High-pressure high-temperature synthesis of NdRe2
- 1Department of Physics, Florida International University, Miami, FL, United States
- 2Department of Chemistry and Biochemistry, Florida International University, Miami, FL, United States
- 3Department of Mechanical and Materials Engineering, Florida International University, Miami, FL, United States
- 4Applied Research Center, Florida International University, Miami, FL, United States
- 5Center for Advanced Radiation Sources, The University of Chicago, Chicago, IL, United States
In this study, we report the synthesis of a new cubic neodymium-rhenium metallic alloy NdRe2 through the utilization of high pressure and laser heating in a diamond anvil cell. NdRe2 crystallizes in the
1 Introduction
The management of nuclear waste is a complex and multifaceted issue that requires meticulous attention to safety for long-term sustainability. Nuclear energy produces sizeable quantities of nuclear waste (World Nuclear Association, 2021; U.S. Energy Information Administration, 2022), which can impose danger if not properly contained. In this context, materials science plays a vital role in developing innovative solutions for the safe storage and study of nuclear waste (Beierschmitt et al., 2017).
Rare-earth (RE) compounds, a class of materials with unique properties, play a pivotal role in forming substances to solve the nuclear waste challenges the country faces (Chong et al., 2022). Depending on the level of waste, the primary types of materials used include glass, ceramics, cement, and various composites (Hyatt and Ojovan, 2019; Rafiuddin et al., 2022). Stored nuclear waste holds varying levels of lanthanides and actinides that can form unknown compounds depending on their environment. As actinides exhibit high radioactivity, studying their compounds can pose safety risks that prevent a thorough analysis. Lanthanides offer a safer alternative. As both are in the f-block, actinides can be modeled by their lanthanide counterpart to safely study potential formations occurring in stored waste.
Among the 4f elements, or the lanthanides, neodymium has been of particular interest due to its remarkable magnetic properties (Behrendt et al., 1957), ductility (Wu et al., 2013), and its influence as a dopant in various compounds (Roy et al., 2012; Butenkov et al., 2023). More notably, neodymium is commonly known for its involvement in the permanent magnet Nd2Fe14B (Boller and Oesterreicher, 1984) and in the lasing medium of an Nd:YAG laser. Alongside its popular uses, neodymium can be used to substitute the actinide uranium. Since the two hold the same number of electrons within the outermost shell, they may exhibit similar bonding formations in their respective compounds. In addition, rhenium can be used to study compounds formed between technetium and actinides during containment. Technetium, a long-lived radioactive element produced during nuclear fission, is specifically targeted for immobilization due to its radioactive nature and potential environmental impact (Hartmann et al., 2011). Technetium shares similar chemistry to rhenium (Darab and Smith, 1996), allowing rhenium to model technetium during experiments. As uranium and technetium are radioactive, direct studies prove to be challenging due to the inherent safety risks. Using similar nonradioactive elements allows us to mimic the radioactive compounds found in nuclear waste for further study.
Laves phase compounds have previously been of immense interest due to their potential applications, such as usage in superalloys and high-temperature steels (Stein and Leineweber, 2021). A large portion of known Laves phase compounds hold an RE element (Villars and Calvert, 1991), and while many are known, there may be an abundance of unknown compounds. Within the neodymium rhenium system, only the hexagonal NdRe2 (space group P63/mmc) (Elliott, 1964) has been previously synthesized. This hexagonal structure is common among the lanthanides (excluding La, Ce, Pm) (Elliott, 1964; Savitskii and Khamidov, 1965) and some actinides (Th, U, Np, Pu) (Giorgi and Szklarz, 1970; Lam and Mitchell, 1972; Haines et al., 1976). It is also seen in several RE-Tc (Darby et al., 1962; Darby et al., 1964; Szklarz and Giorgi, 1981) and actinide-Tc (Darby et al., 1965) compounds. RE-Re alloys have been previously synthesized through arc-melting and liquid-phase sintering (Elliott, 1964). While useful, these methods often have difficulties in providing pure crystalline phases. High-pressure high-temperature (HPHT) synthesis could be a great alternative. Compared to other methods, HPHT synthesis allows for greater control of sample composition and enables us to obtain metastable materials. In addition, its ease of use and compatibility with X-ray diffraction and spectroscopy tools encourage extensive exploration of material behavior under extreme conditions.
