Estimation of Minimum Biofilm Eradication Concentration (MBEC) on In Vivo Biofilm on Orthopedic Implants in a Rodent Femoral Infection Model
- Department of Orthopaedic Surgery, Graduate School of Medicine, Kyoto University, Kyoto, Japan
The formation of a biofilm on the implant surface is a major cause of intractable implant-associated infection. To investigate the antibiotic concentration needed to eradicate the bacteria inside a biofilm, the minimum biofilm eradication concentration (MBEC) has been used, mostly against in vitro biofilms on plastic surfaces. To produce a more clinically relevant environment, an MBEC assay against biofilms on stainless-steel implants formed in a rat femoral infection model was developed. The rats were implanted with stainless steel screws contaminated by two Staphylococcus aureus strains (UAMS-1, methicillin-sensitive Staphylococcus aureus; USA300LAC, methicillin-resistant Staphylococcus aureus) and euthanized on days 3 and 14. Implants were harvested, washed, and incubated with various concentrations (64–4096 μg/mL) of gentamicin (GM), vancomycin (VA), or cefazolin (CZ) with or without an accompanying systemic treatment dose of VA (20 μg/mL) or rifampicin (RF) (1.5 μg/mL) for 24 h. The implant was vortexed and sonicated, the biofilm was removed, and the implant was re-incubated to determine bacterial recovery. MBEC on the removed biofilm and implant was defined as in vivo MBEC and in vivo implant MBEC, respectively, and the concentrations of 100% and 60% eradication were defined as MBEC100 and MBEC60, respectively. As for in vivo MBEC, MBEC100 of GM was 256–1024 μg/mL, but that of VA and CZ ranged from 2048–4096 μg/mL. Surprisingly, the in vivo implant MBEC was much higher, ranging from 2048 μg/mL to more than 4096 μg/mL. The addition of RF, not VA, as a secondary antibiotic was effective, and MBEC60 on day 3 USA300LAC biofilm was reduced from 1024 μg/mL with GM alone to 128 μg/mL in combination with RF and the MBEC60 on day 14 USA300LAC biofilm was reduced from 2048 μg/mL in GM alone to 256 μg/mL in combination with RF. In conclusion, a novel MBEC assay for in vivo biofilms on orthopedic implants was developed. GM was the most effective against both methicillin-sensitive and methicillin-resistant Staphylococcus aureus, in in vivo biofilms, and the addition of a systemic concentration of RF reduced MBEC of GM. Early initiation of treatment is desired because the required concentration of antibiotics increases with biofilm maturation.
Introduction
Orthopedic infections remain a major clinical problem, with catastrophic costs to healthcare systems (Castaneda et al., 2016). Infections associated with orthopedic implants continue to be life-threatening and devastating complications after orthopedic surgery. Staphylococcus aureus (S. aureus) and coagulase-negative staphylococci (CNS) are commonly found bacteria in implant-related infections. Among them, methicillin-resistant S. aureus (MRSA) is known to be the most difficult to treat organism. Previous studies have reported a 72% cure rate for methicillin-sensitive S. aureus (MSSA) infections, whereas the cure rate dropped to 57% for MRSA (Teterycz et al., 2010).
The formation of biofilms on implants is one of the major reasons for failure in cases of implant-associated infection (Masters et al., 2019; Saeed et al., 2019). Biofilms act as a physical barrier and protect bacteria from attack by the host’s immune cells or antimicrobial agents (Zhang et al., 2020) Bacteria on the surface of implants are hidden within the biofilm, preventing host immune cells and antibiotics from attacking them (Costerton, 2005; Flemming and Wingender, 2010; Masters et al., 2019). In addition, the bacteria present in the biofilm acquire pathogenicity and antimicrobial resistance through their ability to exchange information with each other through the quorum sensing mechanism (Rutherford and Bassler, 2012). Biofilm formation occurs in several stages. These stages, which have been observed in both in vitro and in vivo biofilms, begin with initial attachment, followed by proliferation, biofilm formation, and spread of the mature biofilm (Costerton et al., 1987; O’Toole et al., 2000; Nishitani et al., 2015). In the very early stages of infection, when the biofilm is immature, it may be possible to deal with an implant-associated infection by the administration of standard systemic antibiotics. However, once the biofilm matures, it is difficult to eradicate bacteria within the biofilm using standard antimicrobial treatment (Tomizawa et al., 2021). A high-dose, local antibiotic regimen may have the potential to treat mature biofilms, but effective antibiotics and their effective concentrations are not known.
The efficacy of antibiotics against bacteria is commonly represented by the use of the minimum inhibitory concentration (MIC). MIC is defined as “the minimum concentration of an antibiotic to inhibit bacterial growth” (Li et al., 2017). MIC is generally ascertained by exposing defined amounts of bacteria to increasing concentrations of an antibiotic. However, the methods to derive the measurement are performed on planktonic bacteria in a growth medium. Most bacteria are in biofilm form in orthopedic infections and not in planktonic form (Stoodley et al., 2011; Li et al., 2017). Currently available antibiotic treatments are based on the MIC against the planktonic form of bacteria, and these concentrations are less effective against bacteria in the biofilm, which needs to be up to 1000 times higher. (Verderosa et al., 2019) Thus, the usefulness of MIC for orthopedic implant-associated infections may be limited.
The concept of the minimum biofilm eradication concentration (MBEC) has been proposed as an antibiotic concentration to eradicate bacteria in biofilms (Evans and Holmes, 1987; Girard et al., 2010; Macià et al., 2014). Although MBEC seemed more useful in clinical practice, there are still questions regarding the most appropriate methodologies to measure MBEC. In most current MBEC assays, biofilms are formed on abiotic surfaces, such as polystyrene microtiter well plates, which likely have a different affinity for the attachment of bacteria from the materials used in orthopedic implants, such as titanium alloy or stainless steel (Ceri et al., 2001). Many studies have investigated the effects of antibiotics on biofilms in vitro or ex vivo, but no study has investigated the real in vivo biofilms formed in host animals (Fujimura et al., 2008; Rose and Poppens, 2009; Zimmerli and Sendi, 2019). Compared to in vitro biofilms, in vivo biofilms are more complex structures composed of bacterial polysaccharides, proteins, DNA, and host structures such as fibrin, fibrinogen, and collagen (Høiby et al., 2010; Nishitani et al., 2015).
To overcome these issues, this study measured MBEC using several related methodologies, First, MBEC was measured using an in vitro biofilm formed on a stainless-steel screw. Furthermore, an ambitious attempt was made to evaluate MBEC on in vivo biofilms with orthopedic implants using immature and mature in vivo biofilms formed on stainless steel screw implants in a rodent infection model.
A related aim of the study was to investigate the clinical utility of standard of care (SOC) antibiotics on in vivo biofilms. To that end, this study investigated the in vitro and in vivo MBEC of gentamicin (GM), cefazolin (CZ), and vancomycin (VA). A selection of these initial assays was then assessed in combination with a systemic dose of either VA or rifampicin (RF) to reflect the usual clinical practice of accompanying local antibiotic therapy with systemic antibiotics.
The purposes of this study were to establish an MBEC assay for orthopedic implants, with a special focus on using in vivo biofilms formed in rodents, and to investigate the utility of standard of care (SOC) antibiotics on in vivo biofilms.
Materials and Methods
Two S. aureus strains were used in this study. One was UAMS-1, which is a widely used methicillin-sensitive S. aureus (MSSA) strain isolated from an osteomyelitis patient (Gillaspy et al., 1995), and the other was USA300LAC, which is one of the most prevalent and virulent methicillin-resistant S. aureus (MRSA) strains (Kourbatova et al., 2005). All strains were cultured in a tryptic soy broth (TSB) medium. MIC of two strains was determined by measuring the lowest concentration of antibiotics which prevents visible growth of S. aureus on the TSB agar plate.
