Protective efficacy of Toxoplasma gondii GRA12 or GRA7 recombinant proteins encapsulated in PLGA nanoparticles against acute Toxoplasma gondii infection in mice
- 1Institute of Animal Husbandry and Veterinary Medicine, Department of Animal Parasitology, Zhejiang Academy of Agricultural Sciences, Hangzhou, China
- 2Institute of Animal Science and Technology, Department of Animal Diseases Diagnosis and Control of Xinjiang Production & Construction Corps, Tarim University, Alar, China
- 3Department of Animal Epidemic Surveillance, Zhejiang Provincial Animal Disease Prevention and Control Center, Hangzhou, China
Background: Toxoplasma gondii is an apicomplexan parasite that affects the health of humans and livestock, and an effective vaccine is urgently required. Nanoparticles can modulate and improve cellular and humoral immune responses.
Methods: In the current study, poly (D, L-lactic-co-glycolic acid) (PLGA) nanoparticles were used as a delivery system for the T. gondii dense granule antigens GRA12 and GRA7. BALB/c mice were injected with the vaccines and protective efficacy was evaluated.
Results: Mice immunized with PLGA+GRA12 exhibited significantly higher IgG, and a noticeable predominance of IgG2a over IgG1 was also observed. There was a 1.5-fold higher level of lymphocyte proliferation in PLGA+GRA12-injected mice compared to Alum+GRA12-immunized mice. Higher levels of IFN-g and IL-10 and a lower level of IL-4 were detected, indicating that Th1 and Th2 immune responses were induced but the predominant response was Th1. There were no significant differences between Alum+GRA7-immunized and PLGA+GRA7-immunized groups. Immunization with these four vaccines resulted in significantly reduced parasite loads, but they were lowest in PLGA+GRA12-immunized mice. The survival times of mice immunized with PLGA+GRA12 were also significantly longer than those of mice in the other vaccinated groups.
Conclusion: The current study indicated that T. gondii GRA12 recombinant protein encapsulated in PLGA nanoparticles is a promising vaccine against acute toxoplasmosis, but PLGA is almost useless for enhancing the immune response induced by T. gondii GRA7 recombinant protein.
1 Introduction
Toxoplasma gondii is an obligate intracellular eukaryotic parasite that can invade and replicate in almost all warm-blooded animals including mammals and birds (Dubey et al., 1970; Dubremetz, 1998). Cats (wild and domestic felids) are the only known definitive hosts for T. gondii and can excrete millions of environmentally resistant oocysts (Dubey, 2001). Approximately one third of people worldwide are chronically infected with T. gongii. Although the majority of infections in healthy adults are usually asymptomatic or have relatively mild symptoms, in immunocompromised individuals (such as AIDS patients, transplant recipients, and cancer patients) or in congenitally infected children, infection can lead to severe disease or even death (Hill and Dubey, 2002). Toxoplasmosis is one of the main causes of death in immunocompromised patients. It can be found in most parts of the world (Tenter et al., 2000), and can lead to indirect economy loss due to reproductive disorders (Boughattas et al., 2011). The life cycle of T. gondii is complex, there are three infectious stage of T. gondii: the tachyzoites, the bradyzoites (in tissue cysts) and the sporozoites (in oocysts). The sexual phase only occurs in the intestine of definitive hosts (felids) (Konradt et al., 2016). In intermediate hosts, T. gondii is present in two stages, rapid-replicating tachyzoites and slow-growing bradyzoites. The tachyzoites are responsible for acute infection, and the bradyzoites are responsible for chronic infection (Dubey et al., 1998; Montoya and Liesenfeld, 2004). Humans acquire infection by consuming food or water contaminated with oocysts shed from cats or raw or undercooked meat containing tissue cysts (Tenter et al., 2000).
Currently the only available toxoplasmosis vaccine is Toxovax (Intervet Inc., Angers, France), a live vaccine used for prevention in goats and sheep, which is unlikely to be used in humans (Buxton and Innes, 1995; Jongert et al., 2009). Conventional anti-T. gondii drugs have several disadvantages such as serious adverse side effects, limited therapeutic efficacy, emergence of drug-resistant parasites, and poor effects against T. gondii cysts in tissues (Zhang et al., 2013; Liu et al., 2017). Furthermore, reinfection can occur because of the complicated life cycle of the parasite (Konstantinovic et al., 2019). Hence, the development of an effective vaccine against toxoplasmosis has long been a goal. In recent years the protective effects of numerous T. gondii antigens have been evaluated, and the dense granule antigens (GRAs) are potential candidates for vaccines which may prevent T. gondii infection (Golkar et al., 2007; Hiszczyńska-Sawicka et al., 2011; Zheng et al., 2019). The presence of GRA7 in a DNA vaccine triggered strong antibody responses and higher levels of IFN-γ, indicating that GRA7 should be a main component of vaccines against T. gondii (Jongert et al., 2007). GRA12 is a key T. gondii virulence factor that resists host innate immunity triggered by IFN-γ (Fox et al., 2019). In a bioinformatics study GRA12 exhibited good antigenicity and several excellent B cell and T cell epitopes, indicating that GRA12 may be a worthwhile inclusion in a vaccine against T. gondii infection (Ghaffari et al., 2020).
Several synthetic delivery systems have facilitated enhanced immunogenicity of antigenic vaccine components (Jongert et al., 2009), such as Poly (D, L-lactic-co-glycolic acid) (PLGA) nanoparticles (NPs) (Allahyari and Mohit, 2016), which can be metabolized to their monomers in aqueous media and taken up by antigen presenting cells (APCs) through binding to pattern recognition molecules such as Toll like receptors (TLRs). PLGA could efficiently promote antigen targeting towards dendritic cells (DCs) and the uptake of PLGA particles by DCs can be occurred without any specific recognition because of the size of the particles were similar to the pathogens. PLGA NPs can inhibit protein degradation and prolong the release of the protein (Roozbehani et al., 2018). PLGA approved by the Food and Drug Administration (FDA) is characterized by nontoxicity, good biocompatibility and can provide sustained protein release, which is important to elicit potent immune responses (Allahyari et al., 2016; Chereddy et al., 2016). They are considered a promising T. gondii vaccine delivery vehicle.
In the current study vaccines containing recombinant T. gondii GRA7 and GRA12 proteins encapsulated in PLGA NPs were designed and administered to mice, and immunogenicity and protective efficacy were evaluated. In other mice Alum was included as an adjuvant. In fact, one candidate antigen can induce only partial protective immunity against Toxoplasma, and the development of a combined vaccine consisting of various stages of the parasite’s life history (tachyzoites, bradyzoites, and sporozoites) may be necessary for complete protective immunity in the future study.
2 Materials and methods
2.1 Mice
BALB/c mice aged 6–8 weeks were obtained from the Laboratory Animal Center of Zhejiang Academy of Agricultural Sciences. All protocols were approved by the Animal Care Committee of Zhejiang Academy of Agricultural Sciences in accordance with the recommendations of the National Institutes of Health Guide for the Care and Use of Laboratory Animals (Ethics protocol No. 2021ZAASLA65). All mice were kept under the conditions specified in these approved protocols.
2.2 Cell culture and parasite acquisition
Vero cells were cultured in Dulbecco’s Modified Eagle Medium (DMEM, Gibco, CA, USA) supplemented with 10% fetal bovine serum (FBS), 100 U/mL penicillin, and 100 μg/mL streptomycin. The cells were maintained at 37°C in a humidified incubator containing 5% CO2. T. gondii RH strain tachyzoites were maintained in Vero cells, then tachyzoites from cell cultures were collected by passing the cells through a 27-gauge needle 3–5 times. Lastly, the parasites were washed with phosphate-buffered saline (PBS) three times, then the cell fragments were filtered through a 3-μm pore membrane filter.
2.3 Prokaryotic expression and preparation of polyclonal antibody
Total RNA from T. gondii RH strain tachyzoites was obtained using TRIzol reagent in accordance with the manufacturer’s instructions (Invitrogen, USA). The cDNA was amplified via a reverse transcription kit (TransGen Biotech, China). The CDS sequence of GRA12 without the hydrophobic signal peptide was amplified via the polymerase chain reaction (PCR) with primers, 5’-GCGAATTCATGCGACATGTTGGCGGTTTCTCGG-3’ (forward), 5’-CCAAGCTTTCAGTTGTGTTTGCTGCCTGCAGAG-3’ (reverse). The primers contain EcoRI and HindIII restriction sites (underlined). PCR product was recovered using a DNA purification kit and constructed into the pEASY-T1 vector (TransGen Biotech, China). The clones were sequenced (Sangon Biotech, China), then constructed into the expression vector pET32-a (Takara, Japan). The recombinant plasmid was transferred into Escherichia coli BL21 cells (Takara, Japan), then bacterial cultures were induced by 1 mM Isopropyl-beta-D-thiogalactopyranoside (IPTG) and the recombinant protein was identified by sodium dodecyl sulfate-polyacrylamide gel electrophoresis (SDS-PAGE) with a 12% polyacrylamide gel. Western blotting analyses were performed to verify the structure of the recombinant protein. GRA12-pET32a-his fusion protein was then purified using Ni-NTA purification agarose (GE, USA).
he protein was injected intraperitoneally into mice aged 6–8 weeks to generate polyclonal antibody. Briefly, the protein was fully emulsified with Freund’s complete adjuvant (Thermo Fisher Scientific, USA) at a ratio of 1:1 and the injection dose was 100 μg/mouse for the first immunization. For the second and third immunizations the protein was emulsified with Freund’s incomplete adjuvant (Thermo Fisher Scientific, USA) and the injection dose was 50 μg/mouse. After the final immunization antibody titers were analyzed via western blotting and enzyme-linked immunosorbent assays (ELISAs). Recombinant GRA7 protein was obtained as described above, and the primers used were 5’-GGGGTACCATGGCCACCGCGTCAGATGACG-3’ (forward) and primer, 5’-CGGATATCCTGGCGGGCATCCTCCCCATC-3’ (reverse), with restriction enzyme sites KpnI and EcoRV (underlined).
