Second booster dose improves antibody neutralization against BA.1, BA.5 and BQ.1.1 in individuals previously immunized with CoronaVac plus BNT162B2 booster protocol
- 1Laboratório de Pesquisas em Virologia (LPV), Faculdade de Medicina de São José do Rio Preto (FAMERP), São José do Rio Preto, Brazil
- 2Diagnosis and Therapy of Infectious Diseases and Cancer, Oswaldo Cruz Foundation (Fiocruz), Belo Horizonte, Brazil
- 3Laboratório de Imunologia de Doenças Virais, Instituto Rene Rachou - Fundação Oswaldo Cruz, Belo Horizonte, Brazil
- 4Laboratório de Imunologia Celular e Molecular, instituto Rene Rachou - Fundação Oswaldo Cruz, Belo Horizonte, Brazil
- 5Laboratório de Estudos Genômicos, Departamento de Biologia, Instituto de Biociências Letras e Ciências Exatas (IBILCE), Universidade Estadual Paulista (Unesp), São José do Rio Preto, Brazil
- 6Department of Infectious Diseases, College of Veterinary Medicine, University of Georgia, Athens, GA, United States
- 7Hospital de Base, São José do Rio Preto, Brazil
- 8Department of Pathology, University of Texas Medical Branch, Galveston, TX, United States
Introduction: SARS-CoV-2 vaccines production and distribution enabled the return to normalcy worldwide, but it was not fast enough to avoid the emergence of variants capable of evading immune response induced by prior infections and vaccination. This study evaluated, against Omicron sublineages BA.1, BA.5 and BQ.1.1, the antibody response of a cohort vaccinated with a two doses CoronaVac protocol and followed by two heterologous booster doses.
Methods: To assess vaccination effectiveness, serum samples were collected from 160 individuals, in 3 different time points (9, 12 and 18 months after CoronaVac protocol). For each time point, individuals were divided into 3 subgroups, based on the number of additional doses received (No booster, 1 booster and 2 boosters), and a viral microneutralization assay was performed to evaluate neutralization titers and seroconvertion rate.
Results: The findings presented here show that, despite the first booster, at 9m time point, improved neutralization level against omicron ancestor BA.1 (133.1 to 663.3), this trend was significantly lower for BQ.1.1 and BA.5 (132.4 to 199.1, 63.2 to 100.2, respectively). However, at 18m time point, the administration of a second booster dose considerably improved the antibody neutralization, and this was observed not only against BA.1 (2361.5), but also against subvariants BQ.1.1 (726.1) and BA.5 (659.1). Additionally, our data showed that, after first booster, seroconvertion rate for BA.5 decayed over time (93.3% at 12m to 68.4% at 18m), but after the second booster, seroconvertion was completely recovered (95% at 18m).
Discussion: Our study reinforces the concerns about immunity evasion of the SARS-CoV-2 omicron subvariants, where BA.5 and BQ.1.1 were less neutralized by vaccine induced antibodies than BA.1. On the other hand, the administration of a second booster significantly enhanced antibody neutralization capacity against these subvariants. It is likely that, as new SARS-CoV-2 subvariants continue to emerge, additional immunizations will be needed over time.
1 Introduction
The COVID-19 pandemic has highlighted the necessity of developing fast and effective control measures to avoid viral dispersion, disease severity, and health system collapse (Martins et al., 2023; Thakkar et al., 2023). These control procedures included varied approaches, from social distancing to the development of antivirals and vaccines for a long-term protection strategy (Golpour-Hamedani et al., 2023; Thakkar et al., 2023). In this scenario, many pharmacological interventions were proposed to improve immune coverage against SARS-CoV-2, resulting in different vaccines, with different mechanisms to induce immune response, being approved to the population (Zhou et al., 2022). This was a remarkable moment in the science history, since many efforts have been made to test and approve these vaccines in record time (Bravo et al., 2022; Falsey et al., 2021; Palacios et al., 2020; Sadoff et al., 2021).
Vaccination is the main process to provide mass immunity protection against infectious diseases, including viral infections, using safe mechanisms and approaches (Moradpour et al., 2023). The worldwide vaccination against SARS-CoV-2 played a crucial role on the control of the disease, reducing the number of cases, hospitalizations and deaths (Haas et al., 2022; Martinez-Baz et al., 2024; Steele et al., 2022). However, the process to produce and distribute the vaccines globally could not follow the rapid viral dispersion and evolution (Kupferschmidt, 2020; Lopez-Cortes et al., 2022). In fact, the delayed and gradual vaccination, associated with the high transmissibility and high mutation rate of the virus, contributed to the emergence and adaptation of SARS-CoV-2 variants capable to evade immune response induced by vaccination or prior infections (Mathieu et al., 2021; Rzymski et al., 2021).
Most of COVID-19 vaccines use Spike protein as the target of immunization action. The Spike protein is the viral envelope protein recognized by cell receptors during viral adsorption and entry steps, and this recognition is mainly mediated by the Receptor Binding Domain (RBD) present in the Spike (Shang et al., 2020). However, some mutations, especially those in the RBD, are capable to confer a lower affinity between the viral protein and antibodies induced by vaccination, resulting in a neutralization decrease (Martin et al., 2021). The majority of SARS-CoV-2 variants of concern (VOCs), responsible for the highest infections peak during the pandemic, present mutations in this region, such as Delta and Omicron, and many studies have already demonstrated that these variants are less neutralized by serological immune response induced by vaccination (CDC, 2021; Hoffmann et al., 2022; Planas et al., 2021; Planas et al., 2022).
Several strategies have been developed to avoid this immune response evasion. Initially, one approach involved administering a supplementary dose of the same vaccine to those who had received complete vaccination according to any SARS-CoV-2 vaccine’s primary protocol. In general, this procedure presented an enhancement on the protection against these VOCs, but in a small proportion (Furukawa et al., 2022; Schultz et al., 2022; Wen et al., 2023). Since different immunization mechanisms have been approved (inactivated virus, attenuated viral vector, viral subunit and mRNA), an alternative to improve the immune response induction is the administration of a heterologous booster, using a vaccine different from the one used as the primary protocol, where the immune system could be stimulated in different ways, generating a more complete immune response (Hillus et al., 2021; Atmar et al., 2022). The results were widely positive, showing that the heterologous booster, independently of the immunization mechanisms, indeed presented a higher antibody and cellular response enhancement when compared to the homologous dose approach, especially against omicron (Perez-Then et al., 2022; Yoo et al., 2023).
The improved serological response, generated by the booster dose, showed to be an important tool to oppose and decelerate SARS-CoV-2 evolution and adaptation by reducing viral spread (Theparod et al., 2023). Even though, subvariants of omicron continued to emerge (Callaway, 2022; Callaway, 2023; Tegally et al., 2022; Wang and Cheng, 2022), and some concerns were raised regarding durability of protection and coverage against these new subvariants (Willett et al., 2024). Studies have shown that, even after a booster dose, humoral response tends to decay over time (Ozbay Kurt et al., 2022; Favresse et al., 2023). In addition, the scientific literature already exposed the potential evasion of these subvariants from immune response induced by vaccination and prior infections (Iketani et al., 2022; Wang Q. et al., 2022; Wang et al., 2023). The fact is that, as long as new subvariants continue to emerge, new immunization approaches will likely be needed, such as additional booster from time to time and updated vaccine technologies.
