The repositioned drugs disulfiram/diethyldithiocarbamate combined to benznidazole: Searching for Chagas disease selective therapy, preventing toxicity and drug resistance
- 1Innovations in Therapies, Education and Bioproducts Laboratory, Oswaldo Cruz Institute, Oswaldo Cruz Foundation, Rio de Janeiro, RJ, Brazil
- 2Parasite Biology Laboratory, Gonçalo Moniz Institute, Oswaldo Cruz Foundation, Salvador, BA, Brazil
- 3Laboratory of Clinical Research on Chagas Disease, Evandro Chagas Infectious Disease Institute, Oswaldo Cruz Foundation, Rio de Janeiro, RJ, Brazil
- 4Experimental Pharmacotechnics Laboratory, Department of Galenic Innovation, Institute of Drug Technology - Farmanguinhos, Oswaldo Cruz Foundation, Rio de Janeiro, RJ, Brazil
- 5Project Management Technical Assistance, Institute of Drug Technology - Farmanguinhos, Oswaldo Cruz Foundation, Rio de Janeiro, RJ, Brazil
- 6Experimental Chagas Disease Laboratory, Gonçalo Moniz Institute, Oswaldo Cruz Foundation, Salvador, BA, Brazil
Chagas disease (CD) affects at least 6 million people in 21 South American countries besides several thousand in other nations all over the world. It is estimated that at least 14,000 people die every year of CD. Since vaccines are not available, chemotherapy remains of pivotal relevance. About 30% of the treated patients cannot complete the therapy because of severe adverse reactions. Thus, the search for novel drugs is required. Here we tested the benznidazole (BZ) combination with the repositioned drug disulfiram (DSF) and its derivative diethyldithiocarbamate (DETC) upon Trypanosoma cruzi in vitro and in vivo. DETC-BZ combination was synergistic diminishing epimastigote proliferation and enhancing selective indexes up to over 10-fold. DETC was effective upon amastigotes of the BZ- partially resistant Y and the BZ-resistant Colombiana strains. The combination reduced proliferation even using low concentrations (e.g., 2.5 µM). Scanning electron microscopy revealed membrane discontinuities and cell body volume reduction. Transmission electron microscopy revealed remarkable enlargement of endoplasmic reticulum cisternae besides, dilated mitochondria with decreased electron density and disorganized kinetoplast DNA. At advanced stages, the cytoplasm vacuolation apparently impaired compartmentation. The fluorescent probe H2-DCFDA indicates the increased production of reactive oxygen species associated with enhanced lipid peroxidation in parasites incubated with DETC. The biochemical measurement indicates the downmodulation of thiol expression. DETC inhibited superoxide dismutase activity on parasites was more pronounced than in infected mice. In order to approach the DETC effects on intracellular infection, peritoneal macrophages were infected with Colombiana trypomastigotes. DETC addition diminished parasite numbers and the DETC-BZ combination was effective, despite the low concentrations used. In the murine infection, the combination significantly enhanced animal survival, decreasing parasitemia over BZ. Histopathology revealed that low doses of BZ-treated animals presented myocardial amastigote, not observed in combination-treated animals. The picrosirius collagen staining showed reduced myocardial fibrosis. Aminotransferase de aspartate, Aminotransferase de alanine, Creatine kinase, and urea plasma levels demonstrated that the combination was non-toxic. As DSF and DETC can reduce the toxicity of other drugs and resistance phenotypes, such a combination may be safe and effective.
Introduction
At least 6-7 million people have Chagas disease (CD), mostly in Latin America (WHO - World Health Organization, 2021), where over 10% of the population is at risk of infection (Pérez-Molina and Molina, 2018). There are at least 4.6 million infected people in Brazil, which can reach 1.5% of the Brazilian population. In addition, about 70 million are at risk of infection by Trypanosoma cruzi (Dias et al., 2016). The parasitosis, also known as American trypanosomiasis, is already considered a public health problem on a global scale (Franco-Paredes et al., 2009; Coura and Viñas, 2010; Parker and Sethi, 2011).
CD causes economic losses in excess of U$1.2 billion/year to endemic countries in South America, in addition to more than $7 billion/year at global levels (Lee et al., 2013), including treatment and loss of productivity, not including the losses caused by infections by tourists and emigrants to North America, Europe, and Asia (Coura and Viñas, 2010) coming from South and Central America. Therefore, it can be inferred that effective drugs, besides promoting the quality of life of patients and their families, can provide considerable socioeconomic benefit.
Since CD discovery by the Brazilian researcher Carlos Chagas over a century ago, the disease is intensely studied, but only two drugs, benznidazole (BZ) and nifurtimox (NFX), are employed in CD treatment. However, BZ side effects lead to therapy discontinuation from approximately 30% of the cases up to eventually reaching 50% of the patients (Guggenbühl Noller et al., 2020). The option is NFX but a recent study (Crespillo-Andújar et al., 2018) reported that the use of NFX in patients who had been discontinued from BZ treatment still led to more than 12% of elevated toxicity, forcing physicians to permanently discontinue treatment. Therefore, there is pressing demand for the development of new drugs or therapeutic regimens for CD.
Different chemotherapy targets have been approached during the last decades (Duschak, 2011; Duschak, 2016; Beltran-Hortelano et al., 2017; Duschak, 2019; Beltran-Hortelano et al., 2022), and much was learned about the biochemistry and cell biology of T. cruzi, but new agents are still not in clinical use. Drug combinations may be promising for allowing dosing reduction (Bustamante et al., 2014), hampering resistance selection (Hill and Cowen, 2015), and enhancing selectivity (Zimmermann et al., 2007; Lehár et al., 2009a; Lehár et al., 2009b). In addition, drug combinations can promote effectivity of repositioning (Sun et al., 2016).
The use of repositioned drugs (approved by the FDA), with well-established data on bioavailability, safety, etc., allows accelerating drug development, significantly increasing the percentage of success, but reducing their costs (Zheng et al., 2018). Such innovations can be of great value in the therapy of neglected diseases, highly prevalent in South America, caused by parasitic protozoa (Müller and Hemphill, 2016), including CD (Palos et al., 2017).
The repositioning of a low-cost drug such as Disulfiram (DSF, Antabuse®) can be considered a “salvation” for global health care (Cvek, 2012). DSF, a drug used for the therapy of alcoholism, is widely used and well tolerated in humans (Jørgensen et al., 2011; Sinclair et al., 2016) and is even considered less toxic than aspirin (Gessner and Gessner, 1992) and trials employing 200-250 mg/d daily or 800 mg/twice a week are regularly performed, with no reports of adverse effects (Sinclair et al., 2016). The DSF first derivative sodium diethyldithiocarbamate (DETC), also known as imuthiol, has been successfully used as an immunostimulant in HIV patients, reducing opportunistic infections (Hersh et al., 1991). DSF is used for different purposes (e.g. Kona et al., 2011), such as cancer therapy (Cvek, 2012; Meraz-Torres et al., 2020; Kannappan et al., 2021; Lu et al., 2021; Lu et al., 2022) and chemoprevention (Askgaard et al., 2014; Yang et al., 2015; Harrington et al., 2020). The DSF and/or DETC combination can enhance antitumoral activities of drugs such as cisplatin (O’Brien et al., 2012; Nechushtan et al., 2015), but diminish adverse reactions (Wysor et al., 1982; Elliott et al., 1983; Bodenner et al., 1986; Roemeling et al., 1986). In addition, DSF can overcome resistance, via different mechanisms (Schmidtova et al., 2019; Yang et al., 2019). The data presented here indicate that the BZ-DSF combination may comprise a promising alternative for CD therapy.
Materials and methods
Drugs
BZ (Nortec Química, Rio de Janeiro, Brazil) and DSF (Corden Pharma Bergamo S.p.A) were provided by Farmanguinhos (Fiocruz, Rio de Janeiro) and DETC was purchased from Sigma-Aldrich. Drugs were dissolved in dimethyl sulfoxide (DMSO) and stored at -20°C until use.
Parasites and mammalian cells
T. cruzi Y and the Colombiana strains epimastigote forms were maintained in LIT (Liver Infusion Trypticase) medium, supplemented with 10% fetal bovine serum (FBS), 100 µg/mL penicillin, and streptomycin at 25°C (dos Anjos et al., 2016). Cultures were harvested at the exponential growth phase. Then 5x105 parasites were incubated in the presence of the isolated compounds. Trypomastigote forms were obtained by cardiac puncture, at the peak of parasitemia of infected Swiss Webster mice (Sueth-Santiago et al., 2016) and maintained through co-culture with epithelial cells, VERO, previously in Dulbecco’s modified Eagle’s medium (DMEM) supplemented with 10% FBS, at 37°C, 5% CO2, as well as the amastigote form obtained after 8 days of cell infection (Monteiro et al., 2001). Axenic amastigotes were obtained from cell cultures of trypomastigotes in BHT medium incubated at 28°C, being collected after three passages of 56-64 h, in an initial concentration between 5x106 cells/mL (Engel et al., 1987). Macrophages from BALB/c mice were collected by peritoneal lavage in Hank’s balanced solution, seeded in 24-well plates or bottles (Falcon, New Jersey, USA), and kept at 37°C in atmosphere of 5% CO2 in DMEM medium supplemented with 10% FBS.
Parasite-host interaction
Assays were performed using a parasite:cell ratio of 10:1, and infection quantification was performed by direct counting cultured cells Giemsa (Laborklin)-stained coverslips under light microscopy, approximately 1000 cells per coverslip. Association indices (AI) were obtained by multiplying the percentage of infected macrophages by the average number of parasites per host cell, as previously described (Martiny et al., 1996).
In vitro evaluation of trypanocidal activity and cytotoxicity
T. cruzi epimastigotes (107 cells/mL) incubated with DETC and BZ, to determine IC50 values each drug, at 24 h at 28°C, determined by the Alamar Blue assay at 570 nm and 600 nm. For the combinations, both for trypanocidal activity and for the selectivity index (107 Swiss Webster mouse peritoneal macrophages/mL), six fixed doses were prepared based on the IC50 value of the isolated drugs, in the proportions 5:0, 4:1, 3:2, 2:3, 1:4 and 0:5 (Fivelman et al., 2004). Concentration-response curves were plotted and the IC50 and CC50 values of the compounds (inhibition and cytotoxicity, respectively), alone or in combination, were calculated using GraphPad Prism, 7.
ROS detection
ROS were detected using the H2-DCFDA probe using a confocal microscope Fluoview 1000, Olympus.
Lipid peroxidation
Lipid peroxidation was determined by the production of thiobarbituric acid (TBA) reactive substances (TBARS), by parasites incubated or not in the presence of the compounds for 24 h. Subsequently, the cells were centrifuged three times in phosphate-buffered saline (PBS). After washing, the parasites were resuspended in 200 μL PBS and 200 μL TBA at a final concentration of 1%. After homogenization, the material was incubated at 99°C for a period of 3 h and measured in a spectrophotometer at 532 nm (Menezes et al., 2006).
Thiol group measurements
The determination of the concentration of low molecular weight thiols was carried out using 5,5′-dithiobis (2-nitrobenzoic acid) (DTNB) and methanol assay after protein removal with 10% trichloro acetic acid. Subsequently, the supernatant was read in a spectrophotometer at 412 nm (Hitachi U-1100), as previously described (Sedlak and Lindsay, 1968).
Dosage of superoxide dismutase
Parasite samples untreated and treated with DETC alone and in combination with BZ, for 1 and 24 hours, were evaluated using a colorimetric method for superoxide dismutase (SOD) measurement (Sigma-Aldrich Kit-WST- SOD Assay), which is based on the generation of the radial superoxide from the xanthine-xanthine oxidase (XOD) system, where the superoxide reacts with the sample and converts the tetrazolium salt to formazan. After reactions, the measurements spectrophotometrically performed in a VersaMax at 440 nm (Moukdar et al., 2009).
Electron microscopy
Parasites were washed with PBS and fixed in Karnovsky for 24 h, at 4°C. Then, samples were post-fixed in 1% osmium tetroxide, 5 mM calcium chloride, and 0.8% potassium ferricyanide in 0.1M sodium cacodylate buffer, protected from light for 40 min at room temperature. For scanning, electron microscopy (SEM) samples were dehydrated in ethanol series, critical point-dried, mounted on stubs, gold-metalized, and observed in a JEOL 5310 scanning electron microscope. For transmission, electron microscopy (TEM) samples were dehydrated in acetone and embedded in Polybed epoxy resin (Polysciences, Inc). After 72 h at 60°C, the samples were sectioned on an ultramicrotome (Reichert, Leica), using a diamond knife (Diatome, Hatfield, PA), and the sections were collected on 300 mesh copper grids and counterstained with 3% lead citrate and 5% uranyl acetate in water. Samples were observed in a transmission electron microscope Zeiss EM 109 at 80kV, as previously described (Vannier-Santos and Lins, 2001).
In vivo infection
Swiss Webster mice were infected with Y or Colombian strain, intraperitoneally (i.p.), with 104 bloodstream trypomastigotes. The infected animals were divided into the following groups (15 animals per group): positive control (BZ); negative control (PBS + 1% Kolliphor); DETC + BZ combination; DSF + BZ. The therapy was initiated 5 days from the beginning of the parasitemia and carried out for 30/60 days, administered through the intragastric route (Salomão et al., 2010). Parasitemia was verified by direct microscopic, slide analysis of parasites in 5 μL of blood, and mortality rates were checked daily/weekly up to 30 days post-treatment.
Systemic toxicity
Thirty days after the end of the treatment, uninfected mice blood was collected through the brachial plexus and stored at -80°C for biochemical analysis of the enzymes: urea, for renal monitoring, total creatine kinase (CK), for evaluation of cardiac or skeletal musculature lesions, alanine aminotransferase (ALT) and aspartate aminotransferase (AST), assessment of liver damage, all being determined in whole blood by the Reflotron® reactive test strip system (Roche Diagnostics, F Hoffmann-La Roche Ltd, Basel, Switzerland) using reflectance photometry (Salomão et al., 2010).
Histopathology
Tissue samples were fixed in formaldehyde solution pH 7.2, embedded in paraffin, and 5 µm-thick sections were stained with hematoxylin and eosin (H&E) and picrosirius red to stain collagen fibers (Andrade et al., 1994).
Statistical analysis
The data obtained are representative of at least three independent experiments carried out in triplicate. Statistically significant differences were analyzed using the ANOVA test and Tukey or Dunn post-tests with p < 0.05, using GraphPad Prism, 7.
Results
DETC inhibited dose-dependently the axenic proliferation of epimastigote forms (Figure 1). The IC50 value obtained was 1.48 µM. The BZ IC50 observed was 2.28 μM (Table 1). The selectivity indexes revealed that the combination selectivity was increased over an order of magnitude (about 13-fold), as compared to the drug of choice, BZ. Based on the IC50 values obtained DETC and BZ were combined at different proportions. Isobolograms were plotted to analyze the possible synergism between BZ and DETC on the trypanocidal activity. There was synergistic activity, particularly at 3:2 and 2:3 concentration ratios (Figure 2). Afterward, we observed that 5 µM of either BZ or DETC significantly inhibited parasite growth, whereas the combination of 2.5 µM of each compound was even more effective (Figure 3). In order to approach the DETC effects upon general parasite structure, we employed scanning electron microscopy. DETC-treated epimastigotes were often bizarrely shaped, eventually presenting surface discontinuities and reduced cell body volume (Figure 4). We used transmission electron microscopy to determine the DTEC effects on epimastigote subcellular architecture. DETC-treated parasites displayed remarkably enlarged endoplasmic reticulum (ER) cisternae as well as reduced mitochondrial electrondensity (Figure 5). Morphometric analysis indicate the ER lumen was enhanced over 1000-fold (not shown). Some parasites displayed disorganized kinetoplast DNA and loss of cell ultrastructural compartmentation.
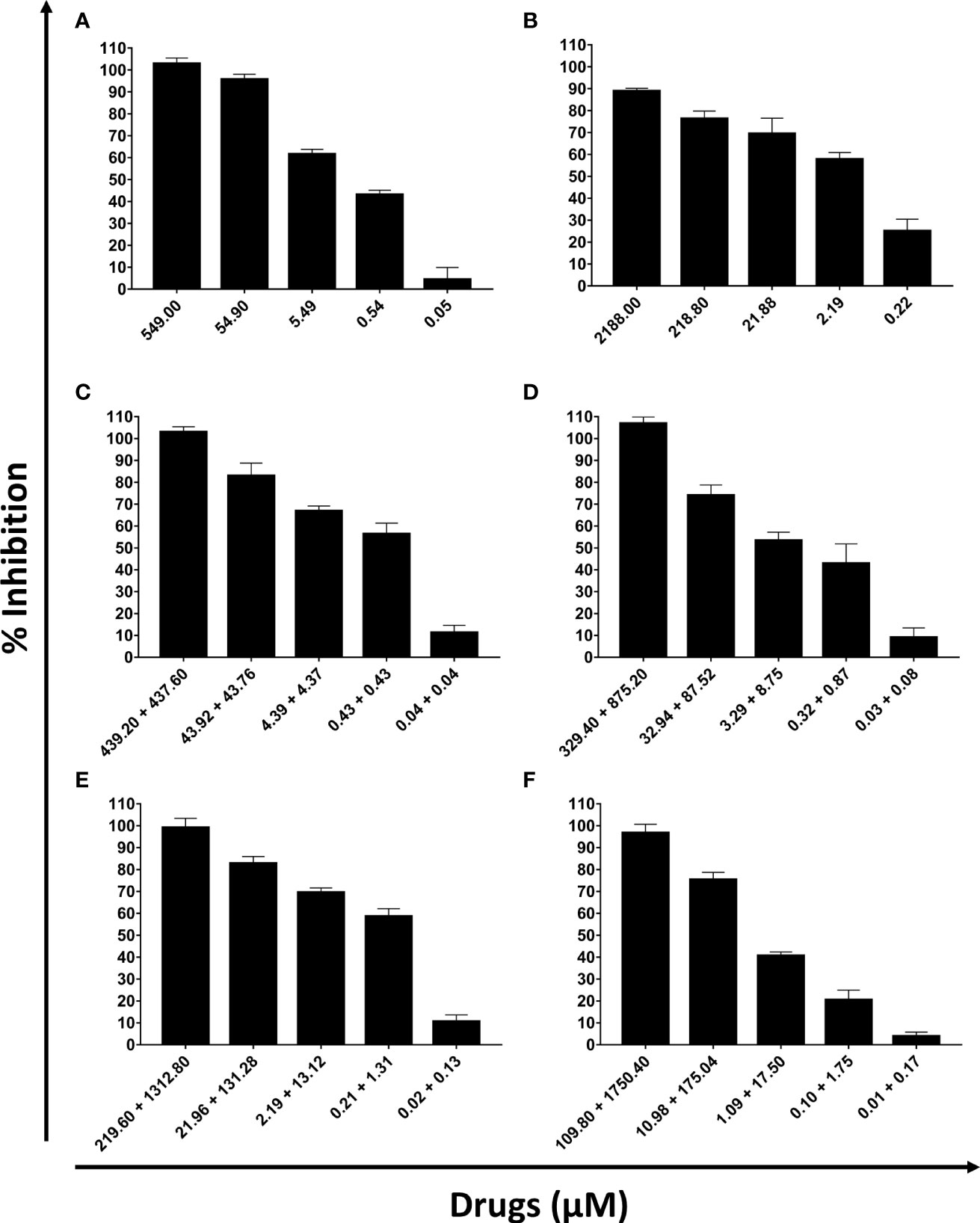
Figure 1 Evaluation of the activity of sodium diethyldithiocarbamate (DETC) and benznidazole (BZ) in the in vitro proliferation of Trypanosoma cruzi epimastigote forms (107 parasites/mL, Y strain). The proportions of the combinations were: 5 x IC50 DETC (A); 5 x IC50 BZ (B), 4 x IC50 DETC + IC50 BZ (C), 3 x IC50 DETC + 2 x IC50 BZ (D), 2 x IC50 DETC + 3 x IC50 BZ (E) and IC50 DETC + 4 x IC50 BZ (F). After 24h incubation, the inhibitory effects were determined using Alamar Blue.
