Unveiling the contemporary progress of graphene-based nanomaterials with a particular focus on the removal of contaminants from water: a comprehensive review
- 1Department of Chemistry, School of Chemical Engineering and Physical Sciences, Lovely Professional University, Phagwara, India
- 2Department of Chemistry, National Institute of Technology, Srinagar, Jammu and Kashmir, India
- 3Department of Mechanical Engineering, Nalanda College of Engineering, Bihar Engineering University, Department of Science, Technology and Technical Education, Government of Bihar, Patna, India
- 4Department of Chemistry, Nalanda College of Engineering, Bihar Engineering University, Department of Science, Technology and Technical Education, Government of Bihar, Patna, India
Water scarcity and pollution pose significant challenges to global environmental sustainability and public health. As these concerns intensify, the quest for innovative and efficient water treatment technologies becomes paramount. In recent years, graphene-based nanomaterials have emerged as frontrunners in this pursuit, showcasing exceptional properties that hold immense promise for addressing water contamination issues. Graphene, a single layer of carbon atoms arranged in a hexagonal lattice, exhibits extraordinary mechanical, electrical, and chemical properties. These inherent characteristics have led to a surge of interest in leveraging graphene derivatives, such as graphene oxide (GO), reduced graphene oxide and functionalized graphene, for water treatment applications. The ability of graphene-based nanomaterials to adsorb, catalyze, and photocatalyze contaminants makes them highly versatile in addressing diverse pollutants present in water sources. This review will delve into the synthesis methods employed for graphene-based nanomaterials and explore the structural modifications and functionalization strategies implemented to increase their pollutant removal performance in water treatment. By offering a critical analysis of existing literature and highlighting recent innovations, it will guide future research toward the rational design and optimization of graphene-based nanomaterials for water decontamination. The exploration of interdisciplinary approaches and cutting-edge technologies underscores the evolving landscape of graphene-based water treatment, fostering a path toward sustainable and scalable solutions. Overall, the authors believe that this review will serve as a valuable resource for researchers, engineers, and policymakers working toward sustainable and effective solutions for water purification.
1 Introduction
There is a global increase in soil, air, and water pollution due to the world’s rising urbanization and industry (Celik, 2020). A clean and safe environment is therefore essential for survival and maintaining a healthy physique. As water is a vital component of life, water pollution is the most serious form of pollution and has the greatest negative influence on public health and the environment (Mahmoud, 2020). Water has a profound effect on every facet of human existence, such as food, energy, economy, and health (Yin et al., 2020). A fresh water supply is necessary for the protection of children and the impoverished, in addition to the negative effects that inadequate sanitation and water availability have on the environment, the economy, and society (Amin et al., 2014). The textile, pharmaceutical, and metal industries, among others, discharge toxic substances into the environment that damage freshwater bodies. Pesticides, organic dyes, heavy metal ions (HMIs), and other pollutants are among these hazardous substances (Yap et al., 2021). The most hazardous to the environment among these pollutants are HMIs and organic dyes because of their immunogenic, carcinogenic, and mutagenic qualities (Wani et al., 2022). This is because of their low degradability and strong accumulative impacts (Mohd et al., 2022). In humans, animals, and plants, these consequences can reduce neurological, hormonal, and reproductive capacities. When present in appropriate proportions, several heavy metals are considered important nutrients; however, when their concentration surpasses a threshold, they become hazardous to the organism (Lu and Astruc, 2018), as shown in Figure 1.
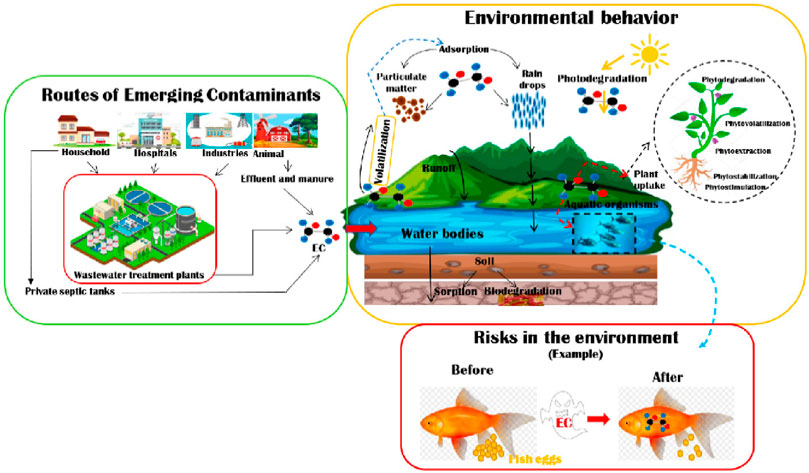
FIGURE 1. Schematic representation of different entry pathways of contaminants into the environment, their action, and influences (Almeida-Naranjo et al., 2023).
Waterborne infections are thought to be the cause of 10–20 million deaths annually, while non-fatal infections claim the lives of over 200 million people (Leonard et al., 2003). As per estimates, diarrhea, a water-related issue, claims the lives of 5,000–6,000 children per day (Ashbolt, 2004). Currently, over 0.78 billion people lack safe water supplies globally, which has a negative impact on their health (Amin et al., 2014). The existing water supply will drop by one-third in a few decades, and it is projected that over a billion people worldwide lack access to clean water (Liu and Qiu, 2020). Therefore, a global effort is currently being made to understand how human activity impacts the environment and develop new technologies to reduce any detrimental consequences on public health and the environment in which they live (Gusain et al., 2020). Given that aquifers worldwide are being depleted as a result of various factors, including surface water contamination and saltwater intrusion, now is the ideal time to address water-related issues. Improving purification technology can lessen issues related to energy, water scarcity, health, and climate change (Krishna et al., 2023). Reusing wastewater (WW) can result in a significant reduction in the amount of potable water used, but doing so needs the development of dependable, efficient, and affordable materials and techniques. The burden of micro-pollutants downstream can be reduced by diluting complicated WW effluents, but many of these compounds still pass through conventional water treatment as they exist in micrograms or even nanograms per liter (Verstraeten et al., 2003).
Various treatment technologies have been utilized to eradicate harmful contaminants from water and WW efficiently and comprehensively. Flocculation, membrane filtration, photo-catalysis, chemical precipitation, electrochemical elimination, ion exchange, and adsorption are a few of these methods (Wani et al., 2021). Even though these technologies exist, very few of them are used by enterprises to treat their sewage due to several drawbacks, including high maintenance costs, energy requirements, complex operational procedures, a lack of a circular economy perspective, and sustainability. For the removal of dyes, HMIs, and other hazardous pollutants, the adsorption process is regarded as among the most extensively utilized, valuable, adaptable, and highly efficient techniques available (Khan et al., 2019). Adsorption can be carried out using a variety of materials, such as red mud, fly ash, crop residues, and microbiological cells. Adsorption has several advantages, but despite these, its application in the commercial sector is still quite restricted due to its slow removal effectiveness after a few operating cycles. Adsorbents should ideally have enough binding sites to allow for the effective adsorption of harmful pollutants. If the adsorbent is recyclable, this can further reduce the cost of the adsorption process.
Recent advances in the science of nanotechnology (NT) have sparked considerable attention regarding harnessing the special features of NMs for environmental remediation as one approach to address these urgent environmental concerns (Xu et al., 2012; Assad et al., 2022b). Because of their nanoscale size, NMs have unique properties that can be used to develop new technologies or improve the performance of ones that already exist. A growing body of literature discusses how innovative NMs might be applied to solve significant environmental problems (Assad et al., 2022a). Advanced water systems are developing more effective treatment methods thanks to the use of NMs like CNTs and dendrimers (Kuchi et al., 2021; Ganjoo et al., 2023). To preserve the stability of the ecosystem, NT can be used in numerous ways to address the many water quality issues. The use of NPs and NMs in nanoremediation is the process of eliminating environmental pollutants from contaminated areas. Both chemical and biological processes, including those involving plants, fungi, and bacteria, can produce these NPs and NMs (Nikam et al., 2022). As seen in Figure 2, various studies found that NMs, including GBNs (GBNs), silver (Ag), cerium oxide (CeO2), titanium oxide (TiO2), zinc oxide (ZnO2), nano zero valent iron (nZVI), and nano carbon black (NCB), were effective at removing pollutants (Masindi and Muedi, 2018).
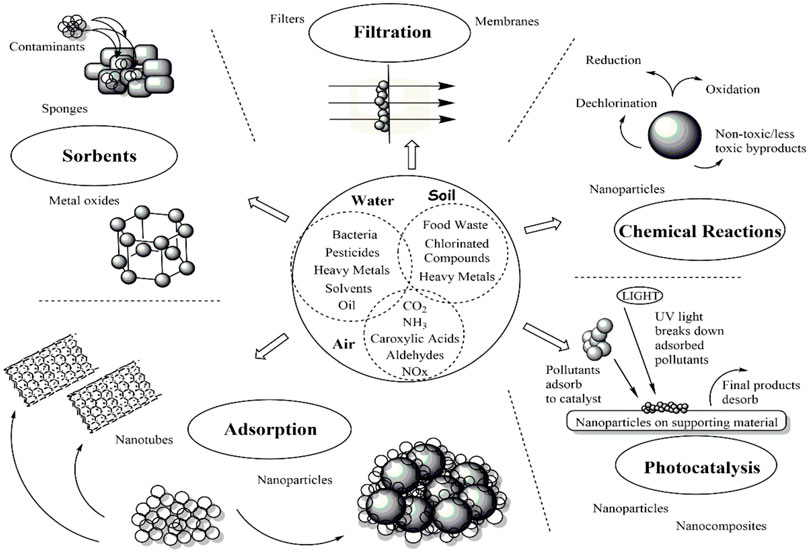
FIGURE 2. An introduction to general techniques for environmental remediation using nanotechnology (Mathur et al., 2022).
Graphene, a 2D material comprised of layers of carbon atoms that create six-membered rings, is considered the ultimate graphitic form (fullerenes, CNTs, etc.), Zhi and Müllen (2008) and has caught the attention of scientists. The unusual physicochemical characteristics of graphene, particularly its extraordinarily high SA, ē heat mobility, and mechanical potency, are what initially sparked interest in it Assad et al. (2023c). Theoretically, graphene has the largest specific surface area (SA) of any substance at 2,630 m2 g-1 and is considered the perfect material for adsorption or surface reaction processes (Perreault et al., 2015). In addition, graphene provides good support for securing chemical functions or NMs; as a result, GBNs and nanocomposites have attracted a lot of consideration from researchers looking for new materials (Al Faruque et al., 2021). The total number of publications retrieved from Google Scholar for the past 2 decades is shown below in the chart graph (Figure 3), in which the gradual increase in the use of graphene-based materials is clearly discernible.
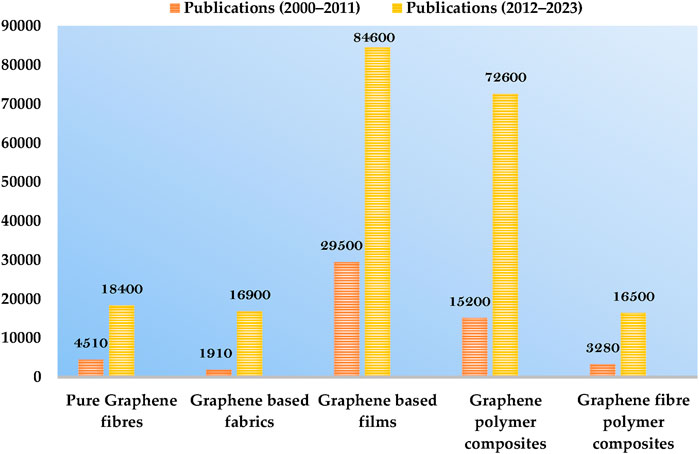
FIGURE 3. Graphical representation of the growing number of publications on graphene-based materials. The bars represent the number of publications retrieved from Google Scholar. The examined time interval was (left) 2000–2011 and (right) 2012–2023.
Graphene or graphite can be employed as the initial material for making GBNs, which are graphene-like nanostructures. They differ from each other due to surface chemistries, the quantity of imperfections, and lateral dimensions yet both include sp2 and sp3 hybridized C atoms. Graphene oxide (GO), reduced graphene oxide (rGO), and graphene quantum dots (GQDs) are examples of GBNs (Lúcio et al., 2021). Moreover, graphene-based nanomaterial production and application-related articles and patents have grown rapidly and continue to do so (Zou et al., 2018). Unique characteristics of GBNs have been discovered, and new processes for the quick and effective fabrication of graphene-based nanocomposites with applications in numerous domains have been covered extensively in review articles over the past few decades (Ghany et al., 2017; Ganjoo et al., 2023). Molina et al. examined the most important studies concerning graphene-based electrochemical sensors for the measurement of toxic ions (Molina et al., 2016). Contemporary progress in the preparation and use of GBNs for drug delivery were reviewed by Wang et al. (2017). Additionally, Madni et al. (2018) examined the biological and physicochemical features of GBNs and described the approaches for producing them. Jiang et al. (2019) created a flexible LED employing laser-induced reduced graphene oxide (LI-rGO), which has a wall plug efficiency (WPE) of 1.4% and a luminous endurance of over 60 h.
In actuality, materials based on graphene provide a wide variety of opportunities for restoring the environment and using electricity. Furthermore, numerous 2D graphene NMs, including pure graphene, GO, and reduced GO, have been developed as important crucial NMs for decontaminating soil (Fathizadeh et al., 2017). These nanoparticles are well renowned for their prowess as adsorbents and antifouling experts. They also possess PC qualities. When compared with ordinary polyamide membranes, graphene membranes have nuclear-level fineness, which increases filtering (Homaeigohar and Elbahri, 2017). The tendency of graphene to form prolonged aggregates or even regain graphite stacking and van der Waals interactions represents the biggest barrier to deploying GBNs in the realm of environmental protection, which results in insufficient separation between the sheets (Khalid et al., 2020). Toxic contaminants can be removed from WW with the use of graphene-based nanoparticles, as evidenced by the numerous excellent evaluations that have been published to date. However, the majority of these assessments concentrate on a single class of pollutant or are restricted to a specific kind of material or adsorbent. We have covered in-depth the adsorption and photodegradation of different pollutants, such as antibiotics, pesticides, dyes, and HMIs, on graphene and GBNs in this review. These processes are essential for the effective use of GBNs to treat contaminated WW. In the meanwhile, an evaluation of the materials' risks, as well as the difficulties and prospects of getting rid of the pollutants that have been clarified, are presented to bring about additional fascinating advancements in this relatively new but extremely promising subject in the future. By concentrating on the difficulties facing future research, the authors hope that this work will offer a distinctive viewpoint on the fundamental studies of GBNs for the management of water and WW.
2 Fabrication methods
It would be ideal if the fabrication of graphene and materials based on it could be managed to give rise to features that would be useful in particular contexts. As is well known, there are two primary approaches for fabricating graphene: top-down and bottom-up (Jana et al., 2017). Top-down methods require the segregation of assembled graphite sheets to produce solitary graphene sheets, while the bottom-up process brings the production of graphene from different carbon sources (Yang et al., 2016), as shown in Figure 4.
How to generate effective substance in a repeatable means, on a huge level, and at a reasonable price is one of the key difficulties in commercializing graphene. Although this is still a significant difficulty, several other ways to make graphene have been explored recently. Given that a significant portion of graphene-based filters are crafted using top-down methodologies (Li et al., 2021), this section will exclusively elucidate the top-down approach and its associated techniques. By tearing apart the stacked layers of graphite, this technology uses procedures to create a single graphene sheet (Sengupta and Hussain, 2019; Vesel et al., 2019). Moreover, graphite or other C-sources, like CNTs (carbon nanotubes), fullerenes, or bigger graphene sheets that are sliced into minor monoatomic C bits, are the starting point for top-down techniques for creating GBNs (Jana et al., 2017; Pedrosa et al., 2020). These techniques could be physical, chemical, or mechanical. The next section discusses a few top-down strategies.
2.1 Mechanical exfoliation from graphite
The first high-quality graphene was produced utilizing scotch tape and mechanical exfoliation from raw graphite (Sahu et al., 2021). The original two-dimensional atomic lattices of graphene are completely preserved despite the method’s primitiveness and low efficiency (Dasari Shareena et al., 2018). This technique includes regularly rubbing SiO2/Si substrates with scotch tape to exfoliate graphite (Jana et al., 2017). Single or few-layer graphene of very good quality can be distinguished with realistic discrimination thanks to the distinctive color contrast, despite being laborious and poor yielding (Chang and Wu, 2013). The extensiveness of mechanically exfoliated graphene means ultrahigh mobility and a variety of fascinating electrical characteristics are easily observable (Geim, 2009). A great deal of attention toward GBNs and other 2D atomic crystals was sparked by the effective mechanical exfoliation of the first high-worth graphene from graphite (Chang and Wu, 2013).
2.2 Unzipping carbon nanotubes
Unzipping carbon nanotubes is another technique that is frequently used to investigate the physics of graphene because of its comparatively good quality. Oxidized graphene nanoribbons were produced by longitudinally slicing multi-walled CNTs employing laser irradiation, plasma etching techniques, or wet chemical methods (Sahu et al., 2021). This unlocking results in the production of graphene nanoribbons. The nanoribbon widths are determined by the tube diameter. After immersion in H2SO4, the nanotubes were cut and treated in KMnO4 (Sahu et al., 2021). Uneven sides emerge from CeC fission, which is often started at the distortion sites to decompress the nanotubes (Cho et al., 2011). Owing to the existence of oxygen deformation sites, the resulting graphene nanoribbons are strong conductors but have a lower electrical quality than commercial-scale graphene layers (Kosynkin et al., 2009). Later, the unzipping of flattened CNTs, where outbreak arises beside the bent edges, was carried out (Saeed et al., 2020) to generate nanoribbons with smooth edges.
2.3 Chemical reduction of graphene oxide
An alternative method of making graphene is the reduction of GO (De Silva et al., 2017). The presence of polar O and OH groups causes the graphite oxide to become hydrophilic throughout the oxidation process (Pei and Cheng, 2012; Dasari Shareena et al., 2018). Water is one of the solvents used in the chemical peeling process of this GO. Numerous graphite oxide nanoplatelets are produced by the sonicated graphite oxide solution (Li et al., 2021). The reduction process involves the removal of oxygen units using reducing chemicals. Stankovich and others employed this method by considering a hydrazine-reducing agent; however, it was found that the reduction advancement was insufficient, leaving some residual oxygen. When graphene is being manufactured, GO is created as a precursor (Stankovich et al., 2007). GO is advantageous over graphite due to its hydrophilic behavior (Pedrosa et al., 2020). Sonication is used to suspend GO in water. After that, spin coating or filtering is used to deposit it onto surfaces to create single/double layer GO. Then, to create graphene films, this GO is reduced by thermal or chemical actions (Marcano et al., 2010). The methods (Sieradzka et al., 2021) used to create GO include.