Multiple high-pressure studies were conducted on various compounds holding RE and transition metal elements. This includes the HPHT synthesis of YbZn2 (Salamatin et al., 2023) and REFe2 (RE = Pr, Nd, Yb) (Cannon et al., 1972), the pressure-induced structural transitions in R1-xNi2 (R = Y, Sm, Gd, Tb) (Gratz et al., 1996), and the pressure-induced disordering of vacancies in RNi2 (R = Y, Sm, Gd, Tb) (Lindbaum et al., 2002). While useful, detailed studies on the HPHT synthesis of RE rhenium alloys have been scarce; therefore, it is essential to determine how the system behaves and develops at elevated conditions.
In this work, we used the advantages provided by single-crystal high-pressure crystallography (Bykova, 2014; Bykova et al., 2015) to synthesize NdRe2 for the first time and determine its crystal structure at 24 (1) GPa and 2,200 (100) K. In situ X-ray diffraction (XRD) was used in a laser-heated diamond anvil cell (DAC) to characterize the synthesized single-crystal compound.
2 Materials and methods
2.1 Diamond anvil cell experiment
A mini BX80-type DAC (DACTools, 2012) with diamonds with culet diameters of 100 µm was used in high-pressure experiments. Rhenium gaskets were squeezed between the diamonds to create an indentation with a thickness of 19 µm. A round hole of 52 µm in diameter was then drilled in the center of the indentation. A preselected single crystal of γ-B of size 8 µm and a piece of Nd (commercially purchased from Fischer Scientific, 99.9% purity) of size 10 µm were placed next to each other in the center of the hole. Neon served as the pressure transmitting medium and was loaded at 13 IDD at the Advanced Photon Source (APS) at Argonne National Lab (ANL) (Rivers et al., 2008; Sutton et al., 2022). Alongside the sample, a 3 µm Au piece and a 10 µm ruby ball (DACTools, LLC) were placed near the sample and used as pressure markers. Using ruby fluorescence (Dewaele et al., 2008) and the XRD of Au and He (Fei et al., 2007), pressure values were determined.
2.2 Single-crystal X-Ray diffraction
In situ XRD measurements were performed at the 13ID-D beamline of GSECARS at APS, ANL using a wavelength λ = 0.2952 Å (42 keV), and the beam size was 3.8 × 2.3 µm2. The diffraction data were collected using the Dectris Pilatus3 X CdTe 1M pixel array detector. During the measurement, 140 frames were collected in the scanning range of ± 35° with a step of 0.5° and the exposure time set to 1 s per step. Using the double-sided laser-heating setup at the 13ID-D beamline, the sample was heated to 2,200 (100) K. The laser was set to burst mode with a heating duration of one or 3 s. XRD measurements were taken before, during, and after laser heating. After each laser heating period, the sample was mapped around the heated spot to identify if the reaction occurred. Then single-crystal data were collected in a few spots for further analysis of the phase composition. Initially, pressure in the DAC was increased to 8.0 (5) GPa, but XRD data showed that the sample did not crystallize sufficiently and was not optimal for single-crystal analysis. To overcome this issue, the pressure was further increased to 24 (1) GPa, and after laser heating, we observed a notable reaction.
CrysAlisPro (Rigaku Oxford Diffraction, 2019) was used to perform data reduction and determine the lattice parameters, which can be found in Table 1. Structure solution and refinement were done using JANA 2006 (Petříček et al., 2014) implemented with SHELXT (Sheldrick, 2015b) and SHELXL (Sheldrick, 2015a). DAFi (Aslandukov et al., 2022) was used to search for and identify single-crystal domains in the XRD dataset. Crystal structure visualization was made using VESTA (Momma and Izumi, 2011) and Diamond software (Putz and Brandenburg, 1999).
2.3 Scanning electron microscopy
The sample was decompressed to ambient pressure and recovered after the experiment. The composition of the sample was analyzed by means of scanning electron microscopy (SEM) using the JOEL JSM-IT500HR scanning electron microscope (JEOL USA, Inc., Peabody, MA, USA). The chemical composition was checked at 15 kV using energy-dispersive X-ray spectroscopy (EDS) of QUANTAX EDS System with XFlash 6,160 detector (Bruker Nano GmbH, Berlin, Germany). The SEM image is shown in Figure 1A, and the resulting spectrum is shown in Figure 1B.
