The role of post-translational modifications in driving abnormal cardiovascular complications at high altitude
- 1Department of Cardiology, Chengdu Third People’s Hospital, Cardiovascular Disease Research Institute of Chengdu, Affiliated Hospital of Southwest Jiaotong University, Chengdu, China
- 2School of Material Science and Engineering, Southwest Jiaotong University, Chengdu, China
- 3Department of Gastroenterology and Hepatology, Chengdu First People’s Hospital, Chengdu, China
- 4Department of Medicine, Faculty of Medicine, University of British Columbia, Vancouver, BC, Canada
- 5Center for Heart and Lung Innovation, St. Paul’s Hospital, University of British Columbia, Vancouver, BC, Canada
- 6Department of Vascular Surgery, Renji Hospital, School of Medicine, Shanghai Jiao Tong University, Shanghai, China
The high-altitude environment is characterized by hypobaric hypoxia, low temperatures, low humidity, and high radiation, which is a natural challenge for lowland residents entering. Previous studies have confirmed the acute and chronic effects of high altitude on the cardiovascular systems of lowlanders. Abnormal cardiovascular complications, including pulmonary edema, cardiac hypertrophy and pulmonary arterial hypertension were commonly explored. Effective evaluation of cardiovascular adaptive response in high altitude can provide a basis for early warning, prevention, diagnosis, and treatment of altitude diseases. At present, post-translational modifications (PTMs) of proteins are a key step to regulate their biological functions and dynamic interactions with other molecules. This process is regulated by countless enzymes called “writer, reader, and eraser,” and the performance is precisely controlled. Mutations and abnormal expression of these enzymes or their substrates have been implicated in the pathogenesis of cardiovascular diseases associated with high altitude. Although PTMs play an important regulatory role in key processes such as oxidative stress, apoptosis, proliferation, and hypoxia response, little attention has been paid to abnormal cardiovascular response at high altitude. Here, we reviewed the roles of PTMs in driving abnormal cardiovascular complications at high altitude.
Introduction
Nowadays, a large number of lowlanders ascend to high altitudes (elevation ≥ 2,700 m) for military training, commercial working, mountaineering, and traveling every year. However, high-altitude environments, with the characteristics of lower barometric pressure, lower oxygen partial pressure, lower temperature, lower ambient humidity, and higher ultraviolet ray radiation, induce a series of system complications for the low-altitude human body (1, 2), especially for the cardiovascular system (3–5). Generally, people at low altitudes are prone to high altitude pulmonary edema (HAPE) due to acute hypoxia when suffering with rapid high-altitude exposure (2, 6). And lowlanders who live in a high altitude for a long time (> 3 months) are more likely to suffer from chronic high-altitude heart disease (HAHD), such as cardiac hypertrophy and pulmonary arterial hypertension (2, 6, 7). During acute and chronic high-altitude exposure in lowlanders, the ability of cardiovascular adaptation to high altitude is regarded as a key factor for the occurrence of abnormal cardiovascular complications. Therefore, effectively exploring relevant factors to evaluate the cardiovascular adaptation ability of lowlanders could be a promising strategy to decrease the occurrence of HAPE and HAHD.
Protein post-translational modifications (PTMs) refer to the covalent addition process of functional groups mediated by enzymes during or after protein translation (8). These modifications greatly increase the complexity of organisms and result in order-of-magnitude changes between the various proteins encoded in the genome and their biological functions (9, 10).
After translation, most proteins pass through different PTMs to maintain their structure, stability, and interactions with other actors in complex biological systems (11, 12). Moreover, transcription factors, signaling molecules proteins involved in hypoxia-induced myocardial cell proliferation, inflammation and reparation are subjected to various PTMs (13, 14). Therefore, the pathogenesis of dysregulation expression of enzymes in the PTMs steps is related to abnormal cardiovascular complications at high altitude.
Proteome-wide PTMs analysis in these high-altitude cardiovascular diseases will help clarify the underlying molecular mechanisms and provide new therapeutic targets for the prevention, diagnosis, and treatment of abnormal cardiovascular complications at high altitude. Here, we summarize recent findings on the roles of the most common types of PTMs in high-altitude cardiovascular complications, with emphasis on acetylation, phosphorylation, methylation, glycosylation, citrullination, crotonylation, lactylation, and sumoylation. In addition, we discussed the challenges and prospects of targeted PTMs application for abnormal cardiovascular responses at high altitude.
High-altitude heart diseases
The description of abnormal cardiovascular complications at high altitude
Apart from the original residents of the plateau for generations, high altitude could result in abnormal cardiovascular complications for low-altitude individuals. It may take days, weeks, and even months for people who previously lived at low-altitude to adapt to hypobaric hypoxia, thereby acute high-altitude sickness, with an incidence of 10–85% or chronic high-altitude sickness, with an incidence of nearly 100% may occur.
The abnormal adaptation response to high altitude can cause physio-pathological changes of varying organs and tissues. Specifically, it was reported that high altitude could induce irreversible/reversible damage to high oxygen and energy demanding tissues, such as the brain, heart, liver, gastrointestinal tract, and ocular tissue, over a certain period of time (15–20). The clinical characteristics commonly include blood pressure fluctuation, loss of memory function (declined cognitive function), absence of equilibrium function, headache, indigestion, decreased appetite, and so on (21–24).
As is well known, heart is one of the most metabolically active organs in the human body. In previous studies, much attention has paid to high altitude pulmonary edema (HAPE), a typical acute mountain sickness (AMS). They have been demonstrated to be implicated in acute altitude reactions for the reduction of partial oxygen pressure, and the possible mechanism may be related to uneven pulmonary vasoconstriction and disruption of the air-blood barrier, especially intracellular edema (25). Moreover, high altitude heart disease (HAHD) is used to describe the abnormal cardiovascular complications caused by high altitude. Generally, the narrow sense of HAHD refers in particular to high altitude pulmonary hypertension, a typical chronic high altitude disease. In a broad sense, HAHD refers to all kinds of heart damage caused by high altitude, including cardiac hypertrophy, arrhythmia, heart failure, pulmonary hypertension and so on. Evidences from professor Huang in Army Medical University considered that the pathogenesis of HAHD was related with abnormal cardiovascular adaptation response to high altitude (26).