• Wet chemical synthesis
• Plasma functionalization
• Radio frequency plasma
2.4 Arc discharge
This process creates a few layers of graphene by applying direct current under high pressure from hydrogen gas between electrodes constructed of ultra-pure graphite (Li et al., 2021). Shen et al. found that the combination of helium (He) and hydrogen (H2) gas accounts for the material’s remarkable crystallinity out of all the different gases considered (Shen et al., 2012). The development method comprises a progressive route of graphite evaporation and reactive-gas-restrictive crystallization of the vaporized carbon units. Aqueous arc discharge technology, according to Kim et al., can generate bi- and tri-layer graphene (Kim et al., 2016). With the aid of a temperature increase, heat transfer, and aqueous turbulence, graphene was extracted from the graphite electrodes. A median proportion of graphene sheets with fewer oxygen-related defects were created by varying the voltage of the arc discharge (Li et al., 2021). Owing to a lack of scalability, intricacy, and the high expense of the current technique of synthesizing graphene, GBN research is still a long way from having any important uses.
3 Structure and characteristics of graphene-based NMs
Graphene is regarded as the basic component of the family of carbon-based substances, as it can roll into 1D CNTs, wrap into 0D fullerenes, and stack into 3D graphite, enriching the family of carbon compounds. GBNs are usually classified based on layers, oxygen-containing group matter, and other chemical components. According to one study, the structural variations of GBNs, which control their physical and chemical characteristics, are numerous (Yang et al., 2018a). Graphene is criticized as an “impractical material" as it has not been demonstrated that monolayer graphene is stable, even though past research on GBNs concentrated mostly on its configuration and capabilities. Landau, who asserted that rigid 2D crystals are thermodynamically impossible, was proven wrong by the planar 2D framework of graphene (O’Hare et al., 2012). Furthermore, it was discovered that graphene is not a perfect 2D crystal using analysis methods like Raman spectroscopy, TEM, and AFM. To improve stability, graphene sheets are distorted. The chemical makeup of GBNs is depicted in Figure 5.
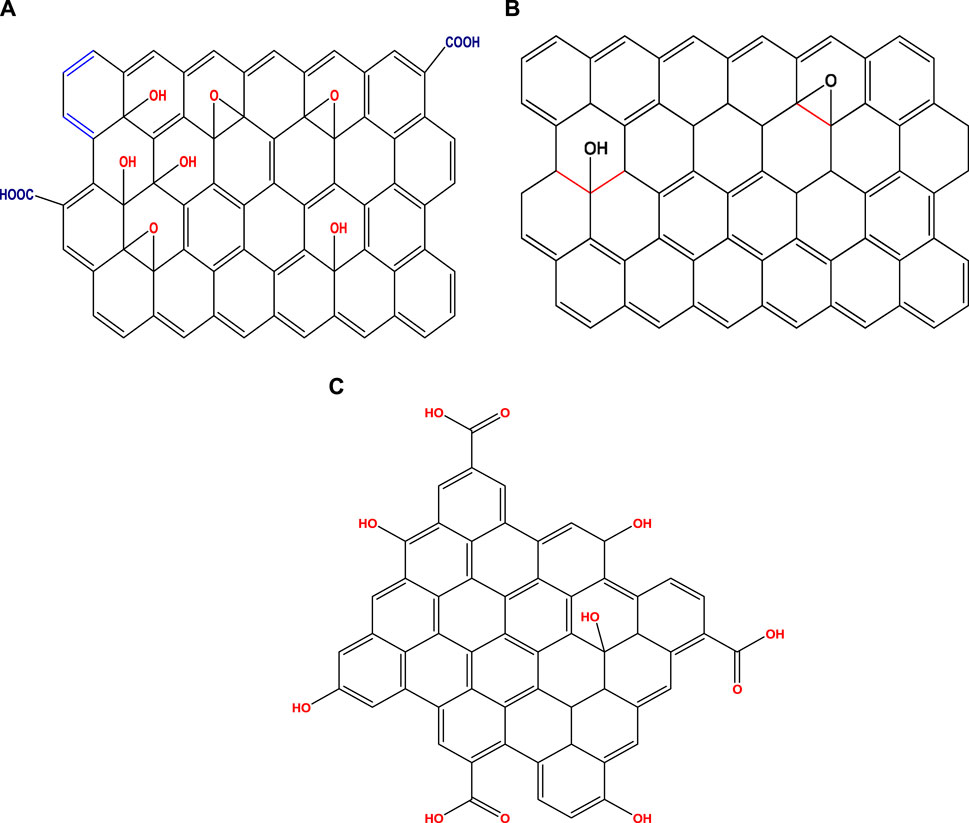
FIGURE 5. Schematic depiction of several graphene variants. (A) Graphene (B) Graphene oxide (C) Reduced graphene oxide.
Standard graphene is a monolayer of graphite with a hexagonal planar organization of C-atoms. With a reported width of approximately 0.335 nm, graphene is the reediest 2D constituent ever to be found. It was initially produced using CVD to mechanically or chemically exfoliate graphite (Zhao et al., 2017a). Three identical carbon atoms are linked to one carbon atom. A C–C bond is a σ-bond created by sp2 hybrid orbitals and recognized by its bond length and bond angle, which are approximately 1.42 nm and 120°, respectively. A carbon atom contributes one electron to the system by joining the p orbitals of its neighboring carbon atoms. As п-bonds and a cloud of ē pairs are honeycombed, graphene’s skeleton is made of them. Identical in structure and functionality to graphene, GO also possesses outstanding physiochemical qualities. GO is a single-layer form of graphene that is created when powerful acids and oxidants react. The outer layer of the material encompasses several functional moieties, including -OH, -O, and -CH (O) CH-. In contrast to graphene, the GO structure uses a very specific approach for the oxygen atoms' bonding with the carbon atoms. In addition to having a monolithic configuration akin to graphene and an extremely selective surface area as a consequence of the oxidation reaction, GO also has several functional units on its upper surface that make it simpler for it to endure additional surface reconfiguration. This is the main driver behind GO’s extensive adoption in a variety of industries. GO simultaneously possesses sp2 and sp3 hybrid orbitals, which stand for the aromatic and aliphatic domains, respectively. GO reduces the conductivity of graphene, but reduction can restore it (rGO). A thermal or chemical reduction of graphite oxide or graphene can result in the intergradation known as rGO (Cheng et al., 2020). Between the perfect sheets of graphene and extremely oxidized GO, rGO is regarded as an intermediary structure (Reina et al., 2017). By using a reducing procedure, such as chemical and physical reduction procedures, the oxygen units on the interface of GO can also be lowered in the case of rGO. Moreover, owing to the lowering of faults brought on by the inclusion of functional groups (FGs), such reduction aids in strengthening the graphitic nature of GO. Moreover, GQDs, a most recent variety of GBNs, are often created utilizing artificial processes including thermal plasma jets. They are thinner (1–20 nm, less than 10 layers), have carbon bonds that are similar to those in graphene, have a greater SA, and splicing that is appropriate for surface modification (Zhao et al., 2017b). Additionally, owing to the edge effect and the same quantum limitations as the C point, GQDs display a novel phenomenon known as steady PL. GQDs have hydrophilic groups like -OH and -COOH at their edges, which enable researchers to alter them as required (Li et al., 2018; Chung et al., 2021).
Since 2004, numerous intriguing characteristics of graphene, including its high SA, great electrical and mechanical conductivity, and outstanding thermal characteristics, have been identified. There are many resources of knowledge concerning the characteristics of graphene (Soldano et al., 2010). Here, we emphasized how the exemplary structure of graphene and GBNs are related to the unique honeycomb pattern of graphene and its products. We concentrated on the characteristics of graphene and GBNs that are of utmost importance for their implementation in a variety of domains, such as biomedicine, the environment, and industry.
3.1 Mechanical properties
Each C atom in a graphene layer is covalently connected to three nearby atoms as a result of the 2D honeycomb configuration of carbon atoms. A single defect-free graphene sheet is therefore approximately 200 times mechanically extremely durable due to the tight C-C covalent connections that give graphene its exceptional structural stiffness. This explains the remarkable mechanical properties of graphene, which include its 1 TPa Young’s modulus, 0.149 GPa Poisson’s ratio, and 130 GPa fracture strength (Lee et al., 2010). Owing to its remarkable mechanical qualities, interest in using graphene as a filler to improve the tensile strength of lighter substances has grown (Potts et al., 2011). In comparison to graphene, the surface moieties and imperfections left behind from oxidation or other handling operations have a considerable impact on the mechanical characteristics of GO and rGO. However, these GBNs still have a very high stiffness. These NMs may be employed to fill or reinforce the frameworks of medical apparatuses, hydrogels, biodegradable coverings, electrospun fibers, and other tissue engineering scaffolds because of the amazing mechanical stability of graphene and the good mechanical qualities of GBNs (Zhao et al., 2020). When juxtaposed with CNTs, graphene can dramatically improve the characteristics of polymers that have also been thoroughly examined as nanofillers for polymer matrixes. This is because the large SA of the planar graphene layers permits better relationships between the sheets and the polymer matrix (Perreault et al., 2015).
3.2 Physicochemical properties
The enormous SA and sp2 network of graphene are its primary unique physicochemical properties. These two qualities give graphene a high degree of reactivity. The electrophilic replacement processes that the graphene planar and ē arrangements can participate in include click reactions, cycloadditions, and reactions to carbine insertion. Additionally, the sp2 network allows for п-п stacking interactions with aromatic compounds seen in biomolecules or pharmaceuticals. Finally, while the hydrophobic character of pure graphene is indicated by its water contact angle of 95°–100°, medicinal substances may potentially create hydrophobic connections with graphene through van der Waals interactions. Owing to graphene’s strong hydrophobicity, it is difficult to disperse it in aqueous environments, necessitating the addition of stabilizing agents such as surfactants to prevent clumping in biological fluids (Goenka et al., 2014). The hydrophobic unaltered regions of graphene that are preserved by GO can form п-п interactions that are suitable for drug loading and non-covalent functionalization. Nonetheless, it may be claimed that GO has a higher loading potency as it has more hydroxyl and epoxide moieties that can interact weakly with other groups of medicinal drugs and create hydrogen bonds with them. As additional oxygen functionalized entities in GO are ionized at specific pH levels (for instance, at pH values >4.5, carboxyl FGs are negatively charged), GO also exhibits an amphiphilic character. The reactivity of GO is increased by the occurrence of ionizable moieties and negative charges because more electrostatic interactions with therapeutic substances can be generated. Additionally, charged groups decrease GO’s water contact angle to 30.7°, increasing aqueous dissolvability and, as a result, colloidal stability (Zhao et al., 2017a). As opposed to graphene, rGO has more flaws that occur during the oxygen removal process in GO, rendering it more hydrophobic and less reactive than GO.
3.3 Thermal properties
Graphene has high thermal and electrical conductivity due to the п-п bonds beneath and above the carbon atomic plane. In actuality, the C atom typically has 4 ēs available for reactions; however, in graphene, each atom is given one unbound ē that may move randomly across the crystal framework, resulting in exceptionally good TC (TC) (Balandin et al., 2008). Therefore, it has been noted that defect-free graphene has a TC of between 4,500 and 5200 W/mK (Balandin et al., 2008). Manufacturing flaws in GO and rGO break the sp2 orbitals of graphene and add a lot of outer layer moieties that block heat transport, lowering the TC of these GBNs (Goenka et al., 2014; Zhao et al., 2017a). Even though increased TC has been useful for many purposes, it is not necessary for all of them. Offering greater thermal insulation features, such as flame retardants and in-house insulation, might be advantageous in some circumstances. It has been found in several recent studies that GO is a useful filler that can improve the flame-retardant qualities of several PNCs. To create superinsulation flame retardant foam, the scientists tried to create CNFs by combining GO oxide and sepiolite clay nanorods. The TC of the produced films has been observed to be 15 mW m-1K−1 (Zhang et al., 2016). For various application needs, maintaining heat conductivity has therefore been crucial for GO materials.
4 Functional modifications of graphene-based NMs
Owing to their superior performance, GBNs have seen extensive use; nonetheless, unaltered GBNs still have several drawbacks. For instance, graphene is extremely hydrophobic, which harms how well it disperses in water. Owing to the charge-defensive properties of the surface moieties, GO tends to assemble in the physiological environment (Jiang et al., 2020). GO has a potent protein-adsorption action and is quickly identified and absorbed in living tissue by macrophages, resulting in inflammation (Kumari et al., 2020). Additionally, in biomedical applications, GBNs lack in vivo targeted, delayed, and controlled release capabilities. These flaws collectively restrict the use of GBNs in several domains, especially in biomedicine. The functional modulation by the outermost layers functional of GBNs is a significant method for improving their biological functions as it promotes the stability and water solubilization of GBNs and offers them advanced features such as directed, slow, and controlled release. Covalent and non-covalent alterations make up the majority of surface changes nowadays.
Productive double bonds, polymeric materials, and characteristic FGs are just a few examples of the groups that can be added to the surface of GBNs through covalent modification. Amidate, free radicals, and other chemical methods are used in acidic environments to interact chemically with the active surface FGs of GBNs and produce covalent bonds, which subsequently confer the required activities. Owing to the plentiful FGs that contain oxygen and their potential for covalent modification, GO is the primary method employed to modify GBNs. The possibility of using GBNs for drug delivery, imaging, and diagnostics is increased by covalent modification, which results in fewer electron networks. Additionally, the structure of GO may change in a high-acid environment, improving its physical and chemical properties. Utilizing free radical interactions, Peng and coworkers created a functionalized styrene copolymer alteration of graphene (Peng et al., 2017). The outcomes showed that the distribution and conducting properties of graphene were appreciably improved by free radical transplantation via polymerization. Covalent alteration of GBNs may also lead to a faster and more efficient release of drugs at the malignant tumor site, leading to more precise and effective therapy. GBNs that react to stimuli include those that are glutathione, light, heat, and pH responsive. Additionally, small molecules can be used to covalently functionalize GO, leading to new molecular identification techniques for the creation of GBN-targeted formulations. The manufactured Fa-GO may maintain enduring uniform diffusion and steadiness in physiological solutions by covalently grafting folic acid (Fa) to active GO using SO3H units, for instance. Additionally, the drugs loaded on GO can be directed at tumor sites exaggerating folate receptors. To create the multifunctional GO that is so vitally needed in biomedical sectors such as drug delivery, biosensing, imaging, tissue engineering, and photo-thermal therapy, this covalent version of GO is especially appropriate for coupling with biomolecules (nucleic acids and others).
On the other hand, the non-covalent modification strategy relies on non-covalent forces to achieve the goal, including ionic and hydrogen bond, van der Waals, п-п, electrostatic, and coordination interactions between the changed moieties and GBNs (Li and Papadakis, 2020). Interestingly, the units of GBNs have a very high degree of aversion to H2O molecules, with clear van der Waals bonds and п-п layering, which makes the requirements for the non-covalent transformation of these molecules quite simple. In general, GBNs can undergo non-covalent modification through surface absorption or polymer/biomacromolecule encapsulation. The capabilities of GBNs for dispersion, safety, reactive activation, and biosensors are improved by non-covalent modification. Non-covalent modification does not create chemical bonds; therefore, it has a weaker force of modification, less stability, and is more susceptible to environmental influences than covalent alteration. As a result, in vitro and in vivo non-covalently modified GBNs are less stable. The active unit or architecture on the outer layer of GBNs is not compromised, and the structure and characteristics of the GBNs are completely preserved. GO (PVA-GO) was functionalized by Chen et al. using covalent and non-covalent methods (Chen et al., 2018). The findings revealed that compared with covalently altered PVA-GO, non-covalently modified PVA-GO had fewer strata and a lower defect concentration while still maintaining all of graphene’s inherent properties. As it can adsorb organic and inorganic components, п-п bonding is the most efficient non-covalent alteration technique. According to a previous study, gold nanoparticles, naphthalene, phenanthrene, and porphyrin complexes with amantane grafts can all form non-covalent bonds with graphene and GO (Sun et al., 2019). By generating stable GO colloidal suspension, which has the potential to be engaged as a carrier substance for biomedical purposes, the active units on GO mix with other functional moieties quickly and efficiently without the presence of contaminants.
5 Removal of water contaminants by graphene-based nanomaterials
Because of their exceptional structural and functional characteristics, GBNs are used in a huge range of cutting-edge applications (Yang et al., 2017b). The intensive study of graphene over the past few decades has led to its widespread use in industries ranging from aircraft to agriculture. Owing to the numerous applications of NMs based on graphene, many areas of research have undergone a revolution. It has piqued a broad array of attentiveness and acknowledgment primarily because of its promising prospective applications in fields of research such as metal-eradication sensors and nuclear waste optimization (Jaiswal et al., 2018). The quantity of pollutants discharged into the environment has dramatically increased as a consequence of the rapid population rise and strengthening of agricultural and industrial operations. These extremely diversified pollutants pose a stern threat to environment and general health (Adel et al., 2022). As a result, there is an international effort underway to create reliable technologies that can efficiently remove toxins from the air and H2O. Adsorption is a rapid, low-cost, and efficient technique for removing toxins from aquatic habitats among these approaches (Tara et al., 2021). Through physicochemical interactions, the contaminant (adsorbate) is bound to the nanomaterial (adsorbent) during the adsorption process. The use of graphene-based materials has sparked studies at the intersections of many fields, notably environmental restoration, as shown in Figure 6.
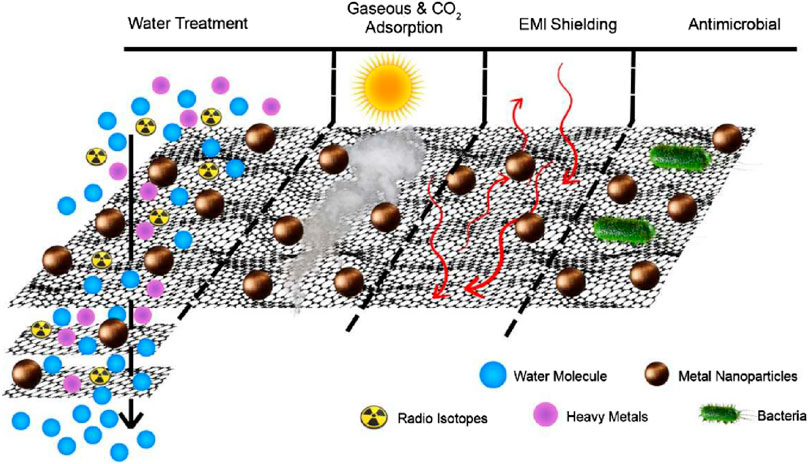
FIGURE 6. Diagrammatic representation of graphene oxide hybrids used in water filtration for environmental applications (Joel and Lujanienė, 2022).