2.4 Preparation of different NPs
With minor modifications, PLGA NPs were prepared using the water/oil/water (w/o/w) solvent evaporation technique (Jeffery et al., 1993). Briefly, 10 mg of PLGA was dissolved in 1 mL of dichloromethane (DCM) to obtain a uniform PLGA solution (w/o), and this solution was sonicated at 30 W for 10 min (run for 3 s and pause for 3 s). Then, 10 mL of 2% (w/v) polyvinyl alcohol (PVA) was dissolved dropwise in PLGA solution, and the PLGA/PVA (w/o/w) solution was emulsified for 10 min as above. Subsequently, 0.5% PVA was added to a final volume of 20 mL with continuous stirring at 400 rpm on a magnetic stirrer at room temperature for 4 h to completely remove DCM. After centrifugation at 14,000 g for 30 min at 4°C the NPs were collected and dissolved in distilled water. The PLGA NPs with recombinant proteins (T. gondii GRA7 and T. gondii GRA12) and non-proteins were prepared together. The recombinant GRA7 and GRA12 proteins were used at a concentration of 1 mg/mL, and the supernatant was also collected to calculate the total amount of protein. All prepared NPs were stored at -80°C until use.
2.5 NPs characterization and encapsulation efficiency
A dynamic light-scattering instrument (Anton Paar Litesizer 500) and transmission electron microscopy (TEM) were used to determine the average size and distribution of NPs. A total of 5 mg of recombinant T. gondii GRA7 or T. gondii GRA12 protein was encapsulated in NPs as described above. Concentrations of unbound protein were assessed using a BCA protein assay kit (Sangon Biotech, China). Encapsulation efficiency (EE), (LC), and cumulative release (CR) were determined using the following equations:
2.6 Immunization and challenge
Seven groups of female BALB/c mice (13 mice/group) were intraperitoneally immunized with PLGA-NPs-TgGRA7 (100 μg/mouse), PLGA-NPs-TgGRA12 (100 μg/mouse), TgGRA7 emulsified in Aluminum hydroxide (100 μg/mouse) (Sigma Aldrich, Germany), TgGRA12 emulsified in Aluminum hydroxide (100 μg/mouse), Aluminum (100 μL/mouse), PLGA-NPs (100 μg/mouse), or PBS (100 μL/mouse), and an eighth group was left unimmunized. For second and third immunizations the amount of protein administered was 50 μg/mouse, and the interval between each immunization was 14 d. Five weeks after the last immunization 10 mice from each group were challenged with 1 × 103 T. gondii RH strain tachyzoites. The status of infected mice was monitored each day, and the survival time of each mouse was recorded daily.
2.7 Preparation of T. gondii lysate antigen
T. gondii lysate antigen (TLA) was prepared as described previously with minor modifications (Holec-Gasior et al., 2010). Briefly, tachyzoites were collected and washed with sterile PBS three times, then centrifuged at 1000 rpm for 10 min, and the supernatant was discarded. The precipitation was then dissolved in 3 mL PBS and sonicated for 10 min at 70 W/s on ice, then the tachyzoites were repeatedly freeze-thawed at –80°C and 37°C. Lastly, the concentration of TLA was measured using a BCA protein assay kit, and the TLA was stored at –80°C until use.
2.8 Spleen cell proliferation and cytokine assays
Three mice from each group were euthanized 5 weeks after the last immunization, and their spleens were collected. A splenocyte suspension was passed through a mesh sieve and treated with erythrocyte lysate buffer to remove red blood cells (Solarbio, Beijing, China). The splenocytes were then resuspended in DMEM medium supplemented with 10% FBS, 100 U/mL penicillin, and 100 μg/mL streptomycin. Next, the spleen cells were cultured in a 96-well plate at a concentration of 1 × 105 cells/well and incubated with 20 μg/mL TLA (Roozbehani et al., 2018), or 7.5 μg/mL concanavalin A (ConA, Sigma, USA) as a positive control, or DMEM medium as a negative control. After culturing at 37°C with 5% CO2 for 72 h, splenocyte proliferation was analyzed using cell counting kit-8 (Biosharp, Anhui, China). Average optical density (OD) at 450 nm was measured using a microplate reader. All samples were run in triplicate.
The supernatants of splenocytes stimulated with TLA were collected at 24, 48 and 96 h, and cytokines were detected using ELISA kits in accordance with the manufacturer’s instructions (MultiSciences, Hangzhou, China). The experiments were done in triplicate. Cytokine concentrations were calculated by referring to standard curves, and the data were expressed as means ± SDs for each group.
2.9 Measurement of antibody responses
Blood samples were collected from the tail vein for each group (three mice/group) 5 weeks after the last immunization, and antibody levels (IgG, IgG1, and IgG2a) were assessed via ELISAs in accordance with the manufacturer’s instructions (MultiSciences, Hangzhou, China). Briefly, 96-well plates were coated with TLA (20 μg/mL) overnight at 4°C, then washed three times with PBS containing 0.05% Tween 20 (PBST). The plates were then blocked with 1% bovine serum albumin (BSA) for 2 h at 37°C. After washing with PBST, the sera (diluted 1:100 using PBS-1%BSA) were incubated in the wells for 2 h at 37°C. After washing, horseradish peroxidase-conjugated goat anti-mouse IgG (diluted 1:1000 in PBS-1%BSA), anti-mouse IgG1 (diluted 1:1000 in PBS-1%BSA), or anti-mouse IgG2a (diluted 1:1000 in PBS-1%BSA) were added to the wells and incubated for 1 h at 37°C. After washing with PBST three times, the immune complexes were developed by incubation with tetramethylbenzidine (TMB) substrate solution for 15 min at 37°C, and the reaction was terminated by using 2 M H2SO4. Absorbance was determined at 450 nm. All tests were performed in triplicate.
2.10 Determination of parasite loads
To evaluate parasite loads, heart, liver, spleen, and lung tissues were collected from three mice in each group that exhibited clinical symptoms but did not die. Genomic DNA was extracted using a tissue DNA extraction kit (Coolaber, Beijing, China) in accordance with the manufacturer’s instructions. Parasite loads were determined via SYBR-green quantitative real-time PCR (Q-PCR) using the repeated element (RE) primers 5’-AGGGACAGAAGTCGAAGGGG-3’ (forward) and 5’-GCAGCCAAGCCGGAAACATC-3’ (reverse). Q-PCR was performed with an Applied Biosystems Inc. 7500 fluorescence quantitative PCR instrument. The protocol used was initial denaturation at 95°C for 10 min, followed by 40 cycles of denaturation at 95°C for 15 s, annealing at 60°C for 30 s, and amplification at 72°C for 30 s. Melting curve analysis was performed to estimate the specific amplification of the primers. The standard curve was obtained via amplification of the target sequence from the genomic DNA of T. gondii tachyzoites, and parasite loads were calculated based on the standard curve (Y = -2.62X + 30.206; R2 = 0.996). The data represent three independent experiments.
2.11 Statistical analysis
Statistical analyses were performed using GraphPad Prism version 5 (GraphPad Prism, San Diego, CA, USA). One-way analysis of variance was used to analyze differences in antibody and cytokine levels, the Kaplan–Meier approach was used to assess survival. The t-test was used to compare means between two groups. p< 0.05 was deemed to indicate statistical significance.
3 Results
3.1 Recombinant protein expression
GRA7 and GRA12 target products were observed as single bands of approximately 45 kDa (GRA7) and 61 kDa (GRA12) on SDS-PAGE (Figures 1A, C). In western blotting analysis recombinant TgGRA7 and TgGRA12 proteins were recognized by mouse anti-T. gondii serum polyclonal antibodies (Figures 1B, D).
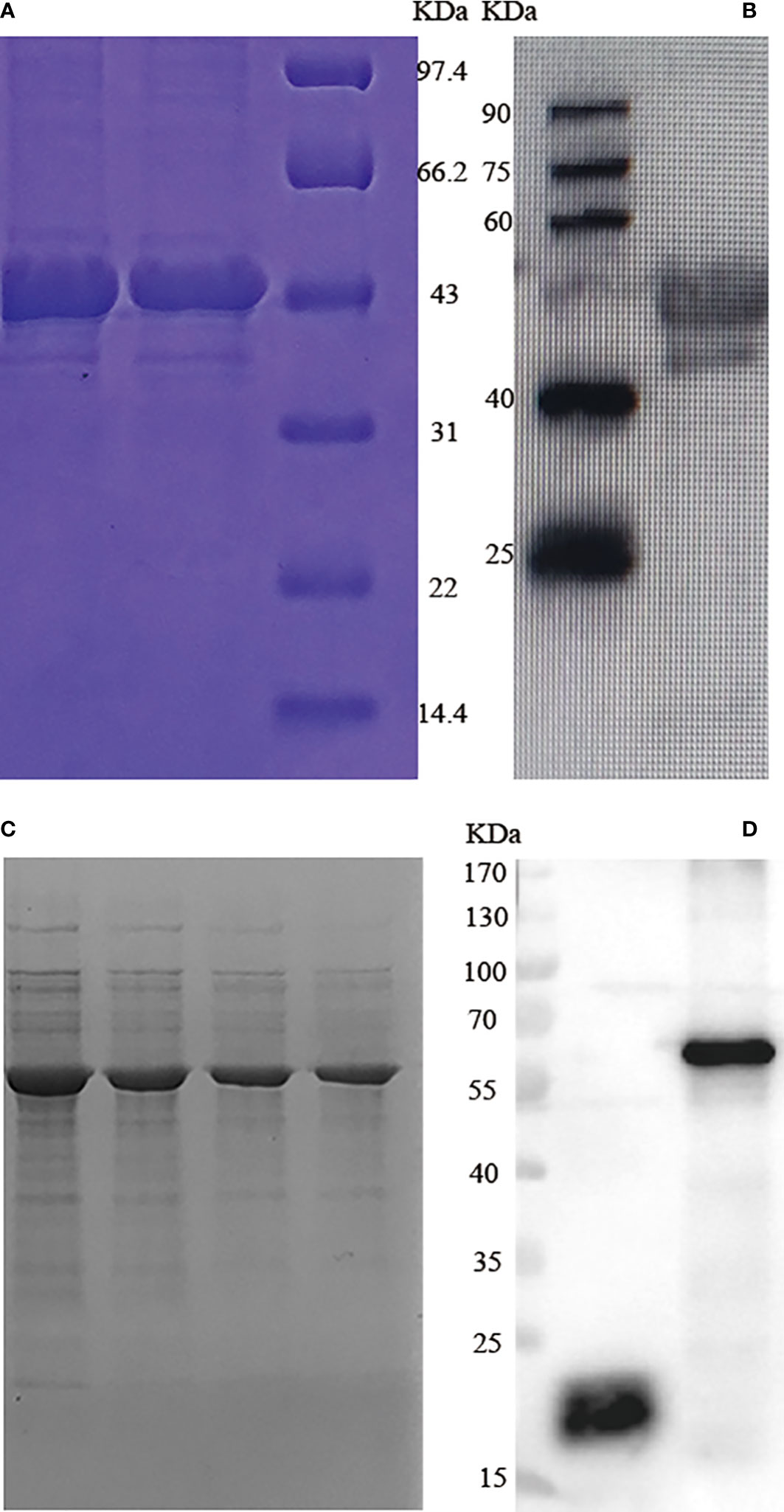
Figure 1 SDS-PAGE and western blotting analysis of T. gondii GRA7 and GRA12 recombinant proteins. (A) SDS-PAGE analysis of recombinant T. gondii GRA7 protein purified via Ni-NTA purification agarose. (B) Western blotting analysis of recombinant T. gondii GRA7-pET-32a protein. (C) SDS-PAGE analysis of recombinant T. gondii GRA12 protein purified via Ni-NTA purification agarose. (D) Western blotting analysis of recombinant T. gondii GRA12 pET-32a protein. The left band represents pET-32a, and the right band represents the recombinant T. gondii GRA12- pET-32a.