In Brazil, the immunization program started in January 2021 with a primary vaccination protocol with the virus inactivated vaccine CoronaVac, the first approved by the national regulatory agency (ANVISA, 2021a; Wu et al., 2021; Zhang et al., 2021). The course of the pandemic led to the approval of a first heterologous booster dose with the mRNA vaccine BNT162b2 during the huge omicron infection wave that started at the end of 2021 (ANVISA, 2021b; Lamarca et al., 2023). After this infection peak, a second booster dose with BNT162b2 or viral vector vaccines (ChAdOx1-S or Ad26.CoV2.S) was available to avoid immune escape and another wave of cases, but the adherence was not high as expected by the Brazilian health ministry (while more than 167 million people were fully vaccinated with the primary protocol, only 43 million people received the first and second booster doses) (accessed on January 14th, 2024) (Brasil, 2023). At the time, the panorama of omicron lineages was constantly changing, with many subvariants being introduced, highlighting BA.5 and BQ.1.1, the most frequent in the country at that moment (de Menezes et al., 2023; de Sousa et al., 2023).
In this scenario of many doubts, our study aimed to investigate the impact of the first and second booster doses, in a cohort vaccinated with CoronaVac primary protocol, against the omicron subvariants (BA.1, BA.5 and BQ.1.1) circulating in the country during 2022.
2 Methods
2.1 Cohort and vaccination groups
In order to analyze the induction of neutralizing antibodies against different sublineages of SARS-CoV-2 Omicron variant, serum samples were collected from individuals that received the primary protocol and were subsequently submitted to the booster doses over time. Samples were collected in three different time points after the CoronaVac initial protocol: 9 months (9m); 12 months (12m); and 18 months (18m). To assess the effectiveness of the booster doses, individuals were divided into three subgroups based on whether they had not received any additional doses (No booster), had received one additional dose (1 booster), or had received two additional doses (2 boosters), and all were monitored across the three time points (Figure 1).
For the first booster dose, the mRNA vaccine BNT162b2 was administered exclusively to the participants. Regarding the second booster dose, it was selected by the competent authorities the use of three different vaccines, BNT162b2 and the attenuated adenoviral vector vaccines Ad26.COV2.S and ChAdOx1-S. It is important to emphasize that bivalent vaccines were not used for this additional dose, only the first generation of vaccines were administered, and the choice of the vaccine was determined under availability. All the participants who were submitted to the booster doses were vaccinated at the same time, independent of the time-point group under analysis, which allows a fair comparison between the groups, subgroups and Omicron sublineages.
All individuals consented to be part of the study, which was approved by the institutional review board (IRB) of the Ethics Committee of the Oswaldo Cruz Foundation, under the protocol number CAAE 42898621.9.0000.5091.
2.2 Cell culture and SARS-CoV-2 omicron sublineages
For the in vitro tests, Vero cells (ATCC CCL-81) were cultivated in DMEM supplemented with 10% fetal bovine serum, 100 U/ml of penicillin and 100 µg/ml of streptomycin. Cells were maintained in a water-jacked incubator, at 37°C and 5% CO2.
To perform a comparison analysis on neutralization capacity, three SARS-CoV-2 Omicron sublineages were used in this study, the first Omicron variant introduced and isolated in Brazil, from sublineage BA.1, and two of the most recent sublineages that dominated the circulation in Brazil between 2022 and 2023, BQ.1.1 and BA.5. Sublineages BA.1 (HIAE –W.A) and BQ.1.1 (EPI_ISL_18277185) were isolated and kindly donated by Dr. Edison Durigon, from Universidade de São Paulo (USP), while BA.5 (EPI_ISL_18277186) was isolated in this study from a clinical sample. In this case, 300 µl of saline buffer from a nasopharyngeal swab sample was inoculated in Vero cells, at a confluence of 95%. Cells were incubated for 1h, for virus adsorption, and after this period, DMEM supplemented with 2% fetal bovine serum, 100 U/ml of penicillin and 100 µg/ml of streptomycin was added, followed by an incubation of 72h at 37°C. At this point, supernatant was collected and stored in a -80°C freezer until the neutralization tests. Before neutralization, virus was titrated by TCID50 (median tissue culture infectious dose), a method used do define the viral concentration necessary to infect 50% of the cultured cells, as previously described (Pastorino et al., 2020).
2.3 Viral microneutralization assay (VNT50)
The neutralization capacity of each sample, for each Omicron sublineage, was assessed independently by a viral microneutralization assay, as previously described (Campos et al., 2022). A total of 8 serum concentrations for each sample were tested, from 1:20 to 1:2560. After sera dilution, 50 TCID50/ml of virus was added to each well and incubated for 1h, at 37°C. After incubation, media from the cell plates was replaced by the mix containing virus and diluted sera, with a subsequent incubation at 37°C for 72h. Cell plates were fixed with 10% formaldehyde after incubation and stained with crystal violet. The absence of cytopathic effect is a result of viral neutralization, and the neutralization capacity was determined by the reciprocal dilution titer capable of inhibiting 50% of cytopathic effect in all replicates (VNT50).
The VNT50 calculation was performed using the Spearman-Karber algorithm (Spearman, 1908; Kärber, 1931). Seroconvertion, defined as the production and detection of neutralizing antibodies after vaccination with the primary protocol and the booster doses, was considered for all samples that presented VNT50 value over the cutoff of 20. This cutoff was defined according to the literature to maintain a stricter criterion, with higher specificity and avoiding false seroconvertion results (Favresse et al., 2021; Souza et al., 2021).
2.4 Statistical analysis
In order to compare the neutralization response of each omicron sublineage, in each time point and inside the vaccination groups, a two-way ANOVA was performed to evaluate the occurrence of any significant variance among the tested groups. After the variance analysis, the data from each time point was submitted to a Bonferroni’s multiple comparisons test, comparing to each other the VNT50 means of each sublineage, inside each vaccination group. For all analyses, the software GraphPad Prism 8 was used, and was considered statistically significant when the two-sided P value was lower than 0.05.
3 Results
3.1 Cohort samples collection
To evaluate the induction of neutralizing antibodies, individuals vaccinated with the two doses primary protocol of CoronaVac joined the study. Serum samples were collected 9 months (9m), 12 months (12m) and 18 months (18m) after the CoronaVac primary protocol. Inside each time point, samples were divided into three different subgroups according to vaccination schemes: no booster, 1 booster and 2 boosters, except for the 9m group, where the second booster was not available yet.
In total, 160 individuals were included in this study. For the 9m group, 41 samples were collected (15 for no booster; 26 for 1 booster). Regard the 12m group, 69 samples were obtained (20 for no booster; 30 for 1 booster; and 19 for 2 boosters); and for the 18m collection point, 50 samples were included (11 for no booster; 19 for 1 booster; and 20 for 2 boosters). The main characteristics of the cohort can be found in Table 1.
3.2 Neutralization levels of BA.1, BA.5 and BQ.1.1 over time, with and without booster doses
The viral microneutralization assay allowed the evaluation of two parameters: the seroconvertion rates and the neutralization mean titers. The seroconvertion rates were determined by the number of individuals that presented a VNT50 value over the cutoff, and were represented by percentages. The neutralization mean titers were determined by the VNT50 mean of each group of samples tested against the omicron sublineages.
At the 9m time point, only the first booster was approved, and marked exactly one month after the administration of this additional dose. Thus, only two subgroups were evaluated at this moment: No booster and 1 booster. The findings here presented highlight that the first booster dose was capable to improve neutralization levels against all omicron sublineages. The VNT50 mean against BA.1 increased almost 5-fold, enhancing from 133.1 to 663.3. When compared to the neutralization improvement for BQ.1.1 and BA.5 (132.4 to 199.1 and 63.2 to 100.2, respectively), this two sublineages presented a significantly lower antibody neutralization induced by the first booster dose, when compared to BA.1 (p-values of 0.0042 and 0.0004, respectively). For seroconvertion rates, an improvement was observed for all sublineages after the booster dose (33.3% to 88.4%, 80.7% and 69.2% for BA.1, BQ.1.1 and BA.5, respectively) (Figure 2A).