GBNs have several π bonds, and GO has numerous FGs with oxygen that are helpful. Every material based on graphene has a large surface area. Five possible interactions might occur when GBNs are adsorbed together: hydrophobic, electrostatic, covalent, and hydrogen bonding (Zhu et al., 2010). Graphene-based materials work well as adsorbents for a variety of waterborne contaminants. In this section, we have briefly elucidated the use of NMs based on graphene as adsorbents to confiscate inorganic and organic contaminants from H2O, as elucidated below.
5.1 Removal of heavy metals
Industrialization has led to a rise in WW discharge. Metals are typical pollutants that may unintentionally contaminate drinking water supplies and aquatic ecosystems as a consequence of anthropological actions, including mining and industrial waste generation, soldered joints, and plumbing material corrosion (Assad and Kumar, 2021; Assad et al., 2023a; Assad and Kumar, 2023; Assad et al., 2023b; Assad et al., 2024). The presence of heavy metals in water can be hazardous to human health as well as negatively impact aquatic life (Yu et al., 2019). Heavy metals do not biodegrade like organic substances. Industrial effluent contains a variety of pollutants, including cobalt, chromium, zinc, lead, mercury, arsenic, and cadmium (Wang et al., 2021). These metals have the potential to be harmful and carcinogenic to living things, even in small amounts (Priyadharshini et al., 2022). Consequently, there is an increasing interest in reducing the amount of hazardous metals in aqueous medium (Zhan et al., 2019). Metals and organic molecules have been eliminated using a variety of physical and chemical techniques, as previously mentioned (Zhu et al., 2010). These techniques, however, have poor removal efficiency. The adsorption process is the most popular approach to treating water as it is inexpensive, simple to use, effective, and environmentally benign. Additionally, adsorbents can be recycled via the desorption process (Elsagh et al., 2017). Furthermore, adsorption does not result in the creation of toxic materials (Ahmaruzzaman, 2011). Using GBNs as adsorbents may have several benefits. First, two basal planes in single-layered graphene materials are available for the adsorption of pollutants. By contrast, the adsorbates cannot access the inner walls of CNTs. Second, without the need for complicated machinery or metallic catalysts, GO and rGO can be produced simply through the chemical exfoliation of graphite (Zhao et al., 2014). There are no catalyst remnants in the finished graphene material; therefore, no additional purifying procedures are required. Concerning GO, no extra acid treatments are necessary to give the material a hydrophilic character and reactivity because it already has a significant amount of oxygen-comprising functional moieties. Given that those FGs probably cause metal ions to adhere to GO sheets, this is a huge advantage. Numerous studies have discussed the use of GBNs as adsorbents to confiscate inorganic species from water. For the majority of these investigations, GO was used as a model adsorbent to eliminate metallic ions from H2O (Sitko et al., 2013). Because GO has a higher concentration of oxygen groups that can communicate with metallic ions than pristine graphene, GO is preferred for metal ion adsorption. The effectiveness of Pb (II) adsorption on unoxidized and oxidized graphene sheets was compared to highlight the significance of these oxygen-comprising functional units. To incorporate oxygen functional units, pristine graphene was initially processed using a vacuum-promoted low-temperature exfoliation. This was shadowed by heat treatments (GNS500 and GNS700) at 500°C and 700°C. In comparison with pristine graphene, the abovementioned structures displayed a greater capacity for adsorbing divalent lead ions, which emphasizes the significance of carboxyl units in the adsorption procedure of lead ions (Huang et al., 2011). The adsorption efficiency of GO was discovered to be influenced by several variables, including the strength of the ions, pH, GO layer count, and the occurrence of natural organic matter. Recently, Tan et al. (2015) created GO membranes, and they were employed as adsorbents to remove Cu (II), Cd (II), and Ni (II) with the highest adsorption capabilities of 72.6, 83.8, and 62.3 mg/g, respectively. The greater interlayer spacing of the GO membranes allowed the adsorption to achieve an equilibrium state faster (10–15 min), which is advantageous for promoting the interstitial diffusion of HMIs to functional sites. Over six regeneration cycles of the GO membranes resulted in a minor reduction in their adsorption capability (Tan et al., 2015). Zhao and coworkers revealed that at pH 6.0, copper and cadmium divalent ions were successfully adsorbed by GO sheets (Zhao et al., 2011), whereas Sitko et al. verified the elimination of copper, zinc, cadmium, and lead divalent ions at pH 5.0 (Sitko et al., 2013). Yari et al. (2016) employed GO as an adsorbent to remove Pb (II). The equilibrium time of adsorption on the GO surface was 60 min in this experiment. In this study, the adsorption capacity (AC) of Pb (II) on the GO surface developed in proportion to ambient temperature, i.e., when the temperature rose from 288 to 308 K, the AC increased from 15.9 to 19.7 mg/g. The endothermic character of Pb (II) adsorption on GO is demonstrated by this result. Higher temperatures hence promote adsorption. GO provided a ΔH° value of 22.70 (kJ/mol). This suggests that Pb (II) was adsorbed physicaly on the GO surface. The results of a thermodynamic investigation showed that Pb (II) ion adsorption on the surface of GO was endothermic and spontaneous (Yari et al., 2016). Moreover, the pHpzc (pzc, point of zero charge) of GO in aqueous solution controls how it behaves. The GO surface is negatively charged when pHpzc < solution pH due to the proton removal from carboxyl and hydroxyl fragments. The electrostatic interaction with positively charged metal ions is more advantageous when the GO outer layer is negatively charged, improving AC. The most popular method for creating GBNs (composites) for the elimination of metallic ions is the conjugation of graphene with magnetic NPs, such as Fe or iron oxide (Gollavelli et al., 2013). The elimination of anionic contaminants from aqueous systems, like phosphate (PO4−), perchlorate (ClO4−), and fluoride (Kamangerpour et al., 2002), has also been examined using GBNs, although the preponderance of reports focus on the adsorption of cationic metallic ions (Shi et al., 2012). The mechanism of anion adsorption, such as that of halide ions, was traditionally attributed to anion interactions rather than the immobilization of cationic metal species. The basis for this anion association is the interaction between the anion (or lone electron pair) and an aromatic framework on the graphene sheet that is electron deficient. Additionally, Alejandro presents findings on the synthesis of an aerogel incorporating reduced graphene oxide (rGO) and polyethylenimine (PEI) using a supercritical CO2 method (Borrás et al., 2022). The synthesized rGO/PEI aerogel demonstrates high efficiency as a sorbent for treating Hg (II)-contaminated water. Sorption tests show the rapid removal of Hg (II) from water, achieving residual concentrations as low as 3.5 μg L−1 in a short period, nearing the legal limits for drinking water. The aerogel displays a remarkable maximum sorption capacity of approximately 219 mg g−1 for Hg (II), making it a promising candidate for treating Hg (II) contaminated wastewater. Based on the analysis of surface charge, Figure 7 schematically illustrates the potential interactions involved in the removal of Hg (II).
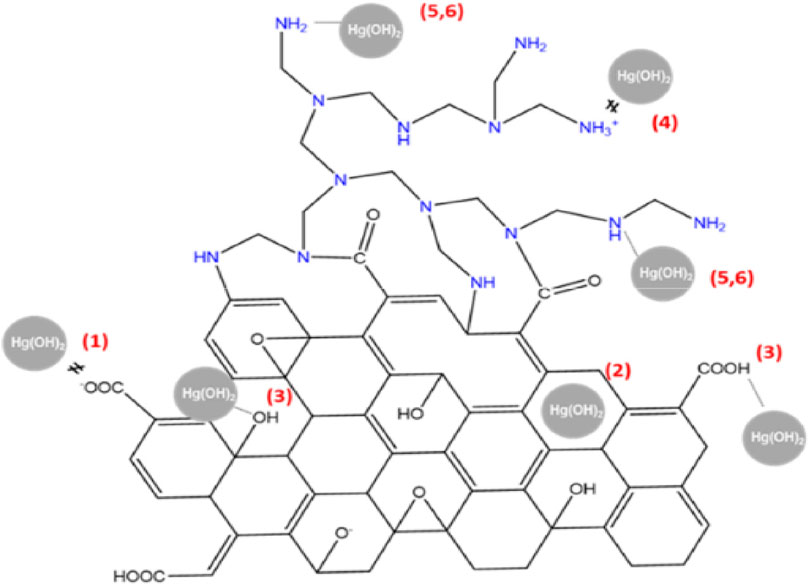
FIGURE 7. Schematic representation of the potential interactions involved in the removal of Hg (II) (Borrás et al., 2022).
Still, there are undoubtedly challenges with the way that heavy metals are now treated in both industrial effluent and point-of-use water. Current methods typically face constraints and difficulties when it comes to naturally removing certain heavy metals from point-of-use water. The heavy metals in industrial effluent are precipitated as sludge that requires additional treatment, which greatly reduces the value of the metals. Using a GO-modified carbon felt (CF/GO) electrode, an electrochemical method has been devised that can handle heavy metal pollution at low and high concentrations. The methodology uses both direct-current (dc) and alternating-current (ac) electrodeposition (ED) (Liu et al., 2019). Because of the high density of surface FGs in GO, which allows for electrodeposition with >29 g of heavy metal per 1 g of GO, the ensuing AC is two orders of magnitude greater than that of traditional adsorption methods. When used on point-of-use water with low levels of heavy-metal contamination, Dc ED with a CF/GO anode may minimize contamination from single HMIs (Cu, Pb, and Cd) and multiple-ion composites to levels considered safe for human consumption. As with conventional adsorption techniques, this approach can handle a broad array of HM pollutants in point-of-use water. The study’s findings indicate that dc ED is capable of recovering over 99.9% of HMIs from industrial WWs that have high levels of pollution (Liu et al., 2019). Furthermore, the ED technique may selectively recover Cu, Pb, and Cd separately by adjusting the voltage and ac frequency; this increases the elimination of HMs. Table 1 provides a comprehensive overview of significant studies investigating the efficacy of GBNs as adsorbents for the removal of heavy metals from water.
5.2 Removal of volatile organic compounds
According to the WHO, different types of volatile organic compounds (VOCs) are to blame for the rise in sarcoma rates among individuals worldwide (Fraga et al., 2019). One VOC and the main indoor air contaminant linked to sick building syndrome is formaldehyde, which is found in varnish and other decorating supplies. Recent studies on VOC eradication through the sorption process and PC degradation have used GBNs, particularly GO, to minimize the damage that VOCs cause to humans and the surroundings (Wang et al., 2013). Owing to the oxygen FGs on its outer-layer, GO is probably going to have a lower hydrophobicity than rGO and pristine graphene. As an outcome, GO might have a lower ability for adsorbing aromatic VOCs than the abovementioned analogs. For instance, rGO and GO were revealed to have adsorption capabilities of 276.4 and 216.2 mg g-1, respectively, in an ongoing flow reactor with an initial loading of 50 ppm C6H6. In comparison with GO, rGO can play a major part in boosting the adsorption capability of aromatic VOCs due to its hydrophobic nature and higher inclination to form π–π bonds. In addition to hydrophobicity, it was hypothesized that the superior adsorptive functioning of rGO was a result of its larger surface area compared with GO. It was discovered that rGO and GO had surface areas of 292.6 and 236.4 m2 g-1, respectively (Yu et al., 2018). Toluene removal tests have also been conducted on the GO and rGO. When demonstrating the desired adsorption of toluene on their outer layers, the ability of GO and rGO to demonstrate π–π bonds, hydrophobic interactions, and electrostatic attraction with toluene can be helpful. Three different forms of GBN-graphene platelets (GP), rGOMW, and KOH were mentioned in a study. Toluene adsorption tests on activated rGOMW (rGOMWKOH) were conducted, and the results obtained contrasted with AC (Kim et al., 2018b) (it is noteworthy that the air conditioner used in this instance is often used as a marketable air conditioner adsorption filter). In removing the toluene, the adsorption capabilities of these GBNs were 2, 7, and 14.4 mg g-1 for GP, rGOMW, and rGOMWKOH respectively, and were ordered as follows:
Additionally, it was noted that the composite of GO and MOF-5 was effective at removing benzene gas, with an elimination capability of 251 mg g-1 (Liu et al., 2015a). Because there were thought to be weak and non-selective adsorption dynamisms between tiny molecules and MOFs, it was hypothesized that, despite their great porosity, MOFs were unable to hold onto tiny molecules in ambient settings. The aforementioned issues in holding small molecules were resolved by combining graphene-based materials with MOFs. In this context, different amounts of GO, such as 1.75, 3.5, 5.25, and 7 wt%, were used to construct the GO/MOF-5 composite. From all of these composites, the one made with 5.25 weight % GO had the maximum SA and volume of pore among the evaluated GO and MOF-5 ratios, making it the best responder in terms of benzene removal capability. Aliphatic VOCs, particularly n-hexane, are commonly released into the environment (Sun et al., 2014). The industries that typically use n-hexane are those that produce shoes, bags, electronics, foodstuffs, lubricant extraction, and chemicals. Adsorption of n-hexane is typically regarded as a secure, quick, and affordable way of mitigating it. It was discovered that using a GO/MIL-101 composite was a virtuous process to erase n-hexane from the atmosphere. Despite the excellent n-hexane elimination performance of graphene-based composites, few studies have been conducted in this field. RCHO and RCOR are the chief carbonyl VOCs influencing the atmosphere. GBNs have also been successfully employed to eliminate carbonyl VOCs. An amino-functionalized graphene aerogel was employed to remove gaseous formaldehyde in a study, both in its pure usage and as a compound with CNTs (Wu et al., 2017a). Chemical and physical adsorption techniques were used to bind formaldehyde to the aforesaid amino-functionalized graphene sheets. The van der Waals interactions via amino and carbonyl moieties of CH2O were principally accountable for the chemical adsorption process. CNTs supported the graphene layers in the CNT-adjusted amino-functionalized graphene aerogel (GN/E), which decreased the pore diameter. The adsorptive functioning of GBNs has also been investigated for ketonic VOCs (acetone and butanone) in addition to aldehydes (Zhou et al., 2014; Guo et al., 2016).
Generally, the elimination of various VOCs showed excellent promise when using graphene materials both on their own and in combination with other strong structures. According to several studies, these GBNs are far superior to traditional adsorbents like AC and zeolites. Nonetheless, experimental variables like high or low partial pressures of the target gaseous molecules may drastically change how well the adsorbent material performs. It is noteworthy to mention that some adsorbents function exceptionally well under controlled circumstances. In more real-world settings, in which the dosage of the target contaminant is lower than in an experiment, these NMs, can function very badly. To eliminate the systematic bias in such concerns, it is crucial to evaluate the effectiveness of adsorbents by using appropriate metrics (including the partition coefficient (PC)) to prevent these difficulties (Szulejko et al., 2019).
5.3 Removal of antibiotics
Pharmaceutical medications are a class of organic pollutants that detrimentally impact public health and the atmosphere. Between 30% and 90% of these substances are still not degradable and are ejected as active molecules in the surroundings, even at trace levels (Siddiqui and Chaudhry, 2018). The application of GBNs for the adsorption of antibiotics has demonstrated potential in research (Zhao et al., 2020). The adherence of organic materials on the interface of GBNs is thought to be caused by five different possible interactions, comprising hydrophobic effects, π-π- stacking, H-bonds, covalent contacts, and electrostatic relations (Kim et al., 2018a). When used as an adsorbent, graphene has great qualities for removing antibiotics. As graphene is composed of just one C-sheet, all of its atoms are easily contacted by antibiotics due to their exposure to their surroundings on both sides (mostly through a π-π interaction). Second, the porous shape and large surface area of graphene adsorbents, as compared with conventional adsorbents, make them a prime option for antibiotic surface reactions or quicker diffusion, resulting in efficient and rapid adsorption (Perreault et al., 2015). Third, the cost of producing graphene adsorbents on a wide scale is less expensive than that of other high-performance adsorbents (such as carboxyl multi-walled carbon nanotubes and single-walled carbon nanotubes) with an equivalent antibiotic AC. Moreover, antibiotic-polluted water and other organic and inorganic pollutants can be efficaciously remediated using GBNs, as shown in Figure 8.
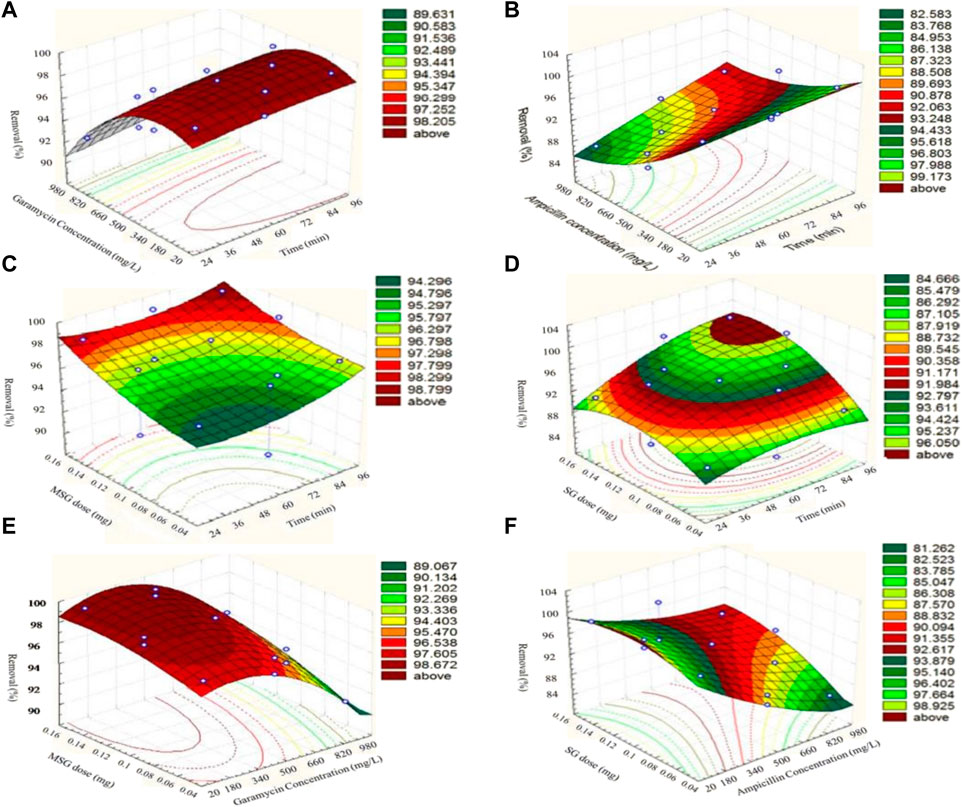
FIGURE 8. Surface plots representing the removal efficiency (%) of antibiotics (garamycin and ampicillin) on magnetic-functionalized graphene nanocomposites (MSG and SG, respectively) (Elessawy et al., 2020). (A, C, E) represent Surface plots of response for removal efficiency (%) of Garamycin on MSG, (B, D, F) represent Surface plots of response for removal efficiency (%) of Ampicillin on SG.