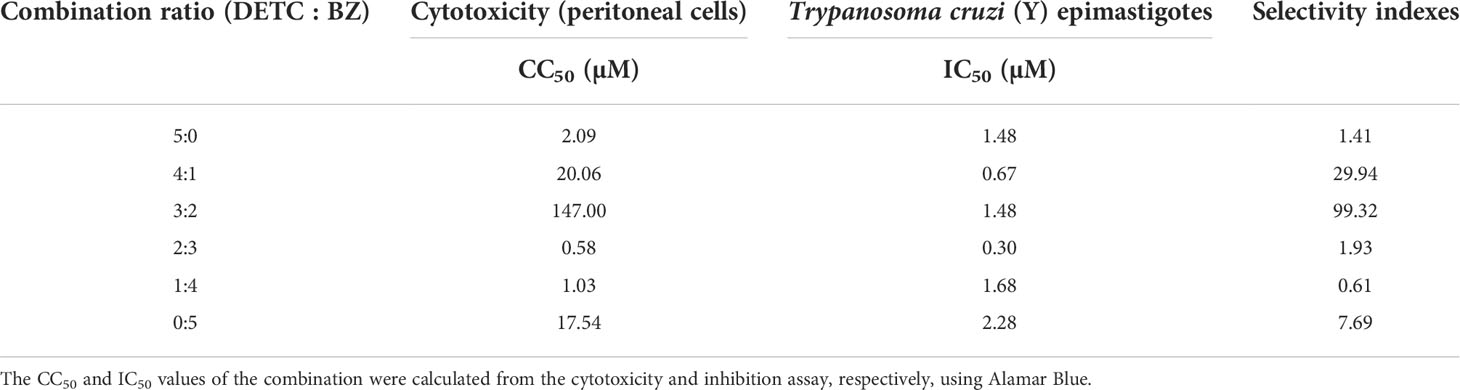
Table 1 Trypanocidal activity, cytotoxicity, and selectivity indexes of the DETC + BZ combination after 24 h of treatment.
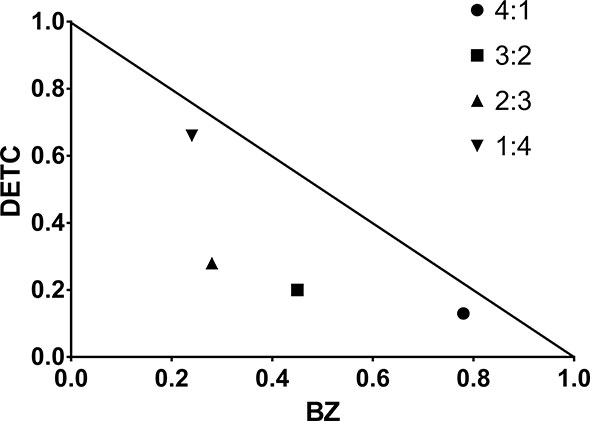
Figure 2 Representative isobologram demonstrating the synergistic interaction between DETC and BZ upon T. cruzi (Y strain) epimastigote forms, cultured for 24 h, based on IC50 values.
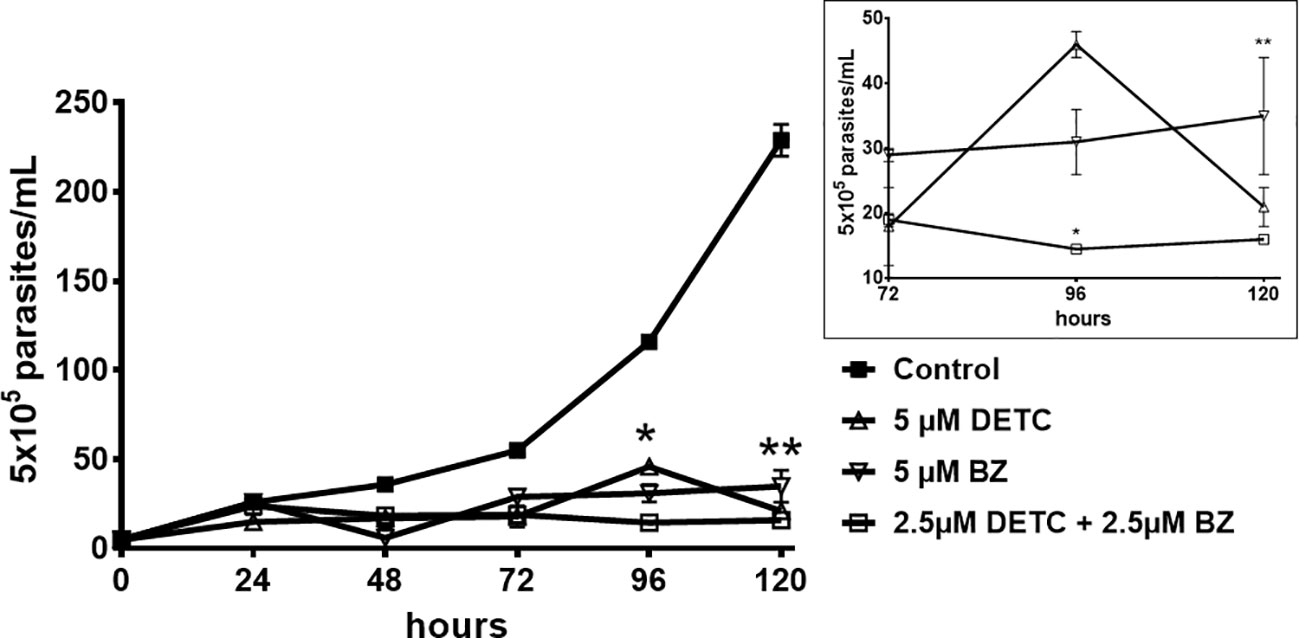
Figure 3 Evaluation of the in vitro proliferation of T. cruzi epimastigote forms (Y strain), challenged with DETC and BZ combined or alone. 105 parasites were incubated for 5 days with 5 µM DETC, 5 µM BZ and the combination of 2.5 µM each compound. The effects of the compounds were evaluated by daily quantitation of parasites by light microscopy. Inset displays 72-120 h data in a different scale. The combined drugs effectivity was significant, (*p < 0.05; **p < 0.01), despite the reduced concentrations, as compared to the parasites treated with BZ by the 2-way ANOVA. Data represent the mean ± SD (n = 3).
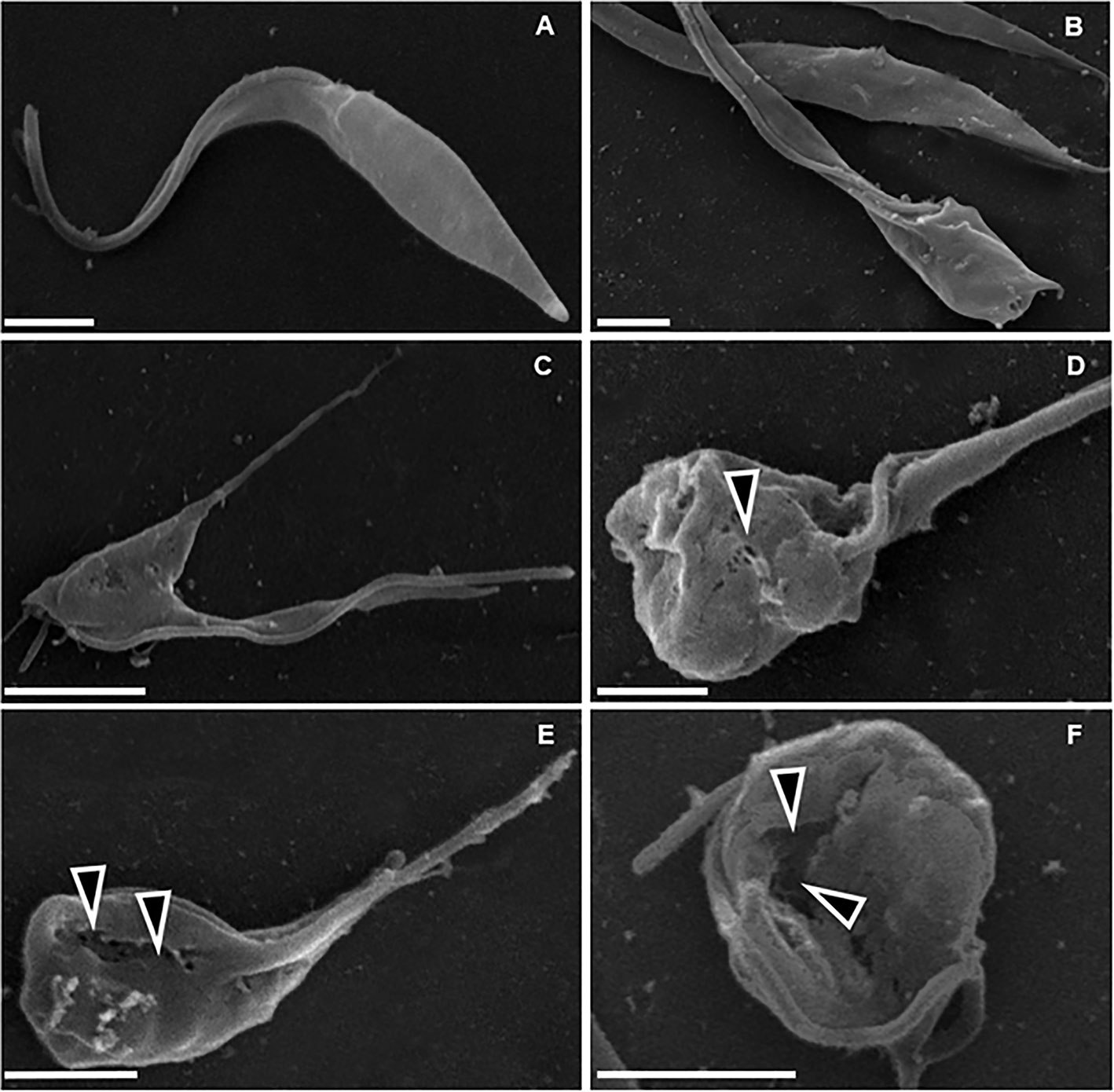
Figure 4 Scanning electron microscopy of T. cruzi epimastigote forms (Y strain). (A) Untreated control, showing normal parasite morphology. B–F) Parasites treated with 200 µM DETC for 24 h, showing cellular disorganization (B, C) and plasma membrane discontinuities (D–F, arrowheads), associated with cell body rounding and volume reduction. Bars correspond to 1 µm.
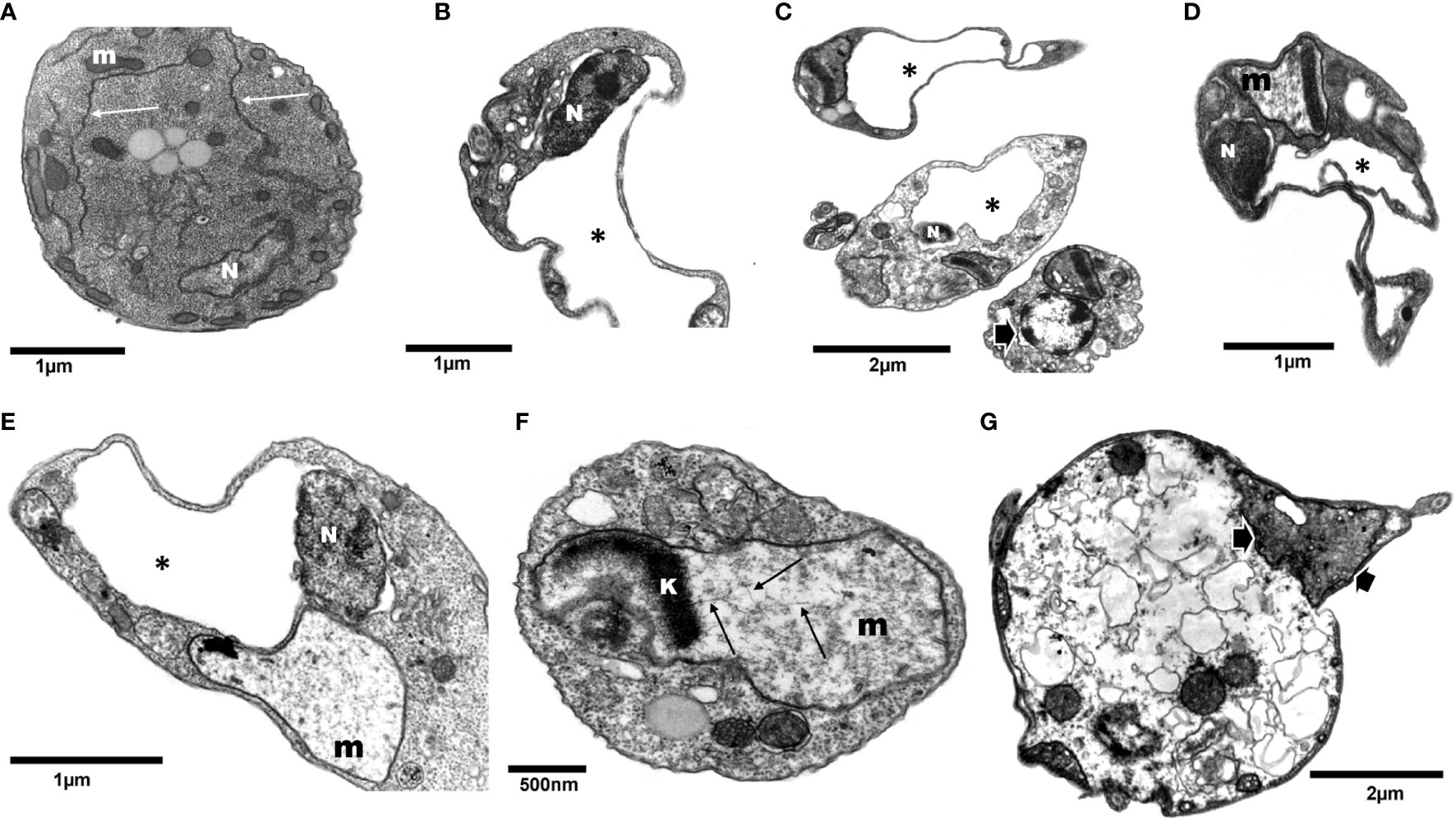
Figure 5 Transmission electron microscopy of T. cruzi epimastigotes (Y strain) incubated with 200 µM DETC for 24 h. Contrary to control cells (A, arrows – endoplasmic reticulum cisternae; m- mitochondria; N- nuclei), DETC treated (B–G) displayed remarkably enlarged endoplasmic reticulum cisternae (B–E*), often replacing an even distorting nuclei (B, C). Some parasites displayed electroluscent nuclear matrix with aggregated peripheral dense chromatin (C, arrow). Mitochondria were enlarged and generally presented reduced electrondensity (D–F) and eventually presenting kinetoplast (k) DNA (kDNA) disorganized fibers (F- arrows). Some cells displaying condensed mitochondria presenting swollen cristae (C, G-arrows) were also observed. In the final stage of treatment, parasites showed loss of normal compartmentation (G).
In order to approach reactive oxygen species (ROS) production in DETC-treated parasites, we used fluorescent probes. Incubation with DETC remarkably enhanced H2DCFDA staining under fluorescence microscopy (Figure 6) and labeling was found in sub-cellular compartments, rather than whole cell. To evaluate the oxidative stress consequence, we measured lipoperoxidation using the TBARS assay (Figure 7). We observed that both BZ and DETC had little effect (p >0.05) on lipid peroxidation, but it was significantly (p < 0.05) increased by their combination. Since sulfhydryl groups are largely involved in the redox regulation in trypanosomatid parasites, we measured thiol expression using the colorimetric Ellman’s reaction DETC diminished thiol expression in the parasite (Figure 8). The biochemical colorimetric approach indicates that DETC produce a dose-dependent effect and that the isolated compounds reduced SH levels (p < 0.05), but the combination was more effective (p < 0.01). As DETC is well-known for its SOD inhibiting capacity, we measured SOD activity in vitro and in vivo, in murine infection, before and after DETC treatment (Table 2). Interestingly, the combination was more inhibitory, and the effects in vitro were more pronounced.