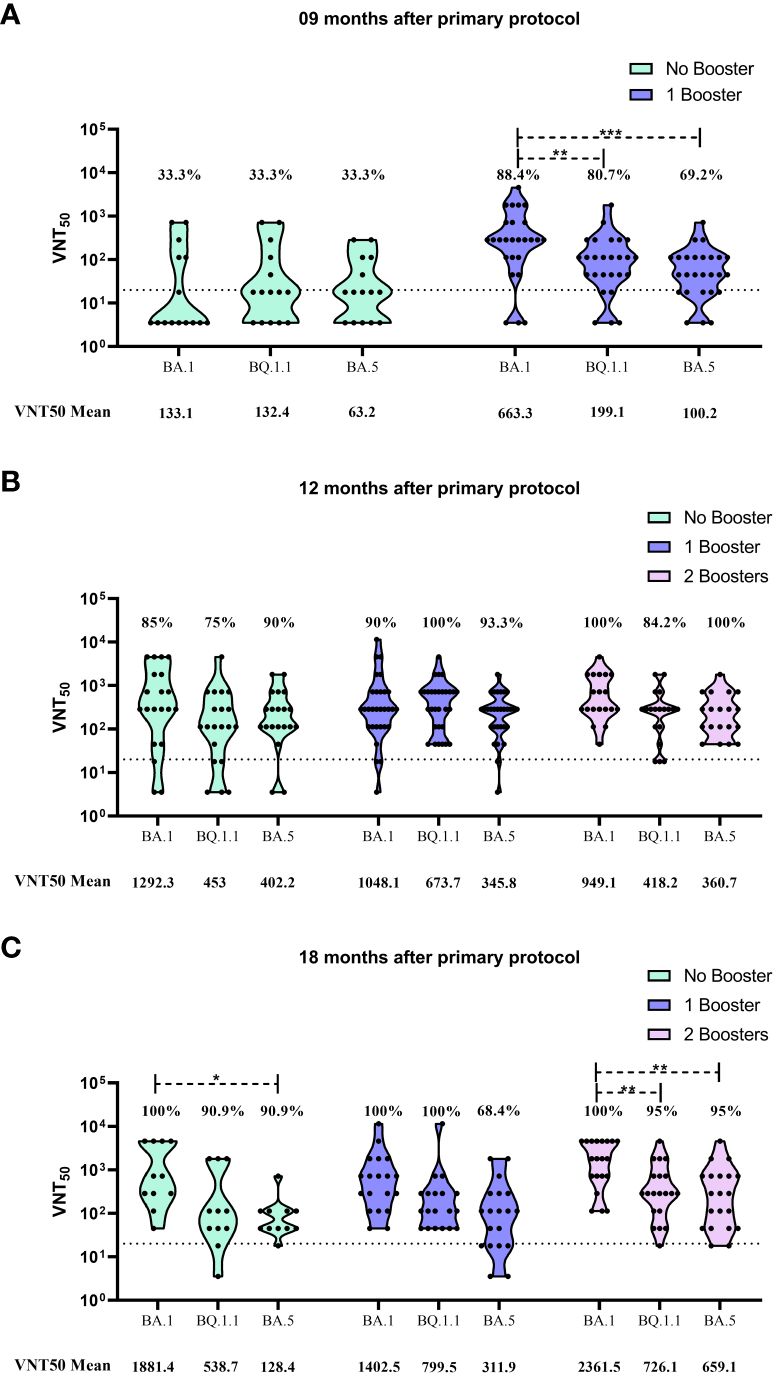
Figure 2 Viral microneutralization assay against omicron sublineages to evaluate neutralization titers (VNT50) and seroconvertion rate. (A) VNT50 for samples collected 9 months after CoronaVac 2 doses protocol. (B) VNT50 for samples collected 12 months after CoronaVac 2 doses protocol; (C) VNT50 for samples collected 18 months after CoronaVac 2 doses protocol. Vaccination subgroups are represented in green (No booster), blue (1 booster) and pink (2 boosters). VNT50 means, for each group, are highlighted under the graphs. Dashed lines represent the seroconvertion dilution cutoff (1:20), while seroconvertion rates are defined as percentages. The significance lines represent the differences among the mean neutralization titers of the groups. It was considered p-values lower than 0.05 for the significance. One asterisk (*) = p-value < 0.05; two asterisks (**) = p-value < 0.01; and three asterisks (***) = p-value < 0.001.
The 12m collection time point marked the moment which the second booster was available and 4 months after the administration of the first booster. The data showed that the groups that received 1 or 2 boosters presented higher seroconvertion rates than those individuals that received only the CoronaVac primary vaccination. However, no difference was observed between the 1 booster and 2 booster groups at that moment. Regarding the neutralization titers, there was no difference when comparing the VNT50 mean values of each sublineage among the vaccination subgroups, but as observed for the 9m group, neutralization titers for BA.1 was higher than BQ.1.1 and BA.5 (Figure 2B).
When serum samples were collected at the 18m time point, 6 months had already passed since the second booster dose was applied, and 10 months after the first booster administration. The neutralization results showed that no significantly difference was observed in the seroconvertion rates among the vaccination subgroups. For all the tested sublineages, the number of seropositive individuals was similar independently of receiving booster doses or not (Figure 2C). An important observation is that, for BA.5, seroconvertion rate for the 1 booster subgroup decayed over time (93.3% at 12m to 68.4% at 18m). However, the second booster was capable to recover seroconvertion rate for BA.5, reaching 95% (Figures 2B, C).
On the other hand, when evaluating the neutralization titers, some significant differences were detected. In the no booster subgroup, the VNT50 mean for BA.1 was significantly higher than the observed for BA.5 (1881.4 and 128.4, respectively, with p-value of 0.0497). When observing the values for the 2 boosters group, it is noticeable that the neutralization levels were considerably improved for all sublineages when compared to the other vaccination subgroups (2361.5 for BA.1, 726.1 for BQ.1.1 and 659.1 for BA.5) (Figure 2C).
Despite this general improvement, the induction of neutralizing antibodies by the second booster was significantly higher for BA.1 when compared to the other omicron sublineages, being 3.25 and 3.58 fold higher than BQ.1.1 (p-value of 0.0099) and BA.5 (p-value of 0.0068), respectively (Figure 2C).
4 Discussion
The approval of the first SARS-CoV-2 vaccines, in the beginning of 2021, allowed the gradual return to normalcy worldwide. After a long time of social isolation, restrictions and uncertainty, the development and distribution of vaccines with different immune platforms enabled the so desired mass immunization.
Differently from most of the countries, the COVID-19 vaccination in Brazil was initiated with CoronaVac, and this vaccine was responsible to protect the most affected individuals at that moment (healthcare workers, elderly and people with comorbidities). This was essential to reduce COVID-19 dispersion, hospitalization and deaths during a critical period (Gamma circulation) (Wu et al., 2021; Banho et al., 2022).
