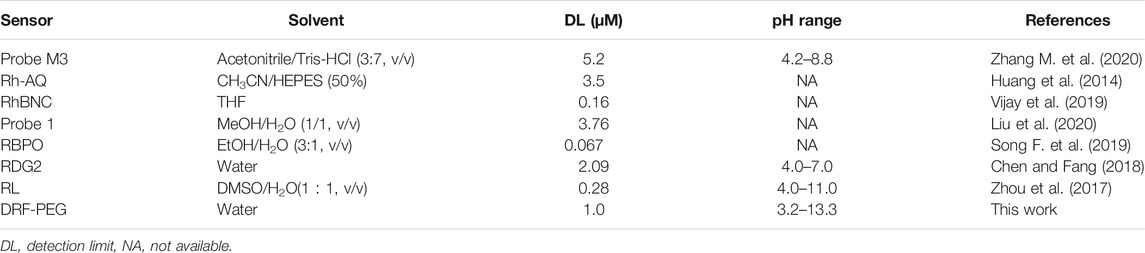
TABLE 1. Comparison of polymeric sensor containing both PEG chain and rhodamine moiety (DRF-PEG) with other rhodamine-based chemosensors for iron (Fe3+).
Conclusion
In summary, a water-soluble dual colorimetric/fluorescent responsive chemosensor (DRF-PEG) for the detection of Fe3+ with good biocompatibility was constructed by combining the specific binding effect of rhodamine moiety and the excellent water solubility of difunctionalized PEG. Based on the strong coordination of N and O atoms from rhodamine moieties and benzene groups from DF-PEG with Fe3+, DRF-PEG can be used for colorimetric/fluorescent sensing of Fe3+ in pure aqueous solutions without any aid of organic solvent or buffer medium in a wide pH range. DRF-PEG possesses excellent biocompatibility for living cell imaging and the intracellular detection of Fe3+ in HeLa cells. By virtue of the anti-fouling properties of PEG groups, DRF-PEG shows great potential in detecting iron ions in complex biological samples, which is promising in biological imaging and medical diagnosis.
Data Availability Statement
The original contributions presented in the study are included in the article/Supplementary Material, further inquiries can be directed to the corresponding author.
Author Contributions
XQ: conceptualization, methodology, investigation, data curation, and writing—original draft. JHuang: investigation, writing, reviewing, and editing. NW: investigation. KZ: investigation. JC: resources, writing, reviewing, and editing. JHao: supervision, writing, reviewing, and editing.
Funding
This work was supported by the China Postdoctoral Science Foundation (2019M662326), Fundamental Research Funds of Shandong University (2019GN013), and the Natural Science Foundation of Shandong Province (ZR2018ZA0547 and ZR2020YQ38).
Conflict of Interest
The authors declare that the research was conducted in the absence of any commercial or financial relationships that could be construed as a potential conflict of interest.
Publisher’s Note
All claims expressed in this article are solely those of the authors and do not necessarily represent those of their affiliated organizations, or those of the publisher, the editors, and the reviewers. Any product that may be evaluated in this article, or claim that may be made by its manufacturer, is not guaranteed nor endorsed by the publisher.