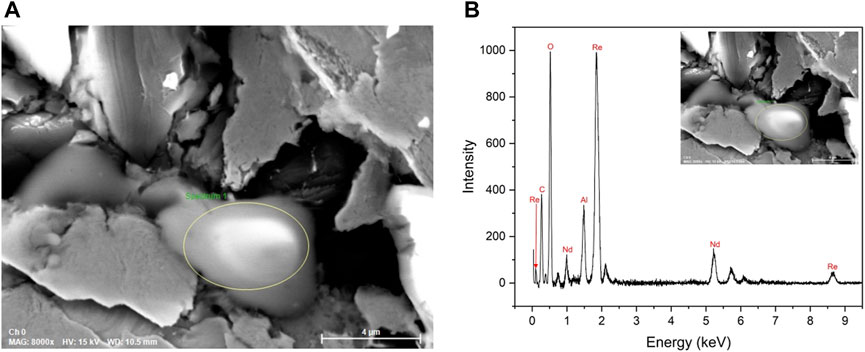
Figure 1. (A) SEM image of the sample with the circled area of the sample; (B) EDS spectrum of the circled site. We can see Nd, Al2O3 (ruby), and Re (gasket). Carbon tape was used as a mount. Boron is not detectable via EDS.
2.4 Ab initio calculations
Density functional theory (DFT) calculations were performed using Vienna ab initio simulation package (VASP 6.3) (Kresse and Furthmüller, 1996a; Kresse and Furthmüller, 1996b). Generalized Gradient Approximation (GGA) was used to describe exchange and correlation effects. The Perdew-Burke-Ernzerhof functional (Perdew et al., 1996) for the exchange-correlation term was used. Electron wave functions were expanded by plane wave with a cutoff energy of 700 eV. Monkhorst and Pack (1976) k-point grids were set as 11 × 11 × 11. Atomic relaxation was performed until the change in the electronic and ionic steps were less than 10–7 eV and 10–6 eV, respectively. VASPKIT package (Wang et al., 2021) was used to extract and analyze the VASP raw output files. Elastic constants were determined using energy-strain method.
3 Results and discussion
The newly synthesized NdRe2 crystallized at 24 (1) GPa in the centrosymmetric cubic
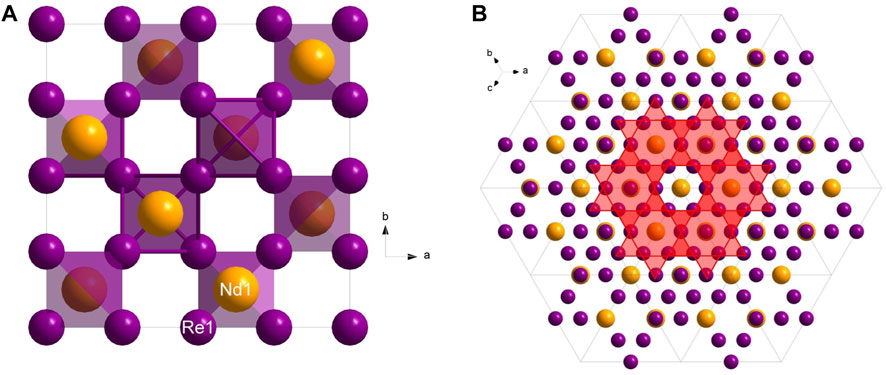
Figure 2. (A) View along the c*-plane of the NdRe2; (B) View along [111] showing the Kagome net pattern.
During the initial single-crystal structure solution and refinement, we used only neodymium and boron, however, our R1 value was never lower than 12% and the residual electron density was very high. To solve this problem, we decided to verify the composition of the synthesized compound using the EDS analysis. Figures 1A, B shows the SEM image of the sample and the spectrum of its composition, respectively. Since the EDS analysis was conducted after sample recovery and the sample in the rhenium gasket was attached to the carbon tape, the precise location of the observed reaction was unknown. Consequently, multiple spots on both sides of the sample were analyzed. In addition to neodymium, the EDS spectrum showed the presence of rhenium and aluminum oxide. The sample was placed close to the ruby (Al2O3) pressure marker and the rhenium gasket, which led to the present compound formation under high pressure and laser heating. Carbon tape was used in the EDS analysis as a mount, which does not allow us to determine if carbon was involved during the synthesis. Although boron was placed in the DAC during the experiment, its presence is not detectable using EDS. Prior to the synthesized compound NdRe2, several other compositions were attempted during the structure solution. Despite our best efforts, it was shown that NdRe2 was synthesized in the experiment.
Due to the limited opening angle of the DAC and, therefore, incomplete datasets, goodness of fit (GOF) values were larger than expected. While additional atom placements were attempted, the GOF value would only marginally decrease while substantially increasing the R1 value. The atom’s thermal parameters were refined in anisotropic approximation and had a data/parameter ratio of 15.5. The residual electron density peaks ranged between 0.5–1.8 e/Å3, indicating that no atoms were missing from the structure.