The success of this immunization protocol may be related with the fact that CoronaVac utilizes an inactivated virus technology, comprising the whole SARS-CoV-2 viral particle as the immunizing agent (Gao et al., 2020). In general, inactivated vaccines are associated with higher safety levels and mild adverse events when compared to attenuated virus and viral vector vaccines (Murdin et al., 1996; Vellozzi et al., 2009; Xia et al., 2020; Ghattas et al., 2021). Additionally, when compared to mRNA, viral vector and subunit vaccines, known to induce the immune system using only a part of the virion, the use of a completely inactivated virus, with many viral epitopes, could allow the induction of a broad-spectrum immune response, with a wider efficacy range when compared to the other platforms, probably providing a greater range of protection against the first emerging variants of concern (Alpha, Gamma and Delta) (Can et al., 2022; Cerqueira-Silva et al., 2022; Grenfell et al., 2022).
Despite all the approved vaccines were efficient in the induction of both serological and cellular immune responses (Costa et al., 2022; Zhang et al., 2022), reducing number of cases, probability of disease progression and deaths, they were not able to inhibit completely the viral circulation, especially in those countries where vaccination programs were still delayed.
This phenomenon allowed, in a lower scale, the continuous process of viral dispersion and evolution, resulting in the emergence of SARS-CoV-2 variants and subvariants capable to escape immune response. It is believed that, despite the divergent evolution of these new variants, with advantageous adaptations over their ancestor lineages, a mutation convergence trend on many hotspots of their RBD exists. These convergent substitutions would be responsible to guarantee evasion from neutralizing antibodies without negative impact on infectivity or transmissibility. These data suggests that herd immunity would not be able to protect from future infections, and could act in the opposite way, as a selective pressure to the emergence of new resistant subvariants (Yeh and Contreras, 2021; Cao et al., 2023).
As a result of this process, some omicron subvariants continued to emerge worldwide, leading to the conduction of studies to evaluate the immune protection of the population and the possibility of new infection waves. Among these subvariants, BA.5 and BQ.1.1 were detected circulating in high frequencies in many countries.
It is believed that BA.5 was responsible for the initiation of the fifth infection wave of COVID-19 in the world, replacing BA.2 and becoming the most predominant subvariant in South Africa, USA and Europe as of June 2022 (Desingu and Nagarajan, 2022; Tegally et al., 2022). After this, in September 2022, BQ.1.1 emerged and became the most frequent variant around the world in January 2023, being responsible for more than 50% of the global cases (Ao et al., 2023; WHO, 2023). In Brazil, the same situation was observed, with these two subvariants being responsible for the majority of the cases in the same period (de Menezes et al., 2023; de Sousa et al., 2023).
The results presented here evidenced that both omicron subvariants BA.5 and BQ.1.1 are less neutralized by antibodies induced by vaccination, when compared to subvariant BA.1. This lower response can be observed in all vaccination groups (no booster, 1 booster and 2 boosters) and it is corroborated by the scientific literature (Wang XJ. et al., 2022; Tauzin et al., 2023). A study published by Cao et al, in June 2022, revealed that BA.5 was capable to escape serological immune response, showing increased capability to evade antibody neutralization when compared to other subvariants (Cao et al., 2022).
It is worth mentioning that this humoral response evasion was described for antibodies from a prior exposure to BA.1 and for vaccination induced antibodies (Cao et al., 2022). In this last situation, individuals were vaccinated with a 2 doses CoronaVac protocol, followed by a booster dose with CoronaVac or RBD protein subunit vaccine (ZF2001) six months later, showing the capacity of BA.5 to escape humoral response from homologous and heterologous booster schemes (Cao et al., 2022). Our results reinforce this reality, where one booster dose was not enough to enhance considerably the neutralization mean titers against BA.5, and this could be observed in all time points.
For BQ.1.1, a similar pattern was described by Planas et al. (2023), presenting capacity to evade neutralization from 6 therapeutic monoclonal antibodies and from sera of individuals vaccinated with a 2 doses BNT162b2 vaccination protocol, followed by a homologous booster shot (Planas et al., 2023). However, in our results, an improvement of neutralization mean titer (199.1 to 673.7) was observed from the 9m to the 12m time point after the first booster (Figure 2B), showing that the use of a heterologous approach was more responsive against BQ.1.1 than BA.5.
The use of additional boosters to improve immune response against some viral infections is widely recommended (Javanian et al., 2021; Pattyn et al., 2021). In some cases, there is no need to reformulate or update the vaccine booster, like the vaccination scheme against hepatitis B virus, for example (Pattyn et al., 2021). In other situations, where it is known that the virus can evolve quickly, the regular administration and update of booster doses is required, such as the annual vaccination against Influenza (Javanian et al., 2021). Since SARS-CoV-2 subvariants are continually emerging, administering additional booster doses from time to time will likely be a necessity.
Our data reinforces this hypothesis, where the administration of a second booster dose enhanced the antibody neutralization titers, against all tested omicron subvariants (especially against BA.1), 18m after the primary protocol and 6m after the second booster (Figure 2C). It is worth highlighting that, when compared to those individuals vaccinated only with one booster, in the same time point, neutralization titer against BA.5 doubled (311.9 to 659.1), showing that this approach could recover immune protection against immunoresistant subvariants.
The same fact was observed in a phase 2-3 study, where a second booster dose with mRNA-1273, after three doses of the same vaccine (homologous booster protocol), was able to recover the antibody neutralization response against BA.1 and BA.5. Additionally, the authors compared these results with a protocol using the updated vaccine mRNA-1273.214 (encoding both spike proteins from Wuhan ancestral lineage and BA.1) as the second booster, and it was observed that neutralization against BA.1 and BA.5 was even greater, suggesting that vaccines platform update will play a key role in the next rounds against SARS-CoV-2 (Chalkias et al., 2022; Chalkias et al., 2023).
Our study aimed to observe the impact of a second booster dose in a real life context, where people are coexisting, in constant contact with the recently emerged subvariants of omicron. In a situation like this, it is normal to observe the activation of memory immune response (Rodda et al., 2022), and this was present in our study, where seroconvertion rate and neutralization mean titers for the no booster group increased after 12m of the CoronaVac primary protocol.
At that moment, one year after the approval of the first COVID-19 vaccine, all of the restrictive measurements in Brazil were abrogated, and a peak of omicron BA.1 infection was on course, increasing exposure to the virus (Lopes et al., 2022; Lamarca et al., 2023). Despite the limitation imposed by this constant activation of memory immunity in the cohort, the results presented here clearly show that the second booster dose, when compared with no booster and 1 booster groups, 18m after the primary vaccination, improved considerably the antibody response against all subvariants (Figure 2C). This antibody response was robust and long-lasting, being detected after six months of the second booster, and was not noticed at the 12m time point (Figure 2B) probably due to the short period between second booster shot and serum collection.
For the conception of this study, some criteria regarding sample inclusion and time-point collection needed to be followed, and this resulted in some limitations. Samples from different individuals were randomly collected, for each time-point, in order to have a more representative and heterogeneous cohort, picturing a real-life scenario without any selection bias. Unfortunately, this approach does not allow an analysis over time of the same individuals, as a follow-up, which could enrich the data regarding the effectiveness of vaccination and boosters. Additionally, this decision led to a predominance of a female population in our cohort.
Other limitations were also present in our study. It was not possible to use of a single vaccine as the second booster, since vaccines for this specific situation were distributed according to availability at that moment, making it unfeasible to evaluate the effectiveness of a specific vaccine as a second booster shot. Another point is that, at the time period of the study, it was almost impossible to evaluate the impact of boosters isolated. As cited earlier here, most of the population is in constant exposure to other people and, consequently, to the virus. Despite not being able to evaluate the boosters in a controlled isolated environment, these limitations allowed an even closer analysis of a real-life situation, one of the aims of our study.