Supplementary Material
The Supplementary Material for this article can be found online at: https://www.frontiersin.org/articles/10.3389/fchem.2022.845627/full#supplementary-material
References
Cao, J., Ding, L., Hu, W., Chen, X., Chen, X., and Fang, Y. (2014). Ternary System Based on Fluorophore-Surfactant Assemblies-Cu2+ for Highly Sensitive and Selective Detection of Arginine in Aqueous Solution. Langmuir 30 (50), 15364–15372. doi:10.1021/la5039798
Carter, K. P., Young, A. M., and Palmer, A. E. (2014). Fluorescent Sensors for Measuring Metal Ions in Living Systems. Chem. Rev. 114 (8), 4564–4601. doi:10.1021/cr400546e
Chen, Q., and Fang, Z. (2018). Two Sugar-Rhodamine "Turn-On" Fluorescent Probes for the Selective Detection of Fe3+. Spectrochim. Acta A: Mol. Biomol. Spectrosc. 193, 226–234. doi:10.1016/j.saa.2017.12.023
Choudhury, N., Ruidas, B., Mukhopadhyay, C. D., and De, P. (2020). Rhodamine-Appended Polymeric Probe: An Efficient Colorimetric and Fluorometric Sensing Platform for Hg2+ in Aqueous Medium and Living Cells. ACS Appl. Polym. Mater. 2, 5077–5085. doi:10.1021/acsapm.0c00878
Dewangan, S., Barik, T., Parida, R., Mawatwal, S., Dhiman, R., Giri, S., et al. (2019). Solvent Free Synthesis of Ferrocene Based Rhodamine - Hydrazone Molecular Probe with Improved Bioaccumulation for Sensing and Imaging Applications. J. Organomet. Chem. 904, 120999. doi:10.1016/j.jorganchem.2019.120999
Dey, S., Sarkar, S., Maity, D., and Roy, P. (2017). Rhodamine Based Chemosensor for Trivalent Cations: Synthesis, Spectral Properties, Secondary Complex as Sensor for Arsenate and Molecular Logic gates. Sens. Actuators B: Chem. 246, 518–534. doi:10.1016/j.snb.2017.02.094
Dong, J. X., Wang, Z. L., Yang, Y., Gao, Z. F., Li, B. L., Jiang, H. H., et al. (2017). Bio-friendly Maillard Reaction Fluorescent Products from Glutathione and Ascorbic Acid for the Rapid and Label-free Detection of Fe3+ in Living Cells. J. Mater. Chem. B 5 (4), 707–713. doi:10.1039/c6tb02449a
Fan, K., Wang, X., Yu, S., Han, G., Xu, D., Zhou, L., et al. (2019). A Chitosan-Based Fluorescent Hydrogel for Selective Detection of Fe2+ Ions in Gel-To-Sol Mode and Turn-Off Fluorescence Mode. Polym. Chem. 10 (37), 5037–5043. doi:10.1039/c9py01179j
Frankowski, M., Zioła-Frankowska, A., and Siepak, J. (2010). New Method for Speciation Analysis of Aluminium Fluoride Complexes by HPLC-FAAS Hyphenated Technique. Talanta 80 (5), 2120–2126. doi:10.1016/j.talanta.2009.11.018
Geng, T.-M., Zhang, W.-Y., Li, D.-K., Xia, H.-Y., Wang, Y., Wang, Z.-Q., et al. (2017). The Chromogenic and Fluorescent Sensing Properties for a Water Soluble Polymeric Chemosensor Bearing Rhodamine Ethanediamine Moieties with Oxethyl (OCH 2 CH 2 ) as a Spacer. J. Environ. Chem. Eng. 5 (1), 906–914. doi:10.1016/j.jece.2017.01.017
Guo, R., Zhou, S., Li, Y., Li, X., Fan, L., and Voelcker, N. H. (2015). Rhodamine-Functionalized Graphene Quantum Dots for Detection of Fe3+ in Cancer Stem Cells. ACS Appl. Mater. Inter. 7 (43), 23958–23966. doi:10.1021/acsami.5b06523
Hou, J.-T., Ren, W. X., Li, K., Seo, J., Sharma, A., Yu, X.-Q., et al. (2017). Fluorescent Bioimaging of pH: from Design to Applications. Chem. Soc. Rev. 46 (8), 2076–2090. doi:10.1039/c6cs00719h
Huang, J., Xu, Y., and Qian, X. (2014). Rhodamine-based Fluorescent Off-On Sensor for Fe3+ - in Aqueous Solution and in Living Cells: 8-aminoquinoline Receptor and 2 : 1 Binding. Dalton Trans. 43 (16), 5983–5989. doi:10.1039/c3dt53159g