In Vitro MBEC Assay
To evaluate MBEC in orthopedic implants, the MBEC assay was replicated using a stainless-steel screw implant (Figure 1A). Sterilized stainless-steel screws (1.2 mm × 8 mm, Esco, Osaka, Japan) were incubated in 250 mL of TSB with S. aureus for 24 h (1 day) or 72 h (3 days) shaking at 150 rpm on 96-well polystyrene flat-bottom microtiter plates (Corning, REF 3596, Corning, NY, USA) to allow bacteria to form a biofilm on the implant. The implants were taken out and washed twice with phosphate buffer saline (PBS) to remove any unattached bacteria. The implants were again placed in microtiter plates and treated with various concentrations of antimicrobial agents (GM, VA, and CZ) with or without a systemic treatment dose of VA (20 μg/mL) or RF (1.5 μg/mL) and shaken at 150 rpm at 37°C for 24 h. After 24 h, the implants were removed and placed in a 1.5 mL Eppendorf tube containing fresh TSB solution, and the biofilm adhering to the implants was removed using the Vortex-Sonication-Vortex Method (VSVM) (Oliva et al., 2013; Kırmusaoğlu, 2019). The TSB supernatant was then replaced in a microtiter plate and shaken at 150 rpm at 37°C for 24 h. The recovery of S. aureus was quantitatively analyzed by the colony-forming units (CFU) assay by counting the colony number on TSB agar plates. The MBEC in this experiment was defined as an in vitro MBEC.
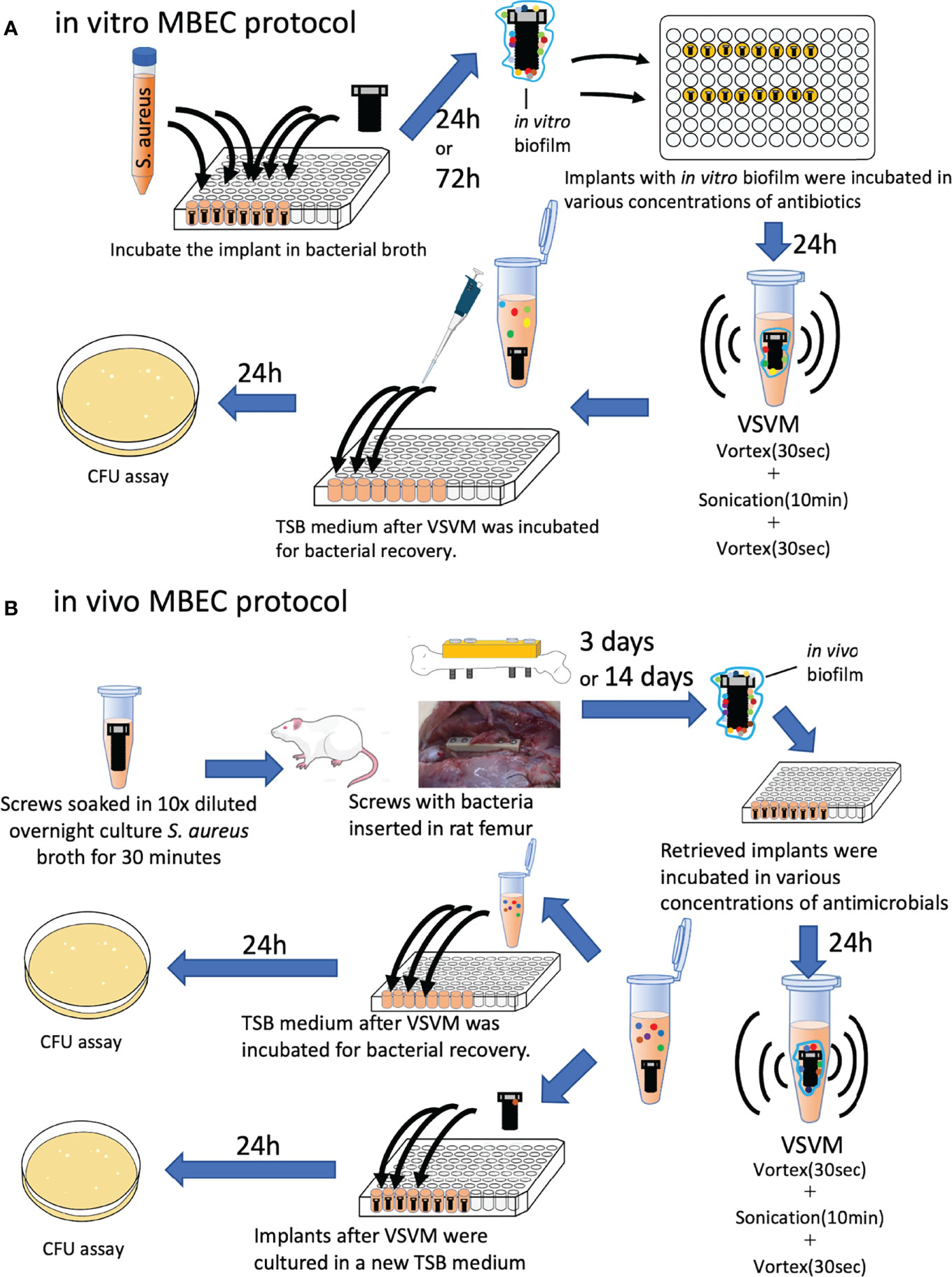
Figure 1 Schematic protocols of (A) in vitro and in vivo (B) MBEC assay in this study. S. aureus: Staphylococcus aureus, VSVM, Vortex-Sonication-Vortex Method, CFU, colony-forming unit.
In Vivo MBEC Assay
All animal experiments were conducted with the approval of the university’s animal resources committee and in accordance with national guidelines. Twenty-week-old male Wistar rats were purchased from Japan SLC (Hamamatsu, Japan) and acclimatized to the new environment for 1 week before the interventions. Two rats were housed in one cage with free access to water and standard rodent chow. A constant 12-h light/dark cycle was maintained. After sterilization, the screws were immersed in a 10-fold dilution of an overnight S. aureus culture for 30 min. The inoculation load was 6× 103 colony forming units (CFU)/implant. Rats were intraperitoneally administered a combination anesthetic containing 0.375 mg/kg medetomidine, 2 mg/kg midazolam, and 2.5 mg/kg butorphanol to induce anesthesia, which was maintained with 3% isoflurane. Approaches and plating were based on the techniques described in previous reports (Poser et al., 2014; Kawai et al., 2021). After iodine disinfection, a 4-cm skin incision was made on the left lateral thigh and the femur was exposed. A polyetheretherketone plate (4-mm wide, 24-mm long, 2-mm thick with six 1.0-mm screw holes arranged in a line along its length, Umihira, Kyoto, Japan) was placed on the femur, and the femur was drilled with a 1.0 mm drill using the two most proximal and distal holes in the plate. After tapping, four contaminated screws were inserted into the drilled holes. Buprenorphine (0.02 mg/kg) was administered until day 2. After 3 and 14 days, the rats were euthanized by carbon dioxide overdose, and implants with in vivo biofilms were harvested (Figure 1B). The implants were washed twice with PBS to remove additional bacteria and extra soft tissues, and screws were incubated with various concentrations of antimicrobial agents (GM, VA, and CZ) with or without a systemic treatment dose of VA (20 μg/mL) or RF (1.5 μg/mL) for 24 h shaking at 150 rpm at 37°C. As previously described, the biofilm adhering to the implants was removed using the VSVM. The TSB supernatant was then replaced in a microtiter plate and shaken at 150 rpm at 37°C for 24 h. The recovery of S. aureus was quantitatively analyzed using the CFU assay, and the MBEC in this experiment was defined as the in vivo MBEC.
As an additional experiment, implants after VSVM were incubated in TSB for 24 h, and CFU recovery was examined to take into account the presence of bacteria in the residual biofilm that had not been removed after VSVM. The MBEC in this experiment was defined as the in vivo implant MBEC.
SEM and EDS Analysis for Biofilm
Scanning electron microscopy (SEM) has been shown to be a suitable tool not only to observe the substratum morphology in detail but also to follow bacterial adhesion and biofilm formation. Energy dispersive X-Ray spectroscopy (EDS) has been shown to be a suitable tool for observing mineral structure formation by bacterial and microalgal biofilms growing on the surface (Abed et al., 2012; Gomes and Mergulhão, 2017). For SEM, additional implants were prepared with USA300LAC incubated for 24 h in vitro and for 3 or 14 days in vivo in the rat femur. With or without antibiotic (GM or GM +RF) treatment, the implants were fixed overnight in 2.5% glutaraldehyde and 4% paraformaldehyde. Biofilms on the implants were evaluated using SEM (JSM-7900F, JEOL, Akishima, Japan) and EDS (JED-2300F, JEOL).