The findings presented here reinforce the concern regarding immunity evasion of the SARS-CoV-2 omicron subvariants, showing that BA.5 and BQ.1.1 are less neutralized by vaccine induced antibodies than their ancestor subvariant, BA.1. However, the use of a second booster dose, after a three doses protocol (two doses of CoronaVac followed by a first booster with BNT162b2) is capable to enhance and recover antibody neutralization against these subvariants. This may indicate that, as new subvariants continue to emerge, additional immunizations will be needed over time.
Data availability statement
The raw data supporting the conclusions of this article will be made available by the authors, without undue reservation.
Ethics statement
The studies involving humans were approved by the Institutional review board (IRB) of the Ethics Committee of the Oswaldo Cruz Foundation - Protocol number CAAE 42898621.9.0000.5091. The studies were conducted in accordance with the local legislation and institutional requirements. The participants provided their written informed consent to participate in this study. Ethical approval was not required for the studies on animals in accordance with the local legislation and institutional requirements because only commercially available established cell lines were used.
Author contributions
GC: Conceptualization, Data curation, Formal analysis, Investigation, Methodology, Software, Validation, Visualization, Writing – original draft, Writing – review & editing. NA: Data curation, Investigation, Writing – review & editing. PF: Data curation, Investigation, Writing – review & editing. CC: Data curation, Investigation, Writing – review & editing. SG: Data curation, Investigation, Writing – review & editing. DM: Data curation, Investigation, Writing – review & editing. JA: Data curation, Investigation, Writing – review & editing. TS: Data curation, Investigation, Writing – review & editing. PA: Data curation, Investigation, Writing – review & editing. GF: Data curation, Investigation, Writing – review & editing. JD: Data curation, Investigation, Writing – review & editing. PR: Resources, Supervision, Writing – review & editing. RG: Conceptualization, Funding acquisition, Project administration, Resources, Supervision, Writing – original draft, Writing – review & editing. MN: Conceptualization, Funding acquisition, Project administration, Resources, Supervision, Writing – original draft, Writing – review & editing.
Funding
The author(s) declare financial support was received for the research, authorship, and/or publication of this article. We acknowledge the funding agencies for their contribution in this study. Part of the funding resources was provided by Oswaldo Cruz Foundation (Inova Fiocruz Program); MN is partly funded by the Centers for Research in Emerging Infectious Diseases (CREID), “The Coordinating Research on Emerging Arboviral Threats Encompassing the Neotropics (CREATE-NEO)” grant U01 AI151807 by the National Institutes of Health (NIH/USA); MN is a Brazilian National Council for Scientific and Technological Development (CNPQ) Research Fellow.
Acknowledgments
We acknowledge Dr. João Renato Rebelo Pinho, from Hospital Israelita Albert Einstein, for sequencing the Omicron subvariants, and Dr. Edison Durigon, from Universidade de São Paulo, for the isolation and distribution of BA.1 and BQ.1.1 subvariants.
Conflict of interest
MN has received research grants from Instituto Butantan, Janssen Vaccines & Prevention B.V., Medicago R&D Inc, and Pfizer/BioNTech SE.
The remaining authors declare that the research was conducted in the absence of any commercial or financial relationships that could be construed as a potential conflict of interest.
Publisher’s note
All claims expressed in this article are solely those of the authors and do not necessarily represent those of their affiliated organizations, or those of the publisher, the editors and the reviewers. Any product that may be evaluated in this article, or claim that may be made by its manufacturer, is not guaranteed or endorsed by the publisher.
Supplementary material
The Supplementary Material for this article can be found online at: https://www.frontiersin.org/articles/10.3389/fcimb.2024.1371695/full#supplementary-material
References
ANVISA (2021a). Parecer Público de avaliação de solicitação de autorização temporária de uso emergencial, em caráter experimental, da vacina adsorvida covid-19 (inativada) – Instituto Butantan (Brasil: Ministério da Saúde). Available at: https://www.gov.br/anvisa/pt-br/assuntos/paf/coronavirus/arquivos/arquivos-vacinas-uso-emergencial/ppam-final-vacina-adsorvida-covid-19-inativada-butantan-2.pdf.
ANVISA (2021b). Anvisa faz recomendações sobre doses de reforço de vacinas contra Covid-19 (Brasil: Ministério da Saúde). Available at: https://www.gov.br/anvisa/pt-br/assuntos/noticias-anvisa/2021/anvisa-faz-recomendacoes-sobre-doses-de-reforco-de-vacinas-contra-covid-19/copy_of_Voto214SEIDIRE2Avaliacaodosesreforco25351.931900_2021_841.pdf.
Ao, D., He, X., Hong, W., Wei, X. (2023). The rapid rise of SARS-CoV-2 Omicron subvariants with immune evasion properties: XBB.1.5 and BQ.1.1 subvariants. MedComm (2020) 4, e239. doi: 10.1002/mco2.239
Atmar, R. L., Lyke, K. E., Deming, M. E., Jackson, L. A., Branche, A. R., El Sahly, H. M., et al. (2022). Homologous and heterologous covid-19 booster vaccinations. N. Engl. J. Med. 386, 1046–1057. doi: 10.1056/NEJMoa2116414
Banho, C. A., Sacchetto, L., Campos, G. R. F., Bittar, C., Possebon, F. S., Ullmann, L. S., et al. (2022). Impact of SARS-CoV-2 Gamma lineage introduction and COVID-19 vaccination on the epidemiological landscape of a Brazilian city. Commun. Med. (Lond) 2, 41. doi: 10.1038/s43856-022-00108-5
Brasil (2023). Vacinômetro COVID-19. Ed. Saúde, M.d. (Brasil: Ministério da Saúde). Available at: https://infoms.saude.gov.br/extensions/SEIDIGI_DEMAS_Vacina_C19/SEIDIGI_DEMAS_Vacina_C19.html.
Bravo, L., Smolenov, I., Han, H. H., Li, P., Hosain, R., Rockhold, F., et al. (2022). Efficacy of the adjuvanted subunit protein COVID-19 vaccine, SCB-2019: a phase 2 and 3 multicenter, double-blind, randomized, placebo-controlled trial. Lancet 399, 461–472. doi: 10.1016/S0140-6736(22)00055-1
Callaway, E. (2022). COVID 'variant soup' is making winter surges hard to predict. Nature 611, 213–214. doi: 10.1038/d41586-022-03445-6
Callaway, E. (2023). Coronavirus variant XBB.1.5 rises in the United States - is it a global threat? Nature 613, 222–223. doi: 10.1038/d41586-023-00014-3
Campos, G. R. F., Almeida, N. B. F., Filgueiras, P. S., Corsini, C. A., Gomes, S. V. C., de Miranda, D. A. P., et al. (2022). Booster dose of BNT162b2 after two doses of CoronaVac improves neutralization of SARS-CoV-2 Omicron variant. Commun. Med. (Lond) 2, 76. doi: 10.1038/s43856-022-00141-4
Can, G., Acar, H. C., Aydin, S. N., Balkan, I. I., Karaali, R., Budak, B., et al. (2022). Waning effectiveness of CoronaVac in real life: A retrospective cohort study in health care workers. Vaccine 40, 2574–2579. doi: 10.1016/j.vaccine.2022.03.032
Cao, Y., Jian, F., Wang, J., Yu, Y., Song, W., Yisimayi, A., et al. (2022). BA.2.12.1, BA.4 and BA.5 escape antibodies elicited by Omicron infection. Nature 608, 593–602. doi: 10.1038/s41586-022-04980-y
Cao, Y., Yisimayi, A., Jian, F., Song, W., Xiao, T., Wang, L., et al. (2023). Imprinted SARS-CoV-2 humoral immunity induces convergent Omicron RBD evolution. Nature 614, 521–529. doi: 10.1038/s41586-022-05644-7
CDC (2021). COVID-19: SARS-CoV-2 variant classifications and definitions. Atlanta, USA: U.S. Department of Health and Human Services.