3.2 Physical characterization of NPs and release characteristics
Transmission electron microscopy (TEM) images revealed that the NPs were spherical with a smooth surface (Figures 2A, B). The mean NP diameter of PLGA+GRA12 was 106.0 ± 22.1 nm, and the mean diameter of PLGA+GRA7 was 130.8 ± 27.1 nm. The initial release of GRA7 protein from PLGA+GRA7 NPs was 6.6% ± 0.5%, and the initial release of GRA12 protein from PLGA+GRA12 NPs was 10.8% ± 3.4%. Antigen release was highest during the first week (GRA7 78.9% ± 2.3%, GRA12 88.9% ± 3.8%), then it slowly increased. In the following days the cumulative release of the recombinant proteins tended to be low, and ultimately 92.7% ± 2.1% of GRA7 and 92.9% ± 3.6% of GRA12 were released (Figure 3).
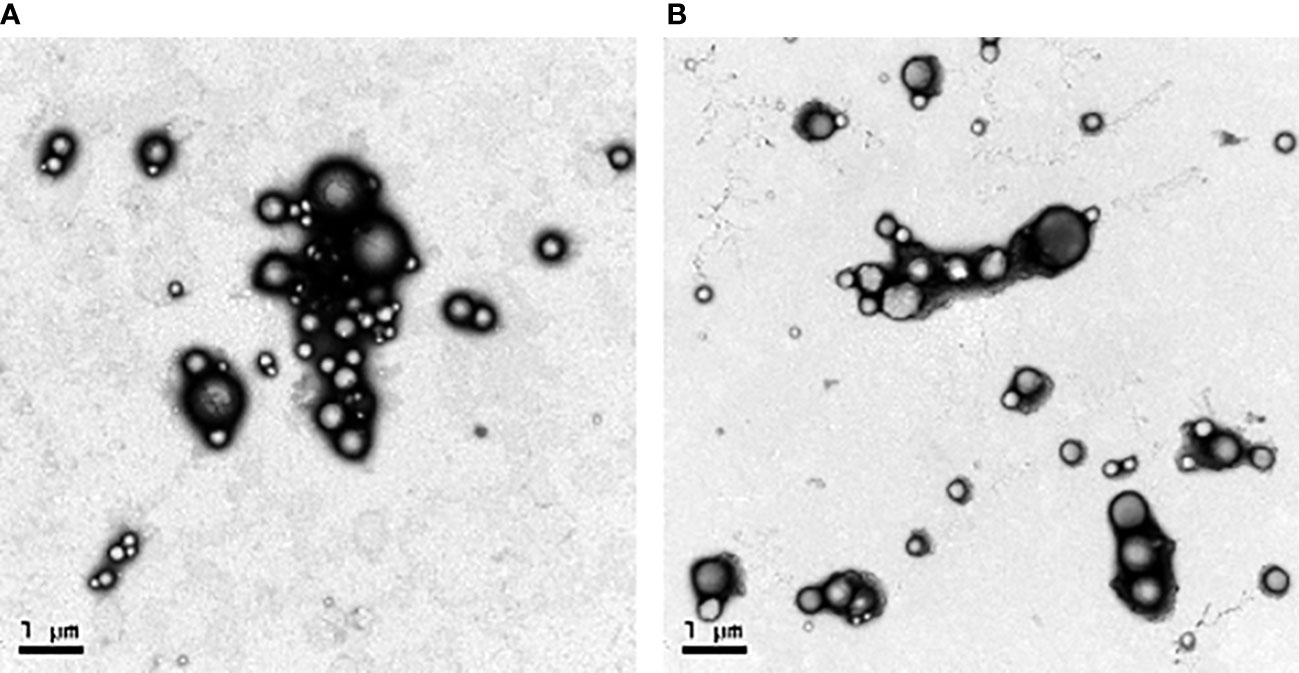
Figure 2 Sizes and distributions of recombinant T. gondii GRA7 and GRA12 proteins encapsulated in PLGA NPs as observed via TEM. (A) PLGA+GRA7 NPs (10,000x, scale bar = 1 μm.) (B) PLGA+GRA12 NPs (10,000x, scale bar = 1 μm).
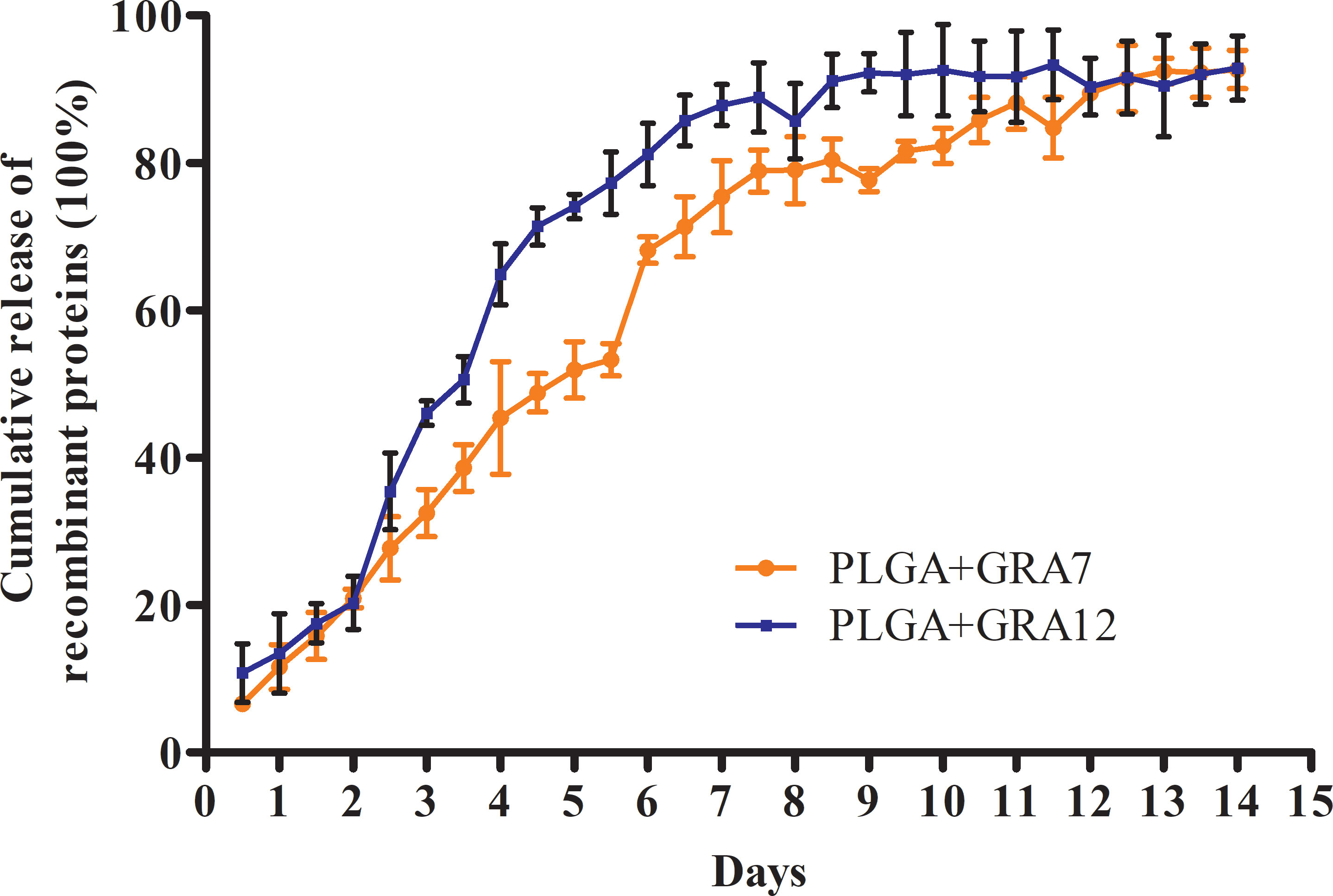
Figure 3 Release characteristics of T. gondii GRA7 and GRA12 proteins using PLGA NPs as a delivery system. Data represent mean ± SD, n = 3 experiments.
3.3 Antibody secretion in mice
Compared with the PBS, Alum alone, and PLGA alone control groups, levels of IgG were significantly higher in the in the Alum+GRA7 and PLGA+GRA7 group (p< 0.01) and the Alum+GRA12 and PLGA+GRA12 groups (p< 0.001). Levels of IgG were significantly higher in the PLGA+GRA12 group than in the Alum+GRA12 group (p< 0.01), whereas there was no significant difference between the Alum+GRA7 group and the PLGA+GRA7 group (p > 0.05) (Figure 4A). Alum+GRA12, PLGA+GRA12, Alum+GRA7, and PLGA+GRA7 induced significantly higher IgG1 and IgG2a titers than those of the PBS, Alum alone, and PLGA alone control groups (p< 0.001). All vaccine-immunized groups generated higher levels of IgG1 and IgG2a than the control groups (Figure 4B). IgG2a levels were significantly higher than IgG1 levels in the PLGA+GRA12, Alum+GRA7, and PLGA+GRA7 groups (p< 0.05) (Figure 4C). IgG2a/IgG1 ratios were significantly higher in all vaccine-immunized groups than in the three control groups. IgG2a levels were higher in the PLGA+GRA12 group than in all other vaccine-immunized groups (p< 0.05), and there was a significant predominance of IgG2a over IgG1 in the PLGA+GRA12 group (p< 0.05) (Figure 4D). The detailed data were presented in Table 1. Collectively, the results indicated that a predominant Th1 immune response was elicited, especially in the PLGA+GRA12 immunization group.