The process and evaluation of abnormal cardiovascular complications at high altitude
Acute cardiovascular caused by high altitude can often be divided into two typical processes. At initial entry to high altitude, cardiac output and heart rate are increased to compensate for low oxygen levels in the coronary artery. After a few days of good acclimatization, cardiac output returns to normal, mainly due to increased heart rate and reduced stroke volume. When inappropriate high-altitude adaptation occurs, ventricular filling patterns and ventricular systolic and diastolic functions would be impaired (27, 28). Then, sustaining hypoxia conditions may result in chronic cardiovascular complications, featured with hypoxic pulmonary vasoconstriction and the enhancement of pulmonary arterial pressure, which increases right ventricular afterload and impairs its function (28).
As for acute cardiovascular complications, signs (cyanosis, rapid heart and respiratory rates, edema of the face, liver enlargement, and rales) and symptoms (headache, dyspnea, cough, and sleeplessness) could be easily recognized. When it comes to chronic cardiovascular complications, there is no consensus on how to quickly identify them. Generally, the pulmonary artery pressure is used to evaluate the process of chronic cardiovascular complications. The international standard for chronic altitude disease is average resting pulmonary artery pressure > 30 mmHg or pulmonary artery systolic pressure > 50 mmHg, and average pulmonary artery pressure > 50 mmHg or pulmonary artery systolic pressure > 65 mmHg in infants. Additionally, the standard of pulmonary hypertension stipulated by the WHO is the mean pulmonary arterial pressure > 20 mmHg with capillary wedge pressure ≤ 15 mmHg.
Therefore, accurately predicting the risk level of abnormal cardiovascular responses at high altitude may be an effective way to reduce HAHD. More recently, cardiac function parameters at sea level were used as predictors of HAHD occurrence by screening echocardiographic parameters of the left ventricle, right ventricle, and pulmonary circulation (29). Moreover, changes in blood pressure (BP) and BP load were clinically found to be higher in HAHD patients, suggesting that BP load can be an effective indicator to evaluate cardiovascular adaptation ability (30). Furthermore, study found subjects with low 25% of the pulmonary volume values at low altitude were shown to be susceptible to high levels of pulmonary arterial pressure (31).
Basic studies of abnormal cardiovascular complications at high altitude
At high altitude, the cardiovascular system must adapt to meet the metabolic need for oxygen (27). Meanwhile, it also increases the oxygen demand of the heart to react for the release of adrenaline and pulmonary artery pressure. It is well known that low-pressure hypoxia is a major challenge at high altitude and causes acute mountain sickness (29). Normal cardiovascular adaptation can effectively promote oxygen delivery to satisfy the body’s mitochondria metabolic demand and abnormal cardiovascular adaptation may lead to ventricular enlargement, pulmonary hypertension, and myocardial fibrosis. Animal studies found that mice exposed to a simulated high-altitude environment could develop myocardial hypertrophy and lesions involving the left and right ventricles (32). Electron microscopy showed dissolved or degenerated myofibrils, swollen mitochondria, dilated endoplasmic reticulum, and decreased glycogen granules (33).
Studies have shown that cardiac remodeling induced by altitude involved multiple mechanisms. Perinatal hypoxia at high altitude can cause neonatal pulmonary hypertension and right heart failure, which is closely related to the production of superoxide anion in mitochondria (34). Moreover, the high-altitude environment could destroy cardiac protein folding homeostasis and cause unbalanced endoplasmic reticulum stress. Animal studies showed that hypoxia stress enhanced the generation of free radicals, resulting in enhanced expression of hypoxia inducible factor 1α (HIF1α) (35). Combined with cold stress, reduced oxygen availability leads to extensive protein oxidative modification, accompanied by cardiac tissue damage and matrix remodeling. The presence of oxidized protein resulted in significantly up-regulated expression of Glucose Regulated Protein 78 (GRP78) and protein disulfide isomerase (PDI) in endoplasmic reticulum chaperone in hypoxia exposed animals (35).
Post-translational modifications
The basic descriptions of post-translational modifications
It is well-known that the human proteome is significantly more complex than the human genome. Specifically, researchers estimated that the human genome may contain between 20,000 and 25,000 genes, while the human proteome was estimated to contain more than 1 million proteins. The increase in complexity from the level of the genome to the proteome is further facilitated by protein PTMs. PTMs occur at different amino acid side chains or peptide bonds via covalently adding functional groups or proteins, proteolytic cleavage regulatory subunits, or entire protein degradation, and are usually mediated by enzyme activity.
PTMs are essential mechanisms to diversify protein functions, controlling protein stability, localization, and conformation. Moreover, PTMs can regulate protein interaction with other cellular molecules such as proteins, nucleic acids, lipids, and cofactors. Therefore, even if the expression level of the protein is not changed, the function of the protein can be significantly changed if the status of the PTMs is changed. Generally, the effect of PTMs on protein function is diverse, which is manifested in the following three aspects: (1) The same protein will be endowed with multiple functions even if only one type of modification occurs; (2) The same PTMs of the same protein can have different functions if it occurs on different amino acids; (3) The same protein may also have different modifications, and its functions and biological processes are more complex.
These modifications affect nearly all aspects of normal cell biology and pathogenesis. In fact, it is estimated that 5% of the proteome contains more than 400 PTMs. These enzymes include kinases, phosphatases, transferases, and ligases, which add or remove functional groups, proteins, lipids, or sugars from amino acid side chains. There are also proteases, which remove specific sequences or modulate subunits by breaking peptide bonds. Many proteins can also modify themselves using autocatalytic domains, such as self-kinases and self-proteolytic domains. Table 1 shows the most common and best studied types of PTMs, including acetylation, phosphorylation, methylation, glycosylation, citrullination, and sumoylation (36, 37). Recently, studies have shown that both bacteria and humans could adapt to new environments through PTMs (38–40), while the role of PTMs in abnormal cardiovascular adaptive response at high altitude has rarely been reported.
The potential application area of post-translational modifications
The understanding of PTM function mainly focuses on phosphorylation, acetylation, ubiquitin, glycosylation, and other modifications. Even a basic understanding of these types of modifications would greatly expand our knowledge of biological processes and regulatory mechanisms. Therefore, the analysis of proteins and their PTMs is particularly important for the study of heart disease, cancer, neurodegenerative diseases, and diabetes.