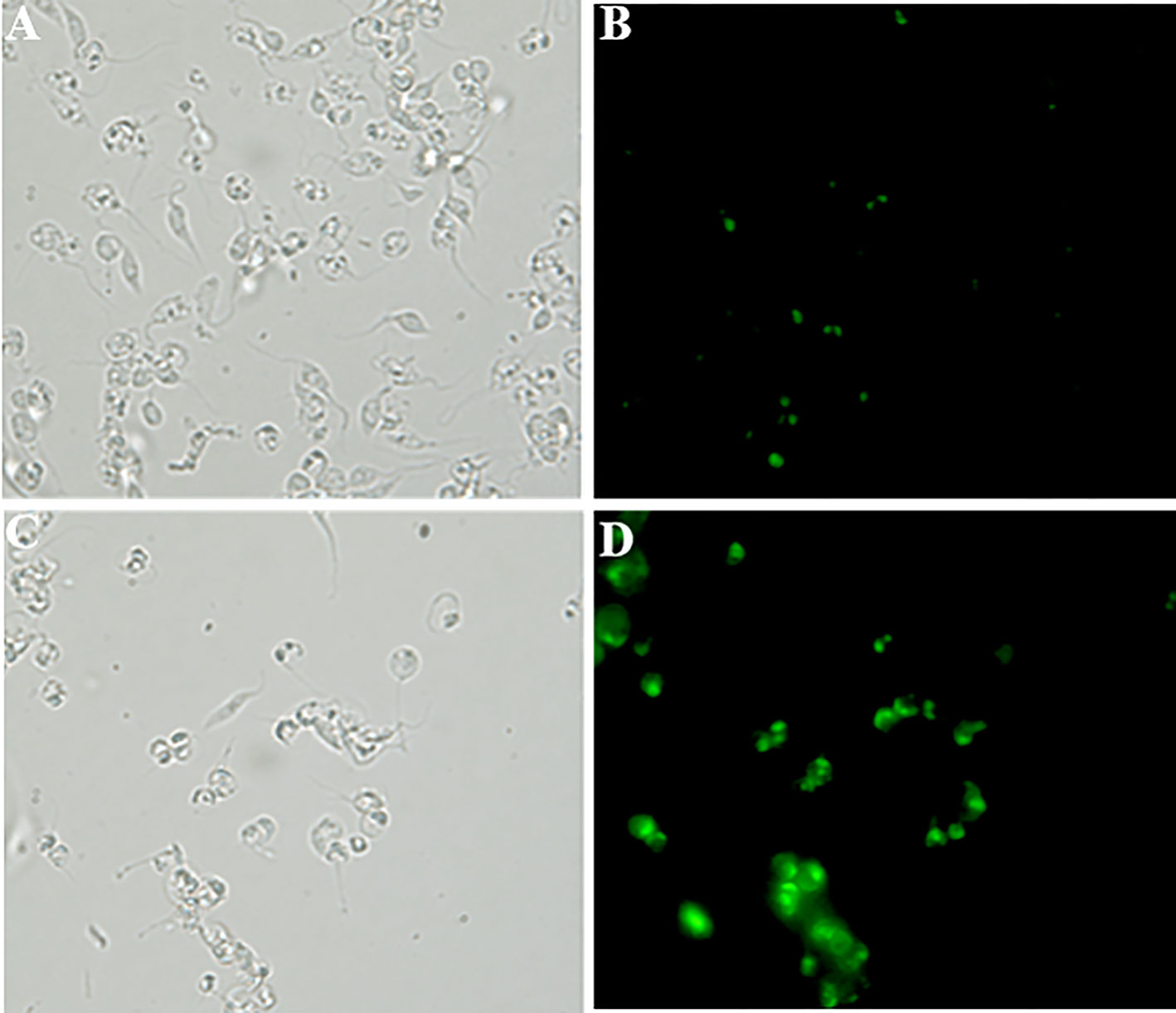
Figure 6 Detection of cellular reactive oxygen species (ROS) in T. cruzi epimastigote forms (Y strain) using the H2DCFDA probe accessed by fluorescence microscopy (B, D). (A, B) - Control and (C, D)- 10µM DETC-treated. (A, C) - phase contrast images. Note intense and compartmented staining in DETC-treated parasites. Magnification - 400X.
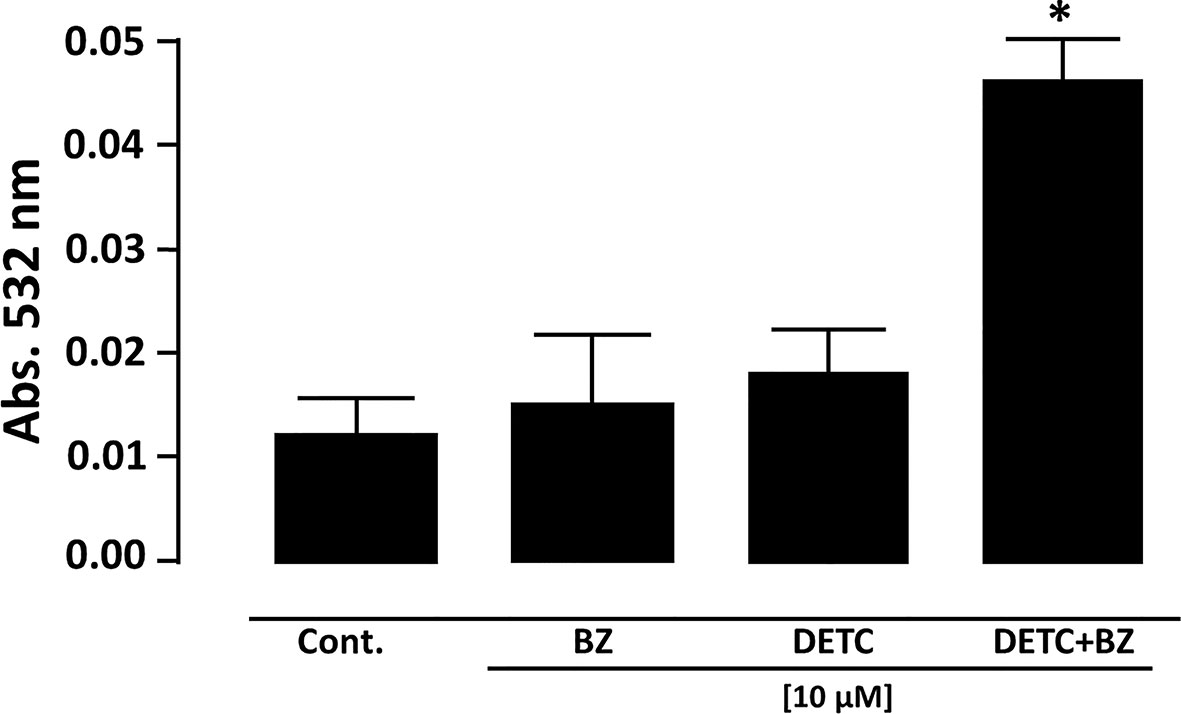
Figure 7 Measurement of lipid peroxidation in T. cruzi epimastigote forms (Y strain) by determination of thiobarbituric acid reactive substances (TBARS). Parasite cells treated with 10 µM DETC and 10 µM BZ combination for 24 h presented a significantly (*p < 0.05, ANOVA and Dunn’s post-test) increased lipoperoxidation. Bars represent mean ± SD (n = 4).
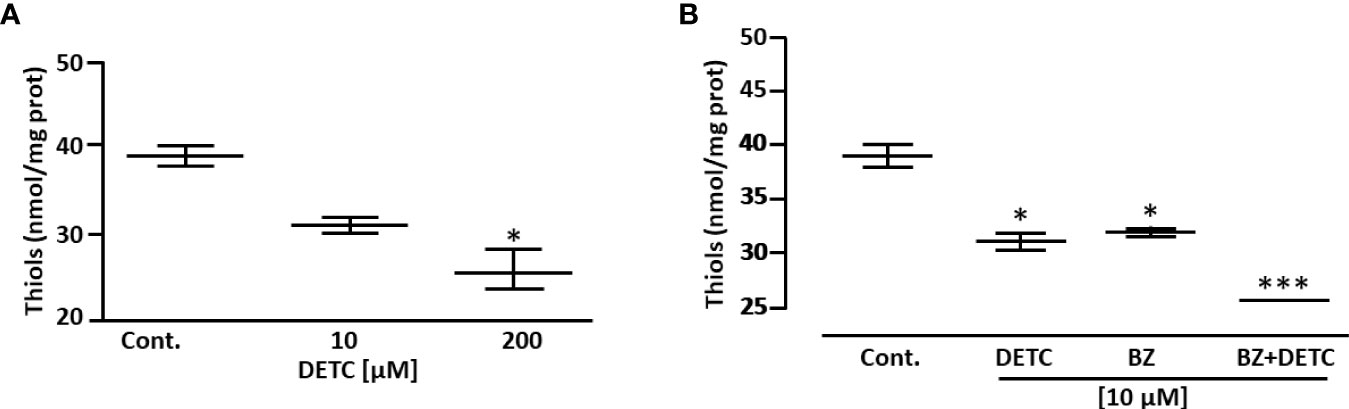
Figure 8 Effect of DETC and BZ on the concentration of low mol. wt. thiols of T. cruzi epimastigote forms (Y strain), determined colorimetrically by the Ellman reaction. DETC reduced thiol levels dose-dependently (A). The DETC + BZ combination significantly potentiated thiol depletion (B). Data represent mean ± SD. *p < 0.05 and ***p < 0.001 (ANOVA and Dunn’s post-test; n = 5).
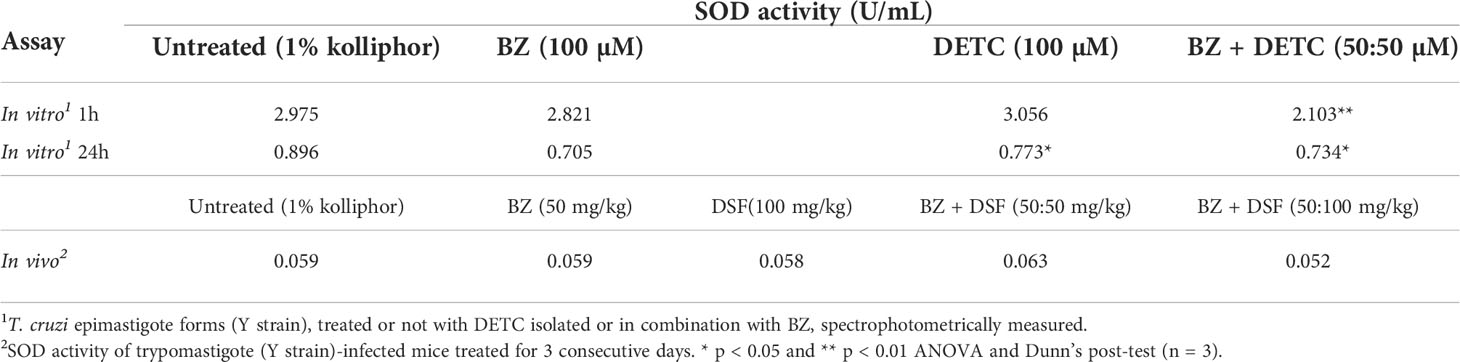
Table 2 Evaluation of T. cruzi superoxide dismutase (SOD) activity in presence of DETC in vitro and DSF in vivo isolated and combined to BZ.
As the Y strain is sensitive to BZ, we decided to test the DETC susceptibility of axenic amastigotes of both Y and Colombiana strains. Although the Colombiana strain, naturally resistant to BZ, was less sensitive to DETC at 0.8-3 µM (p < 0.05), the effects were highly significant (p < 0.01) at 5 µM. Both strains showed significantly decreased parasite survival in a dose-dependent manner (Figure 9).
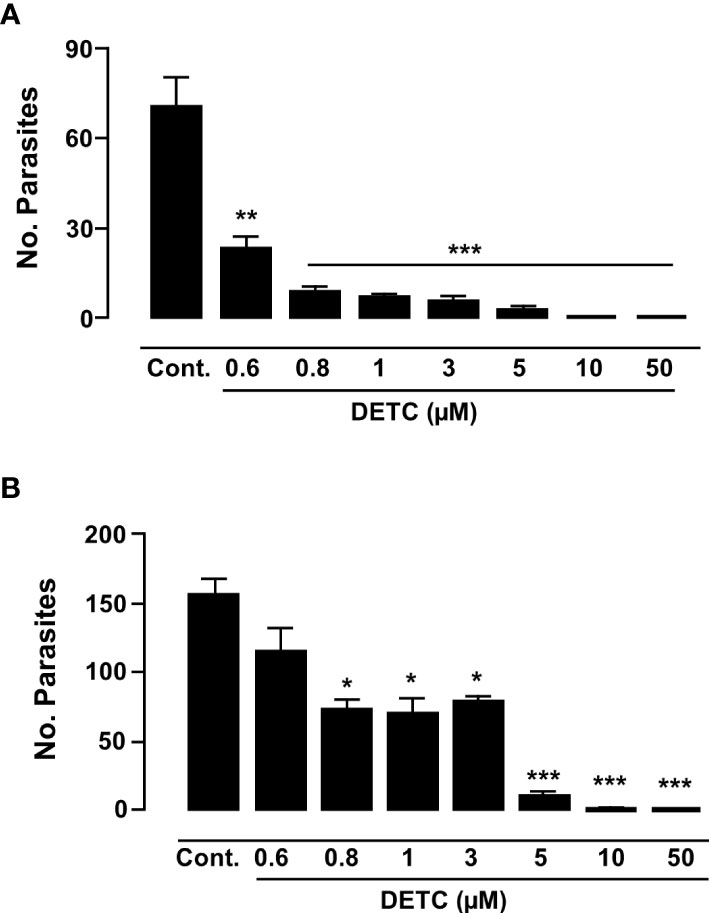
Figure 9 Inhibitory effect of DETC on the proliferation of T. cruzi amastigote forms in Y (A) and Colombiana (B) strains, after incubations of 120 h and 24 h, respectively. * p < 0.05, **p < 0.01 and *** indicates p < 0.001 ANOVA and Dunn’s post-test. Bars represent the mean ± SD (n=3).
In order to test the effects of the combination in intracellular parasites, we infected murine macrophages with blood trypomastigotes in the presence or in the absence of DETC (Figure 10). We noticed that 10 µM DETC remarkably reduced the monolayer parasite load, and a DETC-BZ combination at 5 µM concentration was equally or even more effective.
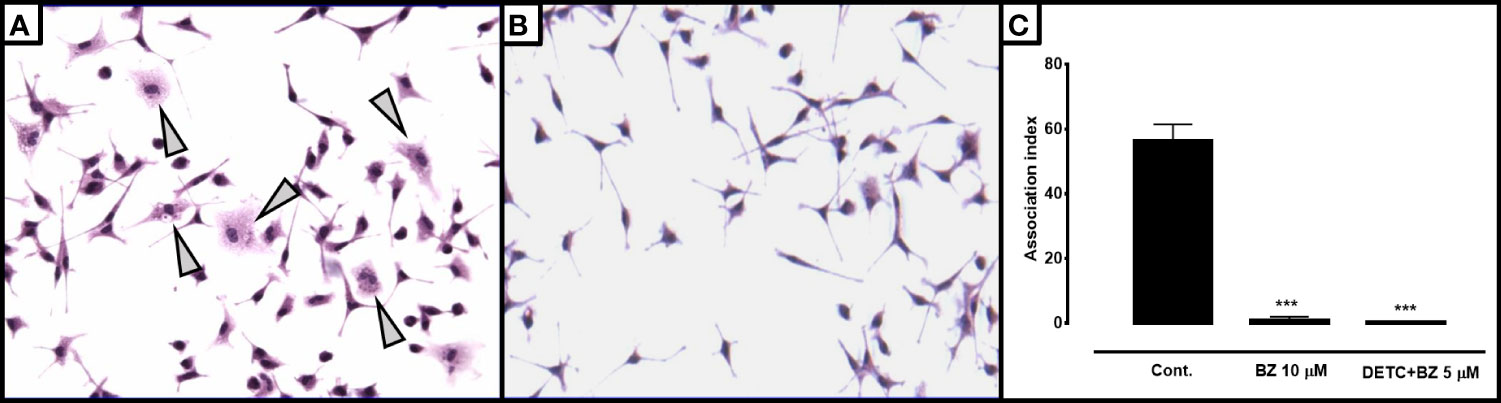
Figure 10 Effect of DETC alone or combined with BZ on the T. cruzi-macrophage interaction. BALB/c mice peritoneal macrophages were infected with Colombiana strain bloodstream trypomastigote forms 1:10 phagocyte/parasite ratio. Control cultures (A) showing several parasitized macrophages as well as larger number of intracellular forms per phagocyte (arrowheads) than cells treated with the combination 5 µM DETC + 5 µM BZ (B). Magnification 200X. The association index in cultures treated with 10 µM BZ or the 5 µM DETC + 5 µM BZ combination after 24 hours of incubation (C) was significantly (***p < 0.001, ANOVA and Dunn’s post-test) reduced. A slightly greater inhibition was observed in cultures incubated with the combination, despite the reduced concentrations. Bars represent mean ± SD.
To approach the activity of the combination in vivo, we tested the murine infection for 30-60 days. The parasitemia of animals infected with both Y and Colombiana strains was reduced by the BZ-DSF combination (Figure 11) as compared to BZ low concentrations (20/50 mg/kg). The cumulative survival of the animas treated with the 10 mg/kg/day (each) DETC-BZ combination was increased by circa 6-fold (p < 0.01) as compared to BZ alone (Figure 12). We used histopathology to evaluate the murine infection. Contrary to animals treated with low BZ concentration (10 mg/kg/d) the ones incubated with the combination (10 mg/kg/d BZ + 10 mg/kg/d DETC) showed no amastigote nests in myocardium (Figure 13). The Sirus red staining was employed to assess tissue fibrosis. Animals treated with the combination displayed less, and focal fibrosis, whereas mice treated with BZ alone showed intense fibrosis staining. To evaluate the systemic toxicity of the treatments we measured the plasma levels of ALT, AST, CK, and urea. Measurements demonstrated (Figure 14) that the combination at 10 mg/kg/day (each) was not as toxic than the isolated drugs at 20 mg/kg/day.
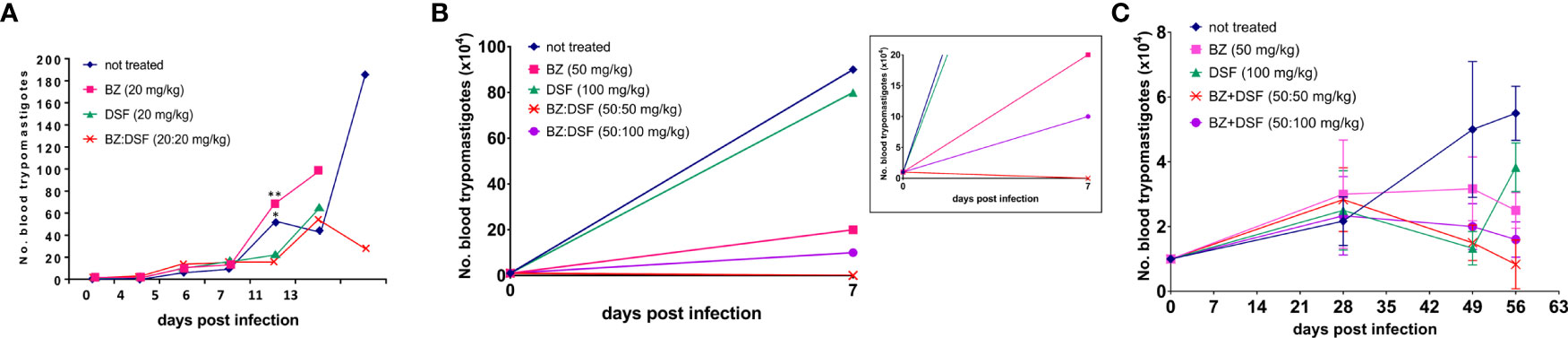
Figure 11 Parasitemia of Swiss Webster mice infected with T. cruzi blood trypomastigotes, Y strain (A, B) and Colombiana (C). The animals were infected with 104 blood trypomastigotes/mL, strain Y, treated for 60 days with vehicle; low doses of BZ (20 mg/kg); DETC (20 mg/kg) or a combination of BZ + DETC (20:20 mg/kg), orally. *p < 0.05 and **p < 0.01 ANOVA test and Dunn’s post-test (A). Parasites treated with vehicle; BZ (50 mg/kg); DSF (50 mg/kg); combination of BZ + DSF (50:50 mg/kg); and BZ + DSF (50:100 mg/kg), administered for 10 consecutive days (B, C, p > 0.05). Insert shows data in a different scale. The parasites were counted daily (A) or weekly (B, C) on Neubauer chambers and the graphs were plotted in GraphPad Prism, 7.