Despite significant advancements in graphene adsorbent technology, many intrinsic drawbacks remain to be addressed. Surface hydrophobicity and facile agglomeration in aqueous solution are graphene’s primary disadvantages, which significantly reduce the material’s adsorption capability in real-world applications (Bai et al., 2015). Thus, the development of functionalized graphene is required to address these limitations (Kumar et al., 2017). Chemically modified graphene has the potential to generate oxygen-containing groups linked to its carbon backbone, improving its dispersion and resulting in a homogenous aqueous suspension. Two significant GBNP branches created by chemically altering graphene are rGO and GO. Research has used GO and rGO as adsorbents in numerous experiments to retrieve several antibiotics from water-based solutions (Rostamian and Behnejad, 2016). According to Chen et al. (2015), GO efficiently adsorbs SMX and CIP at pH 5.0, with maximum sorption capacities of 240 and 379 mg/g, respectively. Liu et al. looked into the adsorption of two sulfonamide pharmaceuticals from water using two rGOs (Liu et al., 2016). These experimental findings indicate that GO and rGO have a great deal of potential for absorbing various antibiotics.
Owing to the existence of functional moieties (such as ●OH and ●O2-) created by photocatalysts, antibiotics can be successfully degraded or reduced into non-hazardous tiny molecular entities under sunlight, VL (VL), or UV light in addition to adsorption (Li et al., 2016a). Thus, PC degradation is among the most well-liked, productive, and eco-friendly techniques for eliminating environmental contamination caused by antibiotics. Its high electrical conductivity, low manufacturing cost for large-scale manufacturing operations, and specific surface area for even distribution, the quick transmission of ēs, and narrow band-gap energies, have made graphene a potentially lucrative photocatalyst that has been comprehensively explored for the PC breakdown of antibiotic pollutants in water. However, studies have shown that GO cannot function in the VL area because its band gap is just 1.79 eV (Anirudhan et al., 2017), and it easily loses its catalytic properties during the self-aggregate procedure between the layers of graphene (Julkapli and Bagheri, 2015). Consequently, to get around these problems and increase the catalytic activity of antibiotics, graphene is frequently mixed with other photocatalysts to create innovative graphene-based photo-catalysts (GBPs). Various efforts (including the use of different semiconductors) have been made to create and manufacture GBPs in the last few years to increase the degradation capacity of antibiotic pollutants. Antennas based on graphene-based nanosheets are an effective solution for mitigating the many drawbacks of individual semiconductors. Rich surface FGs and a large specific SA of graphene materials may be linked to their strong PC qualities, which help to increase adsorption efficiency. Furthermore, combining different graphene materials may hasten the electron transport and separate photo-induced electron-hole pairs more quickly. The rGO/Bi2WO6 composites were produced by Anirudhan et al. (2017) and used to remove CIP in a VL simulation. The rGO/Bi2WO6 composites demonstrated an exceptional VL-driven PC degradation rate of CIP (89.2%). This was most likely caused by the rGO loading, which decreased the rate of electron-hole recombination while simultaneously increasing adsorption and catalytic sites. The produced ē could be effectively transferred from the CB of Bi2WO6 to rGO under VL irradiation, prolonging the lifespan of photo-excited ē/h+ couples. This portion of the electrons could come into touch with the O2 present in the photodegradation system at the same time and react swiftly to create ●O2− groups. The ●O2− and h+ produced by photolysis can react further in water to break down CIP molecules. This study demonstrated that adding Bi2WO6 to rGO as a photocatalyst could effectively increase the PC activity of CIP degradation under VL by ensuring higher electronic conductivity, accelerating the separation of photo-induced electron-hole pairs, and prolonging the electron transfer. Currently, an increasing number of studies have concentrated on linked semiconductor materials in conjunction with graphene, which typically offers the major benefits of encouraging electron-hole pair separation and preserving the reduction and oxidation events at two distinct reaction sites (Tang et al., 2015). For example, the coupling of other semiconductors (ZnS, ZnO, etc.) with CdS-graphene composites has garnered a lot of interest because of its benefits, which include increasing charge separation, extending the life of the charge carrier, and increasing the effectiveness of charge transmission (Huo et al., 2016). Furthermore, an Ag3PO4/BiVO4/rGO heterojunction photocatalyst was successfully prepared by Chen et al. (2017) using a simple in situ deposition method. The catalyst demonstrated 90% removal effectiveness toward TC under VL irradiation, which was significantly higher than that of pure BiVO4 (56%), Ag3PO4/BiVO4 (82%), and rGO/BiVO4 (78%). Compared with single semiconductors or single semi-conductor/graphene composites, the PC activity of coupled semiconductor/graphene composites can be significantly increased. Therefore, creating a novel coupled semiconductor/graphene multi-component photocatalyst and investigating the processes of component interaction are crucial for the removal of antibiotics.
Additionally, by combining the benefits of graphene structure with these materials, other methods such as combinations with other functional nanoparticles, polymers, or optical fibers can also operate as an extremely effective composite substance for eliminating various antibiotics (Cao et al., 2016; Anirudhan and Deepa, 2017; Lin et al., 2017b). These substances could substantially improve the photocatalytic effectiveness for antibiotic elimination by improving the surface area, responsiveness, stability, active sites, charge separation and transfer, and reaction of the photocatalyst. Lin et al. created TiO2-rGO to eradicate SMX pollution in water using an immobilized photoreactor and catalyst-coated side-glowing optical fibers (SOFs). Higher light utilization efficiency was achieved with SOFs by promoting light transmission directly through the inner fiber cores to the photocatalysts coated on the surface and the outer surface of the photocatalysts. This was a cost-effective way to transfer photons in big reactors evenly and efficiently without having to separate the photocatalysts from the water (Lin et al., 2017a). Table 2 provides a comprehensive overview of significant studies investigating the efficacy of GBNs as adsorbents for the removal of antibiotics from water.
Although graphene-based photocatalysts can photodegrade antibiotics, there is currently very little research regarding the application of graphene-based photocatalysts for antibiotic removal and the photodegradation of antibiotics in combination with other materials. Thus, it is crucial to investigate the possibilities of these materials in photocatalysis.
5.4 Persistent organic compounds
Persistent organic compounds (POCs) constitute a class of organic chemicals characterized by their resistance to environmental degradation, stemming from their chemical stability and resistance to breakdown processes (Radjenovic and Sedlak, 2015; Mantovani et al., 2023). Examples include polychlorinated biphenyls (PCBs), dioxins, furans, organochlorine pesticides, poly-brominated diphenyl ethers (PBDEs), and per- and poly-fluoro-alkyl substances (PFAS) (Schulze et al., 2019; Gagliano et al., 2020; Podder et al., 2021). POCs, which are prevalent due to past industrial use, waste incineration, and certain consumer products, pose environmental and human health risks. Their persistence in ecosystems, ability to bio-accumulate, and potential long-range transport underscore the need for global regulatory efforts to mitigate their impact on environmental integrity and public health. Graphene’s unique properties (Khaliha et al., 2021; Mantovani et al., 2021; Lombardi et al., 2022) make it an ideal candidate for adsorption and catalysis in the removal of POCs from water. Graphene oxide (GO) and reduced graphene oxide (rGO), which are derivatives of graphene, exhibit increased adsorption capabilities due to their functional groups and large specific surface area (Mantovani et al., 2022; Mantovani et al., 2023). These nanomaterials can effectively capture POCs through π-π stacking, hydrogen bonding, and other interactions. Additionally, the incorporation of other nanoparticles onto graphene surfaces can further increase their adsorption capacities. The efficient regeneration of graphene-based adsorbents adds to their appeal for long-term use. Thus, the utilization of graphene-based nanomaterials for the removal of POCs has emerged as a promising avenue in environmental remediation. For instance, Ren et al. investigated the adsorption behavior of six polychlorinated biphenyl (PCB) congeners using pristine graphene (GN), graphene oxide (GO), and sulfonated graphene (SG) at environmentally relevant concentrations ranging from picograms to micrograms per liter (Ren et al., 2019). GN demonstrated superior adsorption capacities to GO and SG, which was primarily attributed to increased surface adsorption. A conspicuous planarity effect was observed in the adsorption of PCB congeners on all graphene nanomaterials at low aqueous concentrations (0.01–10 ng L−1), which diminished as the concentration increased. Notably, functionalized graphene, especially SG, exhibited a more pronounced planarity effect than pristine GN, particularly at lower concentrations. Under acidic or alkaline conditions, the planarity effect on GO was attenuated due to the preferential dispersion of GO particles in the solution. However, the planarity effect on SG remained minimally impacted by changes in the pH of the solution. Lower temperatures increased the planarity effect in the adsorption of PCBs on both functionalized graphene materials, with SG displaying a lower increase than GO. Conversely, elevated temperatures resulted in the suppression of the planarity effect. Additionally, a boron-doped graphene sponge anode was synthesized and employed by Nick et al., for the electrochemical oxidation of C4-C8 per- and poly-fluoroalkyl substances (PFASs) (Duinslaeger and Radjenovic, 2022). Operating in low conductivity electrolyte and one-pass flow-through mode, removal efficiencies ranged from 16.7% to 67% at an anodic current density of 230 A m−2, with an energy consumption of 10.1 ± 0.7 kWh m−3. The removal mechanisms included electrosorption (ES) (7.4%–35%) and electro-oxidation (9.3%–32%). Defluorination efficiencies for C4-C8 per-fluoro-alkyl sulfonates and acids ranged from 8% to 24%, with efficient fluoride recovery (74%–87%) suggesting effective C-F bond cleavage. Stoichiometric sulfate recoveries (91%–98%) indicated the proficient cleavage of sulfonate head-groups. Adsorbable organic fluoride analysis revealed the ES of remaining partially defluorinated byproducts during the current application, which were released into the solution after current cessation. This proof-of-concept study highlights the graphene sponge anode’s capability for C-F bond cleavage and defluorination of PFAS, offering the potential for the electrochemical degradation of PFAS-laden wastewaters and brines due to the anode’s electrochemical inertness toward chloride and absence of chlorate and perchlorate formation, even in brackish solutions. Moreover, graphene nanosheets and nanoplatelet-alginate composite hydrogels were synthesized by Francesca et al., through ionic gelation for the removal of emerging contaminants (ECs) from tap water (Tunioli et al., 2023). The resulting gel beads exhibited a porous structure with a uniform distribution of graphene materials on pore surfaces. The adsorption kinetics of graphene-related materials (GRMs) were notably faster than granular activated carbon (GAC), a benchmark industrial sorbent, with an ofloxacin removal capacity 2.9 to 4.3 times higher. Confocal Raman microscopy mapping and SEM confirmed the gel bead structure and GRM distribution. Adsorption isotherm studies, as shown in Figure 9, revealed a high maximum adsorption capacity of 178 mg/g for rhodamine B, which was comparable with powered activated carbon.
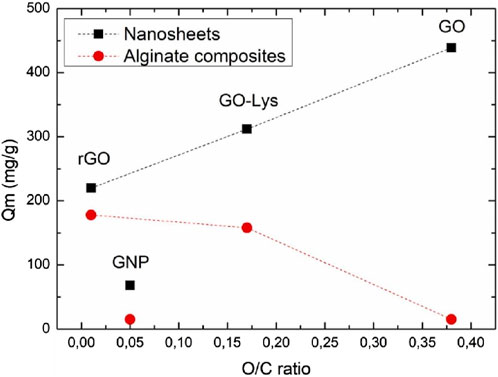
FIGURE 9. Monolayer adsorption capacity (
Regeneration tests, as shown in Figure 10, demonstrated the resilience of the gel beads, maintaining adsorption performance even after saturation and washing with ethanol. Repeated reuse cycles up to the fourth cycle showed no significant loss of adsorption efficiency. These findings highlight the potential of graphene-based composite hydrogels for effective EC removal, including bisphenol A, ofloxacin, and diclofenac, from tap water. The study suggests the promising application of these materials in water treatment, offering advantages in terms of both adsorption kinetics and recyclability.
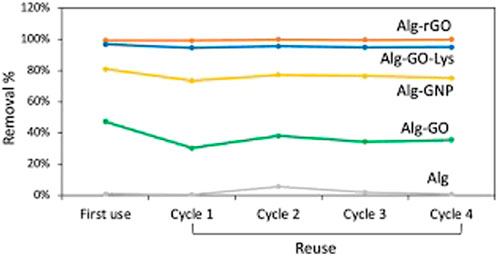
FIGURE 10. Regeneration test of alginate and alginate-graphene beads (Tunioli et al., 2023).
Overall, the application of graphene-based nanomaterials in POC removal showcases a promising and sustainable approach to addressing environmental pollution challenges. Ongoing research continues to explore and optimize these materials for practical and large-scale remediation applications.
5.5 Other contaminants
Surface area and pore size distribution are the main surface characteristics that affect adsorption on graphene. Although there is no porosity, pristine graphene possesses an extremely high specific surface area. Porosity can be added to graphene to significantly increase its adsorption efficacy by mixing it with other porous materials, such as silica, chitosan, and gelatin (Lin et al., 2017a; Fan et al., 2019). By adding various functional groups, good adsorption performance can also be attained. The formation of bonds with the adsorbate is facilitated by these functional groups. The example of GO and rGO, which have many oxygen-containing functions that can form bonds with the adsorbate, makes this quite visible. Consequently, the successful application of functionalized graphene-based nanocomposites in environmental remediation depends on their ability to be fabricated with a wide surface area, high porosity, and oxygen-containing functionalities, as demonstrated in section 4. As this section discusses, graphene-based adsorbents have been used to remove a variety of organic contaminants thus far, but with many changes made to the original graphene structure.
Using graphene-based materials to remove aromatic polycyclic polar and non-polar chemicals has been reported in multiple cases. The adsorptive removal of polar and non-polar PAHS is facilitated by the π-π interactions, hydrophobic effect, and van der Waals forces between graphene-based adsorbents and PAHS. Colloidal graphene oxide nanoparticles (GONPs) were used to study the adsorptive characteristics of a variety of aromatic compounds, including polar (1-naphthylamine and 1-naphthol) and non-polar (PYR, PNT, NAP, and DCB) molecules. This research was conducted by Wang et al. (2014). The strong hydrophobicity of PYR and PN was the cause of their high adsorption affinities. The adsorption affinity order for the hydrophobic effect was determined by normalizing the adsorption data, and it was PYR > PNT > NAP > DCB. In addition to the hydrophobic impact and van der Waals forces, π electron-rich PAHs and electron-depleted portions of strongly polarized graphitic surfaces communicate through π-π electron donor-acceptor (EDA+) interactions. The adsorption capacities of GONPs were increased by the π-π interactions, Lewis acid-base interactions, and H-bonding between 1-naphthylamine and 1-naphthol (Wang et al., 2014).
Just as there are several papers on PAHs, graphene-based adsorbents have also shown promise for phenolic compound removal. In-depth adsorption is influenced by the adsorbent surface area and degree of reduction. According to Wang et al. (2015), PNT and 1-naphthol significantly increase the adsorption affinities of GO nanoparticles (GONPs) in the presence of sulfide. The increased surface hydrophobicity of GO following Na2S treatment was the cause of the higher PNT adsorption. The increase in adsorption in the naphthol example resulted from the phenolic and carboxyl groups on the surface of GO converting from epoxy/ether groups, allowing for a deeper H-bonding between 1-naphthol and GONPs. Another study examined the theoretical and experimental relationships between phenol naphthol and rGO (Yu et al., 2017). Both phenol and naphthol were adsorbed on rGO, according to the pseudo-first-order model. When the pKa value was reached, the removal capacity increased along with the pH, at which point the negatively charged rGO and anionic phenols experienced electrostatic repulsions. The π-π EDA interactions caused the phenols to be adsorbed. Using the projector augmented wave (PAW) approach and the PBE functional at the GGA, the authors performed DFT. The computed Eads further supported the final closest interaction’s finding that naphthol exhibited stronger π-π interactions with the rGO plane than phenol. The adsorption of 4-CP on pure graphene (Gupta et al., 2018), GO, and N- and B-doped graphene was investigated using DFT (Cortés Arriagada et al., 2013). Because of hydrogen bonding, the 4-CP adsorption on GO was stronger than on PG, as demonstrated by a molecular dynamics simulation using the PM6 potential. Because graphene is an acceptor, the charge density distribution showed electron transport from 4-CP to graphene. However, in the case of GO, it was discovered that the dispersion and H-bonding interactions caused the oxygen functions to take an electron from graphene and then transfer it to 4-CP. The adsorption of N- and B-doped graphene was determined by the authors to be equivalent to that of PG for 4-CP. By raising the doping concentration on the surface, it is possible to somewhat increase the adsorption on N-doped graphene. Using DFT-D3 computations with the revPBE/def2-TZVP theory model, Abadee et al. (2019) recently investigated the interaction of phenol and water molecules with graphene and graphene nanobuds. As these forces have an impact on the binding behavior of the interacting species, the DFT-D3 research investigation of non-local dispersion forces is crucial in this situation. In comparison to water molecules, greater phenol adsorption was made possible by the simultaneous presence of π-π stacking and electrostatic interactions on graphene and graphene nanobuds. Because of its higher SSA, graphene nanobuds turned out to be a superior adsorbent to graphene. Another theoretical study used DFT research with GO as the adsorbent to examine the adsorption behavior and mechanism of phenol, 4-CP, 2,4-diCP, and 2,4,6-triCP (Wei et al., 2019). They used DFT-D3 simulations to account for dispersion corrections to determine the most stable geometry because the weak interactions in the sorption system have an impact on the geometry. Theoretical data led the authors to conclude that the adsorption process is primarily driven by the hydrophobic effect, H-bonding, and π-π interactions. MD simulations provided more support for this finding. Additionally, it was discovered that adsorption affinity increased with increasing numbers of hydroxyl groups on GO and decreasing numbers of chloro groups on phenols. Moreover, the presence of polar solvents and acidic environments strengthened H-bonding and electrostatic interactions.