Jia, H., Li, Z., Wang, X., and Zheng, Z. (2015). Facile Functionalization of a Tetrahedron-like PEG Macromonomer-Based Fluorescent Hydrogel with High Strength and its Heavy Metal Ion Detection. J. Mater. Chem. A. 3 (3), 1158–1163. doi:10.1039/c4ta05736h
Jia, X., Gong, D., Zhao, J., Ren, H., Wang, J., and Zhang, X. (2018). Zwitterion-functionalized Polymer Microspheres as a Sorbent for Solid Phase Extraction of Trace Levels of V(V), Cr(III), As(III), Sn(IV), Sb(III) and Hg(II) Prior to Their Determination by ICP-MS. Microchim. Acta 185 (4), 228. doi:10.1007/s00604-018-2766-x
Jiao, Y., Zhou, L., He, H., Yin, J., Gao, Q., Wei, J., et al. (2018). A Novel Rhodamine B-Based "Off-On'' Fluorescent Sensor for Selective Recognition of Copper (II) Ions. Talanta 184, 143–148. doi:10.1016/j.talanta.2018.01.073
Jin, Q., Chen, S., Jiang, H., Wang, Y., Zhang, L., and Liu, M. (2018). Self-Assembly of Amphiphilic Schiff Base and Selectively Turn on Circularly Polarized Luminescence by Al3+. Langmuir 34 (47), 14402–14409. doi:10.1021/acs.langmuir.8b03019
Jung, Y., Ju, I. G., Choe, Y. H., Kim, Y., Park, S., Hyun, Y.-M., et al. (2019). Hydrazine Exposé: The Next-Generation Fluorescent Probe. ACS Sens. 4 (2), 441–449. doi:10.1021/acssensors.8b01429
Li, C.-Y., Zou, C.-X., Li, Y.-F., Tang, J.-L., and Weng, C. (2014). A New Rhodamine-Based Fluorescent Chemosensor for Fe3+ and its Application in Living Cell Imaging. Dyes Pigm. 104, 110–115. doi:10.1016/j.dyepig.2014.01.003
Li, G., Tao, F., Wang, H., Li, Y., and Wang, L. (2015). A Novel Reversible Colorimetric Chemosensor for Rapid Naked-Eye Detection of Cu2+ in Pure Aqueous Solution. Sens. Actuators B: Chem. 211, 325–331. doi:10.1016/j.snb.2015.01.105
Li, G., Tao, F., Liu, Q., Wang, L., Wei, Z., Zhu, F., et al. (2016). A Highly Selective and Reversible Water-Soluble Polymer Based-Colorimetric Chemosensor for Rapid Detection of Cu2+ in Pure Aqueous Solution. New J. Chem. 40 (5), 4513–4518. doi:10.1039/c5nj03526k
Li, G., Bai, L., Tao, F., Deng, A., and Wang, L. (2018). A Dual Chemosensor for Cu2+ and Hg2+ Based on a Rhodamine-Terminated Water-Soluble Polymer in 100% Aqueous Solution. Analyst 143 (22), 5395–5403. doi:10.1039/c8an01130c
Lin, M.-H., Ren, X.-X., Ning, X.-M., Liu, D.-Y., and Qian, J. (2020). Improving Ion Selectivity of 1,4,7-Triazacyclononane-Based Receptor by Zinc Coordination: "Turn-On" Chemosensor for Br- and Fe3+ Ions. Langmuir 36 (44), 13218–13226. doi:10.1021/acs.langmuir.0c02072
Liu, X., Miller II, A. L., Yaszemski, M. J., and Lu, L. (2015). Biodegradable and Crosslinkable PPF-PLGA-PEG Self-Assembled Nanoparticles Dual-Decorated with Folic Acid Ligands and Rhodamine B Fluorescent Probes for Targeted Cancer Imaging. RSC Adv. 5 (42), 33275–33282. doi:10.1039/c5ra04096e
Liu, Z., Purro, M., Qiao, J., and Xiong, M. P. (2017). Multifunctional Polymeric Micelles for Combining Chelation and Detection of Iron in Living Cells. Adv. Healthc. Mater. 6 (17), 1700162. doi:10.1002/adhm.201700162
Liu, Y., Zhao, C., Zhao, X., Liu, H., Wang, Y., Du, Y., et al. (2020). A Selective N,N-dithenoyl-rhodamine Based Fluorescent Probe for Fe3+ Detection in Aqueous and Living Cells. J. Environ. Sci. 90, 180–188. doi:10.1016/j.jes.2019.12.005