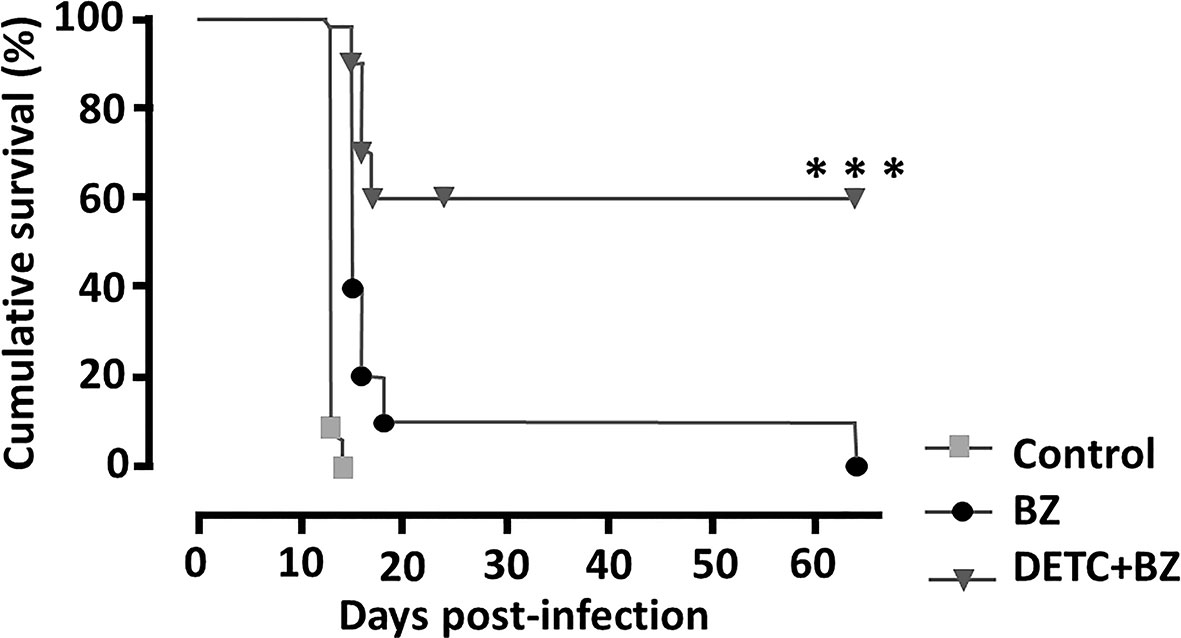
Figure 12 Monitoring the cumulative survival of mice infected intraperitoneally with 104 T. cruzi blood trypomastigote/mL (Y strain) of and treated with 10 mg/kg/day of DETC and BZ alone or in combination, p.o. daily for 60 consecutive days. Note that animals treated with the combination had a survival rate of 60%, whereas the group treated with BZ was 10% and the control group died on the 14th day. ***p < 0.001 ANOVA and Tukey post-test.
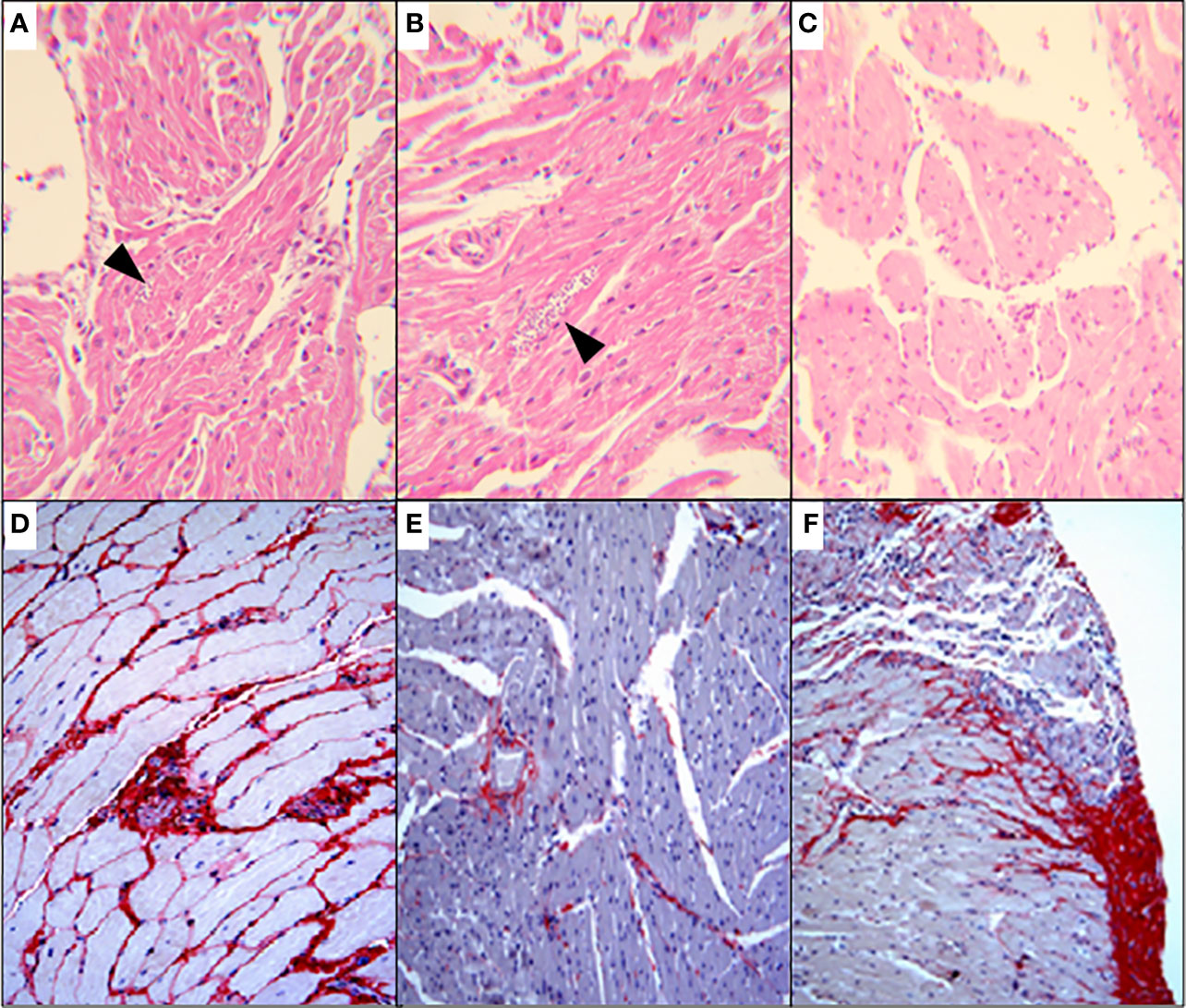
Figure 13 Histopathological analysis (H&E) of murine myocardium T. cruzi (Y strain)-infected mice, treated for 60 consecutive days, p.o. with vehicle (A); 10 mg/kg/d BZ-treated and (B) treated with the of 10 mg/kg/d DETC combined to 10 mg/kg/d BZ (C). Amastigote nests were observed in A and B (arrowheads). Skeletal muscle of mice infected with Colombiana strain, for 100 d and treated for 30 consecutive days orally with 20 mg/kg/d BZ (D), 20 mg/kg/d DSF (E) or combination of 10 mg/kg/d each (F). Picro Sirius red staining was employed to demonstrate fibrosis. The combination using lowered concentrations was associated with reduced fibrosis (F). Magnification: 200X.
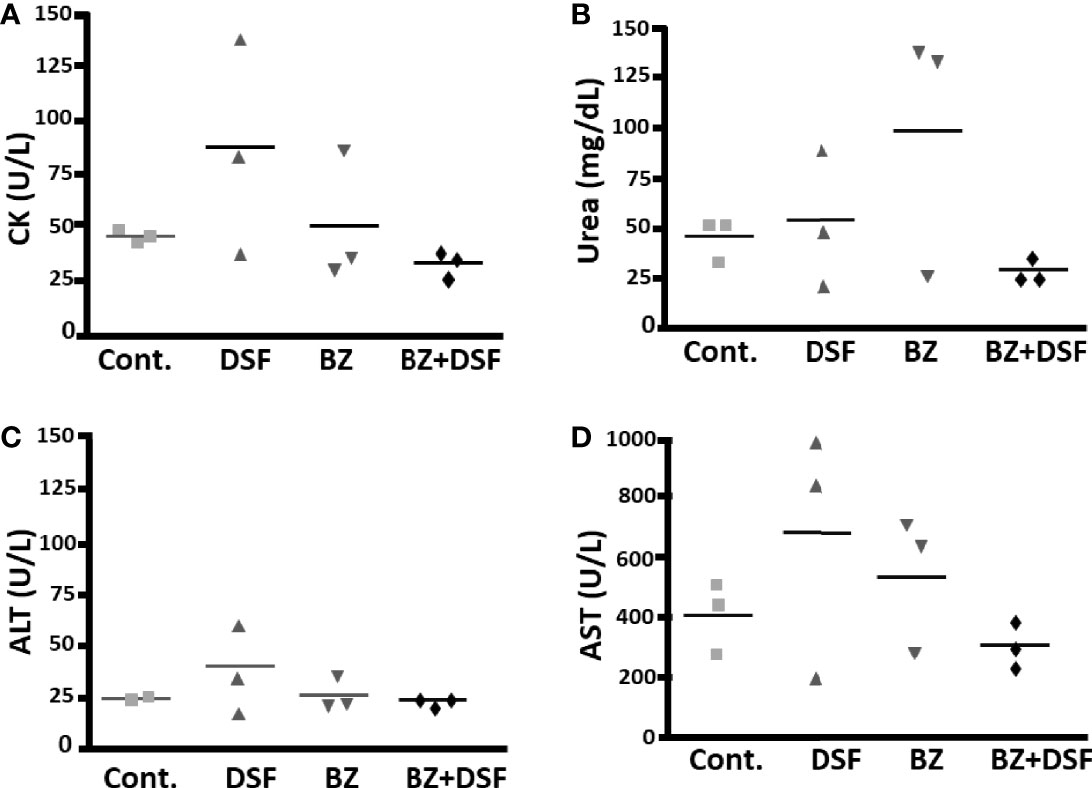
Figure 14 Systemic toxicity of mice treated with DSF and BZ, alone and in combination, by determining blood levels of creatine kinase (A), urea (B), alanine aminotransferase (C) and aspartate aminotransferase (D); after 20 mg/kg BZ or DSF and the 10 mg/kg/d BZ + 10 mg/kg/d DSF. Contrary to isolated drugs, the combination, employing lowered dosages, did not increase plasma levels.
Discussion
CD remains a major public health problem, as most of the infected people and domestic animals such as dogs, important reservoir hosts (Enriquez et al., 2014; Castillo-Neyra et al., 2015), are not treated or diagnosed (Dias et al., 2016). Since both drugs employed in CD chemotherapy are remarkably toxic (Castro and Diaz de Toranzo, 1988; Castro et al., 2006), therapy is frequently associated with severe adverse reactions, often causing treatment suspension (Levi et al., 1996; Olivera et al., 2017; Guggenbühl Noller et al., 2020; Pérez-Molina et al., 2021). Development of new safe and low-cost therapeutic alternatives is urgently required. Drug repositioning and combinations comprise valuable tools for overcoming CD monotherapy limitations (e.g., Aguilera et al., 2019).
Both DETC and DSF express antiparasitic activities upon T. cruzi (Lane et al., 1996; de Freitas Oliveira et al., 2021; de Freitas Oliveira et al., 2022) and Leishmania (Khouri et al., 2010; Celes et al., 2016; Mazur et al., 2019). In macrophage cultures, DETC caused destruction of intracellular amastigotes with little effect on macrophage spreading pattern. Similarly, in the murine infection, the combination reduced parasitemia and significantly (p < 0.001) enhanced animal survival by circa 6-fold. Synergistic combinations can enhance selectivity (Zimmermann et al., 2007; Lehár et al., 2009a; Lehár et al., 2009b). In fact, the BZ + DETC combination was synergistic in vitro and in vivo and produced over 10-fold selectivity index increase. Such synergistic activities may be caused by the prooxidant effects of BZ (Pedrosa et al., 2001; Rigalli et al., 2016), associated with the antagonism of antioxidant defenses of DSF and that can allow dosing reduction (Bustamante et al., 2014), presumably leading to decreased adverse reactions. Besides, DSF and DETC were shown to reduce adverse reactions of other drugs (vide supra). Here we observed that reduced combined drug concentrations did not impair effectivity. Therefore, such combinations may lead to the development of safe and effective medications.
Since one of the major problems in CD therapy is the prompt rise of resistance, we tested the combination in axenic amastigotes as well as in infected macrophages, not only of the Y strain but also of the Colombiana one, highly drug-resistant (Andrade et al., 1985). Interestingly, both strains were sensitive to the thiocarbamates at low µM range. Drug combinations were employed for overcoming drug resistance rising but were not effective in leishmaniasis (García-Hernández et al., 2012) or malaria (Wongsrichanalai et al., 2002) and drug combinations were not effective upon drug-resistant T. cruzi (Bustamante et al., 2014).
CD treatment is confronted with growing frequencies of refractory cases. Over 60% of T. cruzi strains isolated in Colombia display some degree of BZ resistance (Mejía-Jaramillo et al., 2012) and strains isolated in the Brazilian Amazon show natural resistance to this drug (Teston et al., 2013). The rise of readily acquired (Neal and van Bueren, 1988; Mejia et al., 2012) or natural resistance (Zingales, 2018) significantly limit the therapeutic success of CD. Thus, a major relevance of the DSF + BZ stems from the potential revert drug resistance phenotypes, by inhibiting both activity (Loo et al., 2004; Sauna et al., 2004) and expression (Loo and Clarke, 2000) of p-glycoproteins, which are involved in T. cruzi drug resistance (Campos et al., 2013).
Besides ABC cassette pumps (Zingales et al., 2015), natural resistance may rely on aldo-keto reductase and alcohol dehydrogenase (González et al., 2017). In this regard, it is important to notice that DSF inhibits alcohol dehydrogenase activity (Carper et al., 1987) and decreases the expression of aldo-keto reductase accessed by proteomic analysis (Yoshino et al., 2020). In addition, glutathione (GSH) forms conjugates with different drugs that are extruded by ABC transporters (Jedlitschky et al., 1994; Loe et al., 1998), so GSH and trypanothione (TSH) are implicated in detoxication of both NFX and BZ (Repetto et al., 1996). DSF can act as a glutathionylation agent (Hirschenson and Mailloux, 2021). Similarly, DETC derivatives form conjugates with GSH via carbamoylation (Ningaraj et al., 1998) and this reaction inhibits glutathione reductase (Miller and Blakely, 1992). T. cruzi ABC proteins externalize thiol-drug conjugates (da Costa et al., 2018) and inhibition of GSH and so TSH synthesis by buthionine sulfoximine (Maya et al., 2004; Faúndez et al., 2005; Vázquez et al., 2017) augments the effectivity of trypanocidal agents BZ and nifurtimox (Faúndez et al., 2005; Faúndez et al., 2008). Interestingly, DSF/DETC can diminish reduced GSH levels (Strömme, 1963; Ohno et al., 1990; Nagendra et al., 1994; Mittal et al., 2014) and GSH/TSH depletion can trigger T. cruzi programmed cell death (Piacenza et al., 2007). Here we observed reduced low mol. wt. thiol levels in DETC-treated T. cruzi, by colorimetric and cytometric approaches, presumably by the formation of DS/DETC thiol-mediated conjugates with GSH (Jin et al., 1994). Similarly DSF reducing GSH levels, potentiate antiparasitic activity in malaria experimental models (De Jongh, 1953a; De Jongh, 1953b; Deharo et al., 2003). Therefore, the use DSF may overcome T. cruzi drug-resistance via different mechanisms.
The ultrastructural analysis performed here revealed surface membrane discontinuities, which may be explained by lipid peroxidation, corroborated by TBA determination, and the cell surface damage may lead to loss of cytoplasmic content culminating in necrosis and cell collapse observed by SEM. The analysis by TEM revealed remarkable damage on parasite ER and mitochondria, redox-active organelles. Oxidative stress induces ER stress (Liu et al., 2019; Esmaeili et al., 2022) and DSF triggers ROS-dependent ER stress (Shah O’Brien et al., 2019). The ER stress is related to the mitochondrial one (Wang et al., 2016; Kim et al., 2022; Yuan et al., 2022) and the latter can be promoted by the DETC-mediated SOD inhibition as well as by the reductions in GSH levels.
DSF causes ER stress associated with ROS production (Shah O’Brien et al., 2019). ER stress may cause the organelle cisternae dilation (Montalbano et al., 2013), which are not as conspicuous as the remarkable enlargement observed in T. cruzi here, i.e., up to over 1000-fold, revealed by ultrastructural morphometry (not shown) and much higher than observed on mammalian cells. Such difference may be explained at least in part by a selective mechanism of action. It is noteworthy that ER stress was shown to be implicated in the CD cardiomyopathy in a murine model (Ayyappan et al., 2019). DETC can enhance ER stress in a kidney model (Kang et al., 2020), whereas its derivative DETC-MeSO (S-methyl-N, N-diethylthiocarbamate sulfoxide) can decrease ER stress markers in a stroke rat model (Mohammad-Gharibani et al., 2014).