Statistics
To determine in vitro MBEC, five screws were used for each experimental condition, and the antibiotic concentration that achieved 100% eradication of bacteria in the biofilm was defined as MBEC100 and the concentration which achieved 60% eradication of bacteria was defined as MBEC60. For the in vivo experiments, two rats housed in one cage were defined as one set of animals, and the MBEC of each set was determined. Five MBECs were obtained from 10 rats per experimental conditions, and MBEC100 and MBEC60 were defined as described before. For animal experiments, MBEC among the three antibiotics (GM, VA, and CZ) or the effect of additional antibiotics (no additional antibiotic, VA, RF) were also statistically compared using the Kruskal–Wallis test with Dunn’s multiple comparison, and p < 0.05 was considered significant.
Results
In Vitro MBEC
The MIC and MBEC of GM, VA, and CZ on bacteria in the in vitro biofilm against UAMS-1 and USA300LAC are shown in Table 1. The MBEC100 values of the three antibiotics were higher than the MIC. For UAMS-1, VA had the lowest MBEC60 for day 1 biofilm, whereas GM had the lowest MBEC100 for both day 1 and day 3 biofilm. Against USA300LAC, GM had the lowest MBEC60. The MBEC100 values of GM and VA were similar for day 1, but GM had the lowest MBEC100 for day 3. Against both UAMS-1 and USA300LAC, the MBEC60 and MBEC100 of CZ were the highest.
In Vivo MBEC
In this surgical model, infection was limited locally in all rats, and physical debilitation was not observed. No infection-related deaths were observed during the experiment. For UAMS-1, GM had the lowest MBECs among the three antimicrobial agents on both days 3 and 14, although the difference was not statistically significant (Figures 2A, B, Table 2). A very high concentration of more than 1024 μg/mL was required for MBEC60 and MBEC100 for VA and CZ. For GM and VA, with the maturation of the biofilm by day 14, MBEC100 tended to increase. Similarly, in the USA300LAC group, GM was the most effective among the three antimicrobial agents on both days 3 and 14, with statistical significance (Figures 2C, D, Table 2). In the case of GM, the longer the implant was incubated, the higher the MBEC100. However, MBEC100 for VA and CZ did not differ between days 3 and 14.
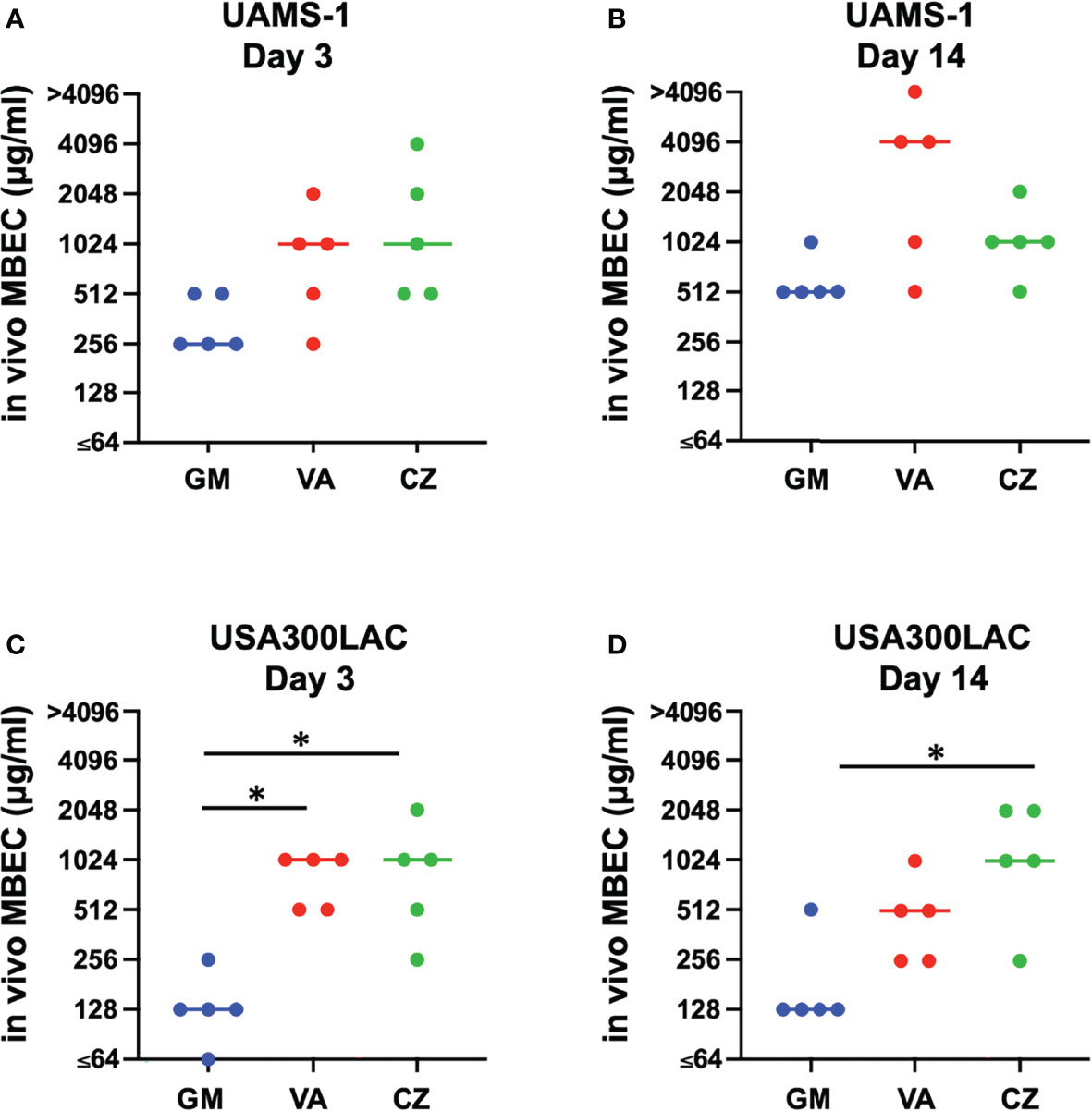
Figure 2 Minimal biofilm eradication concentration of in vivo biofilm formed on the implant incubated in rat femur for 3 days and 14 days (in vivo MBEC) of UAMS-1 (A, B) and USA300LAC (C, D). Bars in each graph indicate the median of five assays. *: p < 0.05 by Kruskal-Wallis test with Dunn’s multiple comparison. GM, gentamicin; VA, vancomycin; CZ, cefazolin.
SEM Analysis for In Vivo Biofilm
A small amount of biofilm was observed in in vitro day 1 and in vivo day 3 infection with USA300LAC (Figures 3A, C). Although simple matrix formation surrounded by bacterial clusters was observed on the in vitro implant, bacterial clusters were entangled in a matrix with fiber-like structures on the in vivo implant (Figures 3B, D). Compared to day 3, a considerable amount of biofilm was observed in the screw groove after 14 days of infection with USA300LAC (Figures 4A, B). Even after GM treatment and VSVM, residual biofilm was still observed on the implant with live bacteria (Figures 4C, D). EDS showed that residual tissue on the implant after VSVM was not metallic material but tissue from an organism containing much more carbon and less iron and chrome (Figures 4E, F).