The compound synthesized in this study is the first single crystal obtained at high pressure conditions and one of two RE-Re compounds with a Laves structure to be observed to crystallize in the space group
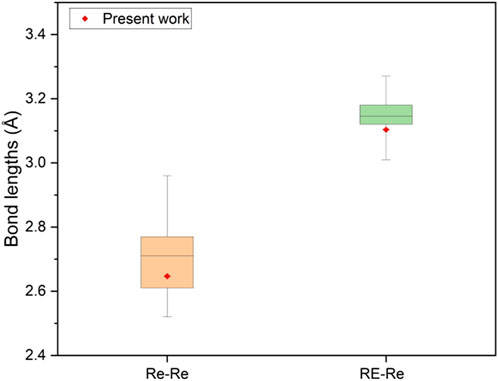
Figure 3. Comparison of interatomic distances of RE-Re and Re-Re in NdRe2 with previous studies, which can be found in Supplementary Table S1.
The calculated mechanical properties of NdRe2 can be found in Tables 3, 4. As the structure is cubic, only three independent elastic constants were needed to calculate the compound’s mechanical properties, such as the bulk and shear modulus. With a Young’s and shear moduli of approximately 53 GPa and 18 GPa, respectively, NdRe2 is located between the only two calculated moduli in the RE-Re system, PrRe2 and YRe2 (Jain et al., 2013; de Jong et al., 2015). The equation of state (EOS) (Figure 4A) provides a bulk modulus of 191.4 GPa. While NdRe2 has a moderate hardness and low stiffness, its B/G value shows that it has high ductility. This corresponds to the positive value of the Cauchy pressure, which indicates that NdRe2 exhibits metallic bonding (Pettifor, 1992; Suetin et al., 2010; Wu et al., 2014). The average wave velocity of 1,275 m/s corresponds to the relatively low Debye temperature of 140.6 K, showing that NdRe2 has low thermal conductivity.
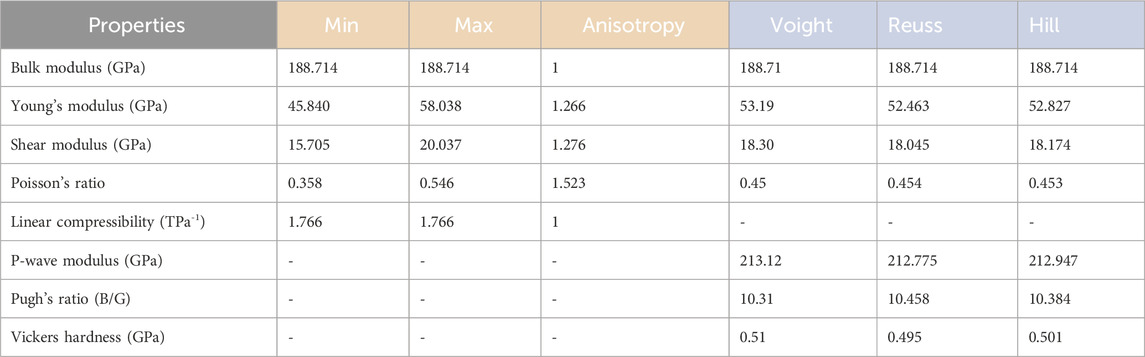
Table 3. Calculated mechanical properties for NdRe2. The red headers correspond to the anisotropic mechanical properties of the bulk single-crystal and the blue headers correspond to the average mechanical properties of the bulk polycrystal.
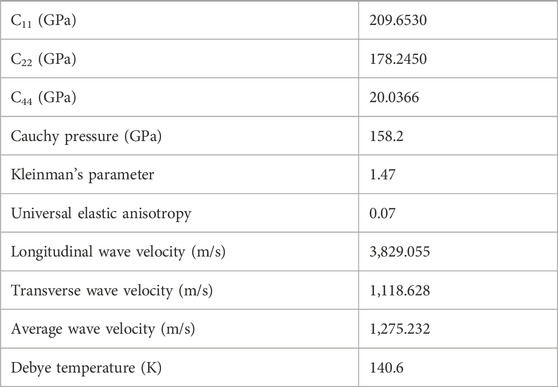
Table 4. Calculated elastic constants Cij and several mechanical properties of the bulk polycrystal.