The ubiquitin system comprises therapy target in diverse disorders (Wertz and Wang, 2019), and proteasome can be exploited as chemotherapy target for parasitic protozoa (Cromm and Crews, 2017; Xie et al., 2019). Parasite COP9 signalosomes comprise potential targets for chemotherapy (Ghosh et al., 2020). Interestingly DSF inhibits the ubiquitin-proteasome system (Cvek and Dvorak, 2008; Kona et al., 2011). The ER stress-associated cisternae dilation can be detected by EM (Riggs et al., 2005; Akiyama et al., 2009), so we employed the ultrastructural approach to help elucidating antiparasitic agents’ mechanisms of action (Vannier-Santos and De Castro, 2009). The inhibition of proteasome function causes the accumulation of precursor proteins within ER cisternae (Hoefer and Groettrup, 2021) and accumulation of misfolded proteins may cause ER dilation (Sun et al., 2014) and trigger oxidation-reduction futile cycles producing H2O2 and depleting reduced GSH pools in the ER (Malhotra et al., 2008).
The T. cruzi intracellular Ca2+ homeostasis is an important therapeutic target (Benaim et al., 2020) and calcium can be transferred from the ER to mitochondria during stress via mitochondria associated membranes (MAM), also termed ER–mitochondria encounter structure (ERMES), and the Ca2+ overload may be implicated in mitochondrial damage (Mohsin et al., 2020). We have previously described the ER-mitochondria connection in Leishmania braziliensis treated with histone deacetylase (HDAC) inhibitors, including the nuclear envelope in the trypanosomatid parasite (Ângelo de Souza et al., 2020). HDAC inhibitors cause ER stress (Chen et al., 2017) and lead to the formation of mitochondria-ER tight binding, associated with ER cisternae enlargement, but it was much less pronounced than reported here. A similar mitochondria-ER association was detected here (Figure 5E), but since both compartments were remarkably enlarged, an eventual connection interpretation could be misleading.
Like the ER alterations, the DETC mitochondrial effects observed on the parasite cells were not detected on the mammalian cells, as previously reported in Leishmania-infected macrophage (Khouri et al., 2010). Remarkably enlarged mitochondria with washed out matrix were previously observed in both T. cruzi (Menezes et al., 2006) and Leishmania amazonensis (Vannier-Santos et al., 2008) exposed to 1,4-diamino-2-butanone a biocide that impairs the T. cruzi redox homeostasis (Soares et al., 2012) and induces lipoperoxidation (Menezes et al., 2006; Vannier-Santos et al., 2008). This putrescine analogue can inhibit polyamine transport and biosynthesis, affecting numerous parasite cell functions (Vannier-Santos and Suarez-Fontes, 2017), therefore involving different pharmaceutical targets (Roberts and Ullman, 2017). Polyamines play a pivotal role in trypanosomatid parasites, including the antioxidant protection from lipid peroxidation (Hernández et al., 2006). Also, this polycation metabolism is important for the antioxidant role of the spermidine-GSH adduct Trypanothione (N1, N8-Bis(glutathionyl)spermidine, TSH). TSH metabolism and SOD are drug targets for T. cruzi infection (Beltran-Hortelano et al., 2017; Piñeyro et al., 2021). Both NFX and BZ interfere with TSH metabolism (Maya et al., 1997; Maya et al., 2007), and TSH confers T. cruzi resistance to BZ and NFX (Maya et al., 2004; Faúndez et al., 2005; Mesías et al., 2019). TSH and tryparedoxin take part in BZ-resistance (González-Chávez et al., 2019) and the enzyme comprises a drug target (González-Chávez et al., 2015).
The fluorescent probe H2DCFDA labeling indicates the ROS production and the oxidative stress triggering may be due both to SOD inhibition by DETC (Heikkila et al., 1976; Heikkila et al., 1978) as well as to lowering reduced glutathione levels (vide supra). The DSF-mediated glutathionylation enhances the O2●- and H2O2 production in mitochondria (Hirschenson and Mailloux, 2021) and SOD inhibition was previously shown to reduce T. cruzi parasitemia in murine infection (Olmo et al., 2015). The SOD inhibition observed here was significant on the parasite in vitro, but not in vivo, again possibly indicating a selective mechanism. Interestingly, the T. brucei SOD gene sodb1 deletion increases sensitivity to NFX and BZ (Prathalingham et al., 2007) and SOD inhibition enhances NFX antitumoral activity (Koto et al., 2011). In this regard, the DSF and DETC strongly inhibit T. cruzi SOD (Giulivi et al., 1988), and is trypanocidal (de Freitas Oliveira et al., 2021), also exerting leishmanicidal effects (Khouri et al., 2010). Interestingly, the T. cruzi Fe-SOD is found within parasite mitochondria, preventing programmed cell death (Piacenza et al., 2007). The immunofluorescence labeling of TcSOD in the parasite kinetoplast is relevant since it can preclude DNA fragmentation (Piacenza et al., 2007). In the TEM images shown here, we observed DETC-treated parasites with kDNA disorganization as well as mitochondrial damage. In this regard, arginase inhibition, causing redox imbalance, lead to kDNA disorganization in Leishmania (Cruz et al., 2013). SOD downmodulation may pose a dual advantage in CD therapy, since the enzymes are involved in intracellular survival, as scape mechanism from macrophage production of superoxide (Martínez et al., 2019), but also drug resistance (Nogueira et al., 2006; Campos et al., 2014; Campos et al., 2017). In this regard, the Sirtuin TcSir2rp3 induces the overexpressing TcFeSOD-A activities increasing T. cruzi resistance to BZ and NFX (dos Santos Moura et al., 2021).
Thiocarbamates chelate copper and trigger its accumulation and lipid peroxidation (Tonkin et al., 2004; Valentine et al., 2009; Viquez et al., 2009). Besides, copper-containing nanoparticles trigger oxidative stress and ER stress (Liu et al., 2021). Copper chelated by DSF induces cancer cells apoptosis in a ROS and mitochondria-dependent manner (Ren et al., 2021).
Ferroptosis is a programed cell death mechanism (Stockwell et al., 2017) characterized by mitochondria with enhanced matrix electron density and swollen cristae (Dixon et al., 2012). Such alterations were observed by TEM here, so this mechanism may be triggered by DSF. It should be kept in mind that different cell death mechanisms such as necrosis, apoptosis, and autophagy may be triggered simultaneously (dos Anjos et al., 2016). Interestingly, in a murine model of sepsis, the induction of pyroptosis and ferroptosis is associated with downregulation of the mitochondrial aldehyde dehydrogenase (Cao et al., 2022) and since DSF and DETC inhibit this enzyme (Deitrich and Erwin, 1971) may also trigger ferroptosis on parasite cells.
Inhibition of cystine uptake, employed in GSH (Badgley et al., 2020) synthesis, transport causes ER stress associated with ferroptosis in cancer cells (Dixon et al., 2014) and tryparedoxin peroxidase-deficient Trypanosoma brucei parasites undergo ferroptosis (Bogacz and Krauth-Siegel, 2018). Mitochondria are involved in cysteine-deprivation leading to ferroptosis (Gao et al., 2019).
The drug combination using DSF offers important advantages, which may be both economical (Cvek, 2012; Soave et al., 2016) and pharmacological, since this compound protects normal cells (Jia and Huang, 2021), can diminish the toxicity of different drugs such as cis-diamminedichloroplatinum (Wysor et al., 1982), including myocardial protection (Sonawane et al., 2018) and circa 30% of the CD patients develop heart disease, characterized by arrhythmias among other cardiac manifestations (Saraiva et al., 2021). It is worth mentioning that DSF can suppress cardiac arrhythmogenesis (Sander et al., 2021). Nevertheless, DETC and DSF were reported to cause neuropathies (Worner, 1982; Frisoni and Di Monda, 1989). In addition, BZ and NFX may cause neuropsychiatric reactions (Jackson et al., 2020). In this regard, it is noteworthy that DSF and its derivatives were also reported to act as neuroprotective agents (Zhao et al., 2000; Ningaraj et al., 2001; Libeu et al., 2012; Mohammad-Gharibani et al., 2014; Prentice et al., 2015). The eventual neurotoxic activity may be due at least in part to high dosages used in the past (Gessner and Gessner, 1992) and oxidative stress produced by copper accumulation leading to lipid peroxidation (Tonkin et al., 2004), although DSF may exert antioxidant effects (Kyle et al., 1989). This property is relevant since both BZ (Pedrosa et al., 2001; Rigalli et al., 2016) and CD (de Oliveira et al., 2007; Gupta et al., 2009) are associated with oxidative stress.
Histopathological samples revealed no amastigote nests detected in tissues of animals treated with low doses of the DSF-BZ combination, which showed mild inflammatory infiltrates and did not display significant fibrosis as assessed a by the picrosirius red staining. CD is an inflammatory infection (Talvani and Teixeira, 2011) associated with fibrosis and pyroptosis (Cerbán et al., 2020). The T. cruzi infection leads to ROS formation in the mitochondria, triggering PARP-1 and NF-kappaB activation, culminating in the production of pro-inflammatory cytokines such as Il-12 and IFN-γ (Ba et al., 2010), involved in cardiomyopathy pathophysiology (Cunha-Neto et al., 1998). In this regard, DSF is anti-inflammatory (Kanai et al., 2011), of potential use for inflammatory disorders (Guo et al., 2022), inhibiting oxidative stress and inflammasome activation, preventing cardiac damage (Wei et al., 2022), suppressing fibrosis (Zhang et al., 2021) and pyroptosis (Hu et al., 2020; Li et al., 2022; Zhou et al., 2022). The immune response to T. cruzi infection associated with myocardial fibrosis involves TGF-β (Chaves et al., 2019), and this cytokine comprises a therapeutic target in the CD cardiac damage (Waghabi et al., 2022). In this regard, DSF can inhibit TGF-β receptor signal transduction (Jiang et al., 2021).
Systemic toxicity assays based on the measurement of plasmatic CK, AST, ALT, and urea indicate decreased or insignificant toxicity. DSF was shown to reduce toxicity of several drugs such as cis-diamminedichloroplatinum, a property termed “disulfiram rescue” for saving the treated organism (Wysor et al., 1982; Roemeling et al., 1986). DETC is also considered a rescue agent (Gandara et al., 1990).
Because of promising in vitro and in vivo results, we are starting a clinical trial with the DSF-BZ combination (Saraiva et al., 2021), comprising a bona fide translational approach. Taken together, the present data and the cytoprotective capacity of DSF/DETC as well as the resistance reversion potential, such a combination may be safe and effective, even in refractory CD cases.
Data availability statement
The raw data supporting the conclusions of this article will be made available by the authors, without undue reservation.
Ethics statement
The animal study was reviewed and approved by Comissão de Ética no Uso de Animais do Instituto Oswaldo Cruz/Fiocruz. lic. no. L012/2020.
Author contributions
JA-S, DM, JF, MA, and DV-d-S: experiment execution; RS, AV, SP, AS-F: Data interpretation, discussion and translational research planning; SA, Histopathological analysis; MV-S: Project conception, study planning, funding obtaining, student supervision, microscopy interpretation; discussion. All authors contributed to the article and approved the submitted version.
Funding
Conselho Nacional de Desenvolvimento Científico e Tecnológico (CNPq), grant number 314717/2020 and Fundação Carlos Chagas Filho de Amparo à Pesquisa do Estado do Rio de Janeiro (FAPERJ) grant number 260475/2021, 259286/2021, provide most of the scholarships of the team as well as consumables used in the experiments. Oswaldo Cruz Institute (IOC)/Oswaldo Cruz Fundation (Fiocruz) provides the general infrastructure and supports the open access publication fees.
Acknowledgments
The authors acknowledge the valuable partnership of Farmanguinhos and INI/Fiocruz as well as the contributions FIOCRUZ technological platforms. To Dr. Marcus Fernandes Oliveira for valuable discussions and critical reading of the manuscript.
Conflict of interest
The authors declare that the research was conducted in the absence of any commercial or financial relationships that could be construed as a potential conflict of interest.
Publisher’s note
All claims expressed in this article are solely those of the authors and do not necessarily represent those of their affiliated organizations, or those of the publisher, the editors and the reviewers. Any product that may be evaluated in this article, or claim that may be made by its manufacturer, is not guaranteed or endorsed by the publisher.
References
Aguilera, E., Alvarez, G., Cerecetto, H., González, M. (2019). Polypharmacology in the treatment of Chagas disease. Curr. Med. Chem. 26 (23), 4476–4489. doi: 10.2174/0929867325666180410101728
Akiyama, M., Hatanaka, M., Ohta, Y., Ueda, K., Yanai, A., Uehara, Y., et al. (2009). Increased insulin demand promotes while pioglitazone prevents pancreatic beta cell apoptosis in Wfs1 knockout mice. Diabetologia 52 (4), 653–663. doi: 10.1007/s00125-009-1270-6
Andrade, S. G., Kloetzel, J. K., Borges, M. M., Ferrans, V. J. (1994). Morphological aspects of the myocarditis and myositis in Calomys callosus experimentally infected with Trypanosoma cruzi: fibrogenesis and spontaneous regression of fibrosis. Mem. Inst. Oswaldo Cruz. 89 (3), 379–393. doi: 10.1590/S0074-02761994000300017
Andrade, S. G., Magalhães, J. B., Pontes, A. L. (1985). Evaluation of chemotherapy with benznidazole and nifurtimox in mice infected with Trypanosoma cruzi strains of different types Bull. World Health Organ. 63 (4), 721–726.
Ângelo de Souza, L., Silva e Bastos, M., de Melo Agripino, J., Souza Onofre, T., Apaza Calla, L. F., Heimburg, T., et al. (2020). Histone deacetylases inhibitors as new potential drugs against Leishmania braziliensis, the main causative agent of new world tegumentary leishmaniasis. Biochem. Pharmacol. 180, 114191. doi: 10.1016/j.bcp.2020.114191
Askgaard, G., Friis, S., Hallas, J., Thygesen, L. C., Pottegård, A. (2014). Use of disulfiram and risk of cancer. Eur. J. Cancer Prev. 23 (3), 225–232. doi: 10.1097/CEJ.0b013e3283647466
Ayyappan, J. P., Lizardo, K., Wang, S., Yurkow, E., Nagajyothi, J. F. (2019). Inhibition of ER stress by 2-aminopurine treatment modulates cardiomyopathy in a murine chronic Chagas disease model. Biomol Ther. (Seoul). 27 (4), 386–394. doi: 10.4062/biomolther.2018.193
Badgley, M. A., Kremer, D. M., Maurer, H. C., DelGiorno, K. E., Lee, H. J., Purohit, V., et al. (2020). Cysteine depletion induces pancreatic tumor ferroptosis in mice. Science 368 (6486), 85–89. doi: 10.1126/science.aaw9872
Ba, X., Gupta, S., Davidson, M., Garg, N. J. (2010). Trypanosoma cruzi induces the reactive oxygen species-PARP-1-RelA pathway for up-regulation of cytokine expression in cardiomyocytes. J. Biol. Chem. 285 (15), 11596–11606. doi: 10.1074/jbc.M109.076984
Beltran-Hortelano, I., Alcolea, V., Font, M., Pérez-Silanes, S. (2022). Examination of multiple Trypanosoma cruzi targets in a new drug Discov approach for Chagas disease. Bioorg. Med. Chem. 58, 116577. doi: 10.1016/j.bmc.2021.116577
Beltran-Hortelano, I., Perez-Silanes, S., Galiano, S. (2017). Trypanothione reductase and superoxide dismutase as current drug targets for Trypanosoma cruzi: An overview of compounds with activity against Chagas disease. Curr. Med. Chem. 24 (11), 1066–1138. doi: 10.2174/0929867323666161227094049
Benaim, G., Paniz-Mondolfi, A. E., Sordillo, E. M., Martinez-Sotillo, N. (2020). Disruption of intracellular calcium homeostasis as a therapeutic target against Trypanosoma cruzi. Front. Cell. Infect. Microbiol. 10, 46. doi: 10.3389/fcimb.2020.00046
Bodenner, D. L., Dedon, P. C., Keng, P. C., Katz, J. C., Borch, R. F. (1986)Selective protection against cis-diamminedichloroplatinum(II)-induced toxicity in kidney, gut, and bone marrow by diethyldithiocarbamate Cancer Res. 46 (6), 2751–2755.
Bogacz, M., Krauth-Siegel, R. L. (2018). Tryparedoxin peroxidase-deficiency commits trypanosomes to ferroptosis-type cell death. ELife 7, e37503. doi: 10.7554/eLife.37503
Bustamante, J. M., Craft, J. M., Crowe, B. D., Ketchie, S. A., Tarleton, R. L. (2014). New, combined, and reduced dosing treatment protocols cure Trypanosoma cruzi infection in mice. J. Infect. Dis. 209 (1), 150–162. doi: 10.1093/infdis/jit420
Campos, M. C. O., Castro-Pinto, D. B., Ribeiro, G. A., Berredo-Pinho, M. M., Gomes, L. H. F., da Silva Bellieny, M. S., et al. (2013). P-glycoprotein efflux pump plays an important role in Trypanosoma cruzi drug resistance. Parasitol. Res. 112 (6), 2341–2351. doi: 10.1007/s00436-013-3398-z
Campos, M. C. O., Leon, L. L., Taylor, M. C., Kelly, J. M. (2014). Benznidazole-resistance in Trypanosoma cruzi: Evidence that distinct mechanisms can act in concert. Mol. Biochem. Parasitol. 193 (1), 17–19. doi: 10.1016/j.molbiopara.2014.01.002
Campos, M. C. O., Phelan, J., Francisco, A. F., Taylor, M. C., Lewis, M. D., Pain, A., et al. (2017). Genome-wide mutagenesis and multi-drug resistance in American trypanosomes induced by the front-line drug benznidazole. Sci. Rep. 7 (1), 14407. doi: 10.1038/s41598-017-14986-6
Cao, Z., Qin, H., Huang, Y., Zhao, Y., Chen, Z., Hu, J., et al. (2022). Crosstalk of pyroptosis, ferroptosis, and mitochondrial aldehyde dehydrogenase 2-related mechanisms in sepsis-induced lung injury in a mouse model. Bioengineered 13 (3), 4810–4820. doi: 10.1080/21655979.2022.2033381
Carper, W. R., Dorey, R. C., Beber, J. H. (1987) Inhibitory effect of disulfiram (Antabuse) on alcohol dehydrogenase activity. Clin. Chem. 33 (10), 1906–1908.