Specifically, phosphorylation is involved in almost all biological processes. In addition to being the core mechanism of signal transduction, it is also involved in mitochondrial function, cytoskeleton regulation, cell membrane protein function and transcriptional regulation. And Non-histone acetylation is widely distributed in cytoplasm, mitochondria and other organelles, participating in signal transduction, energy metabolism, cytoskeleton, transcription factor activity, and so on. Additionally, glycosylation occurs mostly in proteins expressed on membranes and is important for macromolecular recognition. As for methylation, the methylation of DNA and histones is best known to be involved in epigenetic regulation. In recent years, more and more studies have found that methylation also occurs on non-histone proteins and plays an important role in many signal transduction processes. Interestingly, PTMs seem to play an important role in the process of heart remodeling induced by altitude. Table 2 shows the previous studies related with the PTMs patterns and high-altitude heart diseases.
PTM is an important regulatory mechanism of biological functions, as important as transcription and protein expression regulation, but much more complex. However, the current study is only the tip of the iceberg when it comes to the profound understanding of PTMs.
Role of different post-translational modifications in high-altitude cardiac remodeling
Acetylation/deacetylation
Protein acetylation/deacetylation is a reversible PTM in which an acetyl group is added or removed from the amino acid of a protein (59, 60). The process is catalyzed by two groups of enzymes, K lysine acetyltransferase (KAT) and K lysine deacetyltransferase (KADC) (59–61). Acetylation or deacetylation of non-histone proteins modifies a series of biological processes including enzymatic activity, inflammation, autophagy, protein–protein interactions, and protein localization. Either aberrant expression of acetyltransferases/deacetylases or alteration in the status of acetylation of protein targets are found in acute and chronic effects of high altitude on the cardiovascular system of lowlanders.
Sirtuin (SIRT) is a mammalian NAD+-dependent deacetylase family composed of seven SIRT1-7 members, which plays an important role in the abnormal cardiovascular complications at high altitude (62). Recently, the potential mechanisms of SIRTS in HAHD have been extensively investigated from clinical observations to molecular studies (63, 64). Animal studies have shown that SIRT1 expression was reduced in an acute hypoxia-induced pulmonary hypertension rat model. Interestingly, resveratrol (a small molecule agonists of SIRT1) and SRT1720 (a selective activator of SIRT1) could reverse the proliferation of pulmonary smooth muscle cells (65). Moreover, cell studies confirmed that SIRT1 could promote pulmonary artery endothelial cells proliferation and inhibit apoptosis in a simulated hypoxic environment. This mechanism may be related to the activation of protein kinase B (Akt) signaling pathway and B-cell lymphoma-2 (Bcl-2) pathway (66). Moreover, it is well-known that cardiovascular complications are often accompanied with mitochondrial dysfunction (67), and acetylation/deacetylation of mitochondrial proteins are considered as a key regulatory factor of mitochondrial metabolism and function (68). SIRT3 has been reported to be a major mechanism for regulating protein acetylation in mitochondria through pyruvate dehydrogenase and aconitase deacetylation (68). Overexpression of SIRT3 has been shown to prevent the accumulation of reactive oxygen species (ROS) in cardiomyocytes in response to different environmental stressors (69) and α1-adrenergic receptor agonist phenylephrine (70).
Cardiomyopathy hypertrophy is a typical abnormal cardiovascular complication of high altitude, ultimately leading to heart failure (71, 72). Histone acetylation plays an important role in epigenetic remodeling in the pathogenesis of cardiac hypertrophy (73). Specifically, histone deacetylase 2 (HDAC2) can result in severe cardiac hypertrophy, while HDAC2 knockout mice are resistant to exogenous hypertrophy stimulation (73, 74). Additionally, HDAC1 and HDAC5 were elevated in the right ventricles of rats when exposed to acute hypoxia. Valproic acid and class I HDAC inhibitors SAHA could alleviate and reduce the development of hypoxia induced pulmonary hypertension (75).
The role of other members of the histone deacetylase family in PTMs in abnormal cardiovascular complications at high altitude is unclear, but the potential therapeutic effects of SIRT1 agonists or class I histone deacetylase inhibitors have been observed in existing studies. Therefore, histone acetylation and deacetylation modification may be one of the options for the prevention of acute and chronic cardiovascular complications at high altitude.
Phosphorylation
Protein phosphorylation is the addition of a phosphate group to an intermediate metabolite or protein. Enzymes capable of removing phosphate groups are called phosphatases (76). Protein phosphorylation occurs on many types of amino acids (the main unit of proteins), with serine predominating, followed by threonine. Dephosphorylation refers to the removal of phosphate groups and acts as an “on/off” effect for many organisms (77).
It is widely believed that metabolic changes in individuals exposed to high altitude are due to ambient hypoxia and lower atmospheric pressure. The discovery of hypoxia inducible factor 1 (HIF1), a transcription factor, is a breakthrough in the study of high-altitude adaptation response (78). A recent study found that HIF1α transcription primarily regulated metabolic reprogramming, while HIF2α exerted a greater role in regulating angiogenic extracellular signaling, guidance cues, and extracellular matrix remodeling factors (79). HIF1 is a heterodimer composed of oxygen-sensitive HIF1α and oxygen-independent subunit HIF1β. Recently, a key role of PTM of HIF1α in cellular oxygen sensitivity has been identified (80). Under normoxic conditions, HIF1α hydroxylates on specific proline residues, leading to immediate ubiquitination and subsequent proteasome degradation of α subunits (81). In addition, hydroxylation of asparagine residues blocks transcriptional activity of HIF1 by inhibiting its interaction with coactivators. Conversely, the elimination of proline hydroxylation leads to HIF1α stabilization under hypoxia, while the absence of asparagine hydroxylation allows transcriptional activity. In addition, transcriptional activity can be regulated by phosphorylation or methylation modification of HIF1 (81). Figure 1 shows the different PTMs of HIF1α under normoxia and hypoxia conditions, respectively.