Cerqueira-Silva, T., Oliveira, V. A., Boaventura, V. S., Pescarini, J. M., Junior, J. B., Machado, T. M., et al. (2022). Influence of age on the effectiveness and duration of protection of Vaxzevria and CoronaVac vaccines: A population-based study. Lancet Reg. Health Am. 6, 100154. doi: 10.1016/j.lana.2021.100154
Chalkias, S., Harper, C., Vrbicky, K., Walsh, S. R., Essink, B., Brosz, A., et al. (2022). A bivalent omicron-containing booster vaccine against covid-19. N. Engl. J. Med. 387, 1279–1291. doi: 10.1056/NEJMoa2208343
Chalkias, S., Harper, C., Vrbicky, K., Walsh, S. R., Essink, B., Brosz, A., et al. (2023). Three-month antibody persistence of a bivalent Omicron-containing booster vaccine against COVID-19. Nat. Commun. 14, 5125. doi: 10.1038/s41467-023-38892-w
Costa, P. R., Correia, C. A., Marmorato, M. P., Dias, J. Z. C., Thomazella, M. V., Cabral da Silva, A., et al. (2022). Humoral and cellular immune responses to CoronaVac up to one year after vaccination. Front. Immunol. 13, 1032411. doi: 10.3389/fimmu.2022.1032411
de Menezes, M. T., Moreira, F. R. R., Whittaker, C., Santos, F. M., Queiroz, D. C., Geddes, V., et al. (2023). Dynamics of early establishment of SARS-coV-2 VOC omicron lineages in minas gerais, Brazil. Viruses 15. doi: 10.3390/v15020585
Desingu, P. A., Nagarajan, K. (2022). The emergence of Omicron lineages BA.4 and BA.5, and the global spreading trend. J. Med. Virol. 94, 5077–5079. doi: 10.1002/jmv.27967
de Sousa, L. A. F., Ferreira, L. S. S., Lobato, L. F. L., Ferreira, H., Sousa, L., Santos, V. F.D., et al. (2023). Molecular epidemiology of SARS-CoV-2 variants in circulation in the state of Maranhao, Brazil. J. Med. Virol. 95 (9), e29092. doi: 10.1002/jmv.29092
Falsey, A. R., Sobieszczyk, M. E., Hirsch, I., Sproule, S., Robb, M. L., Corey, L., et al. (2021). Phase 3 safety and efficacy of AZD1222 (ChAdOx1 nCoV-19) covid-19 vaccine. N. Engl. J. Med. 385, 2348–2360. doi: 10.1056/NEJMoa2105290
Favresse, J., Gillot, C., Di Chiaro, L., Eucher, C., Elsen, M., Van Eeckhoudt, S., et al. (2021). Neutralizing antibodies in COVID-19 patients and vaccine recipients after two doses of BNT162b2. Viruses 13 (7). doi: 10.3390/v13071364
Favresse, J., Gillot, C., Bayart, J. L., David, C., Simon, G., Wauthier, L., et al. (2023). Vaccine-induced binding and neutralizing antibodies against Omicron 6 months after a homologous BNT162b2 booster. J. Med. Virol. 95 (1), e28164. doi: 10.1002/jmv.28164
Furukawa, K., Tjan, L. H., Kurahashi, Y., Sutandhio, S., Nishimura, M., Arii, J., et al. (2022). Assessment of neutralizing antibody response against SARS-coV-2 variants after 2 to 3 doses of the BNT162b2 mRNA COVID-19 vaccine. JAMA Netw. Open 5, e2210780. doi: 10.1001/jamanetworkopen.2022.10780
Gao, Q., Bao, L., Mao, H., Wang, L., Xu, K., Yang, M., et al. (2020). Development of an inactivated vaccine candidate for SARS-CoV-2. Science 369, 77–81. doi: 10.1126/science.abc1932
Ghattas, M., Dwivedi, G., Lavertu, M., Alameh, M. G. (2021). Vaccine technologies and platforms for infectious diseases: current progress, challenges, and opportunities. Vaccines (Basel) 9 (12). doi: 10.3390/vaccines9121490
Golpour-Hamedani, S., Pourmasoumi, M., Askari, G., Bagherniya, M., Majeed, M., Guest, P. C., et al. (2023). Antiviral mechanisms of curcumin and its derivatives in prevention and treatment of COVID-19: A review. Adv. Exp. Med. Biol. 1412, 397–411. doi: 10.1007/978-3-031-28012-2_21
Grenfell, R. F. Q., Almeida, N. B. F., Filgueiras, P. S., Corsini, C. A., Gomes, S. V. C., de Miranda, D. A. P., et al. (2022). Immunogenicity, effectiveness, and safety of inactivated virus (CoronaVac) vaccine in a two-dose primary protocol and BNT162b2 heterologous booster in Brazil (Immunita-001): A one year period follow up phase 4 study. Front. Immunol. 13, 918896. doi: 10.3389/fimmu.2022.918896
Haas, E. J., McLaughlin, J. M., Khan, F., Angulo, F. J., Anis, E., Lipsitch, M., et al. (2022). Infections, hospitalizations, and deaths averted via a nationwide vaccination campaign using the Pfizer-BioNTech BNT162b2 mRNA COVID-19 vaccine in Israel: a retrospective surveillance study. Lancet Infect. Dis. 22, 357–366. doi: 10.1016/S1473-3099(21)00566-1
Hillus, D., Schwarz, T., Tober-Lau, P., Vanshylla, K., Hastor, H., Thibeault, C., et al. (2021). Safety, reactogenicity, and immunogenicity of homologous and heterologous prime-boost immunization with ChAdOx1 nCoV-19 and BNT162b2: a prospective cohort study. Lancet Respir. Med., 1255–1265. doi: 10.1016/S2213-2600(21)00357-X
Hoffmann, M., Kruger, N., Schulz, S., Cossmann, A., Rocha, C., Kempf, A., et al. (2022). The Omicron variant is highly resistant against antibody-mediated neutralization: Implications for control of the COVID-19 pandemic. Cell 185, 447–456.e11. doi: 10.1016/j.cell.2021.12.032
Iketani, S., Liu, L., Guo, Y., Liu, L., Chan, J. F., Huang, Y., et al. (2022). Antibody evasion properties of SARS-CoV-2 Omicron sublineages. Nature 604, 553–556. doi: 10.1038/s41586-022-04594-4
Javanian, M., Barary, M., Ghebrehewet, S., Koppolu, V., Vasigala, V., Ebrahimpour, S. (2021). A brief review of influenza virus infection. J. Med. Virol. 93, 4638–4646. doi: 10.1002/jmv.26990
Kärber, G. (1931). Beitrag zur kollektiven Behandlung pharmakologischer Reihenversuche. Archiv f experiment Pathol. u Pharmakol 162), 4. doi: 10.1007/BF01863914
Kupferschmidt, K. (2020). Despite obstacles, WHO unveils plan to distribute vaccine. Science 369, 1553. doi: 10.1126/science.369.6511.1553
Lamarca, A. P., Souza, U. J. B., Moreira, F. R. R., Almeida, L. G. P., Menezes, M. T., Souza, A. B., et al. (2023). The omicron lineages BA.1 and BA.2 (Betacoronavirus SARS-coV-2) have repeatedly entered Brazil through a single dispersal hub. Viruses 15. doi: 10.3390/v15040888