Maity, S., Parshi, N., Prodhan, C., Chaudhuri, K., and Ganguly, J. (2018). Characterization of a Fluorescent Hydrogel Synthesized Using Chitosan, Polyvinyl Alcohol and 9-anthraldehyde for the Selective Detection and Discrimination of Trace Fe3+ and Fe2+ in Water for Live-Cell Imaging. Carbohydr. Polym. 193, 119–128. doi:10.1016/j.carbpol.2018.03.073
Nandre, J., Patil, S., Patil, V., Yu, F., Chen, L., Sahoo, S., et al. (2014). A Novel Fluorescent "Turn-On" Chemosensor for Nanomolar Detection of Fe(III) from Aqueous Solution and its Application in Living Cells Imaging. Biosens. Bioelectron. 61, 612–617. doi:10.1016/j.bios.2014.06.017
Nayab, P. S., and Shkir, M. (2017). A Dual Responsive Colorimetric and Fluorescent Reversible Turn-On Chemosensor for Iron (Fe3+): Computational and Spectroscopic Investigations. Sens. Actuators B: Chem. 245, 395–405. doi:10.1016/j.snb.2017.01.072
Park, T. E., and Lee, S. H. (2020). A Micellized Fluorescence Sensor Based on Amplified Quenching for Highly Sensitive Detection of Non-transferrin-bound Iron in Serum. Dalton Trans. 49 (15), 4660–4664. doi:10.1039/d0dt00026d
Qiu, X., Han, S., and Gao, M. (2013). Highly Sensitive and Selective Detection of Cu(ii) by Periodic Mesoporous Rhodamine Derivative-Based Organosilicas with crystal-like Pore walls. J. Mater. Chem. A. 1 (4), 1319–1325. doi:10.1039/c2ta00411a
Qiu, X., Han, S., Hu, Y., Gao, M., and Wang, H. (2014). Periodic Mesoporous Organosilicas for Ultra-high Selective Copper(ii) Detection and Sensing Mechanism. J. Mater. Chem. A. 2 (5), 1493–1501. doi:10.1039/c3ta14314g
Qiu, X., Han, S., Hu, Y., and Sun, B. (2015). Ratiometric Fluorescent Nanosensors for Copper(II) Based on Bis(rhodamine)-Derived PMOs with J-type Aggregates. Chem. Eur. J. 21 (10), 4126–4132. doi:10.1002/chem.201406143
Rong, G., Corrie, S. R., and Clark, H. A. (2017). In Vivo biosensing: Progress and Perspectives. ACS Sens. 2 (3), 327–338. doi:10.1021/acssensors.6b00834
Shi, B., Su, Y., Zhang, L., Huang, M., Liu, R., and Zhao, S. (2016). Nitrogen and Phosphorus Co-doped Carbon Nanodots as a Novel Fluorescent Probe for Highly Sensitive Detection of Fe3+ in Human Serum and Living Cells. ACS Appl. Mater. Inter. 8 (17), 10717–10725. doi:10.1021/acsami.6b01325
Song, Y., Ma, Z., Fang, H., Zhang, Q., Zhou, Q., Chen, Z., et al. (2020). Au Sputtered Paper Chromatography Tandem Raman Platform for Sensitive Detection of Heavy Metal Ions. ACS Sens. 5 (5), 1455–1464. doi:10.1021/acssensors.0c00395
Song, F., Yang, C., Liu, H., Gao, Z., Zhu, J., Bao, X., et al. (2019). Dual-binding Pyridine and Rhodamine B Conjugate Derivatives as Fluorescent Chemosensors for Ferric Ions in Aqueous media and Living Cells. Analyst 144 (9), 3094–3102. doi:10.1039/c8an01915k
Song, Y., Zheng, Y., Zhang, S., Song, Y., Niu, M., Li, Y., et al. (2019). Always-on and Water-Soluble Rhodamine Amide Designed by Positive Charge Effect and Application in Mitochondrion-Targetable Imaging of Living Cells. Sens. Actuators B: Chem. 286, 32–38. doi:10.1016/j.snb.2019.01.107
Vijay, N., Wu, S. P., and Velmathi, S. (2019). Turn on Fluorescent Chemosensor Containing Rhodamine B Fluorophore for Selective Sensing and In Vivo Fluorescent Imaging of Fe3+ Ions in HeLa Cell Line and Zebrafish. J. Photochem. Photobiol. A: Chem. 384, 112060. doi:10.1016/j.jphotochem.2019.112060
Wu, W., Chen, A., Tong, L., Qing, Z., Langone, K. P., Bernier, W. E., et al. (2017). Facile Synthesis of Fluorescent Conjugated Polyelectrolytes Using Polydentate Sulfonate as Highly Selective and Sensitive Copper(II) Sensors. ACS Sens. 2 (9), 1337–1344. doi:10.1021/acssensors.7b00400