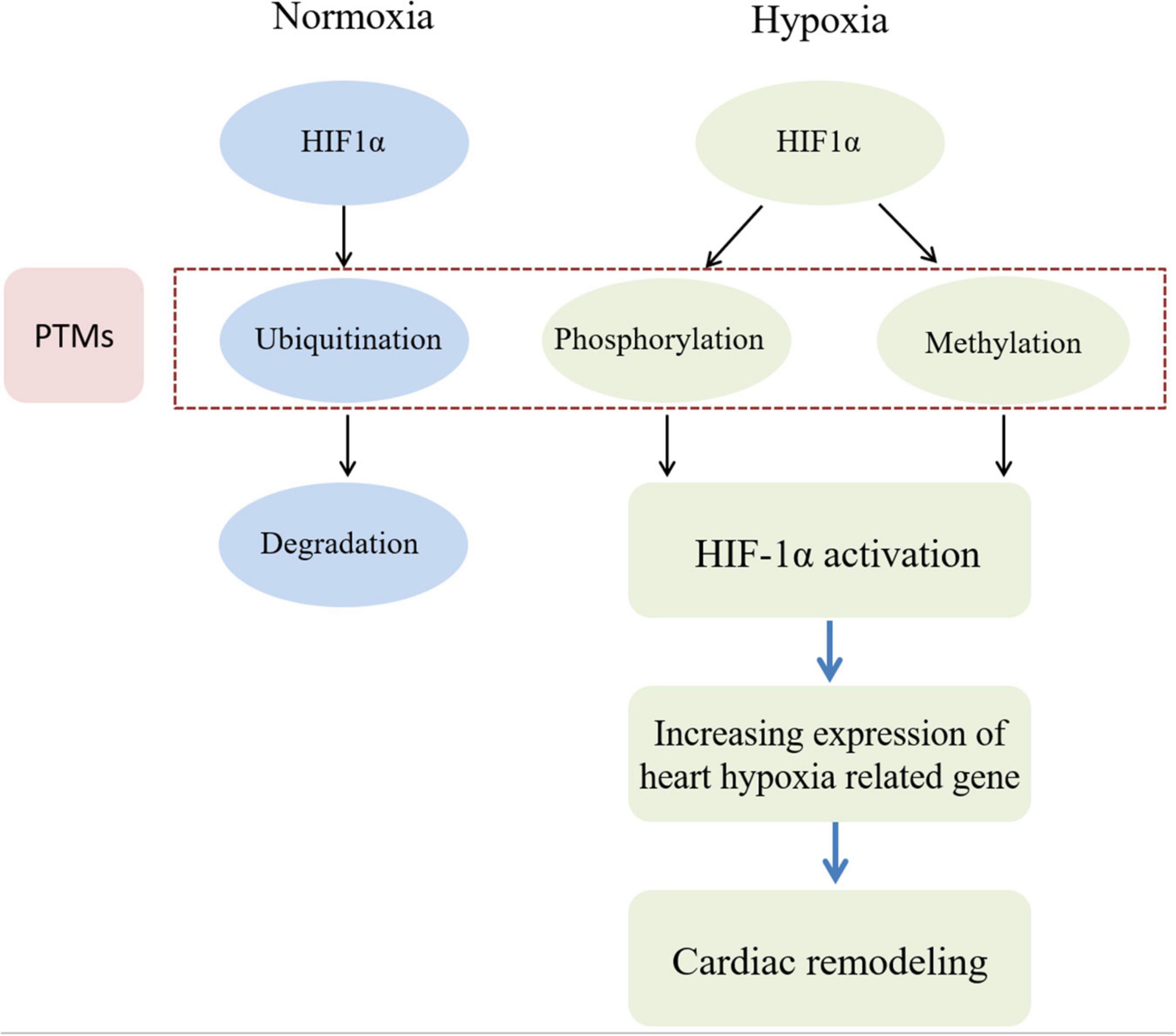
Figure 1. HIF phosphorylation and methylation are involved in cardiac remodeling induced by hypoxia. In the normoxia condition, HIF1α protein in myocardial cell can be degraded by ubiquitin modification. However, in the hypoxia condition, HIF1α protein in myocardial cell be activated by phosphorylation and methylation modification. Then heart hypoxia related down-stream genes can be cascade activated to induce myocardial cell abnormal proliferation. Finally, cardiac remodeling would be occurred during sustaining chronic hypoxia conditions.
Janus kinase 2 (JAK2) was thought to be involved in pulmonary vascular remodeling in pulmonary hypertension. In response to hypoxia-induced activation, JAK2 phosphorylates signal transduction and transcriptional activator 3 (STAT3), which then binds to the Cyclin A2 (CCNA2) promoter to transcription cyclin A2 expression. And JAK2 inhibitor could alleviate hypoxia-induced proliferation of primary human pulmonary artery smooth muscle cells (PASMC) (82). Endothelial dysfunction plays an integral role in HAHD, and AMPK (AMP-activated protein kinase) and ACE2 (angiotensin-converting enzyme 2) are crucial in endothelial homeostasis. Phosphorylation of ACE2 by AMPK can enhance the stability of ACE2, which increased Ang (angiotensin) 1–7 and endothelial nitric oxide synthase-derived NO bioavailability (83).
Methylation
The best well-known methylations are DNA and histones modifications, mainly involved in epigenetic regulation. In recent years, more and more studies have found that methylation also occurs on non-histone proteins and played an important role in various signal transduction processes. Protein methylation is a multifunctional PTM involving a variety of major cellular processes. Methylation can modulate protein activity, stability, localization, and/or interactions, resulting in specific downstream signaling and biological outcomes. Lysine methylation is a dynamic and fine-tuned process, and its irregularities often lead to human pathology (84). HIF1α and HIF2α are the main regulators of cellular responses to hypoxia. PTMs of HIF1α and 2α are required to regulate their function. The methylation of non-histones by the SET domain containing lysine methyltransferase Set7 is a novel mechanism for regulating the function of cellular proteins under various cellular stresses (85). Xing Liu et al. found that Set7 induced HIF1α methylation at lysine 32 and HIF2α methylation at lysine K29. This methylation inhibited HIF1α/2α expression by interfering with the hypoxia response element of HIFα in the promoter of the HIF target gene (85). Moreover, Xiong et al. found that yaks coped with high altitude hypoxia stress by changing the expression of methylation of hypoxia-inducing factors (86). This adaptation has been observed in highland animals and humans (87–90).
Glycosylation
Protein glycosylation is one of the most complex forms of PTMs. Glycosylation is a process in which sugar chain molecules are transferred to proteins and special amino acid residues on proteins form glycosidic bonds under a series of enzyme regulation actions (91). Glycosyltransferases and glycosidases are responsible for catalyzing the transfer of glycosyl-based residues from donor to recipient proteins and the removal of glucose residues from oligosaccharide complexes. Studies have shown that 70% of human proteins contain one or more sugar chains, and 1% of the human genome is involved in the synthesis and modification of sugar chains. Among the various types of glycosylation identified, O-linked N-acetylglucosamine (O-GlcNAcylation) and N-glycosylation are the two most common types (92, 93).