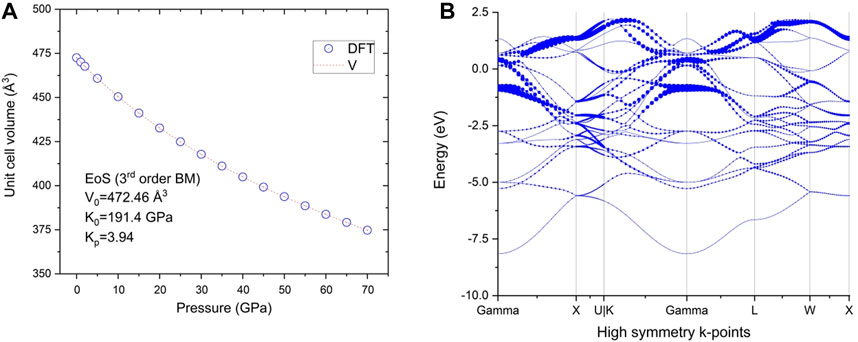
Figure 4. (A) Calculated P-V curve for NdRe2 (symbols) and the fit to Birch–Murnaghan (third order) equation of state; (B) Band structure of NdRe2 at ambient pressure.
In addition to the mechanical properties, the band structure at ambient pressure (Figure 4B) of NdRe2 was calculated. As discussed elsewhere (Jovanovic and Schoop, 2022), Kagome lattices and their band structures can differ depending on the atomic bonding present in the crystal structure of a material. The lattice types are divided between a linked and separated lattice, with the latter being subdivided into categories of interacting, semi-interacting, and isolated compounds. The calculated band structure of NdRe2 indicates that no Kagome bands are present as it belongs to the cubic MgCu2 structure type. Despite this, experimental studies are needed to study the magnetic properties of the material.
4 Conclusion
We report the successful synthesis of a neodymium-rhenium alloy with the formula NdRe2. The structure was obtained by means of single-crystal X-ray diffraction in a diamond anvil cell at 24 (1) GPa and laser heated up to 2,200 (100) K. Its cubic structure belongs to the
Data availability statement
The datasets presented in this study can be found in online repositories. The names of the repository/repositories and accession number(s) can be found below: the joint CCDC/FIZ Karlsruhe online deposition service: https://www.ccdc.cam.ac.uk/structures/? The deposition number CSD-2309202.
Author contributions
ZH: Conceptualization, Data curation, Investigation, Software, Visualization, Writing–original draft. NK: Investigation, Writing–review and editing. KH: Investigation, Writing–review and editing. LV: Investigation, Writing–review and editing. OB: Investigation, Writing–review and editing. VD: Writing–review and editing, Resources, Software. SC: Methodology, Supervision, Writing–review and editing. VP: Methodology, Supervision, Writing–review and editing. IC: Data curation, Funding acquisition, Investigation, Methodology, Project administration, Supervision, Writing–original draft, Writing–review and editing, Validation, Resources.
Funding
The author(s) declare that financial support was received for the research, authorship, and/or publication of this article. We are grateful to the Florida International University and the New Faculty Development Grant 13741410 provided by U.S. Nuclear Regulatory Commission for financial support of this research.
Acknowledgments
We thank Dr. S. Ovsyannikov for providing us with the samples of γ-B used in the experiment. This research used resources of the Advanced Photon Source, a U.S. Department of Energy (DOE) Office of Science User Facility operated for the DOE Office of Science by Argonne National Laboratory under Contract No. DE-AC02-06CH11357. We acknowledge the support of GeoSoilEnviroCARS (Sector 13), which is supported by the National Science Foundation - Earth Sciences (EAR-1634415). This work used Bridges-2 at Pittsburgh Supercomputing Center through allocation Discover MAT230033 from the Advanced Cyberinfrastructure Coordination Ecosystem: Services and Support (ACCESS) program, which is supported by National Science Foundation grants #2138259, #2138286, #2138307, #2137603, and #2138296. The Bridges-2 system is supported by NSF award number ACI-1928147, at the Pittsburgh Supercomputing Center (PSC) (Towns et al., 2014).
Conflict of interest
The authors declare that the research was conducted in the absence of any commercial or financial relationships that could be construed as a potential conflict of interest.
Publisher’s note
All claims expressed in this article are solely those of the authors and do not necessarily represent those of their affiliated organizations, or those of the publisher, the editors and the reviewers. Any product that may be evaluated in this article, or claim that may be made by its manufacturer, is not guaranteed or endorsed by the publisher.