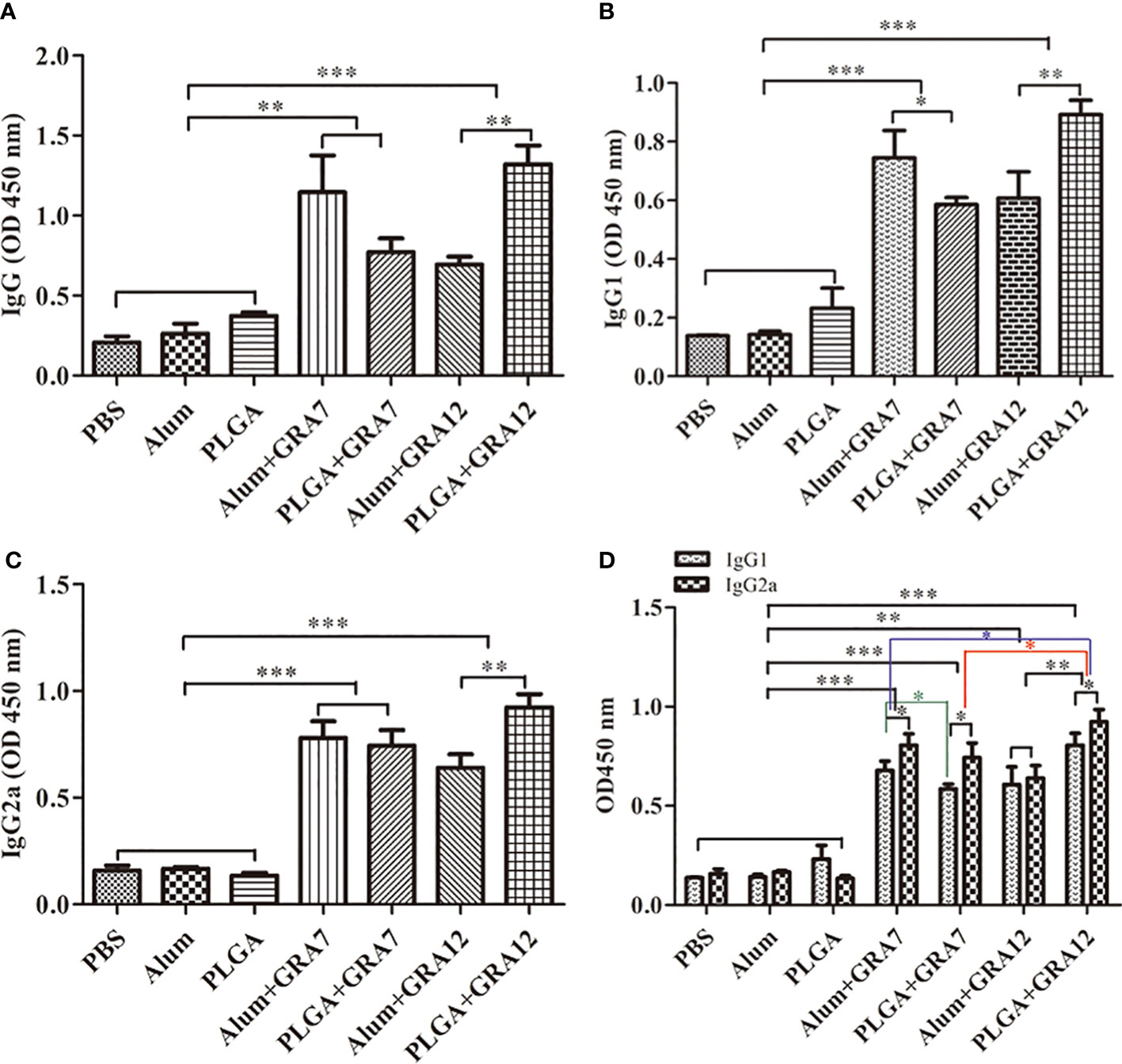
Figure 4 Specific antibody responses in mice immunized with Alum+GRA7, PLGA+GRA7, Alum+GRA12, PLGA+GRA12, PBS, Alum, and PLGA. (A) IgG secretion levels. (B) IgG1 secretion levels. (C) IgG2a secretion levels. (D) Levels of IgG1 and IgG2a. Results are represented as 450 nm optical density means ± SD. *p< 0.05, **p< 0.01, ***p< 0.001.
3.4 Lymphocyte proliferation
Splenocytes from vaccine-immunized groups exhibited significantly greater proliferation than splenocytes from the control groups (p< 0.001). Proliferation in the PLGA+GRA12 group was almost 1.54-fold higher than that in the Alum+GRA12-immunized group (p< 0.05). Proliferation in the Alum+GRA7 and PLGA+GRA7 groups was significantly greater than that in the control groups (p< 0.001), but the difference between these two experimental groups was not significant (p > 0.05) (Figure 5). The PLGA+GRA12 group exhibited the highest lymphocyte proliferation. Data were shown in Table 2.
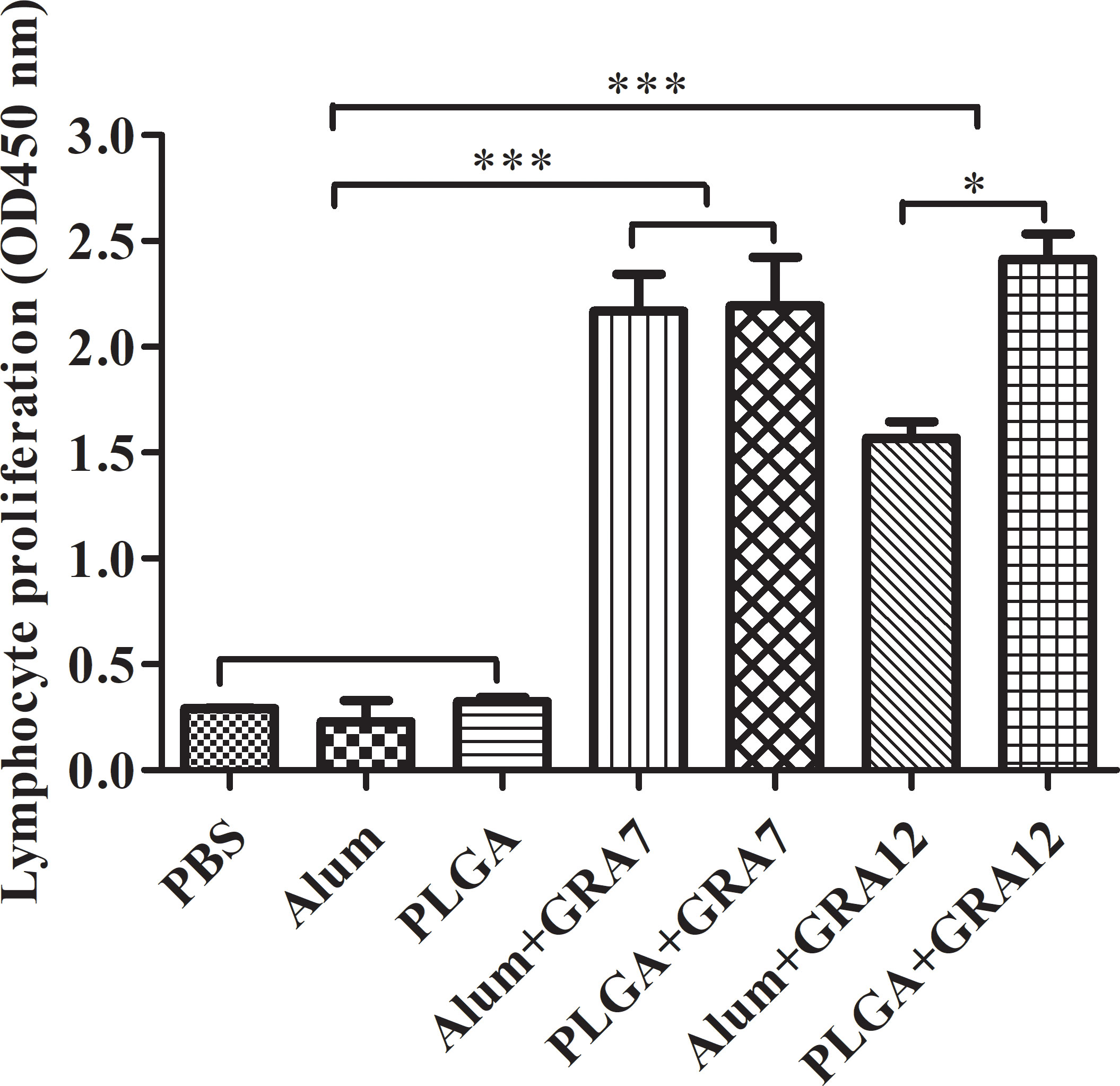
Figure 5 Lymphocyte proliferation was evaluated 5 weeks after the last immunization. Five weeks after the last immunization spleens were collected and lymphocyte proliferation responses were assessed. Results represent 450-nm optical density means ± SD. *p< 0.05, ***p< 0.001.
3.5 Splenocyte cytokine production
Compared to the control groups, splenocyte culture IFN-γ levels were significantly higher in the Alum+GRA12 and PLGA+GRA12 groups (p< 0.001), and in the Alum+GRA7 and PLGA+GRA7 groups (p< 0.01). Splenocyte culture levels of IFN-γ in the Alum+GRA12 and PLGA+GRA12 groups were higher than those in the Alum+GRA7 and PLGA+GRA7 groups (Figure 6A). The mean IFN-γ level in the PLGA+GRA12 group was 2030.50 pg/mL, which was significantly higher than that in the Alum+GRA12 group (1133.14 pg/mL) (p< 0.01). The mean IFN-γ level in the PLGA+GRA7 group was 1219.44 pg/mL and that in the Alum+GRA7 was 898.31 pg/mL, and the difference was not significant (p > 0.05). Splenocyte culture IL-4 was significantly higher in the Alum+GRA12 and PLGA+GRA12 groups compared to the control groups (p< 0.05) (Figure 6B). The Alum+GRA12 and PLGA+GRA12 group splenocytes generated higher levels of IL-10 than splenocytes from the other vaccine-immunized groups and the control groups (p< 0.001). Levels of splenocyte culture IL-10 in the Alum+GRA12-immunized group were significantly greater than those in the PLGA+GRA12 group (p< 0.05) (Figure 6C). The detailed data were shown in Table 3.