Castillo-Neyra, R., Chou Chu, L., Quispe-Machaca, V., Ancca-Juarez, J., Malaga Chavez, F. S., Bastos Mazuelos, M., et al. (2015). The potential of canine sentinels for reemerging Trypanosoma cruzi transmission. Prev. Vet. Med. 120 (3–4), 349–356. doi: 10.1016/j.prevetmed.2015.04.014
Castro, J. A., DeMecca, M. M., Bartel, L. C. (2006). Toxic side effects of drugs used to treat Chagas’ disease (American trypanosomiasis). Hum. Exp. Toxicol. 25 (8), 471–479. doi: 10.1191/0960327106het653oa
Castro, J. A., Diaz de Toranzo, E. G. (1988). Toxic effects of nifurtimox and benznidazole, two drugs used against American trypanosomiasis (Chagas’ disease). Biomed. Environ. Sci. 1 (1), 19–33.
Celes, F. S., Trovatti, E., Khouri, R., Van Weyenbergh, J., Ribeiro, S. J. L., Borges, V. M., et al. (2016). DETC-based bacterial cellulose bio-curatives for topical treatment of cutaneous leishmaniasis. Sci. Rep. 6 (1), 38330. doi: 10.1038/srep38330
Cerbán, F. M., Stempin, C. C., Volpini, X., Carrera Silva, E. A., Gea, S., Motran, C. C. (2020). Signaling pathways that regulate Trypanosoma cruzi infection and immune response. Biochim. Biophys. Acta Mol. Basis Dis. 1866 (5), 165707. doi: 10.1016/j.bbadis.2020.165707
Chaves, A. T., Menezes, C. A. S., Costa, H. S., Nunes, M. C. P., Rocha, M. O. C. (2019). Myocardial fibrosis in Chagas disease and molecules related to fibrosis. Parasite Immunol. 41 (10), e12663. doi: 10.1111/pim.12663
Chen, Y., Tsai, Y.-H., Tseng, S.-H. (2017). HDAC inhibitors and RECK modulate endoplasmic reticulum stress in tumor cells. Int. J. Mol. Sci. 18 (2), 258. doi: 10.3390/ijms18020258
Coura, J. R., Viñas, P. A. (2010). Chagas disease: a new worldwide challenge. Nature 465 (S7301), S6–S7. doi: 10.1038/nature09221
Crespillo-Andújar, C., Chamorro-Tojeiro, S., Norman, F., Monge-Maillo, B., López-Vélez, R., Pérez-Molina, J. A. (2018). Toxicity of nifurtimox as second-line treatment after benznidazole intolerance in patients with chronic Chagas disease: when available options fail. Clin. Microbiol. Infect. 24 (12), 1344.e1–1344.e4. doi: 10.1016/j.cmi.2018.06.006
Cromm, P. M., Crews, C. M. (2017). Targeted protein degradation: from chemical biology to drug Discov. Cell Chem. Biol. 24 (9), 1181–1190. doi: 10.1016/j.chembiol.2017.05.024
Cruz, E., de, M., da Silva, E. R., Maquiaveli, C.do C., Alves, E. S., Lucon, J. F., Jr., et al. (2013). Leishmanicidal activity of Cecropia pachystachya flavonoids: arginase inhibition and altered mitochondrial DNA arrangement. Phytochemistry 89, 71–77. doi: 10.1016/j.phytochem.2013.01.014
Cunha-Neto, E., Rizzo, L. V., Albuquerque, F., Abel, L., Guilherme, L., Bocchi, E., et al. (1998). Cytokine production profile of heart-infiltrating T cells in Chagas’ disease cardiomyopathy. Braz. J. Med. Biol. Res. 31 (1), 133–137. doi: 10.1590/S0100-879X1998000100018
Cvek, B. (2012). Nonprofit drugs as the salvation of the world’s healthcare systems: the case of antabuse (disulfiram). Drug Discov Today 17 (9–10), 409–412. doi: 10.1016/j.drudis.2011.12.010
Cvek, B., Dvorak, Z. (2008). The value of proteasome inhibition in cancer. Drug Discov Today 13 (15–16), 716–722. doi: 10.1016/j.drudis.2008.05.003
da Costa, K. M., Valente, R. C., Salustiano, E. J., Gentile, L. B., Freire-de-Lima, L., Mendonça-Previato, L., et al. (2018). Functional characterization of ABCC proteins from Trypanosoma cruzi and their involvement with thiol transport. Front. Microbiol. 9. doi: 10.3389/fmicb.2018.00205
de Freitas Oliveira, J. W., da Silva, M. F. A., Damasceno, I. Z., Rocha, H. A. O., da Silva Júnior, A. A., Silva, M. S. (2022). In vitro validation of antiparasitic activity of PLA-nanoparticles of sodium diethyldithiocarbamate against Trypanosoma cruzi. Pharmaceutics 14 (3), 497. doi: 10.3390/pharmaceutics14030497
de Freitas Oliveira, J. W., Torres, T. M., Moreno, C. J. G., Amorim-Carmo, B., Damasceno, I. Z., Soares, A. K. M. C., et al. (2021). Insights of antiparasitic activity of sodium diethyldithiocarbamate against different strains of Trypanosoma cruzi. Sci. Rep. 11 (1), 11200. doi: 10.1038/s41598-021-90719-0
Deharo, E., Barkan, D., Krugliak, M., Golenser, J., Ginsburg, H. (2003). Potentiation of the antimalarial action of chloroquine in rodent malaria by drugs known to reduce cellular glutathione levels. Biochem. Pharmacol. 66 (5), 809–817. doi: 10.1016/S0006-2952(03)00396-4
Deitrich, R. A., Erwin, V. G. (1971). Mechanism of the inhibition of aldehyde dehydrogenase in vivo by disulfiram and diethyldithiocarbamate. Mol. Pharmacol. 7 (3), 301–307.
De Jongh, D. K. (1953a). Effect of tetra-ethylthiuramdisulphide (T.T.S.) on the antimalarial activity of quinine in Plasmodium berghei infections in mice. Acta Physiol. Pharmacol. Neerl. 3 (1), 34–37.
De Jongh, D. K. (1953b). Effect of tetra-ethylthiuramdisulphide on the antimalarial activity of quinine in Plasmodium gallinaceum infections in chickens. Acta Physiol. Pharmacol. Neerl. 3 (1), 38–44.
de Oliveira, T. B., Pedrosa, R. C., Filho, D. W. (2007). Oxidative stress in chronic cardiopathy associated with Chagas disease. Int. J. Cardiol. 116 (3), 357–363. doi: 10.1016/j.ijcard.2006.04.046
Dias, J. C. P., Novaes Ramos, A., Dias Gontijo, E., Luquetti, A., Shikanai-Yasuda, M. A., Rodrigues Coura, J., et al. (2016). II consenso brasileiro em doença de chaga. Epid. Serv. Saúde 25 (21), 1–10. doi: 10.5123/S1679-49742016000500002
Dixon, S. J., Lemberg, K. M., Lamprecht, M. R., Skouta, R., Zaitsev, E. M., Gleason, C. E., et al. (2012). Ferroptosis: an iron-dependent form of nonapoptotic cell death. Cell 149 (5), 1060–1072. doi: 10.1016/j.cell.2012.03.042
Dixon, S. J., Patel, D. N., Welsch, M., Skouta, R., Lee, E. D., Hayano, M., et al. (2014). Pharmacological inhibition of cystine–glutamate exchange induces endoplasmic reticulum stress and ferroptosis. ELife 3, e02523. doi: 10.7554/eLife.02523
dos Anjos, D. O., Sobral Alves, E. S., Gonçalves, V. T., Fontes, S. S., Nogueira, M. L., Suarez-Fontes, A. M., et al. (2016). Effects of a novel β–lapachone derivative on Trypanosoma cruzi: Parasite death involving apoptosis, autophagy and necrosis. Int. J. Parasitol. Drugs Drug Resist. 6 (3), 207–219. doi: 10.1016/j.ijpddr.2016.10.003
dos Santos Moura, L., Santana Nunes, V., Gomes, A. A. S., Sousa, A. C., de, C. N., Fontes, M. R. M., et al. (2021). Mitochondrial sirtuin TcSir2rp3 affects TcSODA activity and oxidative stress response in Trypanosoma cruzi. Front. Cell. Infect. Microbiol. 11, 773410. doi: 10.3389/fcimb.2021.773410
Duschak, V. G. (2011). A decade of targets and patented drugs for chemotherapy of cChagas disease. Recent Pat. Anti-Infect. Drug Discov 6 (3), 216–259. doi: 10.2174/157489111796887864
Duschak, V. G. (2016). Targets and patented drugs for chemotherapy of Chagas disease in the last 15 years-period. Recent Pat. Anti-Infect. Drug Discov 11 (2), 74–173. doi: 10.2174/1574891X11666161024165304
Duschak, V. G. (2019). Major kinds of drug targets in Chagas disease or American trypanosomiasis. Curr. Drug Targets 20 (11), 1203–1216. doi: 10.2174/1389450120666190423160804
Elliott, W. C., Newcom, S. R., Houghton, D. C., Baines-Hunter, J., Bennett, W. M. (1983). Cis-diamminedichloroplatinum(II) nephrotoxicity: Tubular function after rescue with sodium diethyldithiocarbamate in rats. Cancer Res. 43 (8), 3759–3762.
Engel, J. C., Franke de Cazzulo, B. M., Stoppani, A. O., Cannata, J. J., Cazzulo, J. J. (1987). Aerobic glucose fermentation by Trypanosoma cruzi axenic culture amastigote-like forms during growth and differentiation to epimastigotes. Mol. Biochem. Parasitol. 26 (1-2), 1–10. doi: 10.1016/0166-6851(87)90123-x
Enriquez, G. F., Bua, J., Orozco, M. M., Wirth, S., Schijman, A. G., Gürtler, R. E., et al. (2014). High levels of Trypanosoma cruzi DNA determined by qPCR and infectiousness to Triatoma infestans support dogs and cats are major sources of parasites for domestic transmission. Infect. Genet. Evol. 25, 36–43. doi: 10.1016/j.meegid.2014.04.002
Esmaeili, Y., Yarjanli, Z., Pakniya, F., Bidram, E., Łos, M. J., Eshraghi, M., et al. (2022). Targeting autophagy, oxidative stress, and ER stress for neurodegenerative disease treatment. J. Control Release 345, 147–175. doi: 10.1016/j.jconrel.2022.03.001
Faúndez, M., López-Muñoz, R., Torres, G., Morello, A., Ferreira, J., Kemmerling, U., et al. (2008). Buthionine sulfoximine has anti-Trypanosoma cruzi activity in a murine model of acute Chagas’ disease and enhances the efficacy of nifurtimox. Antimicrob. Agents Chemother. 52 (5), 1837–1839. doi: 10.1128/AAC.01454-07
Faúndez, M., Pino, L., Letelier, P., Ortiz, C., López, R., Seguel, C., et al. (2005). Buthionine sulfoximine increases the toxicity of nifurtimox and benznidazole to Trypanosoma cruzi. Antimicrob. Agents Chemother. 49 (1), 126–130. doi: 10.1128/AAC.49.1.126-130.2005
Fivelman, Q. L., Adagu, I. S., Warhurst, D. C. (2004). Modified fixed-ratio isobologram method for studying in vitro interactions between atovaquone and proguanil or dihydroartemisinin against drug-resistant strains of Plasmodium falciparum. Antimicrob. Agents Chemother. 48 (11), 4097–4102. doi: 10.1128/AAC.48.11.4097-4102.2004
Franco-Paredes, C., Bottazzi, M. E., Hotez, P. J. (2009). The unfinished public health agenda of Chagas disease in the era of globalization. PloS Negl. Trop. Dis. 3 (7), e470. doi: 10.1371/journal.pntd.0000470
Frisoni, G. B., Di Monda, V. (1989). Disulfiram neuropathy: a review 1971-1988) and report of a case. Alcohol Alcohol. 24 (5), 429–437.
Gandara, D. R., Wiebe, V. J., Perez, E. A., Makuch, R. W., DeGregorio, M. W. (1990). Cisplatin rescue therapy: Experience with sodium thiosulfate, WR2721, and diethyldithiocarbamate. Crit. Rev. Oncol. Hematol. 10 (4), 353–365. doi: 10.1016/1040-8428(90)90010-P
Gao, M., Yi, J., Zhu, J., Minikes, A. M., Monian, P., Thompson, C. B., et al. (2019). Role of mitochondria in ferroptosis. Mol. Cell. 73 (2), 354–363.e3. doi: 10.1016/j.molcel.2018.10.042
García-Hernández, R., Manzano, J. I., Castanys S and Gamarro, F. (2012). Leishmania donovani develops resistance to drug combinations. PloS Negl. Trop. Dis. 6 (12), e1974. doi: 10.1371/journal.pntd.0001974
Gessner, P. K., Gessner, T. (1992). Disulfiram and its metabolite, diethyldithiocarbamate. Springer Netherlands 452 pp. doi: 10.1007/978-94-011-2328-0
Ghosh, S., Farr, L., Singh, A., Leaton, L. A., Padalia, J., Shirley, D. A., et al. (2020). COP9 signalosome is an essential and druggable parasite target that regulates protein degradation. PloS Pathog. 16 (9), e1008952. doi: 10.1371/journal.ppat.1008952
Giulivi, C., Turrens, J. F., Boveris, A. (1988). Chemiluminescence enhancement by trypanocidal drugs and by inhibitors of antioxidant enzymes in Trypanosoma cruzi. Mol. Biochem. Parasitol. 30 (3), 243–251. doi: 10.1016/0166-6851(88)90093-X
González-Chávez, Z., Olin-Sandoval, V., Rodíguez-Zavala, J. S., Moreno-Sánchez, R., Saavedra, E. (2015). Metabolic control analysis of the Trypanosoma cruzi peroxide detoxification pathway identifies tryparedoxin as a suitable drug target. Biochim. Biophys. Acta 1850 (2), 263–273. doi: 10.1016/j.bbagen.2014.10.029
González-Chávez, Z., Vázquez, C., Mejia-Tlachi, M., Márquez-Dueñas, C., Manning-Cela, R., Encalada, R., et al. (2019). Gamma-glutamylcysteine synthetase and tryparedoxin 1 exert high control on the antioxidant system in Trypanosoma cruzi contributing to drug resistance and infectivity. Redox Biol. 26, 101231. doi: 10.1016/j.redox.2019.101231
González, L., García-Huertas, P., Triana-Chávez, O., García, G. A., Murta, S. M. F., Mejía-Jaramillo, A. M. (2017). Aldo-keto reductase and alcohol dehydrogenase contribute to benznidazole natural resistance in Trypanosoma cruzi. Mol. Microbiol. 106 (5), 704–718. doi: 10.1111/mmi.13830
Guggenbühl Noller, J. M., Froeschl, G., Eisermann, P., Jochum, J., Theuring, S., Reiter-Owona, I., et al. (2020). Describing nearly two decades of Chagas disease in Germany and the lessons learned: A retrospective study on screening, detection, diagnosis, and treatment of Trypanosoma cruzi infection from 2000 – 2018. BMC Infect. Dis. 20 (1), 919. doi: 10.1186/s12879-020-05600-8
Guo, W., Chen, S., Li, C., Xu, J., Wang, L. (2022). Application of disulfiram and its metabolites in treatment of inflammatory disorders. Front. Pharmacol. 12. doi: 10.3389/fphar.2021.795078
Gupta, S., Wen, J. J., Garg, N. J. (2009). Oxidative stress in Chagas disease. Interdiscip. Perspect. Infect. Dis. 2009, 1–8. doi: 10.1155/2009/190354
Harrington, B. S., Ozaki, M. K., Caminear, M. W., Hernandez, L. F., Jordan, E., Kalinowski, N. J., et al. (2020). Drugs targeting tumor-initiating cells prolong survival in a post-surgery, post-chemotherapy ovarian cancer relapse model. Cancers 12 (6), 1645. doi: 10.3390/cancers12061645
Heikkila, R. E., Cabbat, F. S., Cohen, G. (1976). In vivo inhibition of superoxide dismutase in mice by diethyldithiocarbamate. J. Biol. Chem. 251 (7), 2182–2185. doi: 10.1016/S0021-9258(17)33675-X
Heikkila, R. E., Cabbat, F. S., Cohen, G. (1978). Inactivation of superoxide dismutase by several thiocarbamic acid derivatives. Experientia 34 (12), 1553–1554. doi: 10.1007/BF02034668
Hernández, S. M., Sánchez, M. S., de Tarlovsky, M. N. S. (2006). Polyamines as a defense mechanism against lipoperoxidation in Trypanosoma cruzi. Acta Trop. 98 (1), 94–102. doi: 10.1016/j.actatropica.2006.02.005
Hersh, E. M., Brewton, G., Abrams, D., Bartlett, J., Galpin, J., Gill, P., et al. (1991). Ditiocarb sodium (diethyldithiocarbamate) therapy in patients with symptomatic HIV infection and AIDS. A randomized, double-blind, placebo-controlled, multicenter study. JAMA 265 (12), 1538–1544. doi: 10.1001/jama.1991.03460120052035
Hill, J. A., Cowen, L. E. (2015). Using combination therapy to thwart drug resistance. Future Microbiol. 10 (11), 1719–1726. doi: 10.2217/fmb.15.68
Hirschenson, J., Mailloux, R. J. (2021). The glutathionylation agent disulfiram augments superoxide/hydrogen peroxide production when liver mitochondria are oxidizing ubiquinone pool-linked and branched chain amino acid substrates. Free Radic. Biol. Med. 172, 1–8. doi: 10.1016/j.freeradbiomed.2021.05.030
Hoefer, F., Groettrup, M. (2021). Silencing of the proteasome and oxidative stress impair endoplasmic reticulum targeting and signal cleavage of a prostate carcinoma antigen. Biochem.Biophys. Res. Commun. 554, 56–62. doi: 10.1016/j.bbrc.2021.03.023
Hu, J. J., Liu, X., Xia, S., Zhang, Z., Zhang, Y., Zhao, J., et al. (2020). FDA-Approved disulfiram inhibits pyroptosis by blocking gasdermin d pore formation. Nat. Immunol. 21 (7), 736–745. doi: 10.1038/s41590-020-0669-6
Jørgensen, C. H., Pedersen, B., Tønnesen, H. (2011). The efficacy of disulfiram for the treatment of alcohol use disorder. Alcoholism: Clin. Exp. Res. 35 (10), 1749–1758. doi: 10.1111/j.1530-0277.2011.01523.x
Jackson, Y., Wyssa, B., Chappuis, F. (2020). Tolerance to nifurtimox and benznidazole in adult patients with chronic Chagas’ disease. J. Antimicrob. Chemother. 75 (3), 690–696. doi: 10.1093/jac/dkz473
Jedlitschky, G., Leier, I., Buchholz, U., Center, M., Keppler, D. (1994). ATP-dependent transport of glutathione s-conjugates by the multidrug resistance-associated protein. Cancer Res. 54 (18), 4833–4836.