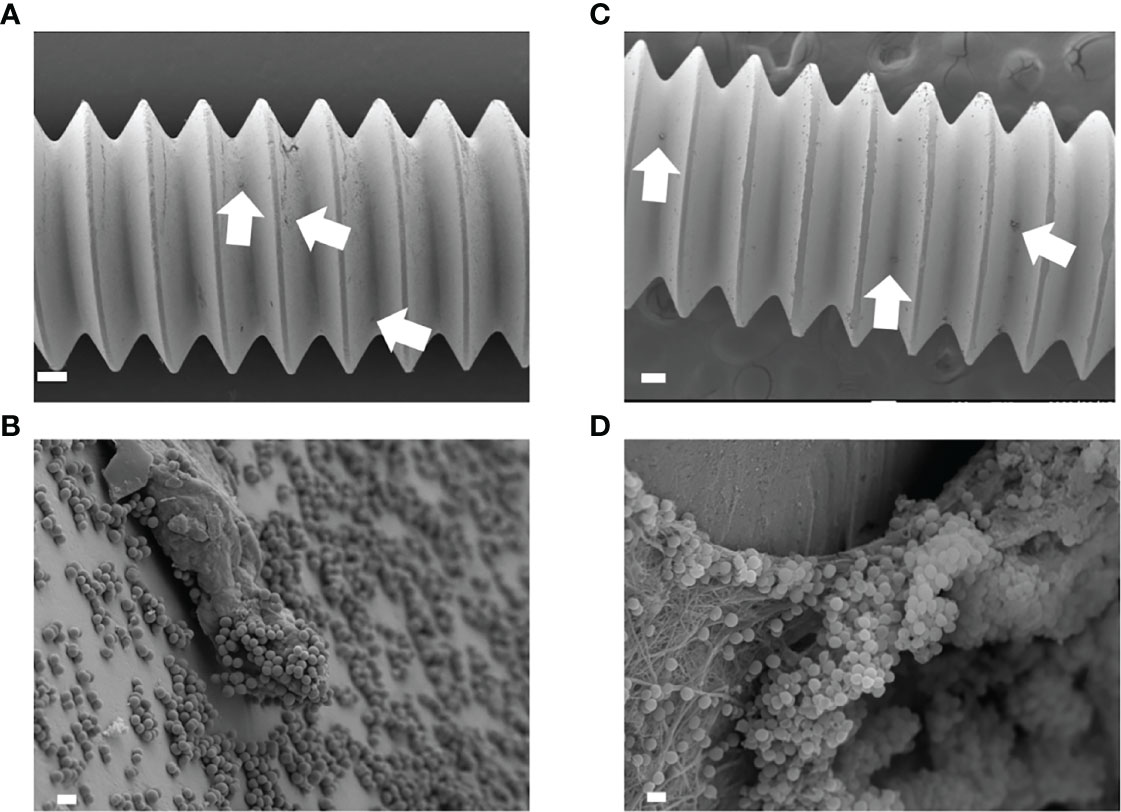
Figure 3 Scanning electron microscopy (SEM) image of USA300LAC infected implant of in vitro day 1 (A, B) and in vivo day 3 (C, D). In low magnification images, a small amount of biofilm formations were observed (white arrow) (A, C). White bars in A, C indicate 100 µm, and white bars in B, D indicate 1 µm.
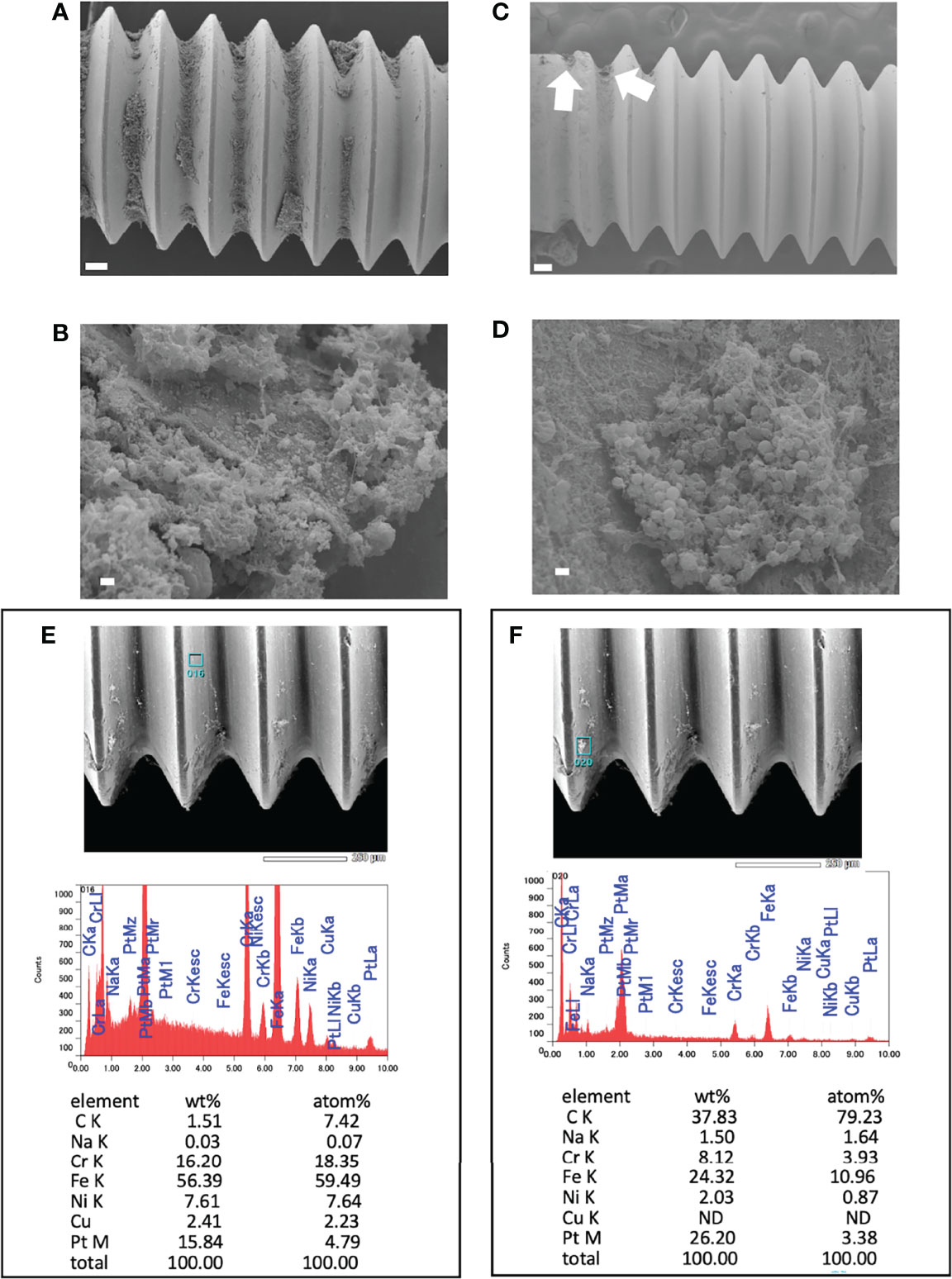
Figure 4 Scanning electron microscopy (SEM) image of USA300LAC infected implant of day 14 in vivo biofilm. (A, B) are images after 24 h of antibiotics treatment with 512 µg/mL gentamicin which showed robust biofilm formation in the screw grooves. (C, D) are images after vortex-sonication-vortex method (VSVM) which has a fare less biofilm compared to before VSVM (A), but a small amount of residual biofilm was observed (white arrows) which has live bacteria (D) in the matrix. Energy Dispersive X-Ray Spectroscopy (EDS) on the implant surface (E) and on the residual biofilm (F) after VSVM was shown with the percentage of containing elements. White bars in A and C indicate 100 μm, and white bars in B and D indicate 1 μm.
In Vivo Implant MBEC on Residual Biofilm
Because residual biofilm was confirmed on the implant after VSVM, bacterial recovery from the residual biofilm was also investigated. Surprisingly, MBECs for bacteria in residual biofilm were far higher than in vivo MBEC (Figure 5, Table 3). Although GM completely eradicated bacteria in the residual biofilm at 2048 μg/mL and 4096 μg/mL for USA300LAC, the other two antibiotics for USA300LAC and all three antibiotics for UAMS-1 on day 14 did not eradicate bacteria completely in the biofilm even with 4096 μg/mL.