It is well known that abnormal glycosylation and impaired glycosyltransferase localization and expression are closely related to the occurrence and development of various hypoxia diseases, including high altitude polycythemia (94), hippocampal neuron damage during chronic hypoxia (95), and brain injury from intracerebral hemorrhage (96). Recently, it has been found that the transmembrane glycoprotein receptor CD147 played an important role in the induction of hypoxia-induced cardiac remodeling. Glycosylated CD147 could significantly attenuate stress-induced pathological cardiac remodeling, accompanied by decreased oxidative stress and ferroptosis (97). Arrhythmia is proved be a typical abnormal cardiovascular complication in high altitude. Ufret-vincenty, C. A. investigated the role of ion channels in arrhythmias and eventually found that deficient glycosylation of Na+ channel contributed to Na+ current-dependent arrhythmogenesis in heart failure (98). Moreover, hypoxia-induced pulmonary hypertension is a progressive disease mainly caused by long-term exposure at high altitude (99). Increased pulmonary vascular resistance and pulmonary artery pressure results in enhancement of right ventricular afterload, leading to right heart failure. Further studies have shown that mitochondrial dysfunction played a key role in high-altitude pulmonary hypertension. Protein O-GlcNAcylation protected heart tissues by attenuating the formation of mitochondrial permeability transition pores (mPTP) and subsequent loss of mitochondrial membrane potential (100). Although much progress has been made in the role of glycosylation in abnormal cardiovascular complications at altitude, the role of different glycosyltransferases in HAHD remains to be further investigated.
Sumoylation
Sumoylation is the process of covalently attaching ubiquitin associated modification small molecules to multiple proteins to regulate their function (101). The Sumoylation of proteins has become an important PTM that modulated cellular responses to different types of stress, including hypoxia, cold, and oxidative stress. Previous studies have shown that ubiquitination and deubiquitination were closely related to tumor invasion and escape (102). In colon cancer and breast cancer, tumor suppressor gene P27 is degraded by ubiquitination, which increases tumor aggressiveness. Conversely, deubiquitination stabilizes tumor suppressor genes and thus inhibits tumor progression (103).
In the hypoxia induced pulmonary arterial hypertension mouse model, SUMO1 expression was significantly increased, which was associated with autophagy activation, pulmonary artery vascular smooth muscle cells dedifferentiation, and pulmonary vascular remodeling. Moreover, SUMO1 knockdown reversed hypoxia-induced pulmonary artery vascular smooth muscle cells proliferation and migration (104). Intact mitochondrial homeostasis has been shown to be critical for cardiac systolic function and cardiomyocyte metabolism, and dynamic-associated protein 1 (Drp1) is a key factor in maintaining mitochondrial homeostasis (105). Studies have shown that Drp1 was affected by many PTMs, including ubiquitination. It was found that Drp1 could be ubiquitinated by Parkin, and Parkin targeted Drp1 for proteasome degradation, thereby affecting the process of mitochondrial fission and fusion (106).
Citrullination
Citrullination refers to the conversion of arginine residues to citrulline residues on the protein peptide chain under the action of protein arginine decarboxylase (107). These modifications are most commonly found in rheumatoid arthritis (RA) and cancer (108). Arginine deamination (also known as citrullination) plays a major role in the progression of rheumatoid arthritis by producing autoantibodies and exacerbating inflammatory responses (109, 110). Studies have shown that citrulline regulates cell apoptosis and differentiation, promotes epithelial–mesenchymal transition and metastasis, and the potential application of citrulline antigen in immunotherapy. Citrullination is also used as a cancer biomarker (109, 111). Currently, the role of citrulline modification in abnormal cardiovascular complications at high altitude has not been reported, but we believe that with the further study, the role of citrulline modification in abnormal cardiovascular complications at high altitude will be gradually discovered.
Succinylation
Back in 2010, the University of Chicago team first discovered the PTMs of succinylation of lysine (112). Compared with methylation and acetylation, lysine succinylation induced more changes in protein properties (113). This is because the succinylation is given two negative charges, the valence state changes from + 1 to − 1, which is higher than the charge changes caused by acetylation (+ 1 to 0) and mono-methylation (no change). In addition, succinylation leads to larger group structures that are more capable of altering protein structure and function (112). Meanwhile, succinyl-CoA is a cofactor of the enzyme that regulates succinylation. As an important intermediate product of metabolic reactions, succinyl-CoA appears in tricarboxylic acid (TCA) cycle, the synthesis of porphyrins and the decomposition of some branched amino acids (114, 115). Its stable state is essential for maintaining normal physiological activities of cells. Genetic mutations in succinyl-CoA metabolism are likely to cause diseases. During the neonatal period, significant maturation changes occur in cardiac energy metabolism, from glycolysis to fatty acid oxidation. Acetylation and succinylation of lysine residues are novel PTM that controls cardiac energy metabolism (116). Researchers investigated the effects of protein succinylation on cardiac energy metabolic maturation at 1, 7, and 21 days of age in rabbits. The results showed that the rate of fatty acid β-oxidation increased at 21 days of age, and the rate of glycolysis and glucose oxidation decreased. The degree of acetylation of fatty acid oxidase, long-chain acyl-CoA dehydrogenase and β-hydroxyacyl-CoA dehydrogenase was positively correlated with their activity and fatty acid β-oxidation rate (117). Sadhukhan et al. analyzed acyl-CoA molecules in different mouse tissues and found that different tissues had different acyl-CoA profiles. Succinyl-CoA is the most abundant acyl-CoA molecule in the heart, and the succinylation of myocardial lysine is regulated by SIRT5 (118). Considering the disorder of cardiometabolic at high altitude environments, protein succinylation needs to be further explored.
Lactylation
Lactylation modification is a newly discovered PTM, first described by Zhang et al. and published in Nature (119). Using a bacterial exposed M1 macrophage model system, researchers demonstrated that histone lactation and acetylation have different temporal dynamics. The results suggested that elevated histone lactate levels induced homeostasis genes, including arginase 1, to participate in wound healing during late polarization of M1 macrophages (120). Hideo Hagihara described the presence of lactate modifications in neurons that affected neural excitability (121). High altitude and lack of oxygen also lead to increased glycolysis and lactic acid production. At present, it is not clear whether excessive lactic acid can cause protein lactylation modification. Hence, systematically exploring the proteins lactylation modification at high-altitude hypobaric hypoxic environment could be a promising way to expand the mechanism of abnormal cardiovascular complications at high altitude.