Lopes, P. H., Wellacott, L., de Almeida, L., Villavicencio, L. M. M., Moreira, A. L. L., Andrade, D. S., et al. (2022). Measuring the impact of nonpharmaceutical interventions on the SARS-CoV-2 pandemic at a city level: An agent-based computational modelling study of the City of Natal. PloS Glob Public Health 2, e0000540. doi: 10.1371/journal.pgph.0000540
Lopez-Cortes, G. I., Palacios-Perez, M., Velediaz, H. F., Hernandez-Aguilar, M., Lopez-Hernandez, G. R., Zamudio, G. S., et al. (2022). The spike protein of SARS-coV-2 is adapting because of selective pressures. Vaccines (Basel) 10 (6). doi: 10.3390/vaccines10060864
Martin, M. A., VanInsberghe, D., Koelle, K. (2021). Insights from SARS-coV-2 sequences. Science 371, 466–467. doi: 10.1126/science.abf3995
Martinez-Baz, I., Trobajo-Sanmartin, C., Miqueleiz, A., Egues, N., Garcia Cenoz, M., Casado, I., et al. (2024). Hospitalizations and deaths averted by COVID-19 vaccination in navarre, Spain, 2021-2022. Vaccines (Basel) 12 (1). doi: 10.3390/vaccines12010058
Martins, J. P., Siqueira, B. A., Sansone, N. M. S., Marson, F. A. L. (2023). COVID-19 in Brazil: a 3-year update. Diagn. Microbiol. Infect. Dis. 107, 116074. doi: 10.1016/j.diagmicrobio.2023.116074
Mathieu, E., Ritchie, H., Ortiz-Ospina, E., Roser, M., Hasell, J., Appel, C., et al. (2021). A global database of COVID-19 vaccinations. Nat. Hum. Behav. 5, 947–953. doi: 10.1038/s41562-021-01122-8
Moradpour, J., Chit, A., Besada-Lombana, S., Grootendorst, P. (2023). Overview of the global vaccine ecosystem. Expert Rev. Vaccines 22, 749–763. doi: 10.1080/14760584.2023.2250433
Murdin, A. D., Barreto, L., Plotkin, S. (1996). Inactivated poliovirus vaccine: past and present experience. Vaccine 14, 735–746. doi: 10.1016/0264-410X(95)00211-I
Ozbay Kurt, F. G., Lepper, A., Gerhards, C., Roemer, M., Lasser, S., Arkhypov, I., et al. (2022). Booster dose of mRNA vaccine augments waning T cell and antibody responses against SARS-CoV-2. Front. Immunol. 13, 1012526. doi: 10.3389/fimmu.2022.1012526
Palacios, R., Patino, E. G., de Oliveira Piorelli, R., Conde, M., Batista, A. P., Zeng, G., et al. (2020). Double-Blind, Randomized, Placebo-Controlled Phase III Clinical Trial to Evaluate the Efficacy and Safety of treating Healthcare Professionals with the Adsorbed COVID-19 (Inactivated) Vaccine Manufactured by Sinovac - PROFISCOV: A structured summary of a study protocol for a randomized controlled trial. Trials 21, 853. doi: 10.1186/s13063-020-04775-4
Pastorino, B., Touret, F., Gilles, M., de Lamballerie, X., Charrel, R. N. (2020). Heat inactivation of different types of SARS-coV-2 samples: what protocols for biosafety, molecular detection and serological diagnostics? Viruses 12 (7). doi: 10.3390/v12070735
Pattyn, J., Hendrickx, G., Vorsters, A., Van Damme, P. (2021). Hepatitis B vaccines. J. Infect. Dis. 224, S343–S351. doi: 10.1093/infdis/jiaa668
Perez-Then, E., Lucas, C., Monteiro, V. S., Miric, M., Brache, V., Cochon, L., et al. (2022). Neutralizing antibodies against the SARS-CoV-2 Delta and Omicron variants following heterologous CoronaVac plus BNT162b2 booster vaccination. Nat. Med. 28, 481–485. doi: 10.1038/s41591-022-01705-6
Planas, D., Veyer, D., Baidaliuk, A., Staropoli, I., Guivel-Benhassine, F., Rajah, M. M., et al. (2021). Reduced sensitivity of SARS-CoV-2 variant Delta to antibody neutralization. Nature 596, 276–280. doi: 10.1038/s41586-021-03777-9
Planas, D., Saunders, N., Maes, P., Guivel-Benhassine, F., Planchais, C., Buchrieser, J., et al. (2022). Considerable escape of SARS-CoV-2 Omicron to antibody neutralization. Nature 602, 671–675. doi: 10.1038/s41586-021-04389-z
Planas, D., Bruel, T., Staropoli, I., Guivel-Benhassine, F., Porrot, F., Maes, P., et al. (2023). Resistance of Omicron subvariants BA.2.75.2, BA.4.6, and BQ.1.1 to neutralizing antibodies. Nat. Commun. 14 (1), 824. doi: 10.1038/s41467-023-36561-6
Rodda, L. B., Morawski, P. A., Pruner, K. B., Fahning, M. L., Howard, C. A., Franko, N., et al. (2022). Imprinted SARS-CoV-2-specific memory lymphocytes define hybrid immunity. Cell 185, 1588–1601.e14. doi: 10.1016/j.cell.2022.03.018
Rzymski, P., Camargo, C. A., Jr, Fal, A., Flisiak, R., Gwenzi, W., Kelishadi, R., et al. (2021). COVID-19 vaccine boosters: the good, the bad, and the ugly. Vaccines (Basel) 9 (11). doi: 10.3390/vaccines9111299
Sadoff, J., Gray, G., Vandebosch, A., Cardenas, V., Shukarev, G., Grinsztejn, B., et al. (2021). Safety and efficacy of single-dose ad26.COV2.S vaccine against covid-19. N. Engl. J. Med. 384, 2187–2201. doi: 10.1056/NEJMoa2101544
Schultz, B. M., Melo-Gonzalez, F., Duarte, L. F., Galvez, N. M. S., Pacheco, G. A., Soto, J. A., et al. (2022). A booster dose of coronaVac increases neutralizing antibodies and T cells that recognize delta and omicron variants of concern. mBio 13, e0142322. doi: 10.1128/mbio.01423-22
Shang, J., Ye, G., Shi, K., Wan, Y., Luo, C., Aihara, H., et al. (2020). Structural basis of receptor recognition by SARS-CoV-2. Nature 581, 221–224. doi: 10.1038/s41586-020-2179-y
Souza, W. M., Amorim, M. R., Sesti-Costa, R., Coimbra, L. D., Brunetti, N. S., Toledo-Teixeira, D. A., et al. (2021). Neutralization of SARS-CoV-2 lineage P.1 by antibodies elicited through natural SARS-CoV-2 infection or vaccination with an inactivated SARS-CoV-2 vaccine: an immunological study. Lancet Microbe 2, e527–e535. doi: 10.1016/S2666-5247(21)00129-4
Spearman, C. (1908). The method of “Right and wrong cases” (Constant stimuli) without gauss’s formula. Br. J. Psychol. (2), 15. doi: 10.1037/h0063767
Steele, M. K., Couture, A., Reed, C., Iuliano, D., Whitaker, M., Fast, H., et al. (2022). Estimated number of COVID-19 infections, hospitalizations, and deaths prevented among vaccinated persons in the US, December 2020 to September 2021. JAMA Netw. Open 5, e2220385. doi: 10.1001/jamanetworkopen.2022.20385