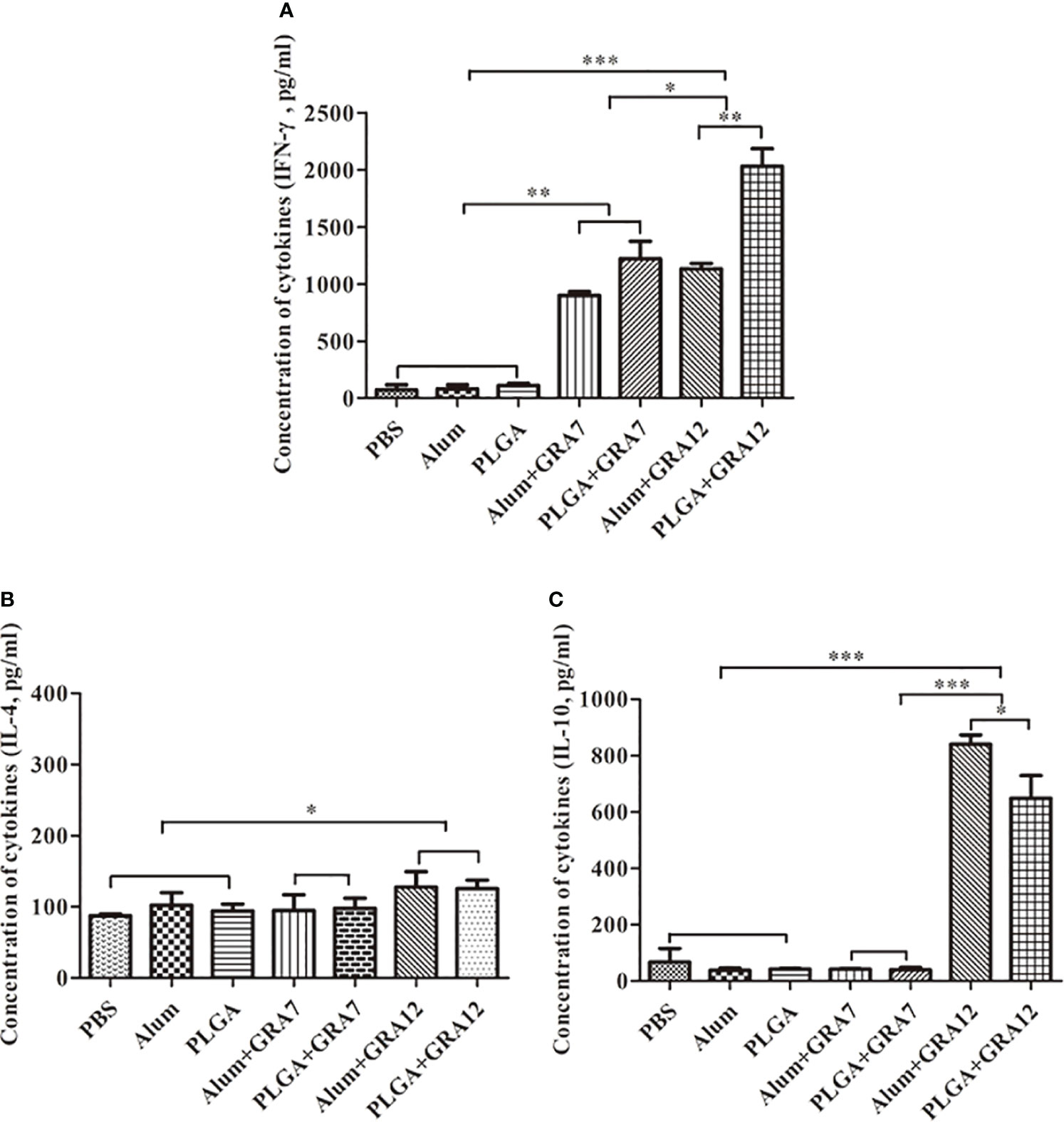
Figure 6 Splenocyte cytokine production after T. gondii lysate TLA stimulation. (A) IFN-γ. (B) IL-4. (C) IL-10. Three mice from each group were analyzed. Data represent means ± SD of three independent experiments. *p< 0.05, **p< 0.01, ***p< 0.001.
3.6 Parasite loads in mice
Compared to mice in the PBS, Alum alone, and PLGA alone control groups, mice in the Alum+GRA7, PLGA+GRA7, Alum+GRA12, and PLGA+GRA12 groups exhibited significantly less parasites in the heart (p< 0.001), spleen (p< 0.001), lungs (p< 0.01), and liver (p< 0.01) (Figures 7A-D). Compared with the Alum+GRA12-vaccinated group, in the PLGA+GRA12 group the mean parasite loads were 3.08-fold lower in the heart, 2.49-fold lower in the liver, 3.16-fold lower in the lung (all p< 0.05), and 6.30-fold lower parasite loads in the spleen (p< 0.01). There were significant no differences between the PLGA+GRA7 group and the Alum+GRA7 group (p > 0.05). The detailed data were shown in Table 4.
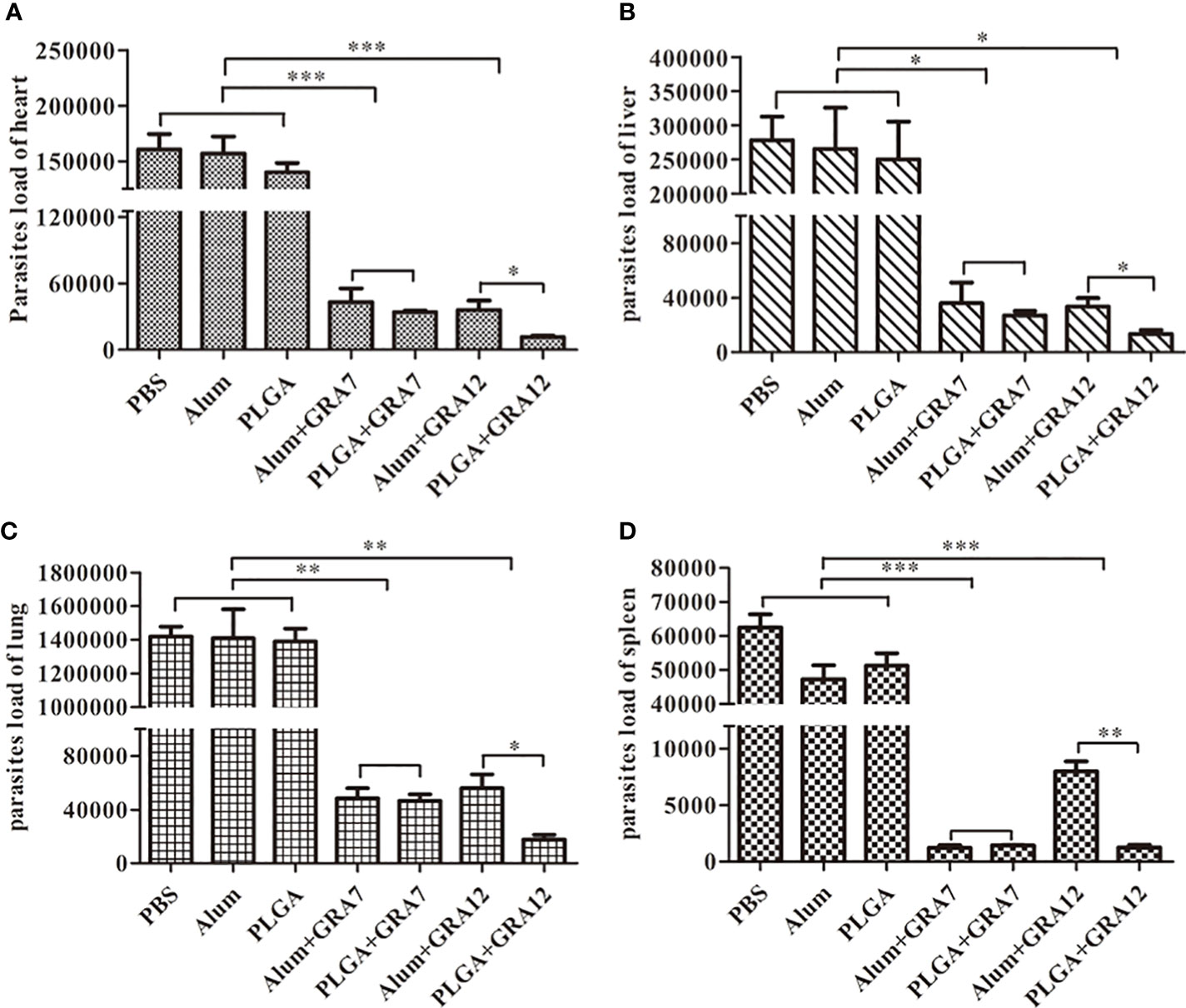
Figure 7 Parasite loads were analyzed using SYBR-green quantification real-time PCR. Heart, liver, lung, and spleen tissues were collected when symptoms of T. gondii infection became evident, and parasite loads were evaluated. (A) Parasite loads in the heart. (B) Parasite loads in the liver. (C) Parasite loads in the lung. (D) Parasites loads in the spleen. Data are presented as means ± SD of three independent experiments. *p<0.05, **p< 0.01, ***p<0.001.
3.7 Vaccine-induced protection
Mice in the PBS, Alum alone, and PLGA alone control groups began dying on day 3, and they were all dead within 4.5 days (Figure 8). Compared to the control groups, survival times were significantly longer in the Alum+GRA7, PLGA+GRA7, and Alum+GRA12 groups (p< 0.05), and they were dramatically longer in the PLGA+GRA12 group (14.5 days; p< 0.01). Survival times were significantly longer in the PLGA+GRA12 group than in the Alum+GRA12 group (mean 9.5 days) (p< 0.05). Mean survival time was longer in the PLGA+GRA7 group than in the Alum+GRA7 group, but the difference was not statistically significant.