Crotonylation
The Crotonylation of histone lysine residues was first identified as being enriched in promoter and enhancer regions of human male reproductive cells (122). Subsequently, non-histone crotonylation was found to be particularly enriched in nucleoproteins involved in RNA processing, nucleic acid metabolism, and chromosomal tissue (123). More studies identified lysine crotonylation (Kcr) in non-histone proteins (124–126). Kcr is conserved and regulated by a range of enzymes and coenzymes, including lysine crotonyltransferase (writer), lysine decarboxylase (eraser), certain YEATS proteins (reader), and crotonyl-coenzyme A (donor) (127). It is well known that P53 is a tumor suppressor protein that binds to specific DNA sequences and transcriptionally activates target genes to regulate critical cellular processes, including cell cycle control, apoptosis, and DNA repair under genotoxic stress. Meanwhile, P53 is also involved in cardiac remodeling induced by altitude hypoxia (128). These results suggest that PTM of P53 signaling pathway may play an important role in high altitude adaptation response (129).
Therapeutics targeting post-translational modifications in the treatment of high-altitude heart disease
A series of studies revealed the role of PTMs in abnormal cardiovascular complications at high altitude, providing a basis for the further prevention, diagnosis, and treatment of PTMs in altitude heart disease (Figure 2).
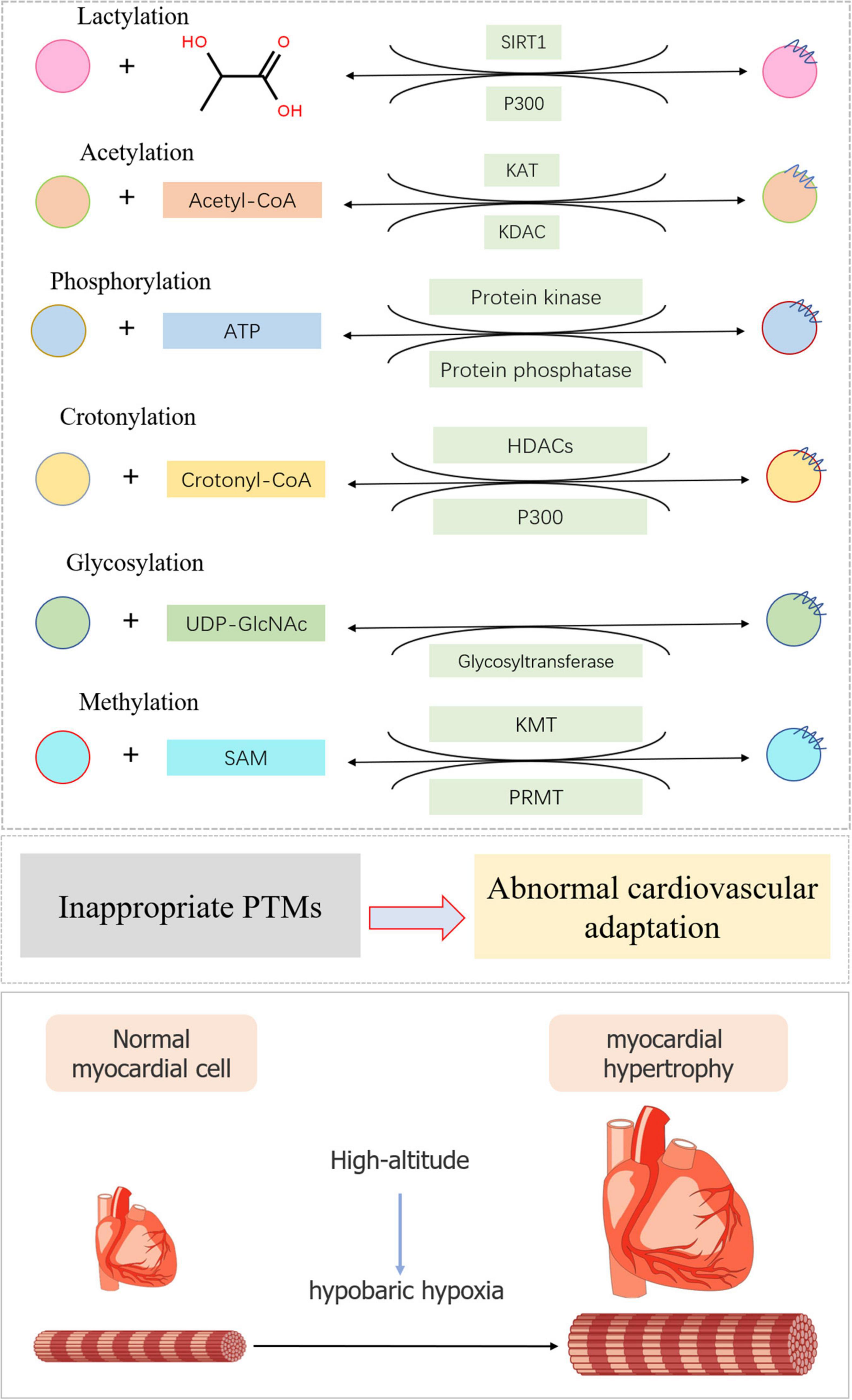
Figure 2. Typical post-translational modifications in high-altitude cardiovascular complications. The substrate and modification enzyme of lactylation, acetylation, phosphorylation, crotonylation, glycosylation, and methylation were exhibited using a diagrammatic plan. When inappropriate PTMs occurred, myocardial cell would become hypertrophic during high-altitude accommodations mainly due to hypobaric hypoxia.
Specifically, analysis of cells and tissues isolated from in vivo preclinical models of pulmonary arterial hypertension and human pulmonary hypertension patients revealed significant changes in the expression levels of various HDACs, SIRT1 and SIRT3 proteins. The roles were demonstrated to be associated with proliferative, inflammatory, and fibrotic phenotypes, and cardiac remodeling processes (59, 61, 69, 130). Due to the reversible ability of PTM acetylation, the efficacy of a number of small molecule inhibitors, such as vorinorestat, valproic acid, sodium butyrate, and resveratrol, have been evaluated in various preclinical models of cardiovascular diseases. This suggests the therapeutic value of targeted histone acetylation pathways in hypoxia-induced pulmonary hypertension and right heart failure induced by pulmonary hypertension (131).
In recent years, high-altitude response caused by short-term intermittent hypoxia exposure has attracted attention again (132). One of the mechanisms of altitude adaptation caused by intermittent hypoxia exposure is proteins PTMs. HIF1 is a major regulator of cellular and systemic oxygen homeostasis. HIF1α is methylated or phosphorylated under intermittent hypoxia induction, activating cardiac genes that encode proteins involved in hypoxia homeostasis response and participate in altitude adaptation. Other types of PTMs, such as methylation, crotonylation, and lactation, have also shown potential therapeutic effects. However, these treatments were still in the research stages and have not been applied to clinical practice.