Supplementary material
The Supplementary Material for this article can be found online at: https://www.frontiersin.org/articles/10.3389/fchem.2024.1259032/full#supplementary-material
References
Aslandukov, A., Aslandukov, M., Dubrovinskaia, N., and Dubrovinsky, L. (2022). Domain Auto Finder (DAFi) program: the analysis of single-crystal X-ray diffraction data from polycrystalline samples. J. Appl. Crystallogr. 55, 1383–1391. doi:10.1107/S1600576722008081
Behrendt, D. R., Legvold, S., and Spedding, F. H. (1957). Magnetic properties of neodymium single crystals. Phys. Rev. 106, 723–725. doi:10.1103/PhysRev.106.723
Beierschmitt, K., Buchanan, M., Clark, A., Robertson, I., Britt, P., Navrotsky, A., et al. (2017). Basic research needs for future nuclear energy: report of the basic energy sciences workshop for future nuclear energy, august 9-11, 2017. United States: USDOE Office of Science. doi:10.2172/1616270
Boller, H., and Oesterreicher, H. (1984). On the structure of Nd2Fe14B. J. Less Common Metals 103, L5–L7. doi:10.1016/0022-5088(84)90381-3
Butenkov, D. A., Slastuhina, A. M., Runina, K. I., Grishechkin, M. B., Petrova, O. B., and Levonovich, B. N. (2023). Synthesis and luminescence properties of neodymium-doped oxochloride lead silicate glasses. Russ. J. General Chem. 93, 680–685. doi:10.1134/S1070363223030222
Bykova, E. (2014). Single-crystal X-ray diffraction at extreme conditions in mineral physics and material sciences. Doctor of Natural Sciences. University of Bayreuth.
Bykova, E., Gou, H., Bykov, M., Hanfland, M., Dubrovinsky, L., and Dubrovinskaia, N. (2015). Crystal structures and compressibility of novel iron borides Fe2B7 and Fe B50 synthesized at high pressure and high temperature. J. Solid State Chem. 230, 102–109. doi:10.1016/j.jssc.2015.06.040
Cannon, J. F., Robertson, D. L., and Hall, H. T. (1972). Synthesis of lanthanide-iron laves phases at high pressures and temperatures. Mater. Res. Bull. 7, 5–11. doi:10.1016/0025-5408(72)90063-3
Chong, S., Riley, B. J., and Nelson, Z. J. (2022). Crystalline compounds for remediation of rare-earth fission products: a review. J. Rare Earths 40, 365–380. doi:10.1016/j.jre.2021.01.016
Dactools, L., Prakapenka, V., Kantor, A., Dera, P., Kurnosov, A., Sinogeikin, S., et al. (2012). BX90: a new diamond anvil cell design for X-ray diffraction and optical measurements. Rev. Sci. Instrum. 83, 125102. doi:10.1063/1.4768541
Darab, J. G., and Smith, P. A. (1996). Chemistry of technetium and rhenium species during low-level radioactive waste vitrification. Chem. Mater. 8, 1004–1021. doi:10.1021/cm950418+
Darby, J. B., Berndt, A. F., and Downey, J. W. (1965). Some intermediate phases in the thorium-technetium and uranium-technetium systems. J. Less Common Metals 9, 466–468. doi:10.1016/0022-5088(65)90132-3
Darby, J. B., Lam, D. J., Norton, L. J., and Downey, J. W. (1962). Intermediate phases in binary systems of technetium-99 with several transition elements. J. Less Common Metals 4, 558–563. doi:10.1016/0022-5088(62)90044-9
Darby, J. B., Norton, L. J., and Downey, J. W. (1964). Technetium compounds with the MgZn2 structure. J. Less Common Metals 6, 165–167. doi:10.1016/0022-5088(64)90120-1
De Jong, M., Chen, W., Angsten, T., Jain, A., Notestine, R., Gamst, A., et al. (2015). Charting the complete elastic properties of inorganic crystalline compounds. Sci. Data 2, 150009. doi:10.1038/sdata.2015.9
Dewaele, A., Torrent, M., Loubeyre, P., and Mezouar, M. (2008). Compression curves of transition metals in the Mbar range: experiments and projector augmented-wave calculations. Phys. Rev. B 78, 104102. doi:10.1103/PhysRevB.78.104102
Fei, Y., Ricolleau, A., Frank, M., Mibe, K., Shen, G., and Prakapenka, V. (2007). Toward an internally consistent pressure scale. Proc. Natl. Acad. Sci. 104, 9182–9186. doi:10.1073/pnas.0609013104
Giorgi, A. L., and Szklarz, E. G. (1970). Superconductivity and lattice parameters of the dirhenides and ditechnides of thorium, hafnium and zirconium. J. Less Common Metals 22, 246–248. doi:10.1016/0022-5088(70)90027-5
Gratz, E., Kottar, A., Lindbaum, A., Mantler, M., Latroche, M., Paul-Boncour, V., et al. (1996). Temperature- and pressure-induced structural transitions in rare-earth-deficient (R = Y, Sm, Gd, Tb) Laves phases. J. Phys. Condens. Matter 8, 8351–8361. doi:10.1088/0953-8984/8/43/026
Haines, H. R., Mardon, P. G., and Potter, P. E. (1976). Some constitutional studies on uranium-carbon- and plutonium-carbon-rhenium and technetium systems. Netherlands: North-Holland.