Jia, Y., Huang, T. (2021). Overview of antabuse® (Disulfiram) in radiation and cancer biology. Cancer Manage. Res. 13, 4095–4101. doi: 10.2147/CMAR.S308168
Jiang, H., Zhao, Y., Tang, H., Duan, S., Li, M., Yang, X., et al. (2021). Tetraethylthiuram disulphide alleviates pulmonary fibrosis through modulating transforming growth factor-β signalling. Pharmacol. Res. 174, 105923. doi: 10.1016/j.phrs.2021.105923
Jin, L., Davis, M. R., Hu, P., Baillie, T. A. (1994). Identification of novel glutathione conjugates of disulfiram and diethyldithiocarbamate in rat bile by liquid chromatography-tandem mass spectrometry. evidence for metabolic activation of disulfiram in vivo. Chem. Res. Toxicol. 7 (4), 526–533. doi: 10.1021/tx00040a008
Kanai, K., Itoh, N., Ito, Y., Nagai, N., Hori, Y., Chikazawa, S., et al. (2011). Anti-inflammatory potency of oral disulfiram compared with dexamethasone on endotoxin-induced uveitis in rats. J. Vet. Med. Science. 73 (4), 517–520. doi: 10.1292/jvms.10-0239
Kang, J., Sun, Y., Deng, Y., Liu, Q., Li, D., Liu, Y., et al. (2020). Autophagy-endoplasmic reticulum stress inhibition mechanism of superoxide dismutase in the formation of calcium oxalate kidney stones. Biomed. Pharmacother. 121, 109649. doi: 10.1016/j.biopha.2019.109649
Kannappan, V., Ali, M., Small, B., Rajendran, G., Elzhenni, S., Taj, H., et al. (2021). Recent advances in repurposing disulfiram and disulfiram derivatives as copper-dependent anticancer agents. Front. Mol. Biosci. 8, 741316. doi: 10.3389/fmolb.2021.741316
Khouri, R., Novais, F., Santana, G., de Oliveira, C. I., Vannier dos Santos, M. A., Barral, A., et al. (2010). DETC induces Leishmania parasite killing in human in vitro and murine in vivo models: A promising therapeutic alternative in leishmaniasis. PloS One 5 (12), e14394. doi: 10.1371/journal.pone.0014394
Kim, S. H., Seo, H., Kwon, D., Yuk, D. Y., Jung, Y. S. (2022). Taurine ameliorates tunicamycin-induced liver injury by disrupting the vicious cycle between oxidative stress and endoplasmic reticulum stress. Life 12 (3), 354. doi: 10.3390/life12030354
Kona, F. R., Buac, D., Burger, A. (2011). Disulfiram, and disulfiram derivatives as novel potential anticancer drugs targeting the ubiquitin proteasome system in both preclinical and clinical studies. Curr. Cancer Drug Targets 11 (3), 338–346. doi: 10.2174/156800911794519798. XXXM.
Koto, K. S., Lescault, P., Brard, L., Kim, K., Singh, R. K., Bond, J., et al. (2011). Antitumor activity of nifurtimox is enhanced with tetrathiomolybdate in medulloblastoma. Int. J. Oncol. 38 (5), 1329–1341. doi: 10.3892/ijo.2011.971
Kyle, M. E., Serroni, A., Farber, J. L. (1989). The inhibition of lipid peroxidation by disulfiram prevents the killing of cultured hepatocytes by allyl alcohol, tert-butyl hydroperoxide, hydrogen peroxide and diethyl maleate. Chem. Biol. Interact. 72 (3), 269–275. doi: 10.1016/0009-2797(89)90003-3
Lane, J. E., Ribeiro-Rodrigues, R., Suarez, C. C., Bogitsh, B. J., Jones, M. M., Singh, P. K., et al. (1996). In vitro trypanocidal activity of tetraethylthiuram disulfide and sodium diethylamine-n-carbodithioate on Trypanosoma cruzi. Am. J. Trop. Med. Hyg. 55 (3), 263–266. doi: 10.4269/ajtmh.1996.55.263
Lee, B. Y., Bacon, K. M., Bottazzi, M. E., Hotez, P. J. (2013). Global economic burden of Chagas disease: a computational simulation model. Lancet Infect. Dis. 13 (4), 342–348. doi: 10.1016/S1473-3099(13)70002-1
Lehár, J., Krueger, A. S., Avery, W., Heilbut, A. M., Johansen, L. M., Price, E. R., et al. (2009a). Synergistic drug combinations tend to improve therapeutically relevant selectivity. Nat. Biotechnol. 27 (7), 659–666. doi: 10.1038/nbt.1549
Lehár, J., Krueger, A. S., Zimmermann, G. R., Borisy, A. A. (2009b). Therapeutic selectivity and the multi-node drug target. Discov Med. 8 (43), 185–190.
Levi, G. C., Lobo, I. M. F., Kallás, E. G., Amato Neto, V. (1996). Etiological drug treatment of human infection by Trypanosoma cruzi. Rev. Inst. Med. Trop. São Paulo. 38 (1), 35–38. doi: 10.1590/S0036-46651996000100007
Libeu, C. A. P., Descamps, O., Zhang, Q., John, V., Bredesen, D. E. (2012). Altering APP proteolysis: Increasing sAPPalpha production by targeting dimerization of the APP ectodomain. PloS One 7 (6), e40027. doi: 10.1371/journal.pone.0040027
Liu, Y., Tong, Z., Shi, J., Li, R., Upton, M., Wang, Z. (2021). Drug repurposing for next-generation combination therapies against multidrug-resistant bacteria. Theranostics 11 (10), 4910–4928. doi: 10.7150/thno.56205
Liu, X., Zhang, R., Huang, L., Zheng, Z., Vlassara, H., Striker, G., et al. (2019). Excessive oxidative stress contributes to increased acute ER stress kidney injury in aged mice. Oxid. Med. Cell. Longev. 2019, 1–15. doi: 10.1155/2019/2746521
Li, N., Wang, Y., Wang, X., Sun, N., Gong, Y.-H. (2022). Pathway network of pyroptosis and its potential inhibitors in acute kidney injury. Pharmacol. Res. 175, 106033. doi: 10.1016/j.phrs.2021.106033
Loe, D. W., Deeley, R. G., Cole, S. P. (1998). Characterization of vincristine transport by the Mr 190,000 multidrug resistance protein (MRP): evidence for cotransport with reduced glutathione. Cancer Res. 58 (22), 5130–5136.
Loo, T. W., Bartlett, M. C., Clarke, D. M. (2004). Disulfiram metabolites permanently inactivate the human multidrug resistance p-glycoprotein. Mol. Pharm. 1 (6), 426–433. doi: 10.1021/mp049917l
Loo, T. W., Clarke, D. M. (2000). Blockage of drug resistance in vitro by disulfiram, a drug used to treat alcoholism. J. Nat. Cancer Inst. 92 (11), 898–902. doi: 10.1093/jnci/92.11.898
Lu, C., Li, X., Ren, Y., Zhang, X. (2021). Disulfiram: a novel repurposed drug for cancer therapy. Cancer Chemother. Pharmacol. 87 (2), 159–172. doi: 10.1007/s00280-020-04216-8
Lu, Y., Pan, Q., Gao, W., Pu, Y., Luo, K., He, B., et al. (2022). Leveraging disulfiram to treat cancer: mechanisms of action, delivery strategies, and treatment regimens. Biomaterials 281, 121335. doi: 10.1016/j.biomaterials.2021.121335
Malhotra, J. D., Miao, H., Zhang, K., Wolfson, A., Pennathur, S., Pipe, S. W., et al. (2008). Antioxidants reduce endoplasmic reticulum stress and improve protein secretion. Proc. Natl. Acad. Sci. 105 (47), 18525–18530. doi: 10.1073/pnas.0809677105
Martínez, A., Prolo, C., Estrada, D., Rios, N., Alvarez, M. N., Piñeyro, M. D., et al. (2019). Cytosolic Fe-superoxide dismutase safeguards Trypanosoma cruzi from macrophage-derived superoxide radical. Proc. Natl. Acad. Sci. 116 (18), 8879–8888. doi: 10.1073/pnas.1821487116
Martiny, A., Vannier-Santos, M. A., Borges, V. M., Meyer-Fernandes, J. R., Assreuy, J., Cunha e Silva, N. L., et al. (1996). Leishmania-induced tyrosine phosphorylation in the host macrophage and its implication to infection. Eur. J. Cell Biol. 71 (2), 206–215.
Maya, J. D., Cassels, B. K., Iturriaga-Vásquez, P., Ferreira, J., Faúndez, M., Galanti, N., et al. (2007). Mode of action of natural and synthetic drugs against Trypanosoma cruzi and their interaction with the mammalian host. Comp. Biochem. Physiol. A. Mol. Integr. Physiol. 146 (4), 601–620. doi: 10.1016/j.cbpa.2006.03.004
Maya, J. D., Repetto, Y., Agosín, M., Ojeda, J. M., Tellez, R., Gaule, C., et al. (1997). Effects of nifurtimox and benznidazole upon glutathione and trypanothione content in epimastigote, trypomastigote and amastigote forms of Trypanosoma cruzi. Mol. Biochem. Parasitol. 86 (1), 101–106. doi: 10.1016/S0166-6851(96)02837-X
Maya, J. D., Rodríguez, A., Pino, L., Pabón, A., Ferreira, J., Pavani, M., et al. (2004). Effects of buthionine sulfoximine nifurtimox and benznidazole upon trypanothione and metallothionein proteins in Trypanosoma cruzi. Biol. Res. 37 (1), 61–69. doi: 10.4067/S0716-97602004000100007
Mazur, K. L., Feuser, P. E., Valério, A., Poester Cordeiro, A., de Oliveira, C. I., Assolini, J. P., et al. (2019). Diethyldithiocarbamate loaded in beeswax-copaiba oil nanoparticles obtained by solventless double emulsion technique promote promastigote death in vitro. Colloids Surf. B. Biointerfaces. 176, 507–512. doi: 10.1016/j.colsurfb.2018.12.048
Mejia, A. M., Hall, B. S., Taylor, M. C., Gómez-Palacio, A., Wilkinson, S. R., Triana-Chávez, O., et al. (2012). Benznidazole-resistance in Trypanosoma cruzi is a readily acquired trait that can arise independently in a single population. J. Infect. Dis. 206 (2), 220–228. doi: 10.1093/infdis/jis331
Mejía-Jaramillo, A. M., Fernández, G. J., Montilla, M., Nicholls, R. S., Triana-Chávez, O. (2012). Trypanosoma cruzi strains resistant to benznidazole occurring in Colombia. Biomedica 32 (2), 196–205. doi: 10.1590/S0120-41572012000300007
Menezes, D., Valentim, C., Oliveira, M. F., Vannier-Santos, M. A. (2006). Putrescine analogue cytotoxicity against Trypanosoma cruzi. Parasitol. Res. 98 (2), 99–105. doi: 10.1007/s00436-005-0010-1
Meraz-Torres, F., Plöger, S., Garbe, C., Niessner, H., Sinnberg, T. (2020). Disulfiram as a therapeutic agent for metastatic malignant melanoma–old myth or new logos? Cancers 12 (12), 3538. doi: 10.3390/cancers12123538
Mesías, A. C., Sasoni, N., Arias, D. G., Pérez Brandán, C., Orban, O. C. F., Kunick, C., et al. (2019). Trypanothione synthetase confers growth, survival advantage and resistance to anti-protozoal drugs in Trypanosoma cruzi. Free Rad. Biol. Med. 130, 23–34. doi: 10.1016/j.freeradbiomed.2018.10.436
Miller, A. C., Blakely, W. F. (1992). Inhibition of glutathione reductase activity by a carbomoylating nitrosourea: Effect on cellular radiosensitivity. Free Rad. Biol. Med. 12 (1), 53–62. doi: 10.1016/0891-5849(92)90058-O
Mittal, M., Khan, K., Pal, S., Porwal, K., China, S. P., Barbhuyan, T. K., et al. (2014). The thiocarbamate disulphide drug, disulfiram induces osteopenia in rats by inhibition of osteoblast function due to suppression of acetaldehyde dehydrogenase activity. Toxicol. Sci. 139 (1), 257–270. doi: 10.1093/toxsci/kfu020
Mohammad-Gharibani, P., Modi, J., Menzie, J., Genova, R., Ma, Z., Tao, R., et al. (2014). Mode of action of s-Methyl-N, n-diethylthiocarbamate sulfoxide (DETC-MeSO) as a novel therapy for stroke in a rat model. Mol. Neurobiol. 50 (2), 655–672. doi: 10.1007/s12035-014-8658-0
Mohsin, A. A., Thompson, J., Hu, Y., Hollander, J., Lesnefsky, E. J., Chen, Q. (2020). Endoplasmic reticulum stress-induced complex I defect: Central role of calcium overload. Arch. Biochem. Biophys. 683, 108299. doi: 10.1016/j.abb.2020.108299
Montalbano, R., Waldegger, P., Quint, K., Jabari, S., Neureiter, D., Illig, R., et al. (2013). Endoplasmic reticulum stress plays a pivotal role in cell death mediated by the pan-deacetylase inhibitor panobinostat in human hepatocellular cancer cells. Transl. Oncol. 6 (2), 143–IN6. doi: 10.1593/tlo.12271
Monteiro, A. C., Abrahamson, M., Lima, A. P., Vannier-Santos, M. A., Scharfstein, J. (2001). Identification, characterization and localization of Chagasin, a tight-binding cysteine protease inhibitor in Trypanosoma cruzi. J. Cell Sci. 114 (Pt 21), 3933–3942. doi: 10.1242/jcs.114.21.3933
Moukdar, F., Robidoux, J., Lyght, O., Pi, J., Daniel, K. W., Collins, S. (2009). Reduced antioxidant capacity and diet-induced atherosclerosis in uncoupling protein-2-deficient mice. J. Lipid. Res. 50 (1), 59–70. doi: 10.1194/jlr.M800273-JLR200
Müller, J., Hemphill, A. (2016). Drug target identification in protozoan parasites. Expert. Opin. Drug Discov 11 (8), 815–824. doi: 10.1080/17460441.2016.1195945
Nagendra, S. N., Shetty, K. T., Rao, K. M., Rao, B. S. (1994). Effect of disulfiram administration on rat brain glutathione metabolism. Alcohol 11 (1), 7–10. doi: 10.1016/0741-8329(94)90004-3
Neal, R. A., van Bueren, J. (1988). Comparative studies of drug susceptibility of five strains of Trypanosoma cruzi in vivo and in vitro. Trans. R. Soc Trop. Med. Hyg. 82 (5), 709–714. doi: 10.1016/0035-9203(88)90208-8
Nechushtan, H., Hamamreh, Y., Nidal, S., Gotfried, M., Baron, A., Shalev, Y. I., et al. (2015). A phase IIb trial assessing the addition of disulfiram to chemotherapy for the treatment of metastatic non-small cell lung cancer. Oncologist 20 (4), 366–367. doi: 10.1634/theoncologist.2014-0424
Ningaraj, N. S., Chen, W., Schloss, J. V., Faiman, M. D., Wu, J. Y. (2001). S-methyl-N,N-diethylthiocarbamate sulfoxide elicits neuroprotective effect against n-methyl-D-aspartate receptor-mediated neurotoxicity. J. Biomed. Sci. 8 (1), 104–113. doi: 10.1007/BF02255978
Ningaraj, N. S., Schloss, J. V., Williams, T. D., Faiman, M. D. (1998). Glutathione carbamoylation with s-methyl N,N-diethylthiolcarbamate sulfoxide and sulfone. Biochem. Pharmacol. 55 (6), 749–756. doi: 10.1016/S0006-2952(97)00513-3
Nogueira, F. B., Krieger, M. A., Nirdé, P., Goldenberg, S., Romanha, A. J., Murta, S. M. F. (2006). Increased expression of iron-containing superoxide dismutase-a (TcFeSOD-a) enzyme in Trypanosoma cruzi population with in vitro-induced resistance to benznidazole. Acta Trop. 100 (1–2), 119–132. doi: 10.1016/j.actatropica.2006.10.004
O’Brien, A., Barber, J. E. B., Reid, S., Niknejad, N., Dimitroulakos, J. (2012). Enhancement of cisplatin cytotoxicity by disulfiram involves activating transcription factor 3. Anticancer Res. 32 (7), 2679–2688.