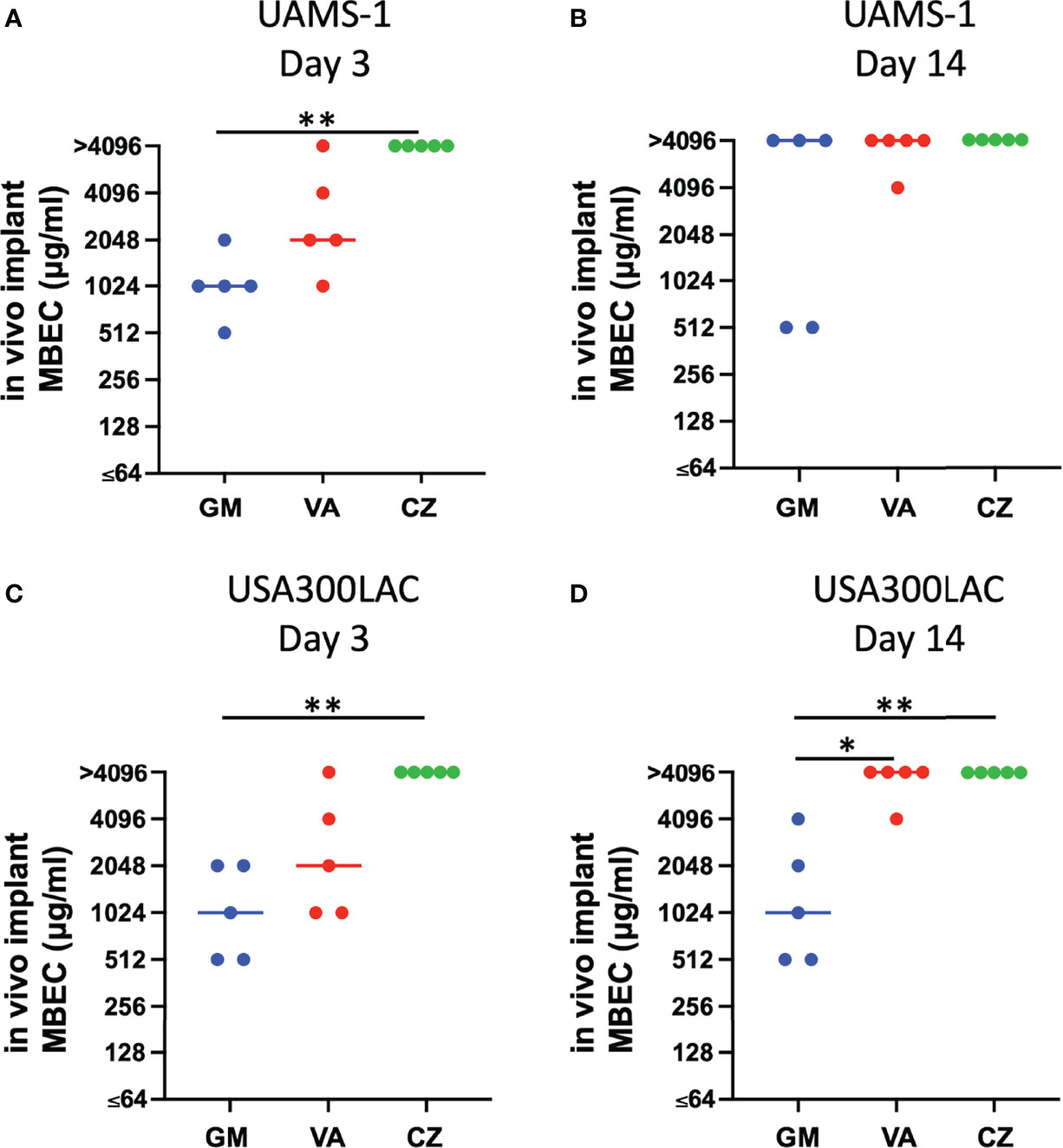
Figure 5 Minimal biofilm eradication concentration against residual in vivo biofilm after vortex-sonication-vortex-method. (in vivo implant MBEC) of UAMS-1 (A, B) and USA300LAC (C, D). Bars in each graph indicate the median of five assays. **: p < 0.01 and *: p < 0.05 by Kruskal–Wallis test with Dunn’s multiple comparison. GM, gentamicin; VA, vancomycin; CZ, cefazolin.
Additional Effect of Second Antibiotics
Local antibiotics are always administered along with systemic antibiotics. Since GM seemed to be the most effective among the three antibiotics for local administration, the additional effect of RF and VA with a systemic concentration on the MBEC of GM was investigated with USA300LAC. The addition of RF reduced the in vitro MBEC60 and MBEC100 of GM in both day 1 and day 3 (Table 4). As for in vivo MBEC, the addition of systemic doses of RF reduced the MBEC60 and MBEC100 of GM on day 14 biofilms (Table 5), but without a statistical difference (Figures 6A, B). For the residual biofilm on the implant after VSVM, although a very high concentration was still needed, RF reduced MBEC60 and MBEC100 of GM on both day 3 and day 14 biofilms (Table 6), with a significant difference on day 3 (Figures 6C, D). In contrast to RF, the addition of a systemic dose of VA had no effect on the MBEC of GM in all experiments. SEM showed very little residual biofilm and debris from the fibrous matrix from the implant treated with 512 GM μg/mL plus 1.5μg/mL RF (Figure 7).
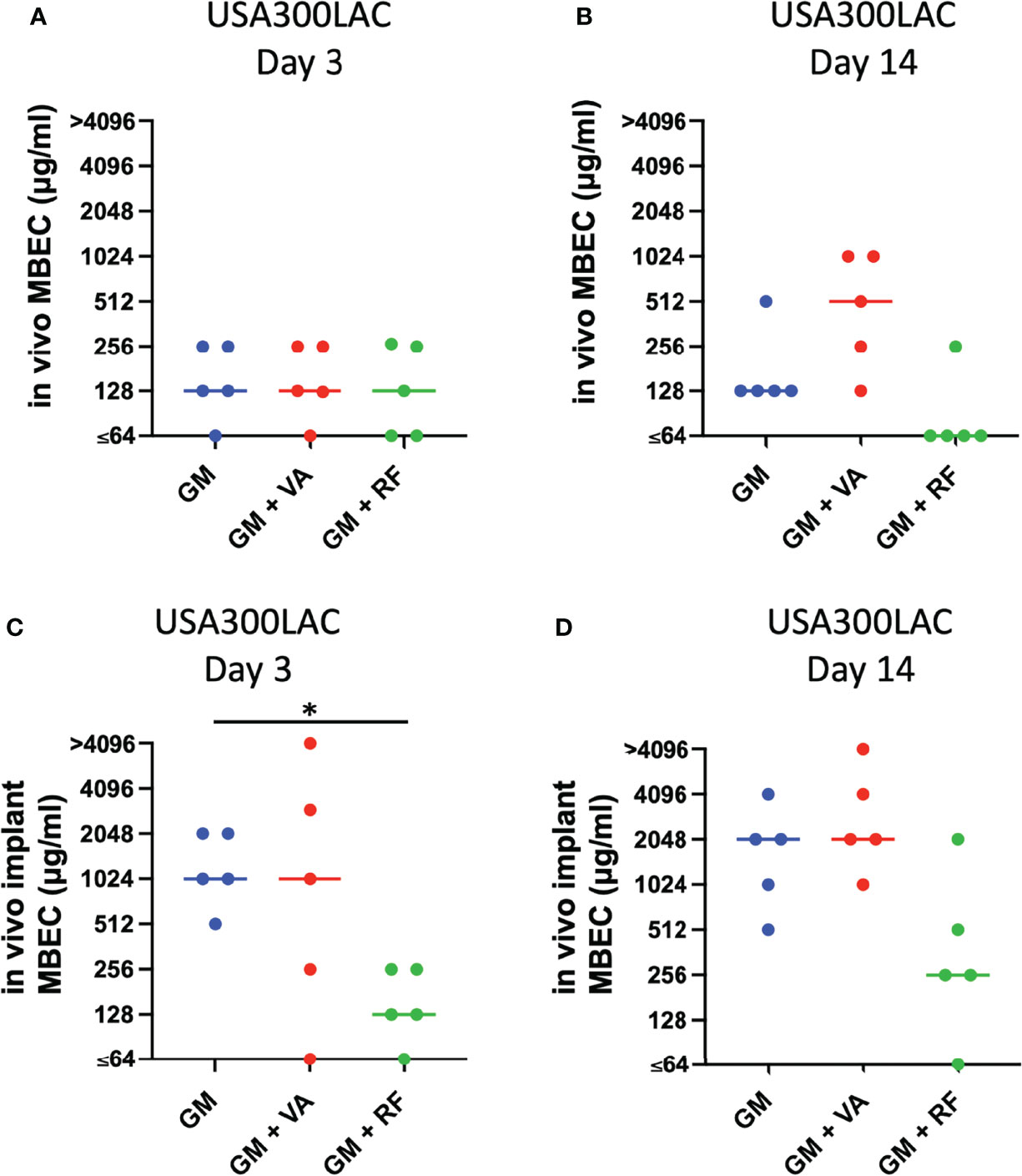
Figure 6 Effect of secondary antibiotics (VA: vancomycin, RF: rifampin) on in vivo minimal biofilm eradication concentration (MBEC) of GM (gentamicin) (A, B) and against residual in vivo biofilm after vortex-sonication-vortex-method on in vivo implant (in vivo implant MBEC) in combination with GM (gentamicin) (C, D) Bars in each graph indicate the median of five assays. *: p < 0.05 by Kruskal–Wallis test with Dunn’s multiple comparison.

Table 6 Additional effect of second antibiotics on MBEC of residual bacteria in in vivo biofilm on screw after VSVM (in vivo implant MBEC) of GM.