Hartmann, T., Alaniz, A., Poineau, F., Weck, P. F., Valdez, J. A., Tang, M., et al. (2011). Structure studies on lanthanide technetium pyrochlores as prospective host phases to immobilize 99technetium and fission lanthanides from effluents of reprocessed used nuclear fuels. J. Nucl. Mater. 411, 60–71. doi:10.1016/j.jnucmat.2011.01.033
Hyatt, N. C., and Ojovan, M. I. (2019). Special issue: materials for nuclear waste immobilization. Materials 12, 3611. doi:10.3390/ma12213611
Jain, A., Ong, S. P., Hautier, G., Chen, W., Richards, W. D., Dacek, S., et al. (2013). Commentary: the Materials Project: a materials genome approach to accelerating materials innovation. Apl. Mater. 1, 011002. doi:10.1063/1.4812323
Jovanovic, M., and Schoop, L. M. (2022). Simple chemical rules for predicting band structures of Kagome materials. J. Am. Chem. Soc. 144, 10978–10991. doi:10.1021/jacs.2c04183
Kresse, G., and Furthmüller, J. (1996a). Efficiency of ab-initio total energy calculations for metals and semiconductors using a plane-wave basis set. Comput. Mater. Sci. 6, 15–50. doi:10.1016/0927-0256(96)00008-0
Kresse, G., and Furthmüller, J. (1996b). Efficient iterative schemes for ab initio total-energy calculations using a plane-wave basis set. Phys. Rev. B 54, 11169–11186. doi:10.1103/PhysRevB.54.11169
Lam, D. J., and Mitchell, A. W. (1972). Laves phases of actinide elements. J. Nucl. Mater. 44, 279–284. doi:10.1016/0022-3115(72)90038-4
Lindbaum, A., Heathman, S., and Gratz, E. (2002). Pressure induced disordering of vacancies in RNi 2 compounds. High Press. Res. 22, 411–414. doi:10.1080/08957950212805
Momma, K., and Izumi, F. (2011). VESTA 3 for three-dimensional visualization of crystal, volumetric and morphology data. J. Appl. Crystallogr. 44, 1272–1276. doi:10.1107/S0021889811038970
Monkhorst, H. J., and Pack, J. D. (1976). Special points for Brillouin-zone integrations. Phys. Rev. B 13, 5188–5192. doi:10.1103/PhysRevB.13.5188
Perdew, J. P., Burke, K., and Ernzerhof, M. (1996). Generalized gradient approximation made simple. Phys. Rev. Lett. 77, 3865–3868. doi:10.1103/PhysRevLett.77.3865
Petříček, V., Dušek, M., and Palatinus, L. (2014). Crystallographic computing system JANA2006: general features. De. Gruyter 229, 345–352. doi:10.1515/zkri-2014-1737
Pettifor, D. G. (1992). Theoretical predictions of structure and related properties of intermetallics. Mater. Sci. Technol. 8, 345–349. doi:10.1179/mst.1992.8.4.345
Rafiuddin, M. R., Donato, G., Mccaugherty, S., Mesbah, A., and Grosvenor, A. P. (2022). Review of rare-earth phosphate materials for nuclear waste sequestration applications. ACS Omega 7, 39482–39490. doi:10.1021/acsomega.2c03271
Rivers, M., Prakapenka, V. B., Kubo, A., Pullins, C., Holl, C. M., and Jacobsen, S. D. (2008). The COMPRES/GSECARS gas-loading system for diamond anvil cells at the Advanced Photon Source. High Press. Res. 28, 273–292. doi:10.1080/08957950802333593
Roy, B., Chakrabarty, S., Mondal, O., Pal, M., and Dutta, A. (2012). Effect of neodymium doping on structure, electrical and optical properties of nanocrystalline ZnO. Mater. Charact. 70, 1–7. doi:10.1016/j.matchar.2012.04.015
Salamatin, D. A., Klementiev, K. V., Krasnorussky, V. N., Magnitskaya, M. V., Chtchelkachev, N. M., Sidorov, V. A., et al. (2023). The new high-pressure hexagonal Laves phase of the YbZn2 compound. J. Alloys Compd. 946, 169275. doi:10.1016/j.jallcom.2023.169275
Savitskii, E., and Khamidov, O. (1965). Crystal structure of compounds of rhenium with praseodymium. Izv. Akad. Nauk. SSSR, Neorg. Mater. 1, 1621–1622.