Ohno, Y., Hirota, K., Kawanishi, T., Takanaka, A. (1990). Loss of viability after disulfiram treatment without preceding depletion of intracellular GSH. J. Toxicol. Sci. 15 (2), 63–73. doi: 10.2131/jts.15.63
Olivera, M. J., Cucunubá, Z. M., Valencia-Hernández, C. A., Herazo, R., Agreda-Rudenko, D., Flórez, C., et al. (2017). Risk factors for treatment interruption and severe adverse effects to benznidazole in adult patients with Chagas disease. PloS One 12 (9), e0185033. doi: 10.1371/journal.pone.0185033
Olmo, F., Urbanová, K., Rosales, M. J., Martín-Escolano, R., Sánchez-Moreno, M., Marín, C. (2015). An in vitro iron superoxide dismutase inhibitor decreases the parasitemia levels of Trypanosoma cruzi in BALB/c mouse model during acute phase. Int. J. Parasitol. Drugs Drug Resist. 5 (3), 110–116. doi: 10.1016/j.ijpddr.2015.05.002
Palos, I., Lara-Ramirez, E. E., Lopez-Cedillo, J. C., Garcia-Perez, C., Kashif, M., Bocanegra-Garcia, V., et al. (2017). Repositioning FDA drugs as potential cruzain inhibitors from Trypanosoma cruzi: Virtual screening, in vitro and in vivo studies. Molecules 22 (6), 1015. doi: 10.3390/molecules22061015
Parker, E. R., Sethi, A. (2011). Chagas disease: Coming to a place near you. Dermatol. Clin. 29 (1), 53–62. doi: 10.1016/j.det.2010.08.011
Pedrosa, R. C., de Bem, A. F., Locatelli, C., Pedrosa, R. C., Geremias, R., Filho, D. W. (2001). Time-dependent oxidative stress caused by benznidazole. Redox Rep. 6 (4), 265–270. doi: 10.1179/135100001101536328
Pérez-Molina, J. A., Crespillo-Andújar, C., Bosch-Nicolau, P., Molina, I. (2021). Trypanocidal treatment of Chagas disease. Enferm. Infecc. Microbiol. Clin. (Engl Ed) 39 (9), 458–470. doi: 10.1016/j.eimce.2020.04.012
Pérez-Molina, J. A., Molina, I. (2018). Chagas disease. Lancet 391 (10115), 82–94. doi: 10.1016/S0140-6736(17)31612-4
Piacenza, L., Irigoín, F., Alvarez, M. N., Peluffo, G., Taylor, M. C., Kelly, J. M., et al. (2007). Mitochondrial superoxide radicals mediate programmed cell death in Trypanosoma cruzi: cytoprotective action of mitochondrial iron superoxide dismutase overexpression. Biochem. J. 403 (2), 323–334. doi: 10.1042/BJ20061281
Piñeyro, M. D., Arias, D., Parodi-Talice, A., Guerrero, S., Robello, C. (2021). Trypanothione metabolism as drug target for trypanosomatids. Curr. Pharm. Des. 27 (15), 1834–1846. doi: 10.2174/1381612826666201211115329
Prathalingham, S. R., Wilkinson, S. R., Horn, D., Kelly, J. M. (2007). Deletion of the Trypanosoma brucei superoxide dismutase gene sodb1 increases sensitivity to nifurtimox and benznidazole. Antimicrob. Agents Chemother. 51 (2), 755–758. doi: 10.1128/AAC.01360-06
Prentice, H., Modi, J. P., Wu, J. Y. (2015). Mechanisms of neuronal protection against excitotoxicity, endoplasmic reticulum stress, and mitochondrial dysfunction in stroke and neurodegenerative diseases. Oxid. Med. Cell. Long. 2015, 1–7. doi: 10.1155/2015/964518
Ren, Y., Lin, Y., Chen, J., Jin, Y. (2021). Disulfiram chelated with copper promotes apoptosis in osteosarcoma via ROS/mitochondria pathway. Biol. Pharm. Bull. 44 (10), b21–00466. doi: 10.1248/bpb.b21-00466
Repetto, Y., Opazo, E., Maya, J. D., Agosin, M., Morello, A. (1996). Glutathione and trypanothione in several strains of Trypanosoma cruzi: Effect of drugs. Comp. Biochem. Physiol. B Biochem. Mol. Biol. 115 (2), 281–285. doi: 10.1016/0305-0491(96)00112-5
Rigalli, J. P., Perdomo, V. G., Ciriaci, N., Francés, D. E., Ronco, M. T., Bataille, A. M., et al. (2016). The antitripanocide benznidazole promotes adaptive response to oxidative injury: Involvement of the nuclear factor-erythroid 2-related factor-2 (Nrf2) and multidrug resistance associated protein 2 (MRP2). Toxicol. Appl. Pharmacol. 304, 90–98. doi: 10.1016/j.taap.2016.05.007
Riggs, A. C., Bernal-Mizrachi, E., Ohsugi, M., Wasson, J., Fatrai, S., Welling, C., et al. (2005). Mice conditionally lacking the wolfram gene in pancreatic islet beta cells exhibit diabetes as a result of enhanced endoplasmic reticulum stress and apoptosis. Diabetologia 48 (11), 2313–2321. doi: 10.1007/s00125-005-1947-4
Roberts, S., Ullman, B. (2017). Parasite polyamines as pharmaceutical targets. Curr. Pharm. Des. 23 (23), 3325–3341. doi: 10.2174/1381612823666170601101644
Roemeling, R. V., Olshefski, R., Langevin, T., Berestka, J., Reusch, J. J., Reusch, J. E. B., et al. (1986). Cisplatin chronotherapy and disulfiram rescue reduce toxicity without interfering with anticancer activity: animal findings and preliminary clinical experiences. Chronobiol. Int. 3 (1), 55–64. doi: 10.3109/07420528609083160
Salomão, K., de Souza, E. M., Carvalho, S. A., da Silva, E. F., Fraga, C. A. M., Barbosa, H. S., et al. (2010). In vitro and in vivo activities of 1,3,4-thiadiazole-2-arylhydrazone derivatives of megazol against Trypanosoma cruzi. Antimicrob. Agents Chemother. 54 (5), 2023–2031. doi: 10.1128/AAC.01241-09
Sander, P., Feng, M., Schweitzer, M. K., Wilting, F., Gutenthaler, S. M., Arduino, D. M., et al. (2021). Approved drugs ezetimibe and disulfiram enhance mitochondrial Ca2+ uptake and suppress cardiac arrhythmogenesis. Br. J. Pharmacol. 178 (22), 4518–4532. doi: 10.1111/bph.15630
Saraiva, R. M., Portela, L. F., Silveira, G.P.Eda, Gomes, N. L. da S., Pinto, D. P., Silva, A. C. A., et al. (2021). Disulfiram repurposing in the combined chemotherapy of Chagas disease. Med.: Case Rep. Study Protoc. 2 (7), e0110. doi: 10.1097/MD9.0000000000000110
Sauna, Z. E., Peng, X.-H., Nandigama, K., Tekle, S., Ambudkar, S. V. (2004). The molecular basis of the action of disulfiram as a modulator of the multidrug resistance-linked ATP binding cassette transporters MDR1 (ABCB1) and MRP1 (ABCC1). Mol. Pharmacol. 65 (3), 675–684. doi: 10.1124/mol.65.3.675
Schmidtova, S., Kalavska, K., Gercakova, K., Cierna, Z., Miklikova, S., Smolkova, B., et al. (2019). Disulfiram overcomes cisplatin resistance in human embryonal carcinoma cells. Cancers 11 (9), 1224. doi: 10.3390/cancers11091224
Sedlak, J., Lindsay, R. H. (1968). Estimation of total, protein-bound, and nonprotein sulfhydryl groups in tissue with ellman’s reagent. Anal. Biochem. 25, 192–205. doi: 10.1016/0003-2697(68)90092-4
Shah O’Brien, P., Xi, Y., Miller, J. R., Brownell, A. L., Zeng, Q., Yoo, G. H., et al. (2019). Disulfiram (Antabuse) activates ROS-dependent ER stress and apoptosis in oral cavity squamous cell carcinoma. J. Clin. Med. 8 (5), 611. doi: 10.3390/jcm8050611
Sinclair, J. M. A., Chambers, S. E., Shiles, C. J., Baldwin, D. S. (2016). Safety and tolerability of pharmacological treatment of alcohol dependence: comprehensive review of evidence. Drug Saf. 39 (7), 627–645. doi: 10.1007/s40264-016-0416-y
Soares, C. O., Colli, W., Bechara, E. J. H., Alves, M. J. M. (2012). 1,4-Diamino-2-butanone, a putrescine analogue, promotes redox imbalance in Trypanosoma cruzi and mammalian cells. Arch. Biochem. Biophys. 528 (2), 103–110. doi: 10.1016/j.abb.2012.09.005
Soave, C., Viola-Rhenals, M., Elghoul, Y. K., Peng, T., Dou, Q. P. (2016). Repositioning an old anti-alcoholism drug: disulfiram as a selective, effective and economical anticancer agent. J. Dev. Drugs 05 (02), 1000e147. doi: 10.4172/2329-6631.1000e147
Sonawane, V. K., Mahajan, U. B., Shinde, S. D., Chatterjee, S., Chaudhari, S. S., Bhangale, H. A., et al. (2018). A chemosensitizer drug: disulfiram prevents doxorubicin-induced cardiac dysfunction and oxidative stress in rats. Cardiovasc.Toxicol 18 (5), 459–470. doi: 10.1007/s12012-018-9458-y
Stockwell, B. R., Friedmann Angeli, J. P., Bayir, H., Bush, A. I., Conrad, M., Dixon, S. J., et al. (2017). Ferroptosis: A regulated cell death nexus linking metabolism, redox biology, and disease. Cell 171 (2), 273–285. doi: 10.1016/j.cell.2017.09.021
Strömme, J. H. (1963). Effects of diethyldithiocarbamate and disulfiram on glucose metabolism and glutathione content of human erythrocytes. Biochem. Pharmacol. 12 (7), 705–715. doi: 10.1016/0006-2952(63)90046-7
Sueth-Santiago, V., Moraes, J. B., Sobral Alves, E. S., Vannier-Santos, M. A., Freire-de-Lima, C. G., Castro, R. N., et al. (2016). The effectiveness of natural diarylheptanoids against Trypanosoma cruzi: cytotoxicity, ultrastructural alterations and molecular modeling studies. PloS One 11 (9), e0162926. doi: 10.1371/journal.pone.0162926
Sun, W., Sanderson, P. E., Zheng, W. (2016). Drug combination therapy increases successful drug repositioning. Drug Discov Today 21 (7), 1189–1195. doi: 10.1016/j.drudis.2016.05.015
Sun, S., Shi, G., Han, X., Francisco, A. B., Ji, Y., Mendonça, N., et al. (2014). Sel1L is indispensable for mammalian endoplasmic reticulum-associated degradation, endoplasmic reticulum homeostasis, and survival. Proc. Natl. Acad. Sci. U.S.A. 111 (5), E582–E591. doi: 10.1073/pnas.1318114111
Talvani, A., Teixeira, M. M. (2011). Inflammation and Chagas disease. Adv. Parasitol. 76, 171–194. doi: 10.1016/B978-0-12-385895-5.00008-6
Teston, A. P. M., Monteiro, W. M., Reis, D., Bossolani, G. D. P., Gomes, M. L., de Araújo, S. M., et al. (2013). In vivo susceptibility to benznidazole of Trypanosoma cruzi strains from the Western Brazilian Amazon. Trop. Med. Int. Health 18 (1), 85–95. doi: 10.1111/tmi.12014
Tonkin, E. G., Valentine, H. L., Milatovic, D. M., Valentine, W. M. (2004). N,N-diethyldithiocarbamate produces copper accumulation, lipid peroxidation, and myelin injury in rat peripheral nerve. Toxicol. Sci. 81 (1), 160–171. doi: 10.1093/toxsci/kfh190
Valentine, H. L., Viquez, O. M., Amarnath, K., Amarnath, V., Zyskowski, J., Kassa, E. N., et al. (2009). Nitrogen substituent polarity influences dithiocarbamate-mediated lipid oxidation, nerve copper accumulation, and myelin injury. Chem. Res. Toxicol. 22 (1), 218–226. doi: 10.1021/tx8003714
Vannier-Santos, M., De Castro, S. (2009). Electron microscopy in antiparasitic chemotherapy: a (close) view to a kill. Curr. Drug Targets 10 (3), 246–260. doi: 10.2174/138945009787581168
Vannier-Santos, M. A., Lins, U. (2001). Cytochemical techniques and energy-filtering transmission electron microscopy applied to the study of parasitic protozoa. Biol. Proced. Online. 3, 8–18. doi: 10.1251/bpo19
Vannier-Santos, M. A., Menezes, D., Oliveira, M. F., de Mello, F. G. (2008). The putrescine analogue 1,4-diamino-2-butanone affects polyamine synthesis, transport, ultrastructure and intracellular survival in. Leishmania amazonensis Microbiol. 154 (10), 3104–3111. doi: 10.1099/mic.0.2007/013896-0
Vannier-Santos, M. A., Suarez-Fontes, A. M. (2017). Role of polyamines in parasite cell architecture and function. Curr. Pharm. Des. 23 (23), 3342–3358. doi: 10.2174/1381612823666170703163458
Vázquez, C., Mejia-Tlachi, M., González-Chávez, Z., Silva, A., Rodríguez-Zavala, J. S., Moreno-Sánchez, R., et al. (2017). Buthionine sulfoximine is a multitarget inhibitor of trypanothione synthesis in Trypanosoma cruzi. FEBS Lett. 591 (23), 3881–3894. doi: 10.1002/1873-3468.12904
Viquez, O. M., Lai, B., Ahn, J. H., Does, M. D., Valentine, H. L., Valentine, W. M. (2009). N,N-diethyldithiocarbamate promotes oxidative stress prior to myelin structural changes and increases myelin copper content. Toxicol. Appl. Pharmacol. 239 (1), 71–79. doi: 10.1016/j.taap.2009.05.017
Waghabi, M. C., Ferreira, R. R., Abreu, R., da, S., Degrave, W., de Souza, E. M., et al. (2022). Transforming growth factor-ß as a therapeutic target for the cardiac damage of Chagas disease. Mem. Inst. Oswaldo Cruz. 117, e210395. doi: 10.1590/0074-02760210395
Wang, J., Yang, X., Zhang, J. (2016). Bridges between mitochondrial oxidative stress, ER stress and mTOR signaling in pancreatic β cells. Cell. Signal. 28 (8), 1099–1104. doi: 10.1016/j.cellsig.2016.05.007
Wei, S., Xiao, Z., Huang, J., Peng, Z., Zhang, B., Li, W. (2022). Disulfiram inhibits oxidative stress and NLRP3 inflammasome activation to prevent LPS-induced cardiac injury. Int. Immunopharmacol. 105, 108545. doi: 10.1016/j.intimp.2022.108545
Wertz, I. E., Wang, X. (2019). From Discov to bedside: targeting the ubiquitin system. Cell Chem. Biol. 26 (2), 156–177. doi: 10.1016/j.chembiol.2018.10.022
WHO - World Health Organization. (2021) Chagas disease (American trypanosomiasis). Available at: https://www.who.int/health-topics/Chagas-disease#tab=tab_1 (Accessed March 13, 2022).
Wongsrichanalai, C., Pickard, A. L., Wernsdorfer, W. H., Meshnick, S. R. (2002). Epidemiology of drug-resistant malaria. Lancet Infect. Dis. 2 (4), 209–218. doi: 10.1016/s1473-3099(02)00239-6
Worner, T. M. (1982). Peripheral neuropathy after disulfiram administration: Reversibility despite continued therapy. Drug Alcohol Depend. 10 (2–3), 199–201. doi: 10.1016/0376-8716(82)90013-8
Wysor, M. S., Zwelling, L. A., Sanders, J. E., Grenan, M. M. (1982). Cure of mice infected with Trypanosoma rhodesiense by cis-diamminedichloroplatinum (ii) and disulfiram rescue. Science 217 (4558), 454–456. doi: 10.1126/science.7201165
Xie, S. C., Dick, L. R., Gould, A., Brand, S., Tilley, L. (2019). The proteasome as a target for protozoan parasites. Expert Opin. Ther. Targets 23 (11), 903–914. doi: 10.1080/14728222.2019.1685981
Yang, Y., Deng, Q., Feng, X., Sun, J. (2015). Use of the disulfiram/copper complex for breast cancer chemoprevention in MMTV-erbB2 transgenic mice. Mol. Med. Rep. 12 (1), 746–752. doi: 10.3892/mmr.2015.3426
Yang, Z., Guo, F., Albers, A. E., Sehouli, J., Kaufmann, A. M. (2019). Disulfiram modulates ROS accumulation and overcomes synergistically cisplatin resistance in breast cancer cell lines. Biomed. Pharmacother. 113, 108727. doi: 10.1016/j.biopha.2019.108727
Yoshino, H., Yamada, Y., Enokida, H., Osako, Y., Tsuruda, M., Kuroshima, K., et al. (2020). Targeting NPL4 via drug repositioning using disulfiram for the treatment of clear cell renal cell carcinoma. PloS One 15 (7), e0236119. doi: 10.1371/journal.pone.0236119
Yuan, M., Gong, M., He, J., Xie, B., Zhang, Z., Meng, L., et al. (2022). IP3R1/GRP75/VDAC1 complex mediates endoplasmic reticulum stress-mitochondrial oxidative stress in diabetic atrial remodeling. Redox Biol. 52, 102289. doi: 10.1016/j.redox.2022.102289
Zhang, Y., Zhang, R., Han, X. (2021). Disulfiram inhibits inflammation and fibrosis in a rat unilateral ureteral obstruction model by inhibiting gasdermin d cleavage and pyroptosis. Inflamm. Res. 70 (5), 543–552. doi: 10.1007/s00011-021-01457-y
Zhao, A., Wu, Z.-Q., Pollack, M., Rollwagen, F. M., Hirszel, P., Zhou, X. (2000). Disulfiram inhibits TNF-alpha-induced cell death. Cytokine 12 (9), 1356–1367. doi: 10.1006/cyto.2000.0725
Zheng, W., Sun, W., Simeonov, A. (2018). Drug repurposing screens and synergistic drug-combinations for infectious diseases. Br. J. Pharmacol. 175 (2), 181–191. doi: 10.1111/bph.13895
Zhou, Q., Wang, W., Yang, F., Wang, H., Zhao, X., Zhou, Y., et al. (2022). Disulfiram suppressed peritendinous fibrosis through inhibiting macrophage accumulation and its pro-inflammatory properties in tendon bone healing. Front. Bioeng. Biotechnol. 10, 823933. doi: 10.3389/fbioe.2022.823933
Zimmermann, G. R., Lehár, J., Keith, C. T. (2007). Multi-target therapeutics: when the whole is greater than the sum of the parts. Drug Discov Today 12 (1–2), 34–42. doi: 10.1016/j.drudis.2006.11.008
Zingales, B. (2018). Trypanosoma cruzi genetic diversity: Something new for something known about Chagas disease manifestations, serodiagnosis and drug sensitivity. Acta Trop. 184, 38–52. doi: 10.1016/j.actatropica.2017.09.017
Keywords: Trypanosoma cruzi, disulfiram, drug combination, repositioning, Chagas disease, chemotherapy, Diethyldithiocarbamate
Citation: Almeida-Silva J, Menezes DS, Fernandes JMP, Almeida MC, Vasco-dos-Santos DR, Saraiva RM, Viçosa AL, Perez SAC, Andrade SG, Suarez-Fontes AM and Vannier-Santos MA (2022) The repositioned drugs disulfiram/diethyldithiocarbamate combined to benznidazole: Searching for Chagas disease selective therapy, preventing toxicity and drug resistance. Front. Cell. Infect. Microbiol. 12:926699. doi: 10.3389/fcimb.2022.926699
Received: 22 April 2022; Accepted: 27 June 2022;
Published: 29 July 2022.
Edited by:
Gustavo Benaim, Fundación Instituto de Estudios Avanzados (IDEA), VenezuelaReviewed by:
Alan Talevi, National University of La Plata, ArgentinaMarta Helena Branquinha, Federal University of Rio de Janeiro, Brazil
Copyright © 2022 Almeida-Silva, Menezes, Fernandes, Almeida, Vasco-dos-Santos, Saraiva, Viçosa, Perez, Andrade, Suarez-Fontes and Vannier-Santos. This is an open-access article distributed under the terms of the Creative Commons Attribution License (CC BY). The use, distribution or reproduction in other forums is permitted, provided the original author(s) and the copyright owner(s) are credited and that the original publication in this journal is cited, in accordance with accepted academic practice. No use, distribution or reproduction is permitted which does not comply with these terms.
*Correspondence: Marcos André Vannier-Santos, marcos.vannier@ioc.fiocruz.br