Another significant class of organic contaminants is dyes. Dyes are released into the water by a wide range of industries, including printing, textile, dyeing, paper manufacture, tanning, and painting. The majority of colors dissolve in water and are either cationic or anionic. Most dyes have complex chemical structures, are long-lasting, and do not break down naturally. Additionally, some colors are toxic to humans. They disrupt natural cycles and present several health risks to living things (US EPA). For instance, it has been found that approximately 10% of the dyes, which are highly carcinogenic and toxic, are released into WW (Uddin et al., 2009). Furthermore, dyes change the color of water, interfering with aquatic plants' ability to photosynthesize, blocking sunlight, and creating an unbalanced aquatic ecosystem (Thakur and Kandasubramanian, 2019). Therefore, taking into account the possibility of environmental toxicity and public health harm, these dyes must be removed. Van der Waals forces, π interactions, and oxygen-containing groups cause the positively charged amino groups of dye molecules to engage electrostatically with the negatively charged surface of the adsorbate, which is how most dye removals are accomplished.
GO-hydrogel porous nanocomposites were created by Pourjavadi et al. (2016), who also investigated the impact of the hydrogel’s porosity on dye adsorption. By incorporating CaCO3 in varying concentrations and then removing it, they were able to get varied porosities. The exceedingly high porosities they discovered allowed for an exceptionally high AC. The Langmuir isotherm model (LIM) and the pseudo-second-order kinetics model provided the best description of the adsorption. To adsorb MB, Mercante et al. (2017) fabricated PMMA nanofibers wrapped with rGO (PMMA-rGO) and found that the spontaneous adsorption was driven by the π-π stacking interactions. The adsorption of MB dye was best characterized by the pseudo-second-order kinetics model and the LIM. Furthermore, Huong et al. (2018) produced magnetic manganese ferrite/GO nanocomposites to adsorb MB dye. According to their proposal, GO nanosheets are primarily involved in the interactions that cause dye molecules to adsorb, such as π-π interactions, oxygen-containing groups, and electrostatic/ionic interactions. To improve electrostatic/ionic interactions, π-π electron coupling, and other oxygen-containing functional groups, such as carboxyl, epoxy, and hydroxyl groups, they raised the concentration of GO, which in turn boosted adsorption activity. The best agreement was found between the adsorption isotherm data and kinetics and the pseudo-second order kinetics model and Langmuir isotherm model. In a different study (Wang, 2017), it was shown that magnetic graphene nanoparticles (Fe3O4@GNs) had increased MB adsorption capability upon a decrease in C=O groups. The kinetics results demonstrated that MB dye and Fe3O4@GNs undergo chemisorption through π-π interactions, as predicted by the pseudo-second-order model.
In addition to the cationic dyes previously described, graphene-based adsorbents have been widely employed in the elimination of diverse anionic dyes. GO was used by Konicki et al. (2017) to adsorb the anionic azo dyes acid orange 8 (AO8) and direct red 23 (DR23). The isotherm model approximated the Langmuir form, while the kinetics model neared pseudo-second-order kinetics. Owing to the deprotonation of the -COOH and -OH groups at basic pH, which created electrostatic repulsive forces with the sulfonate anions (RSO3−) in the dye molecules, the effectiveness of adsorption reduced with increasing pH. Apart from the electrostatic interactions, the adsorption of dyes onto GO was also facilitated by H-bonding and π-π stacking interactions. They proposed that for the DR23 ions to reach the adsorption sites, they must first (at least partially) exit the hydration shell, which necessitates energy input. As a result, for DR23 and AO8, the adsorption process is exothermic and endothermic, respectively. The two color adsorption values for ∆Gº fell within the physisorption range.
The literature makes it abundantly evident that while GO’s anionic groups and anionic dyes experience high electrostatic repulsion, GO shows significant cationic dye adsorption through the formation of electrostatic interactions. Because of the additional stacking interactions, GBNs can effectively behave as excellent adsorbents for cationic and anionic dyes. Wang et al. investigated the adsorption of a neutral dye, acridine orange (AO), in addition to cationic and anionic dyes (Wang et al., 2016). They coated a graphene oxide sheet (GO) with calcium silicate after depositing Fe3O4 nanoparticles, creating an MGSi graphene oxide composite. Because MGSi contains carboxylic groups, which give its surface a negative charge at pH values greater than 2.8, which are electrostatically attracted toward the positively charged AO, these nanocomposites could adsorb up to 193.05 mg g-1. The highest AC was attained at pH 6, and it increased as pH rose. Because AO is neutral and MGSi has a negative charge in the basic medium, the AC is reduced.
Pesticide removal is also a big concern because of the widespread use and careless application of these chemicals in drinking water. Agricultural, dairy, and insect control still use a lot of pesticides, which are organic aromatic compounds. Furthermore, herbicides have been used in veterinary treatment and home gardening. Even in extremely low concentrations, they are dangerous to living things. Because pesticides can cause neurotoxicity and cancer, and are involved in other illnesses, their routine usage is not recommended (Paramasivan et al., 2019). Moreover, acetylcholinesterase enzyme inhibitors, which cause nervous system dysfunction, are the reason that organophosphorus insecticides are hazardous (Fraga et al., 2019). Numerous research teams have also investigated employing graphene-based materials as adsorbents to remove pesticides from aqueous solutions. Strong electrostatic and π-π interactions reduce pesticide absorption. Adsorption efficiencies for ametryn, prometryn, simazine, simetone, and attrazine at pH 5°C and 25°C were reported by Boruah et al. (2017) as 93.61, 91.34, 88.58, 81.22, and 75.24%, respectively, on the Fe3O4/rGO nanocomposite. Because of the greatest electrostatic interactions and the least amount of iron leaching from the adsorbate, the AC reduced as pH increased and reached its maximum at pH 5. It was discovered that the Fe3O4/rGO nanocomposite’s oxygen-containing functions and the pesticide’s ≡N+‒ group interacted electrostatically. The most beneficial adsorption was facilitated by the strong π-π interactions. Additionally, metal-organic framework/graphene oxide hybrid nanocomposites (UiO-67/GO) were created by Yang et al. (2017a) to remove OPP hydrocarbons. According to the findings, 482.69 mg g-1 of glyphosate adsorbed at pH 4 corresponds to the Langmuir model and the pseudo-second-order kinetics model. The XPS tests demonstrated that the favorable adsorption is caused by the interaction between the phosphate group of glyphosate and the oxygen-containing FGs on the surface of UiO-67/GO. The significant increase in crude oil discoveries and the boom in petrol products have had detrimental effects on, and ultimately destroyed, many ecosystems. Furthermore, the main environmental issue that frequently arises in water or along beaches is the seepage of oil from oil drilling facilities. To lessen the negative impact on the marine ecosystem, research on the adsorption of leaky lubricants from contaminated H2O has been essential (Tsang et al., 2017). Successful recent studies on the adhesion of oil suspensions on GBNs have demonstrated strong adsorption capacities. Extremely spongy GBNs, or xerogels, have recently been created as state-of-the-art oil adsorbents; several of them include magnetic metallic nanospheres that are connected to them and characterized by high recyclability. Table 3 provides a comprehensive overview of significant studies investigating the efficacy of GBNs as adsorbents for the removal of antibiotics from water.
6 Risks of GBNs to human health and environment
NMs based on graphene have been applied in a variety of fields, including biomedicine and environmental exposures. However, even so, the level of toxicity needs to be taken into consideration if it is to be used for human and environmental applications. Understanding and categorizing GBNs according to their applications and safety requirements requires an understanding of how biological characteristics interact with them. When used in non-biomedical applications, GBNs have the potential to be harmful when exposed to the environment (Fadeel et al., 2018). Intentionally or accidently, humans come into touch with GBNs, especially those who create and live in the suburbs of an industrial manufacturing setting. GBNs, which are mostly introduced into the ecosystem through waste from industrial or pharmaceutical production, are exposed by regular people. Different organs may be impacted depending on how GBNs enter the body through biological barriers or blood flow. Owing to their nanosize, surface morphology, complexation, charge, impurities, aggregation, corona effects, and physical destructions, GBNs can pass through the blood-air, blood-brain, blood-testis, and blood-placental barriers, as well as the blood-air, blood, and placental barriers. Several cellular processes, including oxidative stress, DNA destruction, inflammatory response, localized cell death, autophagy, and necrosis, are important contributors to the toxicity of GBNs (Ou et al., 2016), as shown in Figure 11.
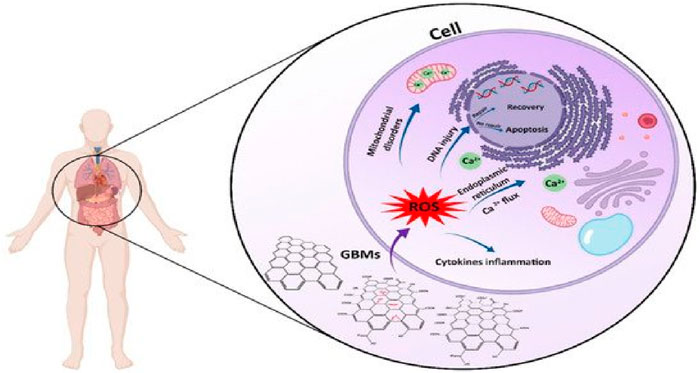
FIGURE 11. Cellular toxicity of graphene oxide-based NMs (Zare et al., 2021).
They represent a significant hazard to the aquatic ecosystem. As a result, GBNs found in soil or water are also ingested by people through a variety of methods, including through the food chain. The environmental effects of GBNs have only been briefly examined by a few researchers (Chowdhury et al., 2015). The immediate impacts of GO on the microbial population in wastewater were examined by Ahmed and Rodrigues (2013). According to their findings, GO was hazardous to microorganisms at concentrations ranging from 50 to 300 mg L-1. Because of the increase in water turbidity and decrease in sludge dewaterability, the effluent’s quality worsened. The harmful effects of GO on microbial populations were also confirmed to be caused by the creation of reactive oxygen species (ROS). Hence, it is important to comprehend how GBNs interact with one another and how harmful they are at the cellular and molecular levels. The dose, the form and chemistry of the surface, the exposure route, purity, etc., are some of the elements that are crucial in figuring out the level of toxicity, as shown in Figure 12.
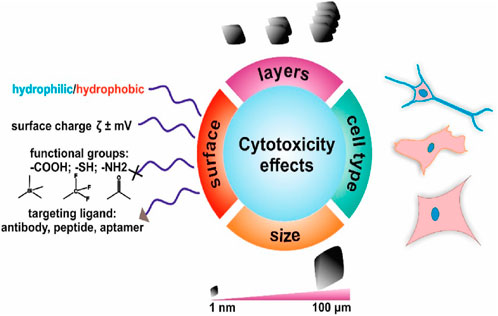
FIGURE 12. Numerous factors affecting the cytotoxicity of graphene-based materials (Tadyszak et al., 2018).
However, Deng et al. looked at characterization elements, including the hazardous impact, destiny, and exposure factor of GO, in the atmosphere to examine the life cycle influence of GO-BNs (Deng et al., 2017). Moreover, the layered architecture of GBNs is controlled by the surface chemistry, which also makes it simpler to comprehend the active surface area and flexural rigidity while carrying out any biofunctionalization feature. The lateral dimension, which is useful for biological aspects including cell uptake, renal clearance, and transit across the blood-brain barrier, can be utilized to ascertain the dimension of the substance. Regarding the use of NMs based on graphene, purity is another issue. The residual and unreacted compounds that are produced throughout the synthesis process must be kept under observation and eliminated. To report the toxicity features of GBNs for biological investigations, numerous characterizations must be carried out. Researchers have suggested using GBNs for a broad array of biomedical purposes by assessing their toxicity and safety in many investigations.
7 Conclusion and outlook
The understanding of utilizing graphene-based nanomaterials in various applications, particularly for addressing environmental challenges, has significantly progressed in recent years. The special characteristics of graphene have created new opportunities for augmenting GBN functioning in a broad array of fields, such as wastewater treatment. However, the advancement made possible by the use of graphene was only marginally better than that made possible by other carbon-based NMs or even by more conventional carbonaceous materials, such as activated carbon. In this review, we examined a few current progressions in the fabrication and use of graphene and GBNs in the elimination of contaminants from water. The extraordinary characteristics of GBNs, such as their large SA, several unsaturated π-bonds, mechanical characteristics, and adsorption capacities, have also been elucidated, with a special emphasis on those that favor sensor platforms and environmental applications of this material, such as water remediation. Nevertheless, the utilization of GBNs as adsorbents in the environment should not be limited to water treatment and should to be expanded to include air and soil filtration. Without causing additional deterioration, the high AC and physisorption can be used to remove and separate contaminants from the soil, air, and water. Traditional contaminants including dyes, insecticides, and organic solvents, have been the subject of the majority of investigations. Therefore, research on new contaminants such as oil, grease, antibiotics, phenolics, oxygen-demanding wastes, and derivatives of octanoic acid will be needed in the future.
Despite the numerous indications that demonstrate the value of GBNs as adsorbents, there is no recognition of their extensive use in environmental cleaning. This is because toxicity, which includes both short- and long-term exposure to individuals and their surroundings, is a problem that is almost completely disregarded. As a result, concerns about the impacts on public health and the environment have been raised in the scientific community. As the waste from GBN manufacture is released rapidly into the biological environment, it was expected and later observed that the marketplace for GBN merchandise would reach millions of dollars in coming years. It is essential to have a thorough understanding of the interaction between GBNs and the biological system as well as the possible toxicity of GBNs to the natural environment to fully exploit their application advantages in biomedicine and minimize their influence on the environment. It is obvious that the chemical manufacture of GBNs and their sensor applications is far from mature, and none of them have yet touched the industrial scale, given the quick display of more intriguing features of these materials. Overall, the discussion makes clear that the progress accomplished thus far is impressive. A cost-effective and practical methodology for fabricating high-quality graphene on a wide gage that is also ecologically benign is still needed. In addition, even though numerous studies have demonstrated that the adsorbents of GBNs can be reprocessed, these studies are yet rare, and future studies must focus on more creative approaches to facilitate the parting and renaissance of GBN’s adsorbents.
Furthermore, research on GBNs is still in its early stages and needs additional inputs, but thus far, they seem to be excellent prospects for water treatment applications. The use of GBNs as adsorbents will be revolutionized by more advancements in several fields. Furthermore, with advancements in nanomaterial fabrication, additional graphene-based materials should be produced in future research. Understanding of the many characteristics and phenomena linked to GBNs, especially as adsorbents for water treatment, can be aided by this review, which can also assist researchers in realizing the full potential of GBNs.
Author contributions
HA: Conceptualization, Writing–original draft. IL: Formal Analysis, Writing–review and editing. AlK: Investigation, Writing–original draft. AsK: Supervision, Validation, Writing–review and editing.
Funding
The author(s) declare that no financial support was received for the research, authorship, and/or publication of this article.
Conflict of interest
The authors declare that the research was conducted in the absence of any commercial or financial relationships that could be construed as a potential conflict of interest.
Publisher’s note
All claims expressed in this article are solely those of the authors and do not necessarily represent those of their affiliated organizations, or those of the publisher, the editors and the reviewers. Any product that may be evaluated in this article, or claim that may be made by its manufacturer, is not guaranteed or endorsed by the publisher.
Abbreviations
2D, two dimensional; CBMs, carbon-based materials; CNTs, carbon nanotubes; CVD, chemical vapor deposition; DSSCs, dye-sensitized solar cells; ECL, electrogenerated chemiluminescence; FLG, few-layer graphene; GBNs, graphene-based nanomaterials; GNRs, graphene nanoribbons; GO, graphene oxide; GPs, graphene platelets; GQD, graphene quantum dots; HC, hydrocarbon; LI-rGO, laser-induced reduced graphene oxide; PC, partition coefficient; PZC, point of zero charge; rGO, reduced graphene oxide; SA, surface area; SAED, selected area electron diffraction; SDS, sodium dodecyl sulphate; TEM, transmission electron microscopy; TPrA, tri-N-propylamine; VOCs, volatile organic compounds; WPE, wall plug efficiency.
References
Abadee, Z. G. N., Hekmati, M., and Ganji, M. D. (2019). Removing phenol contaminants from wastewater using graphene nanobuds: dft and reactive Md simulation investigations. J. Mol. Liq. 286, 110872. doi:10.1016/j.molliq.2019.04.149
Adel, M., Ahmed, M. A., Elabiad, M. A., and Mohamed, A. A. (2022). Removal of heavy metals and dyes from wastewater using graphene oxide-based nanomaterials: a critical review. Environ. Nanotechnol. Monit. Manag. 18, 100719. doi:10.1016/j.enmm.2022.100719
Ahmaruzzaman, M. (2011). Industrial wastes as low-cost potential adsorbents for the treatment of wastewater laden with heavy metals. Adv. Colloid Interface Sci. 166, 36–59. doi:10.1016/j.cis.2011.04.005
Ahmed, F., and Rodrigues, D. F. (2013). Investigation of acute effects of graphene oxide on wastewater microbial community: a case study. J. Hazard. Mater. 256, 33–39. doi:10.1016/j.jhazmat.2013.03.064
Al Faruque, M. A., Syduzzaman, M., Sarkar, J., Bilisik, K., and Naebe, M. (2021). A review on the production methods and applications of graphene-based materials. Nanomaterials 11, 2414. doi:10.3390/nano11092414
Alicanoglu, P., and Sponza, D. T. (2017). Removal of ciprofloxacin antibiotic with nano graphene oxide magnetite composite: comparison of adsorption and photooxidation processes. Desalination Water Treat. 63, 293–307. doi:10.5004/dwt.2017.20176
Almeida-Naranjo, C. E., Guerrero, V. H., and Villamar-Ayala, C. A. (2023). Emerging contaminants and their removal from aqueous media using conventional/non-conventional adsorbents: a glance at the relationship between materials, processes, and technologies. Water 15, 1626. doi:10.3390/w15081626
Amin, M. T., Alazba, A. A., and Manzoor, U. (2014). A review of removal of pollutants from water/wastewater using different types of nanomaterials. Adv. Mater. Sci. And Eng. 2014, 1–24. doi:10.1155/2014/825910
Anirudhan, T., and Deepa, J. (2017). Nano-zinc oxide incorporated graphene oxide/nanocellulose composite for the adsorption and photo catalytic degradation of ciprofloxacin hydrochloride from aqueous solutions. J. Colloid And Interface Sci. 490, 343–356. doi:10.1016/j.jcis.2016.11.042
Anirudhan, T., Deepa, J., and Nair, A. S. (2017). Fabrication of chemically modified graphene oxide/nano hydroxyapatite composite for adsorption and subsequent photocatalytic degradation of aureomycine hydrochloride. J. Industrial Eng. Chem. 47, 415–430. doi:10.1016/j.jiec.2016.12.014
Arshad, F., Selvaraj, M., Zain, J., Banat, F., and Haija, M. A. (2019). Polyethylenimine modified graphene oxide hydrogel composite as an efficient adsorbent for heavy metal ions. Sep. Purif. Technol. 209, 870–880. doi:10.1016/j.seppur.2018.06.035
Ashbolt, N. J. (2004). Microbial contamination of drinking water and disease outcomes in developing regions. Toxicology 198, 229–238. doi:10.1016/j.tox.2004.01.030
Assad, H., Fatma, I., Kumar, A., Kaya, S., Vo, D.-V. N., Al-Gheethi, A., et al. (2022a). An overview of mxene-based nanomaterials and their potential applications towards hazardous pollutant adsorption. Chemosphere 298, 134221. doi:10.1016/j.chemosphere.2022.134221
Assad, H., Kaya, S., Kumar, P. S., Vo, D.-V. N., Sharma, A., and Kumar, A. (2022b). Insights into the role of nanotechnology on the performance of biofuel cells and the production of viable biofuels: a review. Fuel 323, 124277. doi:10.1016/j.fuel.2022.124277
Assad, H., and Kumar, A. (2021). Understanding functional group effect on corrosion inhibition efficiency of selected organic compounds. J. Mol. Liq. 344, 117755. doi:10.1016/j.molliq.2021.117755
Assad, H., and Kumar, A. (2023). “Carbon nanotubes (Swcnts/Mwcnts) and functionalized carbon nanotubes in corrosion prevention,” in Corrosion prevention nanoscience: nanoengineering materials and technologies (Berlin, Boston: Walter de Gruyter), 85–98.