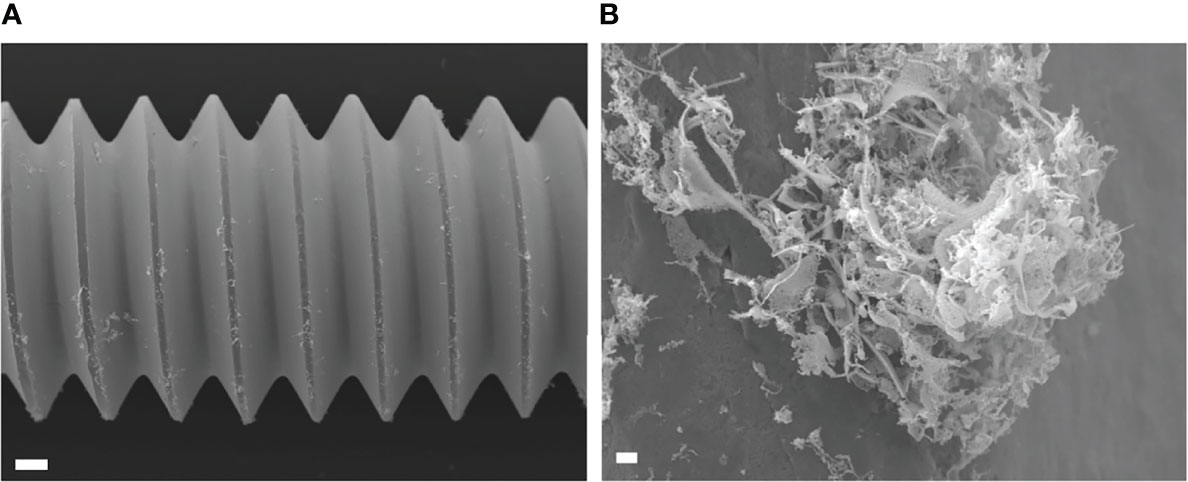
Figure 7 Scanning electron microscopy (SEM) image of USA300LAC infected implant of day 14 in vivo biofilm which was treated with 512 µm/mL gentamicin and rifampicin after vortex-sonication-vortex method. Although some residual tissues remained on the implant surface (A), only debris of fibrous matrix without live bacteria in it (B). White bars in A and B indicate 100 μm and 1 μm, respectively.
Discussion
In this study, the potential of SOC antibiotics to eradicate biofilm bacteria was evaluated. Notably, in vivo biofilms, which are far more resistant to antibiotics than in vitro biofilms, were formed on the implant in the rodent femur and utilized in this study. As expected, the in vivo MBEC was higher than the in vitro MBEC on stainless steel implants. Not surprisingly, although in vitro MBEC ranged from 64–1024 μg/mL, the in vivo MBEC was as high as 512–4096 μg/mL. When the implant was incubated for the same duration (3 days), bacteria in the in vivo biofilm were more resistant to antibiotics than in the in vitro biofilm. SEM analyses showed that remnants of the biofilm were firmly attached to the implant, and far higher concentrations were needed to eradicate bacteria from the residual biofilm. Among the three antibiotics, only GM had the potential to eradicate USA300LAC on day 14 biofilms in 60% of samples at 1024 μg/mL. This high concentration might be possible with a radical local administration, but cytotoxicity is a concern. A combination of systemic doses of second antibiotics was attempted, and the concentration of GM to obtain 60% effectivity was reduced to 256 μg/mL with RF 1.5 μg/mL in day 14 biofilms of USA300LAC.
We also expected that the MBEC would increase as the biofilm matured; thus, MBEC for day 14 biofilms was expected to be much higher than MBEC for day 3 biofilms, because biofilm maturation is reported at 14 days post-infection (Nishitani et al., 2015). Although this trend was confirmed in most cases, within the in vivo biofilms detailed in Table 2, GM used on USA300LAC showed the same MBEC60 on days 3 and 14, and CZ used on UAMS-1 had a lower MBEC100 on day 14 than it did on day 3. It was speculated that mature biofilms were firmly attached to the implant surface and difficult to remove in these cases. Although VSVM is an established method for removing biofilms, all the biofilms cannot be removed, and some biofilm remains on the implant (Rosa et al., 2019). In the present study, the presence of residual biofilm was confirmed using SEM after VSVM. Therefore, if antimicrobial agents do not penetrate deep into the biofilm, surviving bacteria may multiply again in the residual biofilm. To this end, the implants were cultured again for 24 h after VSVM to examine biofilm persistence. It is noteworthy that persistent biofilms on the implant surface needed a much higher concentration to eradicate the bacteria inside. This can be demonstrated by the finding that VA and CZ were almost ineffective at experimental concentrations of up to 4096 μg/mL in this study. GM was the most effective, but its MBEC100 on the residual biofilm was also very high at 2048 μg/mL for day 3 and 4096 μg/mL for day 14 biofilms. Even with the local administration of antibiotics, it seems difficult to reach these high concentrations in actual clinical practice. Therefore, a combination of antibiotics was considered, as a reduction of MBEC was reported by combining multiple antibiotics in previous studies (Deresinski, 2009) (Dall et al., 2018).
We used the concentration of a systemic administration dose to mimic the condition of the combination of local and systemic therapy. As GM had the lowest MBEC among the three antibiotics, the MBEC of GM was evaluated again in combination with a systemic dose of VA and RF. VA and RF were selected because they have been reported to penetrate deep into biofilms (Jacqueline and Caillon, 2014). The addition of a systemic dose of VA did not reduce the MBEC of GM. On the other hand, RF reduced the in vivo MBEC, especially in the in vivo implant MBEC on day 3. The reason for this may be that the bactericidal effect of VA in biofilms is inferior to that of RF and RF is reported to be more effective than VA in MRSA biofilm infections) (Jørgensen et al., 2016). Some in vivo animal studies have demonstrated the benefits of combined treatment with RF, and these studies have reported that the addition of RF significantly reduces CFU (Stavrakis et al., 2014; Thompson et al., 2017). Previous studies have also reported that sublethal doses of VA induce robust biofilm formation through the promotion of extracellular DNA-dependent release (Hsu et al., 2011; Abdelhady et al., 2014). The 20 μg/mL dose used in this study may be insufficient for bacteria in biofilms, but higher blood concentrations increase the risk of side effects. GM is effective in eradicating biofilms formed by S. aureus, including MRSA, and GM’s MBEC has been reported to be lower than that of VA (Mottola et al., 2016; Metsemakers et al., 2018). In addition, the synergistic effect of GM with other antimicrobial agents on staphylococcal biofilms has been reported previously (Dall et al., 2018). GM has a long history of local administration in the form of antibiotic-loaded cements (Vugt et al., 2019), and is also used for systemic administration by intramedullary antibiotic perfusion (Maruo et al., 2021). GM seemed a good candidate for local antibiotic treatment.
This study had some limitations. First, only one bacterial strain of MSSA and MRSA was investigated. Although these bacteria are representative strains that produce robust biofilms (Nishitani et al., 2015), the effectiveness of antibiotics against other S. aureus strains remains unknown. GM was the most effective among the three SOC antibiotics, and whether GM has similar efficiency against GM-resistant MRSA strains is unknown. Moreover, other bacterial strains that cause implant-associated infections have not yet been investigated. However, since we established a novel method to investigate in vivo MBEC with real implants, it became possible to investigate in vivo MBEC with various clinically acquired bacterial strains from patients with implant-associated infections. Second, three SOC antibiotics were selected, but there are many candidate antibiotics, including other anti-MRSA drugs (e.g., linezolid and daptomycin), that might be more effective. Third, stainless steel was used to grow the biofilm, but the MBEC of other implant materials, such as titanium alloy or cobalt-chrome, may be different. Fourth, antibiotic treatment was administered for only 24 h. Some reports have shown that a longer treatment duration reduced the required MBEC (Castaneda et al., 2016; Post et al., 2017). Although very high concentrations were needed for in vivo implant MBEC in this study, these high concentrations may not be needed when biofilms on implants are exposed to antibiotics for longer durations. This study only showed the concentration required to eradicate bacteria in biofilms on orthopedic implants within 24 h of treatment.
In conclusion, a novel MBEC assay for in vivo biofilms on orthopedic implants was developed. GM was the most effective SOC antibiotic against both MSSA and MRSA biofilms in both the in vitro and in vivo experiments. Although in this study GM + RF only showed its greater effect against MRSA on day 14, early initiation of treatment with antimicrobial intervention is still desirable in clinical practice because the required concentration of antibiotics increases with the maturation of the biofilm.