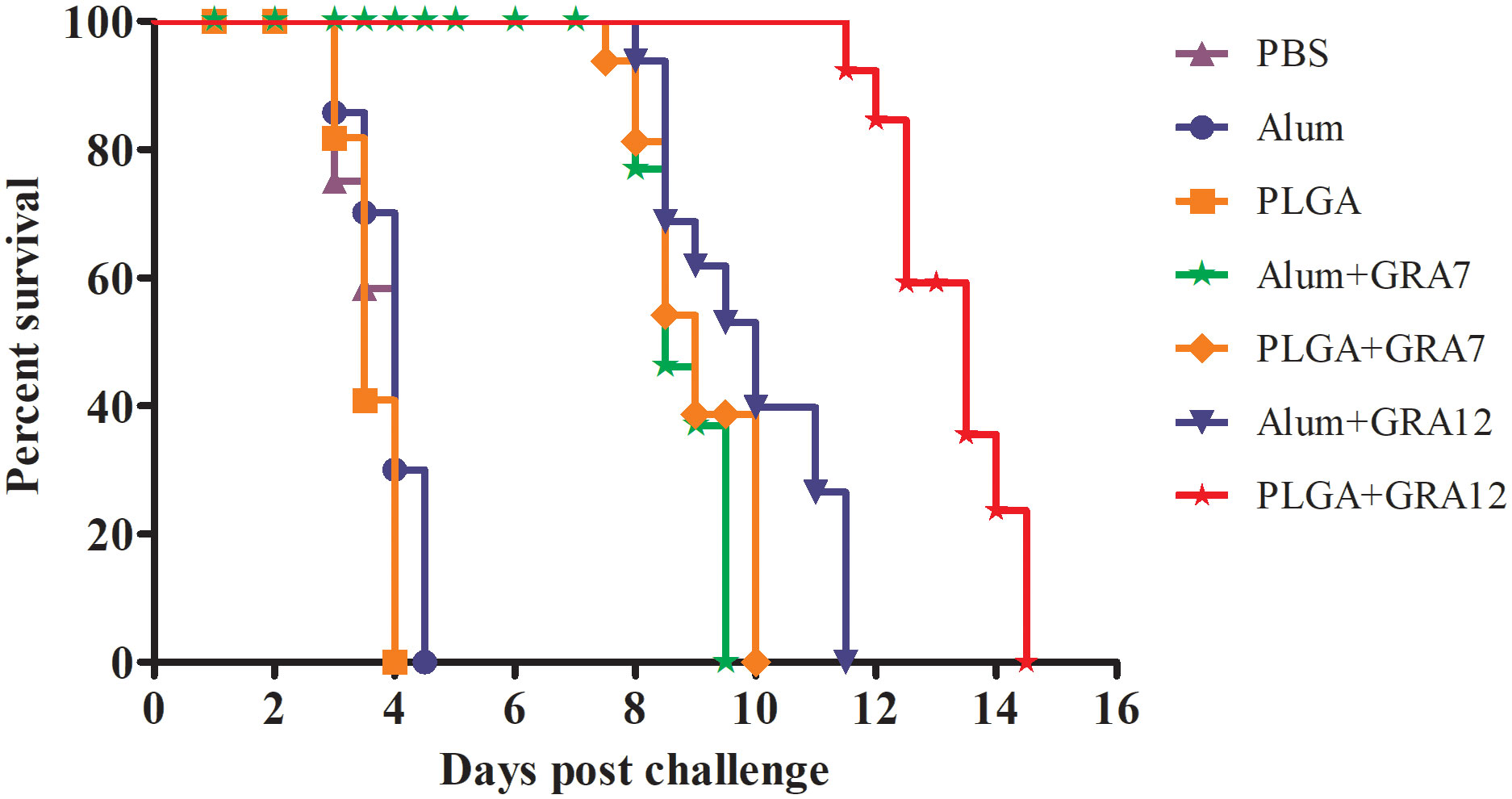
Figure 8 Survival curves of immunized BALB/c mice after T. gondii challenge. Mice were challenged with 1 × 103 T. gondii RH strain tachyzoites 5 weeks after the final immunization (n = 10 per group).
4 Discussion
Vaccines are considered the most effective tools against T. gondii infection, and recombinant immunogenic proteins have predominated in the quest to prevent toxoplasmosis. Nanocarrier delivery systems can facilitate the development of novel vaccines (Naeem et al., 2018), and overcome the disadvantage of short-lasting immune responses associated with some tradition vaccines. PLGA is currently the most widely used biodegradable material used to construct carriers of drugs (Shi et al., 2020), bacterial or viral DNA (Koerner et al., 2019; Lu et al., 2020), and proteins (Zhai et al., 2020). PLGA NPs can enhance immune response by continually releasing antigens, reduce protein degradation, and maintaining protein/drug levels at effective concentrations over a long period (Demento et al., 2011; Allahyari and Mohit, 2016). The sustained release of antigens may promote their uptake by APCs, and in the current study significantly long-lasting immune responses against T. gondii infection were induced (Lim et al., 2009).
In the present study IgG levels the Alum+GR7, PLGA+GRA7, Alum+GRA12, and PLGA+GRA12 groups were higher than those in the PBS, Alum alone, and PLGA alone control groups. Specific IgG antibody can reduce the capacity of T. gondii to adhere to and invade host cells, effectively blocking the connection between the parasite and the host (Kang et al., 2000). Sera of mice immunized with Alum+GR7, PLGA+GRA7, Alum+GRA12, and PLGA+GRA12 exhibited high concentrations of IgG2a, and a high IgG2a:IgG1 ratio was evident in the PLGA+GRA12 group. IgG2a is generally associated with Th1 immune responses, and IgG2a is reportedly an important component of immunity against T. gondii infection (Guo et al., 2018). Although mice in the GA12+PLGA group generated both higher IgG1 and IgG2a, the high IgG2a:IgG1 ratio indicated that the dominant immune response was Th1-type. In a previous study both IgG1 and IgG2a were induced in mice vaccinated with CS-SAG1-NLT, and IgG2a production predominated (Khorshidvand et al., 2022). In another study mice vaccinated with pVAX1-SIR2-CS (chitosan) exhibited a mixed Th1/Th2 immune response, suggesting that certain types of nanospheres could induce a mixed immune response (Yu et al., 2021b).
In the current study all four experimental vaccine preparations induced strong lymphoproliferative responses, but that in the PLGA+GRA12 group was significantly stronger than that in the Alum+GRA12-immunization group, and there was no significant difference between the Alum+GRA7 group and the PLGA+GRA7 group. In a previous study higher levels of splenocyte proliferation were observed in TgSORO-pVAX1/PLGA-vaccinated mice (Yu et al., 2021a). Ahmadpour et al. (2017) reported that a T. gondii vaccine coated with a nano-adjuvant (“GRA14+CaPN”) enhanced lymphocyte proliferation compared to the same vaccine without CaPN (Ahmadpour et al., 2017). In another previous study strong long-lasting lymphocyte proliferation was evident in mice vaccinated with PLG-rSAG1/2 microparticles (Chuang et al., 2017).
IFN-γ is an integral component of Th1-type immune responses, which play a vital role during the acute phase of T. gondii infection (Suzuki et al., 1988). It can activate a series of genes such as inducible nitric oxide synthase (iNOs) and inducibly expressed GTPase (IGTPs) which can inhibit the growth of T. gondii (Der et al., 1998; Taylor et al., 2000). Th2-type cytokines such as IL-4 can inhibit severe immunopathology during T. gondii infection, and IL-4 can also counterbalance the immune response by downregulating IFN-γ secretion (Harris et al., 2005; Hunter and Sibley, 2012), which is consistent with the high IFN-γ and low IL-4 observed in in the present study. IL-10 has a prominent role in the prevention of immune hyperactivity (Wilson et al., 2005), and it inhibits the activity of Th1 cells, thus maintaining immune balance by reducing inflammatory reactions during toxoplasmosis (Dupont et al., 2012). In a previous study IL-10 secretion levels were increased in animals injected with a pVAX1-SIR2/PLGA vaccine (Yu et al., 2021b), and IL-10 was observed at high levels in rTgPLP2-immunized mice (Tian et al., 2021). In that same study mice exhibited a lethal T cell-mediated response due to excessive levels of IFN-γ in the absence of IL-10 (Suzuki et al., 2000; Wille et al., 2001). In the present study IL-10 secretion from splenocytes of mice in the Alum+GRA12 and PLGA+GRA12 groups was evident, which may indicate excessive humoral immunity. Although protection against T. gondii infection mainly depends on cellular immunity, humoral immunity also plays an indispensable role (Lu et al., 2018).
T. gondii parasite loads in tissues have been detected via Q-PCR in many studies (Dadimoghaddam et al., 2014; Montazeri et al., 2016), and the method is evidently highly specific and sensitive. Fatollahzadeh et al. (2021) found that parasite loads were significantly reduced in the spleens and brains of pcGRA14+pcROP13-immunized mice (Fatollahzadeh et al., 2021). In another study parasitic burden was reduced in all tissues investigated in pcGRA14-CaPN-immunized mice (Yu et al., 2021a). In the current study parasite loads were significantly reduced in the experimental vaccination groups compared to the control groups, and parasitic burden in the PLGA+GRA12 group was significantly reduced compared to the Alum+GRA12 group. There was no significant difference between the Alum+GRA7 and PLGA+GRA7 groups. The results of the present study indicate that PLGA NPs can enhance the immune protective effects of recombinant T. gondii GRA12, but are ineffective with respect to recombinant T. gondii GRA7. Numerous studies have confirmed that NP adjuvants enhance immune responses and increase the immunogenicity of vaccines (Roozbehani et al., 2018; Hasan et al., 2021), and PLGA is biocompatible and may generate robust immunity against toxoplasmosis (Zhang et al., 2016).
Monitoring the survival rate of mice infected with T. gondii is currently considered the best way to evaluate the efficiency of candidate T. gondii vaccines (Zheng et al., 2017). In the current study, all four experimental vaccines exhibited extended survival time compared to mice in the control groups. Survival rates in the Alum+GRA7, PLGA+GRA7, and Alum+GRA12 groups were lower than that in the PLGA+GRA12 group, and significantly prolonged survival was evident in the PLGA+GRA12 group. In a previous study, mice vaccinated with rTgH2A1/PLGA exhibited a significantly increased survival time (Yu et al., 2020). In future experiments, it would be informative to evaluate the immune protection induced by PLGA+GRA12 in low-virulence T. gondii strains.
In conclusion, in the current study vaccination with T. gondii GRA12 encapsulated in PLGA NPs triggered strong humoral and cellular immune responses, reduced parasite loads, and prolonged survival time after T. gondii infection in BALB/c mice.