Wu, M., Suo, F., Zhou, J., Gong, Q., Bai, L., Chen, B., et al. (2018). Paper-based Fluorogenic Device for Detection of Copper Ions in a Biological System. ACS Appl. Bio Mater. 1, 1523–1529. doi:10.1021/acsabm.8b00435
Yan, B., Huang, J., Han, L., Gong, L., Li, L., Israelachvili, J. N., et al. (2017). Duplicating Dynamic Strain-Stiffening Behavior and Nanomechanics of Biological Tissues in a Synthetic Self-Healing Flexible Network Hydrogel. ACS Nano 11 (11), 11074–11081. doi:10.1021/acsnano.7b05109
Yang, X.-F., Guo, X.-Q., and Zhao, Y.-B. (2002). Development of a Novel Rhodamine-type Fluorescent Probe to Determine Peroxynitrite. Talanta 57 (5), 883–890. doi:10.1016/s0039-9140(02)00120-0
Yuan, J., Wu, S.-Q., Liu, M.-J., Sato, O., and Kou, H.-Z. (2018). Rhodamine 6G-Labeled Pyridyl Aroylhydrazone Fe(II) Complex Exhibiting Synergetic Spin Crossover and Fluorescence. J. Am. Chem. Soc. 140 (30), 9426–9433. doi:10.1021/jacs.8b00103
Zhang, Y., Tao, L., Li, S., and Wei, Y. (2011). Synthesis of Multiresponsive and Dynamic Chitosan-Based Hydrogels for Controlled Release of Bioactive Molecules. Biomacromolecules 12 (8), 2894–2901. doi:10.1021/bm200423f
Zhang, D., Yao, Y., Wu, J., Protsak, I., Lu, W., He, X., et al. (2019). Super Hydrophilic Semi-IPN Fluorescent Poly(N-(2-hydroxyethyl)acrylamide) Hydrogel for Ultrafast, Selective, and Long-Term Effective Mercury(II) Detection in a Bacteria-Laden System. ACS Appl. Bio Mater. 2 (2), 906–915. doi:10.1021/acsabm.8b00761
Zhang, M., Shen, C., Jia, T., Qiu, J., Zhu, H., and Gao, Y. (2020). One-step Synthesis of Rhodamine-Based Fe3+ Fluorescent Probes via Mannich Reaction and its Application in Living Cell Imaging. Spectrochim. Acta Part A: Mol. Biomol. Spectrosc. 231, 118105. doi:10.1016/j.saa.2020.118105
Zhang, Y., Gutiérrez, M., Chaudhari, A. K., and Tan, J.-C. (2020). Dye-Encapsulated Zeolitic Imidazolate Framework (ZIF-71) for Fluorochromic Sensing of Pressure, Temperature, and Volatile Solvents. ACS Appl. Mater. Inter. 12, 37477–37488. doi:10.1021/acsami.0c10257
Zhou, F., Leng, T.-H., Liu, Y.-J., Wang, C.-Y., Shi, P., and Zhu, W.-H. (2017). Water-soluble Rhodamine-Based Chemosensor for Fe 3+ with High Sensitivity, Selectivity and Anti-interference Capacity and its Imaging Application in Living Cells. Dyes Pigm. 142, 429–436. doi:10.1016/j.dyepig.2017.03.057
Keywords: Fe3+ detection, fluorescence probe, rhodamine, Schiff base, cell imaging
Citation: Qiu X, Huang J, Wang N, Zhao K, Cui J and Hao J (2022) Facile Synthesis of Water-Soluble Rhodamine-Based Polymeric Chemosensors via Schiff Base Reaction for Fe3+ Detection and Living Cell Imaging. Front. Chem. 10:845627. doi: 10.3389/fchem.2022.845627
Received: 30 December 2021; Accepted: 20 January 2022;
Published: 28 February 2022.
Edited by:
Dandan Guo, Upstate Medical University, United StatesCopyright © 2022 Qiu, Huang, Wang, Zhao, Cui and Hao. This is an open-access article distributed under the terms of the Creative Commons Attribution License (CC BY). The use, distribution or reproduction in other forums is permitted, provided the original author(s) and the copyright owner(s) are credited and that the original publication in this journal is cited, in accordance with accepted academic practice. No use, distribution or reproduction is permitted which does not comply with these terms.
*Correspondence: Jingcheng Hao, jhao@sdu.edu.cn