Conclusion
In this review, we discussed the main types of PTMs in high-altitude cardiovascular adaptation response, including acetylation, phosphorylation, methylation, glycosylation, sumoylation, citrullination, succinylation, lactylation, and crotonylation. Many PTMs have been discovered in recent years, and the key role of PTMs has attracted extensive attention from researchers due to the discovery of enzymology, function and mechanism. In addition, with the advent of new proteomic techniques, new PTMs have been shown to play essential roles in hypoxia-induced. However, the role of PTMs in myocardial remodeling, such as lactylation, citrullination, and crotonylation, has not been fully appreciated and required further investigations. We believe that as research continues, the role of PTMs in abnormal cardiovascular complications at high altitude will become clear.
Author contributions
HD and ZZ conceived and designed the review. JH, SX, and XW analyzed literatures. HL and LC collected the literatures. PL and HL validated the method and literatures. JH and PL wrote this manuscript. All authors read and approved the final manuscript.
Funding
This work was supported by the National Natural Science Foundation of China (81900339 and 82001484), the Fundamental Research Funds for the Central Universities (2682022TPY052 and 2682021TPY031), Sichuan Provincial Science and Technology Department Application Foundation Project (2021YJ0198), Scientific Research Project of Sichuan Provincial Health Commission (20PJ196), Chengdu Science and Technology Project (2019-YF05-01161-SN), and Chengdu High-level Key Clinical Specialty Construction Project.
Conflict of interest
The authors declare that the research was conducted in the absence of any commercial or financial relationships that could be construed as a potential conflict of interest.
Publisher’s note
All claims expressed in this article are solely those of the authors and do not necessarily represent those of their affiliated organizations, or those of the publisher, the editors and the reviewers. Any product that may be evaluated in this article, or claim that may be made by its manufacturer, is not guaranteed or endorsed by the publisher.
Abbreviations
ACE2, Angiotensin-converting enzyme 2; α -SMA, Alpha-smooth muscle actin; Akt, Protein Kinase B; AMPK, AMP-activated protein kinase; Ang, Angiotensin; ATG14, Recombinant Human Autophagy Related 14; COA, Coenzyme A; Drp1, Dynamic-associated protein 1; GSK3, Glycogen synthase kinase 3; HAHD, High-altitude heart disease; HDAC2, Histone Deacetylases 2; HIF1, Hypoxia inducible factor 1; HPH, Hypoxia-induced pulmonary hypertension; Inpp5f, Inositol polyphosphate-5-phosphatase F; IHC, Immunohistochemistry; JAK2, Janus kinase 2; KADC, Lysine Deacetylase; KAT, Lysine acetyltransferase; Kcr, Lysine crotonylation; PTMs, Post-translational modifications; LC3, Light chain 3; mPTP, mitochondrial Permeability Transition Pore; NDDs, Neurodegenerative disorders; NO, Nitric oxide; PASMC, Pulmonary arterial smooth muscle cell; PH, Pulmonary hypertension; PI3K, Phosphatidylinositol 3-kinase; RA, Rheumatoid arthritis; ROS, Reactive oxygen species; SIRT1, Silent mating type information regulation 2 homolog 1; SIRT3, Silent mating type information regulation 2 homolog 3; SIRT5, Silent mating type information regulation 2 homolog 5; SM22, Smooth muscle 22; SM-MHC, Smooth muscle-Major histocompatibility complex; STAT3, Signal transduction and transcriptional activator 3; SUMO, Small ubiquitin-associated modified molecules; TAC, Tricarboxylic acid cycle; TP, Triptolide; VSMCs, Vascular smooth muscle cells.
References
1. Ostadal B, Kolar F. Cardiac adaptation to chronic high-altitude hypoxia: beneficial and adverse effects. Respir Physiol Neurobiol. (2007) 158:224–36. doi: 10.1016/j.resp.2007.03.005
2. Zheng C, Wang X, Tang H, Chen Z, Zhang L, Wang S, et al. Habitation altitude and left ventricular diastolic function: a population-based study. J Am Heart Assoc. (2021) 10:e018079. doi: 10.1161/JAHA.120.018079
3. Betge S, Drinda S, Neumann T, Baz L, Pfeil A, Schulze C, et al. Influence of macitentan on the vascular tone and recruitment of finger capillaries under hypobaric hypoxia in high altitude. High Alt Med Biol. (2020) 21:336–45. doi: 10.1089/ham.2019.0120
4. Sarkar S, Banerjee PK, Selvamurthy W. High altitude hypoxia: an intricate interplay of oxygen responsive macroevents and micromolecules. Mol Cell Biochem. (2003) 253:287–305. doi: 10.1023/a:1026080320034
5. Jha KN. High Altitude and the Eye. Asia Pac J Ophthalmol. (2012) 1:166–9. doi: 10.1097/APO.0b013e318253004e
6. Liu G, Zhao L, Xu Q, Lang M, Xiao R. Cardiac adaptation to high altitudes after short- and long-term exposure among Chinese Han lowlanders. Echocardiography. (2022) 39:465–72. doi: 10.1111/echo.15317
7. Qian Z, Fan A, Dawa, Dawaciren, Pan B. Retrospective cohort analysis of heart rate variability in patients with high altitude pulmonary hypertension in Tibet. Clin Cardiol. (2020) 43:298–304. doi: 10.1002/clc.23312
8. Pieroni L, Iavarone F, Olianas A, Greco V, Desiderio C, Martelli C, et al. Enrichments of post-translational modifications in proteomic studies. J Sep Sci. (2020) 43:313–36. doi: 10.1002/jssc.201900804
9. Vanheule V, Metzemaekers M, Janssens R, Struyf S, Proost P. How post-translational modifications influence the biological activity of chemokines. Cytokine. (2018) 109:29–51. doi: 10.1016/j.cyto.2018.02.026
10. Daneri-Becerra C, Zgajnar NR, Lotufo CM, Ramos Hryb AB, Piwien-Pilipuk G, Galigniana MD. Regulation of FKBP51 and FKBP52 functions by post-translational modifications. Biochem Soc Trans. (2019) 47:1815–31. doi: 10.1042/BST20190334