Data availability statement
The raw data supporting the conclusions of this article will be made available by the authors, without undue reservation.
Ethics statement
All experiments performed on animals were approved by the Animal welfare and Ethics Committee of Zhejiang Academy of Agricultural Sciences.
Author contributions
H-cS and T-yS designed the study and revised the manuscript. H-cS drafted the manuscript. H-cS and T-yS supported the study. P-mD, YF, and J-hD performed the experiments for preparation of recombinant proteins, polyclonal antibody and vaccines. R-hX and JH performed the animal experiment and immune protection analysis. MQ analyzed the data. All authors have read and agreed with the submitted version. All authors contributed to the article.
Funding
This work was supported by the National Natural Science Foundation of China (31802183, 32072883), Zhejiang Province “Sannongjiufang” Science and Technology Cooperation Project (2023SNJF059), Hangzhou Agricultural and Social Development Project (202203B19), Hangzhou Science and Technology Commissioner Project (20211122I30), and International Cooperation Projects of Zhejiang Academy of Agricultural Sciences.
Conflict of interest
The authors declare that the research was conducted in the absence of any commercial or financial relationships that could be construed as a potential conflict of interest.
Publisher’s note
All claims expressed in this article are solely those of the authors and do not necessarily represent those of their affiliated organizations, or those of the publisher, the editors and the reviewers. Any product that may be evaluated in this article, or claim that may be made by its manufacturer, is not guaranteed or endorsed by the publisher.
References
Ahmadpour, E., Sarvi, S., Hashemi Soteh, M. B., Sharif, M., Rahimi, M. T., Valadan, R., et al. (2017). Enhancing immune responses to a DNA vaccine encoding Toxoplasma gondii GRA14 by calcium phosphate nanoparticles as an adjuvant. Immunol. Lett. 185, 40–47. doi: 10.1016/j.imlet.2017.03.006
Allahyari, M., Mohit, E. (2016). Peptide/protein vaccine delivery system based on PLGA particles. Hum. Vaccin. Immunother. 12, 806–828. doi: 10.1080/21645515.2015.1102804
Allahyari, M., Mohit, E., Amiri, S., Esmaeili Rastaghi, A. R., Babaie, J., Mahdavi, M., et al. (2016). Synergistic effect of rSAG1 and rGRA2 antigens formulated in PLGA microspheres in eliciting immune protection against Toxoplasama gondii. Exp. Parasitol. 170, 236–246. doi: 10.1016/j.exppara.2016.09.008
Boughattas, S., Bergaoui, R., Essid, R., Aoun, K., Bouratbine, A. (2011). Seroprevalence of Toxoplasma gondii infection among horses in Tunisia. Parasitol. Vectors 4, 218. doi: 10.1186/1756-3305-4-218
Buxton, D., Innes, E. A. (1995). A commercial vaccine for ovine toxoplasmosis. Parasitology 110 Suppl, S11–S16. doi: 10.1017/s003118200000144x
Chereddy, K. K., Vandermeulen, G., Preat, V. (2016). PLGA based drug delivery systems: promising carriers for wound healing activity. Wound Repair. Regener. 24, 223–236. doi: 10.1111/wrr.12404
Chuang, S. C., Chung, Y. C., Yang, C. D. (2017). Protective immunity against toxoplasmosis in mice induced by single-dose immunization with rSAG1/2 protein released from poly(lactide-co-glycolide) microparticles. Parasite 24, 5. doi: 10.1051/parasite/2017004
Dadimoghaddam, Y., Daryani, A., Sharif, M., Ahmadpour, E., Hossienikhah, Z. (2014). Tissue tropism and parasite burden of Toxoplasma gondii RH strain in experimentally infected mice. Asian. Pac. J. Trop. Med. 7, 521–524. doi: 10.1016/S1995-7645(14)60087-0
Demento, S. L., Siefert, A. L., Bandyopadhyay, A., Sharp, F. A., Fahmy, T. M. (2011). Pathogen-associated molecular patterns on biomaterials: a paradigm for engineering new vaccines. Trends Biotechnol. 29, 294–306. doi: 10.1016/j.tibtech.2011.02.004
Der, S. D., Zhou, A., Williams, B. R., Silverman, R. H. (1998). Identification of genes differentially regulated by interferon alpha, beta, or gamma using oligonucleotide arrays. Proc. Natl. Acad. Sci. U. S. A. 95, 15623–15628. doi: 10.1073/pnas.95.26.15623
Dubey, J. P. (2001). Oocyst shedding by cats fed isolated bradyzoites and comparison of infectivity of bradyzoites of the VEG strain Toxoplasma gondii to cats and mice. J. Parasitol. 87, 215–219. doi: 10.1645/0022-3395(2001)087[0215:OSBCFI]2.0.CO;2
Dubey, J. P., Lindsay, D. S., Speer, C. A. (1998). Structures of Toxoplasma gondii tachyzoites, bradyzoites, and sporozoites and biology and development of tissue cysts. Clin. Microbiol. Rev. 11, 267–299. doi: 10.1128/CMR.11.2.267
Dubey, J. P., Miller, N. L., Frenkel, J. K. (1970). Toxoplasma gondii life cycle in cats. J. Am. Vet. Med. Assoc. 157, 1767–1770.
Dubremetz, J. F. (1998). Host cell invasion by Toxoplasma gondii. Trends Microbiol. 6, 27–30. doi: 10.1016/S0966-842X(97)01165-7
Dupont, C. D., Christian, D. A., Hunter, C. A. (2012). Immune response and immunopathology during toxoplasmosis. Semin. Immunopathol. 34, 793–813. doi: 10.1007/s00281-012-0339-3
Fatollahzadeh, M., Eskandarian, A., Darani, H. Y., Pagheh, A. S., Ahmadpour, E. (2021). Evaluation of Th17 immune responses of recombinant DNA vaccine encoding GRA14 and ROP13 genes against Toxoplasma gondii in BALB/c mice. Infect. Genet. Evol. 96, 105150. doi: 10.1016/j.meegid.2021.105150
Fox, B. A., Guevara, R. B., Rommereim, L. M., Falla, A., Bellini, V., Pètre, G., et al. (2019). Toxoplasma gondii parasitophorous vacuole membrane-associated dense granule proteins orchestrate chronic infection and GRA12 underpins resistance to host gamma interferon. mBio 10, e00589–e00519. doi: 10.1128/mBio.00589-19
Ghaffari, A. D., Dalimi, A., Ghaffarifar, F., Pirestani, M. (2020). Antigenic properties of dense granule antigen 12 protein using bioinformatics tools in order to improve vaccine design against Toxoplasma gondii. Clin. Exp. Vaccine Res. 9, 81–96. doi: 10.7774/cevr.2020.9.2.81
Golkar, M., Shokrgozar, M. A., Rafati, S., Musset, K., Assmar, M., Sadaie, R., et al. (2007). Evaluation of protective effect of recombinant dense granule antigens GRA2 and GRA6 formulated in monophosphoryl lipid a (MPL) adjuvant against toxoplasma chronic infection in mice. Vaccine 25, 4301–4311. doi: 10.1016/j.vaccine.2007.02.057
Guo, J., Sun, X., Yin, H., Wang, T., Li, Y., Zhou, C., et al. (2018). Chitosan microsphere used as an effective system to deliver a linked antigenic peptides vaccine protect mice against acute and chronic toxoplasmosis. Front. Cell. Infect. Microbiol. 8. doi: 10.3389/fcimb.2018.00163
Harris, D. P., Goodrich, S., Mohrs, K., Mohrs, M., Lund, F. E. (2005). Cutting edge: the development of IL-4-producing b cells (B effector 2 cells) is controlled by IL-4, IL-4 receptor alpha, and Th2 cells. J. Immunol. 175, 7103–7107. doi: 10.4049/jimmunol.175.11.7103
Hasan, T., Kawanishi, R., Akita, H., Nishikawa, Y. (2021). Toxoplasma gondii GRA15 DNA vaccine with a liposomal nanocarrier composed of an SS-cleavable and pH-activated lipid-like material induces protective immunity against toxoplasmosis in mice. Vaccines 10, 21. doi: 10.3390/vaccines10010021
Hill, D., Dubey, J. P. (2002). Toxoplasma gondii: transmission, diagnosis and prevention. Clin. Microbiol. Infect. 8, 634–640. doi: 10.1046/j.1469-0691.2002.00485.x
Hiszczyńska-Sawicka, E., Olędzka, G., Holec-Gąsior, L., Li, H., Xu, J. B., Sedcole, R., et al. (2011). Evaluation of immune responses in sheep induced by DNA immunization with genes encoding GRA1, GRA4, GRA6 and GRA7 antigens of Toxoplasma gondii. Vet. Parasitol. 177, 281–289. doi: 10.1016/j.vetpar.2010.11.047
Holec-Gasior, L., Kur, J., Hiszczyńska-Sawicka, E., Drapała, D., Dominiak-Górski, B., Pejsak, Z. (2010). Application of recombinant antigens in serodiagnosis of swine toxoplasmosis and prevalence of Toxoplasma gondii infection among pigs in Poland. Pol. J.Vet. Sci. 13, 457–464.