Data Availability Statement
The raw data supporting the conclusions of this article will be made available by the authors, without undue reservation.
Ethics Statement
The animal study was reviewed and approved by Kyoto University Animal Experiment Committee.
Author Contributions
KN conceived the ideas for experimental designs, analyzed data, and wrote the manuscript. YO conducted the experiments, analyzed data, and wrote the manuscript. AS and YK acquired findings and advised on interpretations. TK contributed to the development of the animal model. TT and MS conducted the experiments. SM contributed the concept and supervised the project. All authors approved the final version of the manuscript.
Funding
This study was supported by Grants-in-Aid for Scientific Research (19K09548, 19K09621, 22K09329).
Conflict of Interest
SM received honoraria for meeting chair and a scholarship donation for orthopaedic research from Diichi-Sankyo, outside of this study. This pharmaceutical company has no contribution or influence on this study.
The remaining authors declare that the research was conducted in the absence of any commercial or financial relationships that could be construed as a potential conflict of interest.
Publisher’s Note
All claims expressed in this article are solely those of the authors and do not necessarily represent those of their affiliated organizations, or those of the publisher, the editors and the reviewers. Any product that may be evaluated in this article, or claim that may be made by its manufacturer, is not guaranteed or endorsed by the publisher.
Acknowledgments
The authors thank Editage [http://www.editage.com] for editing and reviewing this manuscript for English language.
References
Abdelhady, W., Bayer, A. S., Seidl, K., Moormeier, D. E., Bayles, K. W., Cheung, A., et al. (2014). Impact of Vancomycin on sarA-Mediated Biofilm Formation: Role in Persistent Endovascular Infections Due to Methicillin-Resistant Staphylococcus Aureus. J. Infect. Dis. 209, 1231–1240. doi: 10.1093/infdis/jiu007
Abed, S. E., Ibnsouda, S. K., Latrache, H., Hamadi, F. (2012). Scanning Electron Microscopy (SEM) and Environmental SEM: Suitable Tools for Study of Adhesion Stage and Biofilm Formation. Scanning Electron Microscopy. Chapter 35. doi: 10.5772/34990
Castaneda, P., McLaren, A., Tavaziva, G., Overstreet, D. (2016). Biofilm Antimicrobial Susceptibility Increases With Antimicrobial Exposure Time. Clin. Orthop Relat. Res. 474, 1659–1664. doi: 10.1007/s11999-016-4700-z
Ceri, H., Olson, M., Morck, D., Storey, D., Read, R., Buret, A., et al. (2001). [25] The MBEC Assay System: Multiple Equivalent Biofilms for Antibiotic and Biocide Susceptibility Testing. Methods Enzymol. 337, 377–385. doi: 10.1016/s0076-6879(01)37026-x
Costerton, J. W. (2005). Biofilm Theory Can Guide the Treatment of Device-Related Orthopaedic Infections. Clin. Orthop Relat. Res. 437, 7–11. doi: 10.1097/00003086-200508000-00003
Costerton, J. W., Cheng, K. J., Geesey, G. G., Ladd, T. I., Nickel, J. C., Dasgupta, M., et al. (1987). Bacterial Biofilms in Nature and Disease. Annu. Rev. Microbiol. 41, 435–464. doi: 10.1146/annurev.mi.41.100187.002251
Dall, G. F., Tsang, S.-T. J., Gwynne, P. J., MacKenzie, S. P., Simpson, A. H. R. W., Breusch, S. J., et al. (2018). Unexpected Synergistic and Antagonistic Antibiotic Activity Against Staphylococcus Biofilms. J. Antimicrob. Chemother. 73, 1830–1840. doi: 10.1093/jac/dky087
Deresinski, S. (2009). Vancomycin in Combination With Other Antibiotics for the Treatment of Serious Methicillin-Resistant Staphylococcus Aureus Infections. Clin. Infect. Dis. 49, 1072–1079. doi: 10.1086/605572
Evans, R. C., Holmes, C. J. (1987). Effect of Vancomycin Hydrochloride on Staphylococcus Epidermidis Biofilm Associated With Silicone Elastomer. Antimicrob. Agents Chemother. 31, 889–894. doi: 10.1128/aac.31.6.889
Flemming, H.-C., Wingender, J. (2010). The Biofilm Matrix. Nat. Rev. Microbiol. 8, 623–633. doi: 10.1038/nrmicro2415
Fujimura, S., Sato, T., Mikami, T., Kikuchi, T., Gomi, K., Watanabe, A. (2008). Combined Efficacy of Clarithromycin Plus Cefazolin or Vancomycin Against Staphylococcus Aureus Biofilms Formed on Titanium Medical Devices. Int. J. Antimicrob. Agents 32, 481–484. doi: 10.1016/j.ijantimicag.2008.06.030
Gillaspy, A. F., Hickmon, S. G., Skinner, R. A., Thomas, J. R., Nelson, C. L., Smeltzer, M. S. (1995). Role of the Accessory Gene Regulator (Agr) in Pathogenesis of Staphylococcal Osteomyelitis. Infect. Immun. 63, 3373–3380. doi: 10.1128/iai.63.9.3373-3380.1995
Girard, L. P., Ceri, H., Gibb, A. P., Olson, M., Sepandj, F. (2010). MIC Versus MBEC to Determine the Antibiotic Sensitivity of Staphylococcus Aureus in Peritoneal Dialysis Peritonitis. Peritoneal Dialysis Int. 30, 652–656. doi: 10.3747/pdi.2010.00010
Gomes, L. C., Mergulhão, F. J. (2017). SEM Analysis of Surface Impact on Biofilm Antibiotic Treatment. Scanning 2017, 2960194. doi: 10.1155/2017/2960194
Høiby, N., Bjarnsholt, T., Givskov, M., Molin, S., Ciofu, O. (2010). Antibiotic Resistance of Bacterial Biofilms. Int. J. Antimicrob. Agents 35, 322–332. doi: 10.1016/j.ijantimicag.2009.12.011
Hsu, C., Lin, M., Chen, C., Chien, S., Cheng, Y., Su, I., et al. (2011). Vancomycin Promotes the Bacterial Autolysis, Release of Extracellular DNA, and Biofilm Formation in Vancomycin-Non-Susceptible Staphylococcus Aureus. FEMS Immunol. Med. Microbiol. 63, 236–247. doi: 10.1111/j.1574-695x.2011.00846.x
Jørgensen, N. P., Skovdal, S. M., Meyer, R. L., Dagnæs-Hansen, F., Fuursted, K., Petersen, E. (2016). Rifampicin-Containing Combinations are Superior to Combinations of Vancomycin, Linezolid and Daptomycin Against Staphylococcus Aureus Biofilm Infection In Vivo and In Vitro. Pathog. Dis. 74, ftw019. doi: 10.1093/femspd/ftw019
Jacqueline, C., Caillon, J. (2014). Impact of Bacterial Biofilm on the Treatment of Prosthetic Joint Infections. J. Antimicrob. Chemother. 69, i37–i40. doi: 10.1093/jac/dku254
Kawai, T., Pan, C.-C., Okuzu, Y., Shimizu, T., Stahl, A. M., Matsuda, S., et al. (2021). Combining a Vascular Bundle and 3D Printed Scaffold With BMP-2 Improves Bone Repair and Angiogenesis. Tissue Eng. Part A 27, 1517–1525. doi: 10.1089/ten.tea.2021.0049
Kırmusaoğlu, S. (2019). The Methods for Detection of Biofilm and Screening Antibiofilm Activity of Agent. Antimicrobials, Antibiotic Resistance, Antibiofilm Strategies and Activity Methods. Chapter 7. doi: 10.5772/intechopen.84411
Kourbatova, E. V., Halvosa, J. S., King, M. D., Ray, S. M., White, N., Blumberg, H. M. (2005). Emergence of Community-Associated Methicillin-Resistant Staphylococcus Aureus USA 300 Clone as a Cause of Health Care-Associated Infections Among Patients With Prosthetic Joint Infections. Am. J. Infect. Control 33, 385–391. doi: 10.1016/j.ajic.2005.06.006