Assad, H., Kumar, S., Saha, S. K., Kang, N., Dahiya, H., Thakur, A., et al. (2023a). A research combined experimental and computational approaches of succinylsulfathiazole hydrate as potent corrosion inhibitor for mild steel in acidic medium. J. Mol. Liq. 388, 122739. doi:10.1016/j.molliq.2023.122739
Assad, H., Kumar, S., Saha, S. K., Kang, N., Fatma, I., Dahiya, H., et al. (2023b). Evaluating the adsorption and corrosion inhibition capabilities of pyridinium-P-toluene sulphonate on ms in 1m hcl medium: an experimental and theoretical study. Inorg. Chem. Commun. 153, 110817. doi:10.1016/j.inoche.2023.110817
Assad, H., Lone, I. A., Sihmar, A., Kumar, A., and Kumar, A. (2023c). “An overview of contemporary developments and the application of graphene-based materials in anticorrosive coatings,” in Environmental science and pollution research (Berlin, Germany: Springer), 1–20. doi:10.1007/s11356-023-30658-7
Assad, H., Saha, S. K., Kang, N., Kumar, S., Sharma, P. K., Dahiya, H., et al. (2024). Electrochemical and computational insights into the utilization of 2, 2-dithio bisbenzothiazole as A sustainable corrosion inhibitor for mild steel in low ph medium. Environ. Res. 242, 117640. doi:10.1016/j.envres.2023.117640
Bai, L., Li, Z., Zhang, Y., Wang, T., Lu, R., Zhou, W., et al. (2015). Synthesis of water-dispersible graphene-modified magnetic polypyrrole nanocomposite and its ability to efficiently adsorb methylene blue from aqueous solution. Chem. Eng. J. 279, 757–766. doi:10.1016/j.cej.2015.05.068
Balandin, A. A., Ghosh, S., Bao, W., Calizo, I., Teweldebrhan, D., Miao, F., et al. (2008). Superior thermal conductivity of single-layer graphene. Nano Lett. 8, 902–907. doi:10.1021/nl0731872
Borrás, A., Henriques, B., Gonçalves, G., Fraile, J., Pereira, E., López-Periago, A. M., et al. (2022). Graphene oxide/polyethylenimine aerogels for the removal of Hg (ii) from water. Gels 8, 452. doi:10.3390/gels8070452
Boruah, P. K., Sharma, B., Hussain, N., and Das, M. R. (2017). Magnetically recoverable Fe3o4/graphene nanocomposite towards efficient removal of triazine pesticides from aqueous solution: investigation of the adsorption phenomenon and specific ion effect. Chemosphere 168, 1058–1067. doi:10.1016/j.chemosphere.2016.10.103
Cao, M., Wang, P., Ao, Y., Wang, C., Hou, J., and Qian, J. (2016). Visible light activated photocatalytic degradation of tetracycline by A magnetically separable composite photocatalyst: graphene oxide/magnetite/cerium-doped titania. J. Colloid Interface Sci. 467, 129–139. doi:10.1016/j.jcis.2016.01.005
Celik, S. (2020). “The effects of climate change on human behaviors,” in Environment, climate, plant and vegetation growth (Chennai: Cambridge University Press), 577–589.
Chang, H., and Wu, H. (2013). Graphene-based nanomaterials: synthesis, properties, and optical and optoelectronic applications. Adv. Funct. Mater. 23, 1984–1997. doi:10.1002/adfm.201202460
Chen, F., Yang, Q., Li, X., Zeng, G., Wang, D., Niu, C., et al. (2017). Hierarchical assembly of graphene-bridged Ag3po4/Ag/Bivo4 (040) Z-scheme photocatalyst: an efficient, sustainable and heterogeneous catalyst with enhanced visible-light photoactivity towards tetracycline degradation under visible light irradiation. Appl. Catal. B Environ. 200, 330–342. doi:10.1016/j.apcatb.2016.07.021
Chen, H., Gao, B., and Li, H. (2015). Removal of sulfamethoxazole and ciprofloxacin from aqueous solutions by graphene oxide. J. Of Hazard. Mater. 282, 201–207. doi:10.1016/j.jhazmat.2014.03.063
Chen, W., Liu, P., Min, L., Zhou, Y., Liu, Y., Wang, Q., et al. (2018). Non-covalently functionalized graphene oxide-based coating to enhance thermal stability and flame retardancy of pva film. Nano-Micro Lett. 10, 39–13. doi:10.1007/s40820-018-0190-8
Cheng, H., Lin, J., Su, Y., Chen, D., Zheng, X., and Zhu, H. (2020). Green synthesis of soluble graphene in organic solvent via simultaneous functionalization and reduction of graphene oxide with urushiol. Mater. Today Commun. 23, 100938. doi:10.1016/j.mtcomm.2020.100938
Cho, S., Kikuchi, K., and Kawasaki, A. (2011). Radial followed by longitudinal unzipping of multiwalled carbon nanotubes. Carbon 49, 3865–3872. doi:10.1016/j.carbon.2011.05.023
Chowdhury, I., Hou, W.-C., Goodwin, D., Henderson, M., Zepp, R. G., and Bouchard, D. (2015). Sunlight affects aggregation and deposition of graphene oxide in the aquatic environment. Water Res. 78, 37–46. doi:10.1016/j.watres.2015.04.001
Chung, S., Revia, R. A., and Zhang, M. (2021). Graphene quantum dots and their applications in bioimaging, biosensing, and therapy. Adv. Mater. 33, 1904362. doi:10.1002/adma.201904362
Cortés Arriagada, D., Sanhueza, L., and Wrighton, K. (2013). Removal of 4-chlorophenol using graphene, graphene oxide, and A-doped graphene (A= N, B): a computational study. Int. J. Quantum Chem. 113, 1931–1939. doi:10.1002/qua.24416
Dasari Shareena, T. P., Mcshan, D., Dasmahapatra, A. K., and Tchounwou, P. B. (2018). A review on graphene-based nanomaterials in biomedical applications and risks in environment and health. Nano-Micro Lett. 10, 53–34. doi:10.1007/s40820-018-0206-4
Deng, Y., Li, J., Qiu, M., Yang, F., Zhang, J., and Yuan, C. (2017). Deriving characterization factors on freshwater ecotoxicity of graphene oxide nanomaterial for life cycle impact assessment. Int. J. Life Cycle Assess. 22, 222–236. doi:10.1007/s11367-016-1151-4
De Silva, K., Huang, H.-H., Joshi, R., and Yoshimura, M. (2017). Chemical reduction of graphene oxide using green reductants. Carbon 119, 190–199. doi:10.1016/j.carbon.2017.04.025
Dong, S., Pi, Y., Li, Q., Hu, L., Li, Y., Han, X., et al. (2016). Solar photocatalytic degradation of sulfanilamide by biocl/reduced graphene oxide nanocomposites: mechanism and degradation pathways. J. Alloys Compd. 663, 1–9. doi:10.1016/j.jallcom.2015.12.027
Duinslaeger, N., and Radjenovic, J. (2022). Electrochemical degradation of per-and polyfluoroalkyl substances (pfas) using low-cost graphene sponge electrodes. Water Res. 213, 118148. doi:10.1016/j.watres.2022.118148
Elessawy, N. A., Gouda, M. H., Ali, S. M., Salerno, M., and Eldin, M. S. M. (2020). Effective elimination of contaminant antibiotics using high-surface-area magnetic-functionalized graphene nanocomposites developed from plastic waste. Materials 13, 1517. doi:10.3390/ma13071517
Elsagh, A., Moradi, O., Fakhri, A., Najafi, F., Alizadeh, R., and Haddadi, V. (2017). Evaluation of the potential cationic dye removal using adsorption by graphene and carbon nanotubes as adsorbents surfaces. Arabian J. Chem. 10, S2862–S2869. doi:10.1016/j.arabjc.2013.11.013
Fadeel, B., Bussy, C., Merino, S., Vázquez, E., Flahaut, E., Mouchet, F., et al. (2018). Safety assessment of graphene-based materials: focus on human health and the environment. Acs Nano 12, 10582–10620. doi:10.1021/acsnano.8b04758
Fan, J., Hui, S., Bailey, T. P., Page, A., Uher, C., and Yuan, F. (2019). Ultralow thermal conductivity in graphene–silica porous ceramics with A special saucer structure of graphene aerogels. J. Mater. Chem. A 7, 1574–1584. doi:10.1039/c8ta10426c
Fathizadeh, M., Xu, W. L., Zhou, F., Yoon, Y., and Yu, M. (2017). Graphene oxide: a novel 2-dimensional material in membrane separation for water purification. Adv. Mater. Interfaces 4, 1600918. doi:10.1002/admi.201600918
Fraga, T. J., Carvalho, M. N., Ghislandi, M. G., and Motta, M. A. D. (2019). Functionalized graphene-based materials as innovative adsorbents of organic pollutants: a concise overview. Braz. J. Chem. Eng. 36, 1–31. doi:10.1590/0104-6632.20190361s20180283
Gagliano, E., Sgroi, M., Falciglia, P. P., Vagliasindi, F. G., and Roccaro, P. (2020). Removal of poly-and perfluoroalkyl substances (pfas) from water by adsorption: role of pfas chain length, effect of organic matter and challenges in adsorbent regeneration. Water Res. 171, 115381. doi:10.1016/j.watres.2019.115381
Ganesan, V., Louis, C., and Damodaran, S. P. (2018). Graphene oxide-wrapped magnetite nanoclusters: a recyclable functional hybrid for fast and highly efficient removal of organic dyes from wastewater. J. Environ. Chem. Eng. 6, 2176–2190. doi:10.1016/j.jece.2018.03.026
Ganjoo, R., Sharma, S., Assad, H., and Kumar, A. (2023). “Applications of magnetic surfactants in water treatment,” in Magnetic surfactants: design, chemistry and utilization (Washington, DC: ACS Publications).
Gao, L., Wang, Y., Yan, T., Cui, L., Hu, L., Yan, L., et al. (2015). A novel magnetic polysaccharide–graphene oxide composite for removal of cationic dyes from aqueous solution. New J. Chem. 39, 2908–2916. doi:10.1039/c4nj01792g
Geim, A. K. (2009). Graphene: status and prospects. Science 324, 1530–1534. doi:10.1126/science.1158877
Ghany, N. A. A., Elsherif, S. A., and Handal, H. T. (2017). Revolution of graphene for different applications: state-of-the-art. Surfaces Interfaces 9, 93–106. doi:10.1016/j.surfin.2017.08.004
Goenka, S., Sant, V., and Sant, S. (2014). Graphene-based nanomaterials for drug delivery and tissue engineering. J. Control. Release 173, 75–88. doi:10.1016/j.jconrel.2013.10.017
Gollavelli, G., Chang, C.-C., and Ling, Y.-C. (2013). Facile synthesis of smart magnetic graphene for safe drinking water: heavy metal removal and disinfection control. Acs Sustain. Chem. Eng. 1, 462–472. doi:10.1021/sc300112z
Guo, Z., Huang, J., Xue, Z., and Wang, X. (2016). Electrospun graphene oxide/carbon composite nanofibers with well-developed mesoporous structure and their adsorption performance for benzene and butanone. Chem. Eng. J. 306, 99–106. doi:10.1016/j.cej.2016.07.048
Gupta, N. K., Joshi, P., Srivastava, V., and Quraishi, M. (2018). Chitosan: a macromolecule as green corrosion inhibitor for mild steel in sulfamic acid useful for sugar industry. Int. J. Biol. Macromol. 106, 704–711. doi:10.1016/j.ijbiomac.2017.08.064
Gusain, R., Kumar, N., and Ray, S. S. (2020). Recent advances in carbon nanomaterial-based adsorbents for water purification. Coord. Chem. Rev. 405, 213111. doi:10.1016/j.ccr.2019.213111
Homaeigohar, S., and Elbahri, M. (2017). Graphene membranes for water desalination. NPG Asia Mater. 9, E427. doi:10.1038/am.2017.135
Hou, P., Xing, G., Tian, L., Zhang, G., Wang, H., Yu, C., et al. (2019). Hollow carbon spheres/graphene hybrid aerogels as high-performance adsorbents for organic pollution. Sep. Purif. Technol. 213, 524–532. doi:10.1016/j.seppur.2018.12.032
Huang, D., Li, B., Wu, M., Kuga, S., and Huang, Y. (2018). Graphene oxide-based Fe–Mg (hydr) oxide nanocomposite as heavy metals adsorbent. J. Chem. Eng. Data 63, 2097–2105. doi:10.1021/acs.jced.8b00100
Huang, Y., Gong, Y., Tang, J., and Xia, S. (2019). Effective removal of inorganic mercury and methylmercury from aqueous solution using novel thiol-functionalized graphene oxide/Fe-Mn composite. J. Hazard. Mater. 366, 130–139. doi:10.1016/j.jhazmat.2018.11.074
Huang, Z.-H., Zheng, X., Lv, W., Wang, M., Yang, Q.-H., and Kang, F. (2011). Adsorption of lead (ii) ions from aqueous solution on low-temperature exfoliated graphene nanosheets. Langmuir 27, 7558–7562. doi:10.1021/la200606r
Huo, P., Zhou, M., Tang, Y., Liu, X., Ma, C., Yu, L., et al. (2016). Incorporation of N–Zno/Cds/Graphene oxide composite photocatalyst for enhanced photocatalytic activity under visible light. J. Alloys Compd. 670, 198–209. doi:10.1016/j.jallcom.2016.01.247
Huong, P. T. L., Tu, N., Lan, H., Van Quy, N., Tuan, P. A., Dinh, N. X., et al. (2018). Functional manganese ferrite/graphene oxide nanocomposites: effects of graphene oxide on the adsorption mechanisms of organic mb dye and inorganic as (V) ions from aqueous solution. Rsc Adv. 8, 12376–12389. doi:10.1039/c8ra00270c
Jaiswal, K. K., Sudhakar, S., and Ramaswamy, A. P. (2018). ‘Graphene’–World’s thinnest material for revolutionizing applications. Everyman’s Sci. 53, 219–223.
Jana, A., Scheer, E., and Polarz, S. (2017). Synthesis of graphene–transition metal oxide hybrid nanoparticles and their application in various fields. Beilstein J. Nanotechnol. 8, 688–714. doi:10.3762/bjnano.8.74
Jiang, G., Tian, H., Wang, X.-F., Hirtz, T., Wu, F., Qiao, Y.-C., et al. (2019). An efficient flexible graphene-based light-emitting device. Nanoscale Adv. 1, 4745–4754. doi:10.1039/c9na00550a
Jiang, X., Ruan, G., Huang, Y., Chen, Z., Yuan, H., and Du, F. (2020). Assembly and application advancement of organic-functionalized graphene-based materials: a review. J. Sep. Sci. 43, 1544–1557. doi:10.1002/jssc.201900694
Joel, E. F., and Lujanienė, G. (2022). Progress in graphene oxide hybrids for environmental applications. Environments 9, 153. doi:10.3390/environments9120153
Julkapli, N. M., and Bagheri, S. (2015). Graphene supported heterogeneous catalysts: an overview. Int. J. Hydrogen Energy 40, 948–979. doi:10.1016/j.ijhydene.2014.10.129
Kamangerpour, A., Ashraf-Khorassani, M., Taylor, L., Mcnair, H., and Chorida, L. (2002). Supercritical fluid chromatography of polyphenolic compounds in grape seed extract. Chromatographia 55, 417–421. doi:10.1007/bf02492270
Kerkez-Kuyumcu, Ö., Bayazit, Ş. S., and Salam, M. A. (2016). Antibiotic amoxicillin removal from aqueous solution using magnetically modified graphene nanoplatelets. J. Industrial Eng. Chem. 36, 198–205. doi:10.1016/j.jiec.2016.01.040
Khalid, Z. B., Nasrullah, M., Nayeem, A., Wahid, Z. A., Singh, L., and Krishnan, S. (2020). “Application of 2d graphene-based nanomaterials for pollutant removal from advanced water and wastewater treatment processes,” in Adapting 2d nanomaterials for advanced applications (Washington, DC: ACS Publications).