Hunter, C. A., Sibley, L. D. (2012). Modulation of innate immunity by Toxoplasma gondii virulence effectors. Nat. Rev. Microbiol. 10, 766–778. doi: 10.1038/nrmicro2858
Jeffery, H., Davis, S. S., O'Hagan, D. T. (1993). The preparation and characterization of poly(lactide-co-glycolide) microparticles. II. the entrapment of a model protein using a (water-in-oil)-in-water emulsion solvent evaporation technique. Pharm. Res. 10, 362–368. doi: 10.1023/a:1018980020506
Jongert, E., de Craeye, S., Dewit, J., Huygen, K. (2007). GRA7 provides protective immunity in cocktail DNA vaccines against Toxoplasma gondii. Parasite Immunol. 29, 445–453. doi: 10.1111/j.1365-3024.2007.00961.x
Jongert, E., Roberts, C. W., Gargano, N., Förster-Waldl, E., Petersen, E. (2009). Vaccines against Toxoplasma gondii: challenges and opportunities. Mem. Inst. Oswaldo. Cruz. 104, 252–266. doi: 10.1590/s0074-02762009000200019
Kang, H., Remington, J. S., Suzuki, Y. (2000). Decreased resistance of b cell-deficient mice to infection with Toxoplasma gondii despite unimpaired expression of IFN-gamma, TNF-alpha, and inducible nitric oxide synthase. J. Immunol. 164, 2629–2634. doi: 10.4049/jimmunol.164.5.2629
Khorshidvand, Z., Khosravi, A., Mahboobian, M. M., Larki-Harchegani, A., Fallah, M., Maghsood, A. H. (2022). Novel naltrexone hydrochloride nanovaccine based on chitosan nanoparticles promotes induction of Th1 and Th17 immune responses resulting in protection against Toxoplasma gondii tachyzoites in a mouse model. Int. J. Biol. Macromol. 208, 962–972. doi: 10.1016/j.ijbiomac.2022.03.146
Koerner, J., Horvath, D., Groettrup, M. (2019). Harnessing dendritic cells for poly (D,L-lactide-co-glycolide) microspheres (PLGA MS)-mediated anti-tumor therapy. Front. Immunol. 10. doi: 10.3389/fimmu.2019.00707
Konradt, C., Ueno, N., Christian, D. A., Delong, J. H., Pritchard, G. H., Herz, J., et al. (2016). Endothelial cells are a replicative niche for entry of Toxoplasma gondii to the central nervous system. Nat. Microbiol. 1, 16001. doi: 10.1038/nmicrobiol.2016.1
Konstantinovic, N., Guegan, H., Stäjner, T., Belaz, S., Robert-Gangneux, F. (2019). Treatment of toxoplasmosis: current options and future perspectives. Food. Waterborne Parasitol. 15, e00036. doi: 10.1016/j.fawpar.2019.e00036
Lim, T. Y., Poh, C. K., Wang, W. (2009). Poly (lactic-co-glycolic acid) as a controlled release delivery device. J. Mater. Sci. Mater. Med. 20, 1669–1675. doi: 10.1007/s10856-009-3727-z
Liu, Q., Li, F. C., Zhou, C. X., Zhu, X. Q. (2017). Research advances in interactions related to Toxoplasma gondii microneme proteins. Exp. Parasitol. 176, 89–98. doi: 10.1016/j.exppara.2017.03.001
Lu, Y., Wu, F., Duan, W., Mu, X., Fang, S., Lu, N., et al. (2020). Engineering a "PEG-g-PEI/DNA nanoparticle-in- PLGA microsphere" hybrid controlled release system to enhance immunogenicity of DNA vaccine. Mater. Sci. Eng. C. Mater. Biol. Appl. 106, 110294. doi: 10.1016/j.msec.2019.110294
Lu, G., Zhou, J., Zhao, Y. H., Wang, L. (2018). DNA Vaccine ROP29 from Toxoplasma gondii containing R848 enhances protective immunity in mice. Parasite Immunol. 40, e12578. doi: 10.1111/pim.12578
Montazeri, M., Ebrahimzadeh, M. A., Ahmadpour, E., Sharif, M., Sarvi, S., Daryani, A. (2016). Evaluation of propranolol effect on experimental acute and chronic toxoplasmosis using quantitative PCR. Antimicrob. Agents. Chemother. 60, 7128–7133. doi: 10.1128/AAC.01323-16
Montoya, J. G., Liesenfeld, O. (2004). Toxoplasmosis. Lancet 363, 1965–1976. doi: 10.1016/S0140-6736(04)16412-X
Naeem, H., Sana, M., Islam, S., Khan, M., Riaz, F., Zafar, Z., et al. (2018). Induction of Th1 type-oriented humoral response through intranasal immunization of mice with SAG1-Toxoplasma gondii polymeric nanospheres. Artif. Cells Nanomed. Biotechnol. 46, 1025–1034. doi: 10.1080/21691401.2018.1478421
Roozbehani, M., Falak, R., Mohammadi, M., Hemphill, A., Razmjou, E., Meamar, A. R., et al. (2018). Characterization of a multi-epitope peptide with selective MHC-binding capabilities encapsulated in PLGA nanoparticles as a novel vaccine candidate against Toxoplasma gondii infection. Vaccine 36, 6124–6132. doi: 10.1016/j.vaccine.2018.08.068
Shi, N. Q., Zhou, J., Walker, J., Li, L., Hong, J. K. Y., Olsen, K. F., et al. (2020). Microencapsulation of luteinizing hormone-releasing hormone agonist in poly (lactic-co-glycolic acid) microspheres by spray-drying. J. Control Release 321, 756–772. doi: 10.1016/j.jconrel.2020.01.023
Suzuki, Y., Orellana, M. A., Schreiber, R. D., Remington, J. S. (1988). Interferon-gamma: the major mediator of resistance against Toxoplasma gondii. Science 240, 516–518. doi: 10.1126/science.3128869
Suzuki, Y., Sher, A., Yap, G., Park, D., Neyer, L. E., Liesenfeld, O., et al. (2000). IL-10 is required for prevention of necrosis in the small intestine and mortality in both genetically resistant BALB/c and susceptible C57BL/6 mice following peroral infection with Toxoplasma gondii. J. Immunol. 164, 5375–5382. doi: 10.4049/jimmunol.164.10.5375
Taylor, G. A., Collazo, C. M., Yap, G. S., Nguyen, K., Gregorio, T. A., Taylor, L. S., et al. (2000). Pathogen-specific loss of host resistance in mice lacking the IFN-gamma-inducible gene IGTP. Proc. Natl. Acad. Sci. U. S. A. 97, 751–755. doi: 10.1073/pnas.97.2.751
Tenter, A. M., Heckeroth, A. R., Weiss, L. M. (2000). Toxoplasma gondii: from animals to humans. Int. J. Parasitol. 30, 1217–1258. doi: 10.1016/s0020-7519(00)00124-7
Tian, X., Sun, H., Wang, M., Wan, G., Xie, T., Mei, X., et al. (2021). A novel vaccine candidate: recombinant Toxoplasma gondii perforin-like protein 2 stimulates partial protective immunity against toxoplasmosis. Front. Vet. Sci. 8. doi: 10.3389/fvets.2021.802250
Wille, U., Villegas, E. N., Striepen, B., Roos, D. S., Hunter, C. A. (2001). Interleukin-10 does not contribute to the pathogenesis of a virulent strain of Toxoplasma gondii. Parasite Immunol. 23, 291–296. doi: 10.1046/j.1365-3024.2001.00389.x
Wilson, E. H., Wille-Reece, U., Dzierszinski, F., Hunter, C. A. (2005). A critical role for IL-10 in limiting inflammation during toxoplasmic encephalitis. J. Neuroimmunol. 165, 63–74. doi: 10.1016/j.jneuroim.2005.04.018
Yu, Z., Cao, W., Gao, X., Aleem, M. T., Liu, J., Luo, J., et al. (2021a). With chitosan and PLGA as the delivery vehicle, Toxoplasma gondii oxidoreductase-based DNA vaccines decrease parasite burdens in mice. Front. Immunol. 12. doi: 10.3389/fimmu.2021.726615
Yu, Z., Lu, Y., Cao, W., Aleem, M. T., Liu, J., Luo, J., et al. (2021b). Nano DNA vaccine encoding Toxoplasma gondii histone deacetylase SIR2 enhanced protective immunity in mice. Pharmaceutics 13, 1582. doi: 10.3390/pharmaceutics13101582
Yu, Z., Zhou, T., Luo, Y., Dong, L., Li, C., Liu, J., et al. (2020). Modulation effects of Toxoplasma gondii histone H2A1 on murine macrophages and encapsulation with polymer as a vaccine candidate. Vaccines 8, 731. doi: 10.3390/vaccines8040731
Zhai, J., Wang, Y. E., Zhou, X., Ma, Y., Guan, S. (2020). Long-term sustained release poly(lactic-co-glycolic acid) microspheres of asenapine maleate with improved bioavailability for chronic neuropsychiatric diseases. Drug Deliv. 27, 1283–1291. doi: 10.1080/10717544.2020.1815896
Zhang, N. Z., Chen, J., Wang, M., Petersen, E., Zhu, X. Q. (2013). Vaccines against Toxoplasma gondii: new developments and perspectives. Expert Rev. Vaccines 12, 1287–1299. doi: 10.1586/14760584.2013.844652
Zhang, N. Z., Xu, Y., Wang, M., Chen, J., Huang, S. Y., Gao, Q., et al. (2016). Vaccination with Toxoplasma gondii calcium-dependent protein kinase 6 and rhoptry protein 18 encapsulated in poly(lactide-co-glycolide) microspheres induces long-term protective immunity in mice. BMC. Infect. Dis. 16, 168. doi: 10.1186/s12879-016-1496-0
Zheng, B., Ding, J., Chen, X., Yu, H., Lou, D., Tong, Q., et al. (2017). Immuno-efficacy of a T. gondii secreted protein with an altered thrombospondin repeat (TgSPATR) as a novel DNA vaccine candidate against acute toxoplasmosis in BALB/c mice. Front. Microbiol. 8. doi: 10.3389/fmicb.2017.00216
Keywords: GRA7, GRA12, nanoparticles, PLGA, Toxoplasma gondii, vaccine
Citation: Sun H-c, Deng P-m, Fu Y, Deng J-h, Xie R-h, Huang J, Qi M and Shi T-y (2023) Protective efficacy of Toxoplasma gondii GRA12 or GRA7 recombinant proteins encapsulated in PLGA nanoparticles against acute Toxoplasma gondii infection in mice. Front. Cell. Infect. Microbiol. 13:1209755. doi: 10.3389/fcimb.2023.1209755
Received: 21 April 2023; Accepted: 26 June 2023;
Published: 12 July 2023.
Edited by:
Fatemeh Ghaffarifar, Tarbiat Modares University, IranReviewed by:
Lixin Xu, Nanjing Agricultural University, ChinaZohreh Sharifi, High Institute for Research and Education in Transfusion Medicine, Iran
Masoud Foroutan, Abadan University of Medical Sciences, Iran
Copyright © 2023 Sun, Deng, Fu, Deng, Xie, Huang, Qi and Shi. This is an open-access article distributed under the terms of the Creative Commons Attribution License (CC BY). The use, distribution or reproduction in other forums is permitted, provided the original author(s) and the copyright owner(s) are credited and that the original publication in this journal is cited, in accordance with accepted academic practice. No use, distribution or reproduction is permitted which does not comply with these terms.
*Correspondence: Tuan-yuan Shi, lstone2008@126.com; Meng Qi, qimengdz@163.com