Li, J., Xie, S., Ahmed, S., Wang, F., Gu, Y., Zhang, C., et al. (2017). Antimicrobial Activity and Resistance: Influencing Factors. Front. Pharmacol. 8. doi: 10.3389/fphar.2017.00364
Macià, M. D., Rojo-Molinero, E., Oliver, A. (2014). Antimicrobial Susceptibility Testing in Biofilm-Growing Bacteria. Clin. Microbiol. Infect. 20, 981–990. doi: 10.1111/1469-0691.12651
Maruo, A., Oda, T., Miya, H., Muratsu, H., Fukui, T., Oe, K., et al. (2021). Intra-Medullary Antibiotics Perfusion (iMAP) for the Control of Fracture-Related Infection Early After Osteosynthesis. J. Orthop Surg. (Hong Kong) 29, 1–10. doi: 10.1177/23094990211051492
Masters, E. A., Trombetta, R. P., Bentley, K. L., de, M., Boyce, B. F., Gill, A. L., et al. (2019). Evolving Concepts in Bone Infection: Redefining “Biofilm”, “Acute vs. Chronic Osteomyelitis”, “The Immune Proteome” and “Local Antibiotic Therapy. Bone Res. 7, 20. doi: 10.1038/s41413-019-0061-z
Metsemakers, W. J., Kuehl, R., Moriarty, T. F., Richards, R. G., Verhofstad, M. H. J., Borens, O., et al. (2018). Infection After Fracture Fixation: Current Surgical and Microbiological Concepts. Injury 49, 511–522. doi: 10.1016/j.injury.2016.09.019
Mottola, C., Matias, C. S., Mendes, J. J., Melo-Cristino, J., Tavares, L., Cavaco-Silva, P., et al. (2016). Susceptibility Patterns of Staphylococcus Aureus Biofilms in Diabetic Foot Infections. BMC Microbiol. 16, 119. doi: 10.1186/s12866-016-0737-0
Nishitani, K., Sutipornpalangkul, W., Bentley, K. L., de, M., Varrone, J. J., Bello-Irizarry, S. N., et al. (2015). Quantifying the Natural History of Biofilm Formation In Vivo During the Establishment of Chronic Implant-Associated Staphylococcus Aureus Osteomyelitis in Mice to Identify Critical Pathogen and Host Factors. J. Orthop Res. 33, 1311–1319. doi: 10.1002/jor.22907
Oliva, A., Nguyen, B. L., Mascellino, M. T., D’Abramo, A., Iannetta, M., Ciccaglioni, A., et al. (2013). Sonication of Explanted Cardiac Implants Improves Microbial Detection in Cardiac Device Infections. J. Clin. Microbiol. 51, 496–502. doi: 10.1128/jcm.02230-12
O’Toole, G., Kaplan, H. B., Kolter, R. (2000). Biofilm Formation as Microbial Development. Annu. Rev. Microbiol. 54, 49–79. doi: 10.1146/annurev.micro.54.1.49
Poser, L., Matthys, R., Schawalder, P., Pearce, S., Alini, M., Zeiter, S. (2014). A Standardized Critical Size Defect Model in Normal and Osteoporotic Rats to Evaluate Bone Tissue Engineered Constructs. BioMed. Res. Int. 2014, 348635. doi: 10.1155/2014/348635
Post, V., Wahl, P., Richards, R. G., Moriarty, T. F. (2017). Vancomycin Displays Time-Dependent Eradication of Mature Staphylococcus Aureus Biofilms. J. Orthop Res. 35, 381–388. doi: 10.1002/jor.23291
Rosa, L., Lepanto, M. S., Cutone, A., Berlutti, F., Angelis, M. D., Vullo, V., et al. (2019). BioTimer Assay as Complementary Method to Vortex-Sonication-Vortex Technique for the Microbiological Diagnosis of Implant Associated Infections. Sci. Rep. 9, 7534. doi: 10.1038/s41598-019-44045-1
Rose, W. E., Poppens, P. T. (2009). Impact of Biofilm on the In Vitro Activity of Vancomycin Alone and in Combination With Tigecycline and Rifampicin Against Staphylococcus Aureus. J. Antimicrob. Chemother. 63, 485–488. doi: 10.1093/jac/dkn513
Rutherford, S. T., Bassler, B. L. (2012). Bacterial Quorum Sensing: Its Role in Virulence and Possibilities for Its Control. Cold Spring Harb. Perspect. Med. 2, a012427. doi: 10.1101/cshperspect.a012427
Saeed, K., McLaren, A. C., Schwarz, E. M., Antoci, V., Arnold, W. V., Chen, A. F., et al. (2019). 2018 International Consensus Meeting on Musculoskeletal Infection: Summary From the Biofilm Workgroup and Consensus on Biofilm Related Musculoskeletal Infections. J. Orthop Res. 37, 1007–1017. doi: 10.1002/jor.24229
Stavrakis, A. I., Niska, J. A., Shahbazian, J. H., Loftin, A. H., Ramos, R. I., Billi, F., et al. (2014). Combination Prophylactic Therapy With Rifampin Increases Efficacy Against an Experimental Staphylococcus Epidermidis Subcutaneous Implant-Related Infection. Antimicrob. Agents Chemother. 58, 2377–2386. doi: 10.1128/aac.01943-13
Stoodley, P., Ehrlich, G. D., Sedghizadeh, P. P., Hall-Stoodley, L., Baratz, M. E., Altman, D. T., et al. (2011). Orthopaedic Biofilm Infections. Curr. Orthop Pract. 22, 558–563. doi: 10.1097/bco.0b013e318230efcf
Teterycz, D., Ferry, T., Lew, D., Stern, R., Assal, M., Hoffmeyer, P., et al. (2010). Outcome of Orthopedic Implant Infections Due to Different Staphylococci. Int. J. Infect. Dis. 14, e913–e918. doi: 10.1016/j.ijid.2010.05.014
Thompson, J. M., Saini, V., Ashbaugh, A. G., Miller, R. J., Ordonez, A. A., Ortines, R. V., et al. (2017). Oral-Only Linezolid-Rifampin Is Highly Effective Compared With Other Antibiotics for Periprosthetic Joint Infection. J. Bone Joint Surg. Am. 99, 656–665. doi: 10.2106/jbjs.16.01002
Tomizawa, T., Nishitani, K., Ito, H., Okae, Y., Morita, Y., Doi, K., et al. (2021). The Limitations of Mono- and Combination Antibiotic Therapies on Immature Biofilms in a Murine Model of Implant-Associated Osteomyelitis. J. Orthop Res. 39, 449–457. doi: 10.1002/jor.24956
Verderosa, A. D., Totsika, M., Fairfull-Smith, K. E. (2019). Bacterial Biofilm Eradication Agents: A Current Review. Front. Chem. 7. doi: 10.3389/fchem.2019.00824
Vugt, T. A. G., Arts, J. J., Geurts, J. A. P. (2019). Antibiotic-Loaded Polymethylmethacrylate Beads and Spacers in Treatment of Orthopedic Infections and the Role of Biofilm Formation. Front. Microbiol. 10. doi: 10.3389/fmicb.2019.01626
Zhang, K., Li, X., Yu, C., Wang, Y. (2020). Promising Therapeutic Strategies Against Microbial Biofilm Challenges. Front. Cell Infect. Microbiol. 10. doi: 10.3389/fcimb.2020.00359
Keywords: antibiotics, biofilm, infection, implant, Staphylococcus aureus - bacteria
Citation: Okae Y, Nishitani K, Sakamoto A, Kawai T, Tomizawa T, Saito M, Kuroda Y and Matsuda S (2022) Estimation of Minimum Biofilm Eradication Concentration (MBEC) on In Vivo Biofilm on Orthopedic Implants in a Rodent Femoral Infection Model. Front. Cell. Infect. Microbiol. 12:896978. doi: 10.3389/fcimb.2022.896978
Received: 15 March 2022; Accepted: 01 June 2022;
Published: 01 July 2022.
Edited by:
Barbara M. Bröker, University of Greifswald, GermanyReviewed by:
Emanuele Chisari, Rothman Institute, United StatesJohn K. Crane, University at Buffalo, United States
Copyright © 2022 Okae, Nishitani, Sakamoto, Kawai, Tomizawa, Saito, Kuroda and Matsuda. This is an open-access article distributed under the terms of the Creative Commons Attribution License (CC BY). The use, distribution or reproduction in other forums is permitted, provided the original author(s) and the copyright owner(s) are credited and that the original publication in this journal is cited, in accordance with accepted academic practice. No use, distribution or reproduction is permitted which does not comply with these terms.
*Correspondence: Kohei Nishitani, nkohei@kuhp.kyoto-u.ac.jp