Khaliha, S., Marforio, T. D., Kovtun, A., Mantovani, S., Bianchi, A., Navacchia, M. L., et al. (2021). Defective graphene nanosheets for drinking water purification: adsorption mechanism, performance, and recovery. Flatchem 29, 100283. doi:10.1016/j.flatc.2021.100283
Khan, A. M., Manea, Y. K., and Nabi, S. A. (2019). Synthesis and characterization of nanocomposite acrylamide tin (iv) silicomolybdate: photocatalytic activity and chromatographic column separations. J. Anal. Chem. 74, 330–338. doi:10.1134/s1061934819040026
Kim, J. M., Kim, J. H., Lee, C. Y., Jerng, D. W., and Ahn, H. S. (2018a). Toluene and acetaldehyde removal from air on to graphene-based adsorbents with microsized pores. J. Hazard. Mater. 344, 458–465. doi:10.1016/j.jhazmat.2017.10.038
Kim, S., Park, C. M., Jang, M., Son, A., Her, N., Yu, M., et al. (2018b). Aqueous removal of inorganic and organic contaminants by graphene-based nanoadsorbents: a review. Chemosphere 212, 1104–1124. doi:10.1016/j.chemosphere.2018.09.033
Kim, S., Song, Y., Wright, J., and Heller, M. J. (2016). Graphene Bi-and trilayers produced by A novel aqueous arc discharge process. Carbon 102, 339–345. doi:10.1016/j.carbon.2016.02.049
Konicki, W., Aleksandrzak, M., Moszyński, D., and Mijowska, E. (2017). Adsorption of anionic azo-dyes from aqueous solutions onto graphene oxide: equilibrium, kinetic and thermodynamic studies. J. Colloid Interface Sci. 496, 188–200. doi:10.1016/j.jcis.2017.02.031
Kosynkin, D. V., Higginbotham, A. L., Sinitskii, A., Lomeda, J. R., Dimiev, A., Price, B. K., et al. (2009). Longitudinal unzipping of carbon nanotubes to form graphene nanoribbons. Nature 458, 872–876. doi:10.1038/nature07872
Krishna, R. H., Chandraprabha, M., Samrat, K., Murthy, T. K., Manjunatha, C., and Kumar, S. G. (2023). Carbon nanotubes and graphene-based materials for adsorptive removal of metal ions–A review on surface functionalization and related adsorption mechanism. Appl. Surf. Sci. Adv. 16, 100431. doi:10.1016/j.apsadv.2023.100431
Kuchi, R., Nguyen, H. M., Dongquoc, V., Van, P. C., Ahn, H., Viet, D. D., et al. (2021). Optimization of feni/swcnt composites by A simple Co-arc discharge process to improve microwave absorption performance. J. Alloys Compd. 852, 156712. doi:10.1016/j.jallcom.2020.156712
Kumar, V., Kim, K.-H., Park, J.-W., Hong, J., and Kumar, S. (2017). Graphene and its nanocomposites as A platform for environmental applications. Chem. Eng. J. 315, 210–232. doi:10.1016/j.cej.2017.01.008
Kumari, S., Sharma, P., Ghosh, D., Shandilya, M., Rawat, P., Hassan, M. I., et al. (2020). Time-dependent study of graphene oxide-trypsin adsorption interface and visualization of nano-protein corona. Int. J. Biol. Macromol. 163, 2259–2269. doi:10.1016/j.ijbiomac.2020.09.099
Labiadh, L., and Kamali, A. R. (2019). 3d graphene nanoedges as efficient dye adsorbents with ultra-high thermal regeneration performance. Appl. Surf. Sci. 490, 383–394. doi:10.1016/j.apsusc.2019.06.081
Lee, G., Chen, C., Yang, S.-T., and Ahn, W.-S. (2010). Enhanced adsorptive removal of fluoride using mesoporous alumina. Microporous Mesoporous Mater. 127, 152–156. doi:10.1016/j.micromeso.2009.07.007
Leonard, P., Hearty, S., Brennan, J., Dunne, L., Quinn, J., Chakraborty, T., et al. (2003). Advances in biosensors for detection of pathogens in food and water. Enzyme Microb. Technol. 32, 3–13. doi:10.1016/s0141-0229(02)00232-6
Li, H., Hu, J., Meng, Y., Su, J., and Wang, X. (2017a). An investigation into the rapid removal of tetracycline using multilayered graphene-phase biochar derived from waste chicken feather. Sci. Total Environ. 603, 39–48. doi:10.1016/j.scitotenv.2017.06.006
Li, H., and Papadakis, R. (2020). Click chemistry enabling covalent and non-covalent modifications of graphene with (poly) saccharides. Polymers 13, 142. doi:10.3390/polym13010142
Li, J., Zeng, H., Zeng, Z., Zeng, Y., and Xie, T. (2021). Promising graphene-based nanomaterials and their biomedical applications and potential risks: a comprehensive review. Acs Biomaterials Sci. Eng. 7, 5363–5396. doi:10.1021/acsbiomaterials.1c00875
Li, K., Zhao, X., Wei, G., and Su, Z. (2018). Recent advances in the cancer bioimaging with graphene quantum dots. Curr. Med. Chem. 25, 2876–2893. doi:10.2174/0929867324666170223154145
Li, Y., Chen, L., Wang, Y., and Zhu, L. (2016a). Advanced nanostructured photocatalysts based on reduced graphene oxide-flower-like Bi2wo6 composites for an augmented simulated solar photoactivity activity. Mater. Sci. Eng. B 210, 29–36. doi:10.1016/j.mseb.2016.03.010
Li, Y., Miao, J., Sun, X., Xiao, J., Li, Y., Wang, H., et al. (2016b). Mechanochemical synthesis of Cu-btc@ go with enhanced water stability and toluene adsorption capacity. Chem. Eng. J. 298, 191–197. doi:10.1016/j.cej.2016.03.141
Li, Z., Qi, M., Tu, C., Wang, W., Chen, J., and Wang, A.-J. (2017b). Highly efficient removal of chlorotetracycline from aqueous solution using graphene oxide/tio2 composite: properties and mechanism. Appl. Surf. Sci. 425, 765–775. doi:10.1016/j.apsusc.2017.07.027
Lin, L., Wang, H., Jiang, W., Mkaouar, A. R., and Xu, P. (2017a). Comparison study on photocatalytic oxidation of pharmaceuticals by Tio2-Fe and tio2-reduced graphene oxide nanocomposites immobilized on optical fibers. J. Hazard. Mater. 333, 162–168. doi:10.1016/j.jhazmat.2017.02.044
Lin, L., Wang, H., and Xu, P. (2017b). Immobilized tio2-reduced graphene oxide nanocomposites on optical fibers as high performance photocatalysts for degradation of pharmaceuticals. Chem. Eng. J. 310, 389–398. doi:10.1016/j.cej.2016.04.024
Liu, C., Wu, T., Hsu, P.-C., Xie, J., Zhao, J., Liu, K., et al. (2019). Direct/alternating current electrochemical method for removing and recovering heavy metal from water using graphene oxide electrode. Acs Nano 13, 6431–6437. doi:10.1021/acsnano.8b09301
Liu, F.-F., Zhao, J., Wang, S., and Xing, B. (2016). Adsorption of sulfonamides on reduced graphene oxides as affected by ph and dissolved organic matter. Environ. Pollut. 210, 85–93. doi:10.1016/j.envpol.2015.11.053
Liu, G.-Q., Wan, M.-X., Huang, Z.-H., and Kang, F.-Y. (2015a). Preparation of graphene/metal-organic composites and their adsorption performance for benzene and ethanol. New Carbon Mater. 30, 566–571. doi:10.1016/s1872-5805(15)60205-0
Liu, H., and Qiu, H. (2020). Recent advances of 3d graphene-based adsorbents for sample preparation of water pollutants: a review. Chem. Eng. J. 393, 124691. doi:10.1016/j.cej.2020.124691
Liu, M., Xu, J., Cheng, B., Ho, W., and Yu, J. (2015b). Synthesis and adsorption performance of Mg (oh) 2 hexagonal nanosheet–graphene oxide composites. Appl. Surf. Sci. 332, 121–129. doi:10.1016/j.apsusc.2015.01.121
Lombardi, L., Kovtun, A., Mantovani, S., Bertuzzi, G., Favaretto, L., Bettini, C., et al. (2022). Visible-light assisted covalent surface functionalization of reduced graphene oxide nanosheets with arylazo sulfones. Chemistry–A Eur. J. 28, E202200333. doi:10.1002/chem.202200333
Lu, F., and Astruc, D. (2018). Nanomaterials for removal of toxic elements from water. Coord. Chem. Rev. 356, 147–164. doi:10.1016/j.ccr.2017.11.003
Lúcio, M., Fernandes, E., Gonçalves, H., Machado, S., Gomes, A. C., and Oliveira, M. E. C. R. (2021). “Graphene-based nanosystems: versatile nanotools for theranostics and bioremediation,” in Theranostics-an old concept in new clothing (United Kingdom: Intechopen).
Madadrang, C. J., Kim, H. Y., Gao, G., Wang, N., Zhu, J., Feng, H., et al. (2012). Adsorption behavior of edta-graphene oxide for Pb (ii) removal. Acs Appl. Mater. Interfaces 4, 1186–1193. doi:10.1021/am201645g
Madni, A., Noreen, S., Maqbool, I., Rehman, F., Batool, A., Kashif, P. M., et al. (2018). Graphene-based nanocomposites: synthesis and their theranostic applications. J. Drug Target. 26, 858–883. doi:10.1080/1061186x.2018.1437920
Mahmoud, A. E. D. (2020). Graphene-based nanomaterials for the removal of organic pollutants: insights into linear versus nonlinear mathematical models. J. Environ. Manag. 270, 110911. doi:10.1016/j.jenvman.2020.110911
Mantovani, S., Khaliha, S., Favaretto, L., Bettini, C., Bianchi, A., Kovtun, A., et al. (2021). Scalable synthesis and purification of functionalized graphene nanosheets for water remediation. Chem. Commun. 57, 3765–3768. doi:10.1039/d1cc00704a
Mantovani, S., Khaliha, S., Marforio, T. D., Kovtun, A., Favaretto, L., Tunioli, F., et al. (2022). Facile high-yield synthesis and purification of lysine-modified graphene oxide for enhanced drinking water purification. Chem. Commun. 58, 9766–9769. doi:10.1039/d2cc03256b
Mantovani, S., Marforio, T. D., Khaliha, S., Pintus, A., Kovtun, A., Tunioli, F., et al. (2023). Amino acid-driven adsorption of emerging contaminants in water by modified graphene oxide nanosheets. Environ. Sci. Water Res. Technol. 9, 1030–1040. doi:10.1039/d2ew00871h
Marcano, D. C., Kosynkin, D. V., Berlin, J. M., Sinitskii, A., Sun, Z., Slesarev, A., et al. (2010). Improved synthesis of graphene oxide. Acs Nano 4, 4806–4814. doi:10.1021/nn1006368
Masindi, V., and Muedi, K. L. (2018). “Environmental contamination by heavy metals,” in Heavy metals (United Kingdom: IntechOpen), 115–132.
Mathur, J., Goswami, P., Gupta, A., Srivastava, S., Minkina, T., Shan, S., et al. (2022). Nanomaterials for water remediation: an efficient strategy for prevention of metal (loid) hazard. Water 14, 3998. doi:10.3390/w14243998
Mercante, L. A., Facure, M. H., Locilento, D. A., Sanfelice, R. C., Migliorini, F. L., Mattoso, L. H., et al. (2017). Solution blow spun pmma nanofibers wrapped with reduced graphene oxide as an efficient dye adsorbent. New J. Chem. 41, 9087–9094. doi:10.1039/c7nj01703k
Mohammadnia, E., Hadavifar, M., and Veisi, H. (2019). Kinetics and thermodynamics of mercury adsorption onto thiolated graphene oxide nanoparticles. Polyhedron 173, 114139. doi:10.1016/j.poly.2019.114139
Mohd, S., Wani, A. A., and Khan, A. M. (2022). Zno/poa functionalized metal-organic framework zif-8 nanomaterial for dye removal. Clean. Chem. Eng. 3, 100047. doi:10.1016/j.clce.2022.100047
Molina, J., Cases, F., and Moretto, L. M. (2016). Graphene-based materials for the electrochemical determination of hazardous ions. Anal. Chim. Acta 946, 9–39. doi:10.1016/j.aca.2016.10.019
Nguyen, C. H., and Juang, R.-S. (2019). Efficient removal of methylene blue dye by A hybrid adsorption–photocatalysis process using reduced graphene oxide/titanate nanotube composites for water reuse. J. Industrial Eng. Chem. 76, 296–309. doi:10.1016/j.jiec.2019.03.054
Nikam, P. B., Salunkhe, J. D., Minkina, T., Rajput, V. D., Kim, B. S., and Patil, S. V. (2022). A review on green synthesis and recent applications of red nano selenium. Results Chem. 4, 100581. doi:10.1016/j.rechem.2022.100581
O’hare, A., Kusmartsev, F., and Kugel, K. (2012). A stable “flat” form of two-dimensional crystals: could graphene, silicene, germanene Be minigap semiconductors? Nano Lett. 12, 1045–1052. doi:10.1021/nl204283q
Ou, L., Song, B., Liang, H., Liu, J., Feng, X., Deng, B., et al. (2016). Toxicity of graphene-family nanoparticles: a general review of the origins and mechanisms. Part. Fibre Toxicol. 13, 57–24. doi:10.1186/s12989-016-0168-y
Paramasivan, T., Sivarajasekar, N., Muthusaravanan, S., Subashini, R., Prakashmaran, J., Sivamani, S., et al. (2019). “Graphene family materials for the removal of pesticides from water,” in A new generation material graphene: applications in water technology (Berlin, Germany: Springer), 309–327.
Pedrosa, M., Da Silva, E. S., Pastrana-Martínez, L. M., Drazic, G., Falaras, P., Faria, J. L., et al. (2020). Hummers’ and brodie’s graphene oxides as photocatalysts for phenol degradation. J. Colloid Interface Sci. 567, 243–255. doi:10.1016/j.jcis.2020.01.093
Pei, S., and Cheng, H.-M. (2012). The reduction of graphene oxide. Carbon 50, 3210–3228. doi:10.1016/j.carbon.2011.11.010
Peng, S., Wu, D., Ge, Z., Tong, M., and Kim, H. (2017). Influence of graphene oxide on the transport and deposition behaviors of colloids in saturated porous media. Environ. Pollut. 225, 141–149. doi:10.1016/j.envpol.2017.03.064
Perreault, F., De Faria, A. F., and Elimelech, M. (2015). Environmental applications of graphene-based nanomaterials. Chem. Soc. Rev. 44, 5861–5896. doi:10.1039/c5cs00021a
Podder, A., Sadmani, A. A., Reinhart, D., Chang, N.-B., and Goel, R. (2021). Per and poly-fluoroalkyl substances (pfas) as A contaminant of emerging concern in surface water: a transboundary review of their occurrences and toxicity effects. J. Hazard. Mater. 419, 126361. doi:10.1016/j.jhazmat.2021.126361
Potts, J. R., Dreyer, D. R., Bielawski, C. W., and Ruoff, R. S. (2011). Graphene-based polymer nanocomposites. Polymer 52, 5–25. doi:10.1016/j.polymer.2010.11.042
Pourjavadi, A., Nazari, M., Kabiri, B., Hosseini, S. H., and Bennett, C. (2016). Preparation of porous graphene oxide/hydrogel nanocomposites and their ability for efficient adsorption of methylene blue. Rsc Adv. 6, 10430–10437. doi:10.1039/c5ra21629j
Priyadharshini, S. D., Manikandan, S., Kiruthiga, R., Rednam, U., Babu, P. S., Subbaiya, R., et al. (2022). Graphene oxide-based nanomaterials for the treatment of pollutants in the aquatic environment: recent trends and perspectives–A review. Environ. Pollut. 306, 119377. doi:10.1016/j.envpol.2022.119377
Radjenovic, J., and Sedlak, D. L. (2015). Challenges and opportunities for electrochemical processes as next-generation technologies for the treatment of contaminated water. Environ. Sci. Technol. 49, 11292–11302. doi:10.1021/acs.est.5b02414
Reina, G., González-Domínguez, J. M., Criado, A., Vázquez, E., Bianco, A., and Prato, M. (2017). Promises, facts and challenges for graphene in biomedical applications. Chem. Soc. Rev. 46, 4400–4416. doi:10.1039/c7cs00363c
Ren, W., Chang, H., Mao, T., and Teng, Y. (2019). Planarity effect of polychlorinated biphenyls adsorption by graphene nanomaterials: the influence of graphene characteristics, solution ph and temperature. Chem. Eng. J. 362, 160–168. doi:10.1016/j.cej.2019.01.027
Rostamian, R., and Behnejad, H. (2016). A comparative adsorption study of sulfamethoxazole onto graphene and graphene oxide nanosheets through equilibrium, kinetic and thermodynamic modeling. Process Saf. Environ. Prot. 102, 20–29. doi:10.1016/j.psep.2015.12.011
Saeed, M., Alshammari, Y., Majeed, S. A., and Al-Nasrallah, E. (2020). Chemical vapour deposition of graphene—synthesis, characterisation, and applications: a review. Molecules 25, 3856. doi:10.3390/molecules25173856
Sahu, D., Sutar, H., Senapati, P., Murmu, R., and Roy, D. (2021). Graphene, graphene-derivatives and composites: fundamentals, synthesis approaches to applications. J. Compos. Sci. 5, 181. doi:10.3390/jcs5070181
Schulze, S., Zahn, D., Montes, R., Rodil, R., Quintana, J. B., Knepper, T. P., et al. (2019). Occurrence of emerging persistent and mobile organic contaminants in European water samples. Water Res. 153, 80–90. doi:10.1016/j.watres.2019.01.008
Sengupta, J., and Hussain, C. M. (2019). Graphene and its derivatives for analytical lab on chip platforms. Trac Trends Chem. 114, 326–337. doi:10.1016/j.trac.2019.03.015
Shahrin, S., Lau, W.-J., Goh, P.-S., Jaafar, J., and Ismail, A. F. (2018). Adsorptive removal of as (V) ions from water using graphene oxide-manganese ferrite and titania nanotube-manganese ferrite hybrid nanomaterials. Chem. Eng. Technol. 41, 2250–2258. doi:10.1002/ceat.201800322
Shen, B., Ding, J., Yan, X., Feng, W., Li, J., and Xue, Q. (2012). Influence of different buffer gases on synthesis of few-layered graphene by arc discharge method. Appl. Surf. Sci. 258, 4523–4531. doi:10.1016/j.apsusc.2012.01.019
Shi, G., Ding, Y., and Fang, H. (2012). Unexpectedly strong anion–Π interactions on the graphene flakes. J. Comput. Chem. 33, 1328–1337. doi:10.1002/jcc.22964
Siddiqui, S. I., and Chaudhry, S. A. (2018). A review on graphene oxide and its composites preparation and their use for the removal of As3+ and As5+ from water under the effect of various parameters: application of isotherm, kinetic and thermodynamics. Process Saf. Environ. Prot. 119, 138–163. doi:10.1016/j.psep.2018.07.020
Sieradzka, M., Ślusarczyk, C., Biniaś, W., and Fryczkowski, R. (2021). The role of the oxidation and reduction parameters on the properties of the reduced graphene oxide. Coatings 11, 166. doi:10.3390/coatings11020166
Sitko, R., Turek, E., Zawisza, B., Malicka, E., Talik, E., Heimann, J., et al. (2013). Adsorption of divalent metal ions from aqueous solutions using graphene oxide. Dalton Trans. 42, 5682–5689. doi:10.1039/c3dt33097d
Soldano, C., Mahmood, A., and Dujardin, E. (2010). Production, properties and potential of graphene. Carbon 48, 2127–2150. doi:10.1016/j.carbon.2010.01.058
Song, W., Yang, T., Wang, X., Sun, Y., Ai, Y., Sheng, G., et al. (2016). Experimental and theoretical evidence for competitive interactions of tetracycline and sulfamethazine with reduced graphene oxides. Environ. Sci. Nano 3, 1318–1326. doi:10.1039/c6en00306k
Stankovich, S., Dikin, D. A., Piner, R. D., Kohlhaas, K. A., Kleinhammes, A., Jia, Y., et al. (2007). Synthesis of graphene-based nanosheets via chemical reduction of exfoliated graphite oxide. Carbon 45, 1558–1565. doi:10.1016/j.carbon.2007.02.034
Sun, W., Huang, S., Zhang, S., and Luo, Q. (2019). Preparation, characterization and application of multi-mode imaging functional graphene Au-Fe3o4 magnetic nanocomposites. Materials 12, 1978. doi:10.3390/ma12121978
Sun, X., Xia, Q., Zhao, Z., Li, Y., and Li, Z. (2014). Synthesis and adsorption performance of mil-101 (Cr)/Graphite oxide composites with high capacities of N-hexane. Chem. Eng. J. 239, 226–232. doi:10.1016/j.cej.2013.11.024
Szulejko, J. E., Kim, K.-H., and Parise, J. (2019). Seeking the most powerful and practical real-world sorbents for gaseous benzene as A representative volatile organic compound based on performance metrics. Sep. Purif. Technol. 212, 980–985. doi:10.1016/j.seppur.2018.11.001
Tabrizian, P., Ma, W., Bakr, A., and Rahaman, M. S. (2019). Ph-sensitive and magnetically separable Fe/Cu bimetallic nanoparticles supported by graphene oxide (go) for high-efficiency removal of tetracyclines. J. Colloid Interface Sci. 534, 549–562. doi:10.1016/j.jcis.2018.09.034
Tadyszak, K., Wychowaniec, J. K., and Litowczenko, J. (2018). Biomedical applications of graphene-based structures. Nanomaterials 8, 944. doi:10.3390/nano8110944
Tan, P., Sun, J., Hu, Y., Fang, Z., Bi, Q., Chen, Y., et al. (2015). Adsorption of Cu2+, Cd2+ and Ni2+ from aqueous single metal solutions on graphene oxide membranes. J. Hazard. Mater. 297, 251–260. doi:10.1016/j.jhazmat.2015.04.068
Tang, S., Xia, D., Yao, Y., Chen, T., Sun, J., Yin, Y., et al. (2019). Dye adsorption by self-recoverable, adjustable amphiphilic graphene aerogel. J. Colloid Interface Sci. 554, 682–691. doi:10.1016/j.jcis.2019.07.041
Tang, Y., Liu, X., Ma, C., Zhou, M., Huo, P., Yu, L., et al. (2015). Enhanced photocatalytic degradation of tetracycline antibiotics by reduced graphene oxide–cds/zns heterostructure photocatalysts. New J. Chem. 39, 5150–5160. doi:10.1039/c5nj00681c
Tara, N., Sharma, A., Choudhry, A., Abdulla, N. K., Rathi, G., Khan, A., et al. (2021). “Graphene, graphene oxide, and reduced graphene oxide-based materials: a comparative adsorption performance,” in Contamination of water (Netherlands: Elsevier), 495–507.