Sheldrick, G. (2015a). Crystal structure refinement with SHELXL. Acta Crystallogr. Sect. C 71, 3–8. doi:10.1107/S2053229614024218
Sheldrick, G. (2015b). SHELXT - integrated space-group and crystal-structure determination. Acta Crystallogr. Sect. A 71, 3–8. doi:10.1107/S2053273314026370
Stein, F., and Leineweber, A. (2021). Laves phases: a review of their functional and structural applications and an improved fundamental understanding of stability and properties. J. Mater. Sci. 56, 5321–5427. doi:10.1007/s10853-020-05509-2
Suetin, D. V., Shein, I. R., and Ivanovskii, A. L. (2010). Structural, elastic, electronic and magnetic properties of perovskite-like Co3WC, Rh3WC and Ir3WC from first principles calculations. Solid State Sci. 12, 814–817. doi:10.1016/j.solidstatesciences.2010.02.013
Sutton, S. R., Rivers, M. L., Chariton, S., Eng, P. J., Lanzirotti, A., Newville, M., et al. (2022). GeoSoilEnviroCARS (Sector 13) at the Advanced Photon Source: a comprehensive synchrotron radiation facility for Earth science research at ambient and extreme conditions. Phys. Chem. Minerals 49, 32. doi:10.1007/s00269-022-01207-4
Szklarz, E. G., and Giorgi, A. L. (1981). Superconductivity of the laves phases YTc2, ScTc2 and LuTc2. J. Less Common Metals 81, 349–351. doi:10.1016/0022-5088(81)90041-2
Towns, J., Cockerill, T., Dahan, M., Foster, I., Gaither, K., Grimshaw, A., et al. (2014). XSEDE: accelerating scientific discovery. Comput. Sci. Eng. 16, 62–74. doi:10.1109/MCSE.2014.80
U.S. Energy Information Administration (2022). Nuclear power and the environment. Available at: https://www.eia.gov/energyexplained/nuclear/nuclear-power-and-the-environment.php.
Villars, P., and Calvert, L. D. (1991). Pearson’s handbook of crystallographic data for intermetallic phases. United States: ASM international.
Wang, V., Xu, N., Liu, J.-C., Tang, G., and Geng, W.-T. (2021). VASPKIT: a user-friendly interface facilitating high-throughput computing and analysis using VASP code. Comput. Phys. Commun. 267, 108033. doi:10.1016/j.cpc.2021.108033
World Nuclear Association (2021). What is nuclear waste, and what do we do with it? Available at: https://www.eia.gov/energyexplained/nuclear/nuclear-power-and-the-environment.php.
Wu, D.-H., Wang, H.-C., Wei, L.-T., Pan, R.-K., and Tang, B.-Y. (2014). First-principles study of structural stability and elastic properties of MgPd3 and its hydride. J. Magnesium Alloys 2, 165–174. doi:10.1016/j.jma.2014.06.001
Keywords: diamond anvil cell, high pressure high temperature, laser heating, neodymium rhenium, crystal structure, synthesis, laves phases, nuclear waste
Citation: Hussein Z, Kazemiasl N, Hussaini K, Vaquero L, Barkova O, Drozd V, Chariton S, Prakapenka V and Chuvashova I (2024) High-pressure high-temperature synthesis of NdRe2. Front. Chem. 12:1259032. doi: 10.3389/fchem.2024.1259032
Received: 14 July 2023; Accepted: 25 March 2024;
Published: 16 April 2024.
Edited by:
Sathish C. I., The University of Newcastle, AustraliaReviewed by:
Yuanpeng Zhang, Oak Ridge National Laboratory (DOE), United StatesYanfeng Guo, ShanghaiTech University, China
Copyright © 2024 Hussein, Kazemiasl, Hussaini, Vaquero, Barkova, Drozd, Chariton, Prakapenka and Chuvashova. This is an open-access article distributed under the terms of the Creative Commons Attribution License (CC BY). The use, distribution or reproduction in other forums is permitted, provided the original author(s) and the copyright owner(s) are credited and that the original publication in this journal is cited, in accordance with accepted academic practice. No use, distribution or reproduction is permitted which does not comply with these terms.
*Correspondence: Irina Chuvashova, irina.chuvashova@fiu.edu; Zain Hussein, zhuss009@fiu.edu