Tauzin, A., Nicolas, A., Ding, S., Benlarbi, M., Medjahed, H., Chatterjee, D., et al. (2023). Spike recognition and neutralization of SARS-CoV-2 Omicron subvariants elicited after the third dose of mRNA vaccine. Cell Rep. 42, 111998. doi: 10.1016/j.celrep.2023.111998
Tegally, H., Moir, M., Everatt, J., Giovanetti, M., Scheepers, C., Wilkinson, E., et al. (2022). Emergence of SARS-coV-2 omicron lineages BA.4 and BA.5 in South Africa. Nat. Med. 28, 1785–1790. doi: 10.1038/s41591-022-01911-2
Thakkar, K., Spinardi, J. R., Yang, J., Kyaw, M. H., Ozbilgili, E., Mendoza, C. F., et al. (2023). Impact of vaccination and non-pharmacological interventions on COVID-19: a review of simulation modeling studies in Asia. Front. Public Health 11, 1252719. doi: 10.3389/fpubh.2023.1252719
Theparod, T., Kreabkhontho, P., Teparos, W. (2023). Booster dose vaccination and dynamics of COVID-19 pandemic in the fifth wave: an efficient and simple mathematical model for disease progression. Vaccines (Basel) 11. doi: 10.3390/vaccines11030589
Vellozzi, C., Burwen, D. R., Dobardzic, A., Ball, R., Walton, K., Haber, P. (2009). Safety of trivalent inactivated influenza vaccines in adults: background for pandemic influenza vaccine safety monitoring. Vaccine 27, 2114–2120. doi: 10.1016/j.vaccine.2009.01.125
Wang, L., Cheng, G. (2022). Sequence analysis of the emerging SARS-CoV-2 variant Omicron in South Africa. J. Med. Virol. 94, 1728–1733. doi: 10.1002/jmv.27516
Wang, Q., Li, Z., Ho, J., Guo, Y., Yeh, A. Y., Mohri, H., et al. (2022a). Resistance of SARS-CoV-2 omicron subvariant BA.4.6 to antibody neutralization. Lancet Infect. Dis. 22, 1666–1668. doi: 10.1016/S1473-3099(22)00694-6
Wang, X. J., Yao, L., Zhang, H. Y., Zhu, K. L., Zhao, J., Zhan, B. D., et al. (2022b). Neutralization sensitivity, fusogenicity, and infectivity of Omicron subvariants. Genome Med. 14, 146. doi: 10.1186/s13073-022-01151-6
Wang, Q., Iketani, S., Li, Z., Liu, L., Guo, Y., Huang, Y., et al. (2023). Alarming antibody evasion properties of rising SARS-CoV-2 BQ and XBB subvariants. Cell 186, 279–286.e8. doi: 10.1016/j.cell.2022.12.018
Wen, G. P., Zhu, M., Li, L. R., Li, X. J., Ye, H. M., Zhou, Y. L. (2023). Homologous booster immunization with an inactivated vaccine induced robust antibody response in healthcare workers: A retrospective study. Front. Immunol. 14, 1099629. doi: 10.3389/fimmu.2023.1099629
Willett, J. D. S., Gravel, A., Dubuc, I., Gudimard, L., Dos Santos Pereira Andrade, A. C., Lacasse, E., et al. (2024). SARS-CoV-2 rapidly evolves lineage-specific phenotypic differences when passaged repeatedly in immune-naive mice. Commun. Biol. 7, 191. doi: 10.1038/s42003-024-05878-3
Wu, Z., Hu, Y., Xu, M., Chen, Z., Yang, W., Jiang, Z., et al. (2021). Safety, tolerability, and immunogenicity of an inactivated SARS-CoV-2 vaccine (CoronaVac) in healthy adults aged 60 years and older: a randomized, double-blind, placebo-controlled, phase 1/2 clinical trial. Lancet Infect. Dis. 21, 803–812. doi: 10.1016/S1473-3099(20)30987-7
Xia, S., Duan, K., Zhang, Y., Zhao, D., Zhang, H., Xie, Z., et al. (2020). Effect of an inactivated vaccine against SARS-coV-2 on safety and immunogenicity outcomes: interim analysis of 2 randomized clinical trials. JAMA 324, 951–960. doi: 10.1001/jama.2020.15543
Yeh, T.-Y., Contreras, G. P. (2021). Full vaccination against COVID-19 suppresses SARS-CoV-2 delta variant and spike gene mutation frequencies and generates purifying selection pressure. MedRxiv. doi: 10.1101/2021.08.08.21261768
Yoo, J., Kim, Y., Cha, Y. M., Lee, J., Jeong, Y. J., Kim, S. H., et al. (2023). Heterologous vaccination (ChAdOx1 and BNT162b2) induces a better immune response against the omicron variant than homologous vaccination. J. Infect. Public Health 16, 1537–1543. doi: 10.1016/j.jiph.2023.07.017
Zhang, Y., Zeng, G., Pan, H., Li, C., Hu, Y., Chu, K., et al. (2021). Safety, tolerability, and immunogenicity of an inactivated SARS-CoV-2 vaccine in healthy adults aged 18-59 years: a randomized, double-blind, placebo-controlled, phase 1/2 clinical trial. Lancet Infect. Dis. 21, 181–192. doi: 10.1016/S1473-3099(20)30843-4
Zhang, Z., Mateus, J., Coelho, C. H., Dan, J. M., Moderbacher, C. R., Galvez, R. I., et al. (2022). Humoral and cellular immune memory to four COVID-19 vaccines. Cell 185, 2434–2451.e17. doi: 10.1016/j.cell.2022.05.022
Keywords: SARS-CoV-2, vaccination, booster, omicron, variants, antibody neutralization
Citation: Campos GRF, Almeida NBF, Filgueiras PS, Corsini CA, Gomes SVC, de Miranda DAP, de Assis JV, Silva TBdS, Alves PA, Fernandes GdR, de Oliveira JG, Rahal P, Grenfell RFQ and Nogueira ML (2024) Second booster dose improves antibody neutralization against BA.1, BA.5 and BQ.1.1 in individuals previously immunized with CoronaVac plus BNT162B2 booster protocol. Front. Cell. Infect. Microbiol. 14:1371695. doi: 10.3389/fcimb.2024.1371695
Received: 16 January 2024; Accepted: 14 March 2024;
Published: 04 April 2024.
Edited by:
Francis O. Eko, Morehouse School of Medicine, United StatesReviewed by:
Gagandeep Singh, Icahn School of Medicine at Mount Sinai, United StatesLaura Pérez-Alós, University of Copenhagen, Denmark
Copyright © 2024 Campos, Almeida, Filgueiras, Corsini, Gomes, de Miranda, de Assis, Silva, Alves, Fernandes, de Oliveira, Rahal, Grenfell and Nogueira. This is an open-access article distributed under the terms of the Creative Commons Attribution License (CC BY). The use, distribution or reproduction in other forums is permitted, provided the original author(s) and the copyright owner(s) are credited and that the original publication in this journal is cited, in accordance with accepted academic practice. No use, distribution or reproduction is permitted which does not comply with these terms.
*Correspondence: Rafaella Fortini Queiroz Grenfell, rafaella.queiroz@fiocruz.br; Maurício L. Nogueira, mauricio.nogueira@edu.famerp.br