Thakur, K., and Kandasubramanian, B. (2019). Graphene and graphene oxide-based composites for removal of organic pollutants: a review. J. Chem. Eng. Data 64, 833–867. doi:10.1021/acs.jced.8b01057
Tsang, C. H. A., Kwok, H., Cheng, Z., and Leung, D. (2017). The applications of graphene-based materials in pollutant control and disinfection. Prog. Solid State Chem. 45, 1–8. doi:10.1016/j.progsolidstchem.2017.02.001
Tunioli, F., Khaliha, S., Mantovani, S., Bianchi, A., Kovtun, A., Xia, Z., et al. (2023). Adsorption of emerging contaminants by graphene related materials and their alginate composite hydrogels. J. Environ. Chem. Eng. 11, 109566. doi:10.1016/j.jece.2023.109566
Uddin, M. T., Islam, M. A., Mahmud, S., and Rukanuzzaman, M. (2009). Adsorptive removal of methylene blue by tea waste. J. Hazard. Mater. 164, 53–60. doi:10.1016/j.jhazmat.2008.07.131
Verstraeten, I. M., Heberer, T., Vogel, J. R., Speth, T., Zuehlke, S., and Duennbier, U. (2003). Occurrence of endocrine-disrupting and other wastewater compounds during water treatment with case studies from lincoln, Nebraska and Berlin, Germany. Pract. Periodical Hazard. Toxic, Radioact. Waste Manag. 7, 253–263. doi:10.1061/(asce)1090-025x(2003)7:4(253)
Vesel, A., Zaplotnik, R., Primc, G., and Mozetič, M. (2019). Synthesis of vertically oriented graphene sheets or carbon nanowalls—review and challenges. Materials 12, 2968. doi:10.3390/ma12182968
Wang, F. (2017). Effect of oxygen-containing functional groups on the adsorption of cationic dye by magnetic graphene nanosheets. Chem. Eng. Res. Des. 128, 155–161. doi:10.1016/j.cherd.2017.10.007
Wang, F., Haftka, J. J.-H., Sinnige, T. L., Hermens, J. L., and Chen, W. (2014). Adsorption of polar, nonpolar, and substituted aromatics to colloidal graphene oxide nanoparticles. Environ. Pollut. 186, 226–233. doi:10.1016/j.envpol.2013.12.010
Wang, F., Wang, F., Zhu, D., and Chen, W. (2015). Effects of sulfide reduction on adsorption affinities of colloidal graphene oxide nanoparticles for phenanthrene and 1-naphthol. Environ. Pollut. 196, 371–378. doi:10.1016/j.envpol.2014.10.027
Wang, H., Chen, Y., and Wei, Y. (2016). A novel magnetic calcium silicate/graphene oxide composite material for selective adsorption of acridine orange from aqueous solutions. Rsc Adv. 6, 34770–34781. doi:10.1039/c6ra07625d
Wang, J., Zhang, J., Han, L., Wang, J., Zhu, L., and Zeng, H. (2021). Graphene-based materials for adsorptive removal of pollutants from water and underlying interaction mechanism. Adv. Colloid Interface Sci. 289, 102360. doi:10.1016/j.cis.2021.102360
Wang, S., Ning, H., Hu, N., Huang, K., Weng, S., Wu, X., et al. (2019). Preparation and characterization of graphene oxide/silk fibroin hybrid aerogel for dye and heavy metal adsorption. Compos. Part B Eng. 163, 716–722. doi:10.1016/j.compositesb.2018.12.140
Wang, S., Sun, H., Ang, H.-M., and Tadé, M. O. (2013). Adsorptive remediation of environmental pollutants using novel graphene-based nanomaterials. Chem. Eng. J. 226, 336–347. doi:10.1016/j.cej.2013.04.070
Wang, X., Liu, Y., Pang, H., Yu, S., Ai, Y., Ma, X., et al. (2018). Effect of graphene oxide surface modification on the elimination of Co (ii) from aqueous solutions. Chem. Eng. J. 344, 380–390. doi:10.1016/j.cej.2018.03.107
Wang, Z., Colombi Ciacchi, L., and Wei, G. (2017). Recent advances in the synthesis of graphene-based nanomaterials for controlled drug delivery. Appl. Sci. 7, 1175. doi:10.3390/app7111175
Wani, A. A., Khan, A. M., Manea, Y. K., Salem, M. A., and Shahadat, M. (2021). Selective adsorption and ultrafast fluorescent detection of Cr (vi) in wastewater using neodymium doped polyaniline supported layered double hydroxide nanocomposite. J. Hazard. Mater. 416, 125754. doi:10.1016/j.jhazmat.2021.125754
Wani, A. A., Shahadat, M., Ali, S. W., Ahammad, S. Z., and Uddin, M. K. (2022). Recent advances and future perspectives of polymer-based magnetic nanomaterials for detection and removal of radionuclides: a review. J. Mol. Liq. 365, 119976. doi:10.1016/j.molliq.2022.119976
Wei, D., Zhao, C., Khan, A., Sun, L., Ji, Y., Ai, Y., et al. (2019). Sorption mechanism and dynamic behavior of graphene oxide as an effective adsorbent for the removal of chlorophenol based environmental-hormones: a dft and Md simulation study. Chem. Eng. J. 375, 121964. doi:10.1016/j.cej.2019.121964
Wu, L., Qin, Z., Zhang, L., Meng, T., Yu, F., and Ma, J. (2017a). Cnt-enhanced amino-functionalized graphene aerogel adsorbent for highly efficient removal of formaldehyde. New J. Chem. 41, 2527–2533. doi:10.1039/c6nj03643k
Wu, S., Zhang, K., Wang, X., Jia, Y., Sun, B., Luo, T., et al. (2015). Enhanced adsorption of cadmium ions by 3d sulfonated reduced graphene oxide. Chem. Eng. J. 262, 1292–1302. doi:10.1016/j.cej.2014.10.092
Wu, Z., Yuan, X., Zhong, H., Wang, H., Jiang, L., Zeng, G., et al. (2017b). Highly efficient adsorption of Congo red in single and binary water with cationic dyes by reduced graphene oxide decorated nh2-mil-68 (Al). J. Mol. Liq. 247, 215–229. doi:10.1016/j.molliq.2017.09.112
Xu, P., Zeng, G. M., Huang, D. L., Feng, C. L., Hu, S., Zhao, M. H., et al. (2012). Use of iron oxide nanomaterials in wastewater treatment: a review. Sci. Total Environ. 424, 1–10. doi:10.1016/j.scitotenv.2012.02.023
Yang, G., Li, L., Lee, W. B., and Ng, M. C. (2018a). Structure of graphene and its disorders: a review. Sci. Technol. Adv. Mater. 19, 613–648. doi:10.1080/14686996.2018.1494493
Yang, Q., Lu, R., Ren, S., Chen, C., Chen, Z., and Yang, X. (2018b). Three dimensional reduced graphene oxide/zif-67 aerogel: effective removal cationic and anionic dyes from water. Chem. Eng. J. 348, 202–211. doi:10.1016/j.cej.2018.04.176
Yang, Q., Wang, J., Zhang, W., Liu, F., Yue, X., Liu, Y., et al. (2017a). Interface engineering of metal organic framework on graphene oxide with enhanced adsorption capacity for organophosphorus pesticide. Chem. Eng. J. 313, 19–26. doi:10.1016/j.cej.2016.12.041
Yang, T., Lin, H., Zheng, X., Loh, K. P., and Jia, B. (2017b). Tailoring pores in graphene-based materials: from generation to applications. J. Mater. Chem. A 5, 16537–16558. doi:10.1039/c7ta04692h
Yang, W., Lucotti, A., Tommasini, M., and Chalifoux, W. A. (2016). Bottom-up synthesis of soluble and narrow graphene nanoribbons using alkyne benzannulations. J. Am. Chem. Soc. 138, 9137–9144. doi:10.1021/jacs.6b03014
Yap, P. L., Nine, M. J., Hassan, K., Tung, T. T., Tran, D. N., and Losic, D. (2021). Graphene-based sorbents for multipollutants removal in water: a review of recent progress. Adv. Funct. Mater. 31, 2007356. doi:10.1002/adfm.202007356
Yari, M., Norouzi, M., Mahvi, A. H., Rajabi, M., Yari, A., Moradi, O., et al. (2016). Removal of Pb (ii) ion from aqueous solution by graphene oxide and functionalized graphene oxide-thiol: effect of cysteamine concentration on the bonding constant. Desalination Water Treat. 57, 11195–11210. doi:10.1080/19443994.2015.1043953
Yin, Z., Cui, C., Chen, H., Duoni, D., Yu, X., and Qian, W. (2020). The application of carbon nanotube/graphene-based nanomaterials in wastewater treatment. Small 16, 1902301. doi:10.1002/smll.201902301
Yu, L., Wang, L., Xu, W., Chen, L., Fu, M., Wu, J., et al. (2018). Adsorption of vocs on reduced graphene oxide. J. Environ. Sci. 67, 171–178. doi:10.1016/j.jes.2017.08.022
Yu, S., Wang, X., Yao, W., Wang, J., Ji, Y., Ai, Y., et al. (2017). Macroscopic, spectroscopic, and theoretical investigation for the interaction of phenol and naphthol on reduced graphene oxide. Environ. Sci. Technol. 51, 3278–3286. doi:10.1021/acs.est.6b06259
Yu, S., Wei, D., Shi, L., Ai, Y., Zhang, P., and Wang, X. (2019). Three-dimensional graphene/titanium dioxide composite for enhanced U (vi) capture: insights from batch experiments, xps spectroscopy and dft calculation. Environ. Pollut. 251, 975–983. doi:10.1016/j.envpol.2019.04.127
Zare, P., Aleemardani, M., Seifalian, A., Bagher, Z., and Seifalian, A. M. (2021). Graphene oxide: opportunities and challenges in biomedicine. Nanomaterials 11, 1083. doi:10.3390/nano11051083
Zhan, W., Gao, L., Fu, X., Siyal, S. H., Sui, G., and Yang, X. (2019). Green synthesis of amino-functionalized carbon nanotube-graphene hybrid aerogels for high performance heavy metal ions removal. Appl. Surf. Sci. 467, 1122–1133. doi:10.1016/j.apsusc.2018.10.248
Zhang, X., Shen, Q., Zhang, X., Pan, H., and Lu, Y. (2016). Graphene oxide-filled multilayer coating to improve flame-retardant and smoke suppression properties of flexible polyurethane foam. J. Mater. Sci. 51, 10361–10374. doi:10.1007/s10853-016-0247-3
Zhang, Y., Jiao, Z., Hu, Y., Lv, S., Fan, H., Zeng, Y., et al. (2017). Removal of tetracycline and oxytetracycline from water by magnetic Fe 3 O 4@ graphene. Environ. Sci. And Pollut. Res. 24, 2987–2995. doi:10.1007/s11356-016-7964-7
Zhang, Y., Yan, L., Xu, W., Guo, X., Cui, L., Gao, L., et al. (2014). Adsorption of Pb (ii) and Hg (ii) from aqueous solution using magnetic cofe2o4-reduced graphene oxide. J. Mol. Liq. 191, 177–182. doi:10.1016/j.molliq.2013.12.015
Zhao, C., Pei, S., Ma, J., Song, Z., Xia, H., Song, X., et al. (2020). Influence of graphene oxide nanosheets on the cotransport of Cu-tetracycline multi-pollutants in saturated porous media. Environ. Sci. Pollut. Res. 27, 10846–10856. doi:10.1007/s11356-020-07622-w
Zhao, G., Li, J., Ren, X., Chen, C., and Wang, X. (2011). Few-layered graphene oxide nanosheets as superior sorbents for heavy metal ion pollution management. Environ. Sci. Technol. 45, 10454–10462. doi:10.1021/es203439v
Zhao, G., Li, X., Huang, M., Zhen, Z., Zhong, Y., Chen, Q., et al. (2017a). The physics and chemistry of graphene-on-surfaces. Chem. Soc. Rev. 46, 4417–4449. doi:10.1039/c7cs00256d
Zhao, H., Ding, R., Zhao, X., Li, Y., Qu, L., Pei, H., et al. (2017b). Graphene-based nanomaterials for drug and/or gene delivery, bioimaging, and tissue engineering. Drug Discov. Today 22, 1302–1317. doi:10.1016/j.drudis.2017.04.002
Zhao, J., Wang, Z., White, J. C., and Xing, B. (2014). Graphene in the aquatic environment: adsorption, dispersion, toxicity and transformation. Environ. Sci. Technol. 48, 9995–10009. doi:10.1021/es5022679
Zheng, H., Gao, Y., Zhu, K., Wang, Q., Wakeel, M., Wahid, A., et al. (2018). Investigation of the adsorption mechanisms of Pb(II) and 1-naphthol by β-cyclodextrin modified graphene oxide nanosheets from aqueous solution. J. Colloid Interface Sci. 530, 154–162. doi:10.1016/j.jcis.2018.06.083
Zhi, L., and Müllen, K. (2008). A bottom-up approach from molecular nanographenes to unconventional carbon materials. J. Mater. Chem. 18, 1472–1484. doi:10.1039/b717585j
Zhou, X., Huang, W., Shi, J., Zhao, Z., Xia, Q., Li, Y., et al. (2014). A novel mof/graphene oxide composite gro@ mil-101 with high adsorption capacity for acetone. J. Mater. Chem. A 2, 4722–4730. doi:10.1039/c3ta15086k
Zhu, Y., Murali, S., Cai, W., Li, X., Suk, J. W., Potts, J. R., et al. (2010). Graphene and graphene oxide: synthesis, properties, and applications. Adv. Mater. 22, 3906–3924. doi:10.1002/adma.201001068
Keywords: graphene, nanomaterials, functionalization, decontamination, photo catalysis, toxicity
Citation: Assad H, Lone IA, Kumar A and Kumar A (2024) Unveiling the contemporary progress of graphene-based nanomaterials with a particular focus on the removal of contaminants from water: a comprehensive review. Front. Chem. 12:1347129. doi: 10.3389/fchem.2024.1347129
Received: 30 November 2023; Accepted: 10 January 2024;
Published: 14 February 2024.
Edited by:
Hyunook Kim, University of Seoul, Republic of KoreaReviewed by:
Wenluan Zhang, University of Electronic Science and Technology of China, ChinaZhenyuan Xia, Chalmers University of Technology, Sweden
Copyright © 2024 Assad, Lone, Kumar and Kumar. This is an open-access article distributed under the terms of the Creative Commons Attribution License (CC BY). The use, distribution or reproduction in other forums is permitted, provided the original author(s) and the copyright owner(s) are credited and that the original publication in this journal is cited, in accordance with accepted academic practice. No use, distribution or reproduction is permitted which does not comply with these terms.
*Correspondence: Ashish Kumar, drashishchemlpu@gmail.com