11. Muller MM. Post-translational modifications of protein backbones: unique functions, mechanisms, and challenges. Biochemistry. (2018) 57:177–85. doi: 10.1021/acs.biochem.7b00861
12. Padmanabhan A, Rao V, De Marzo AM, Bieberich CJ. Regulating NKX3.1 stability and function: post-translational modifications and structural determinants. Prostate. (2016) 76:523–33. doi: 10.1002/pros.23144
13. Klotz LO, Sanchez-Ramos C, Prieto-Arroyo I, Urbanek P, Steinbrenner H, Monsalve M. Redox regulation of FoxO transcription factors. Redox Biol. (2015) 6:51–72. doi: 10.1016/j.redox.2015.06.019
14. Stastna M, Van Eyk JE. Posttranslational modifications of lysine and evolving role in heart pathologies-recent developments. Proteomics. (2015) 15:1164–80. doi: 10.1002/pmic.201400312
15. Dhar P, Sharma VK, Hota KB, Das SK, Hota SK, Srivastava RB, et al. Autonomic cardiovascular responses in acclimatized lowlanders on prolonged stay at high altitude: a longitudinal follow up study. PLoS One. (2014) 9:e84274. doi: 10.1371/journal.pone.0084274
16. Willmann G, Gekeler F, Schommer K, Bartsch P. Update on high altitude cerebral edema including recent work on the eye. High Alt Med Biol. (2014) 15:112–22. doi: 10.1089/ham.2013.1142
17. Luks AM, Swenson ER. Evaluating the risks of high altitude travel in chronic liver disease patients. High Alt Med Biol. (2015) 16:80–8. doi: 10.1089/ham.2014.1122
18. Gonzales GF, Tapia V. Increased levels of serum gamma-glutamyltransferase and uric acid on metabolic, hepatic and kidney parameters in subjects at high altitudes. J Basic Clin Physiol Pharmacol. (2015) 26:81–7. doi: 10.1515/jbcpp-2013-0162
19. Kayser B. Nutrition and high altitude exposure. Int J Sports Med. (1992) 13:S129–32. doi: 10.1055/s-2007-1024616
20. Zhang W, Jiao L, Liu R, Zhang Y, Ji Q, Zhang H, et al. The effect of exposure to high altitude and low oxygen on intestinal microbial communities in mice. PLoS One. (2018) 13:e0203701. doi: 10.1371/journal.pone.0203701
21. Falla M, Papagno C, Dal Cappello T, Vogele A, Hufner K, Kim J, et al. A prospective evaluation of the acute effects of high altitude on cognitive and physiological functions in lowlanders. Front Physiol. (2021) 12:670278. doi: 10.3389/fphys.2021.670278
22. Algaze I, Phillips L, Inglis P, Lathrop G, Gadbois J, Rizzolo K, et al. Incidence of mild cognitive impairment with ascending altitude. High Alt Med Biol. (2020) 21:184–91. doi: 10.1089/ham.2019.0111
23. Wilson MH, Davagnanam I, Holland G, Dattani RS, Tamm A, Hirani SP, et al. Cerebral venous system and anatomical predisposition to high-altitude headache. Ann Neurol. (2013) 73:381–9. doi: 10.1002/ana.23796
24. Vats P, Singh VK, Singh SN, Singh SB. High altitude induced anorexia: effect of changes in leptin and oxidative stress levels. Nutr Neurosci. (2007) 10:243–9. doi: 10.1080/10284150701722299
25. Davis C, Hackett P. Advances in the prevention and treatment of high altitude illness. Emerg Med Clin North Am. (2017) 35:241–60. doi: 10.1016/j.emc.2017.01.002
26. Shen Y, Yang YQ, Liu C, Yang J, Zhang JH, Jin J, et al. Association between physiological responses after exercise at low altitude and acute mountain sickness upon ascent is sex-dependent. Mil Med Res. (2020) 7:53. doi: 10.1186/s40779-020-00283-3
27. Stembridge M, Ainslie PN, Shave R. Short-term adaptation and chronic cardiac remodelling to high altitude in lowlander natives and Himalayan Sherpa. Exp Physiol. (2015) 100:1242–6. doi: 10.1113/expphysiol.2014.082503
28. Stembridge M, Ainslie PN, Shave R. Mechanisms underlying reductions in stroke volume at rest and during exercise at high altitude. Eur J Sport Sci. (2016) 16:577–84. doi: 10.1080/17461391.2015.1071876
29. Ke J, Yang J, Liu C, Qin Z, Zhang J, Jin J, et al. A novel echocardiographic parameter to identify individuals susceptible to acute mountain sickness. Travel Med Infect Dis. (2021) 44:102166. doi: 10.1016/j.tmaid.2021.102166
30. Chen R, Ye X, Sun M, Yang J, Zhang J, Gao X, et al. Blood pressure load: an effective indicator of systemic circulation status in individuals with acute altitude sickness. Front Cardiovasc Med. (2021) 8:765422. doi: 10.3389/fcvm.2021.765422
31. Yang Y, Liu C, Yu S, Qin Z, Yang J, Bian S, et al. Pulmonary function tests at low altitude predict pulmonary pressure response to short-term high altitude exposure. Respir Physiol Neurobiol. (2020) 282:103534. doi: 10.1016/j.resp.2020.103534
32. Genovese A, Accinni A, Spadaro G, Quattrin S, Condorelli M. Myocardial hypertrophy in rats exposed to simulated high altitude. Arch Int Physiol Biochim. (1985) 93:331–8. doi: 10.3109/13813458509079614
33. Wang DR. [Histopathological and ultrastructural changes in 57 cases of high altitude heart disease]. Zhonghua Bing Li Xue Za Zhi. (1992) 21: 302–4.
34. Gonzalez-Candia A, Arias PV, Aguilar SA, Figueroa EG, Reyes RV, Ebensperger G, et al. Melatonin reduces oxidative stress in the right ventricle of newborn sheep gestated under chronic hypoxia. Antioxidants. (2021) 10:1658. doi: 10.3390/antiox10111658
35. Jain K, Suryakumar G, Prasad R, Singh SN, Ganju L. Myocardial ER chaperone activation and protein degradation occurs due to synergistic, not individual, cold and hypoxic stress. Biochimie. (2013) 95:1897–908. doi: 10.1016/j.biochi.2013.06.018
36. Chen L, Kashina A. Post-translational modifications of the protein termini. Front Cell Dev Biol. (2021) 9:719590. doi: 10.3389/fcell.2021.719590
37. Agatemor C, Middleton SAD, Toledo D. How pervasive are post-translational and -transcriptional modifications? Trends Cell Biol. (2022) 32:475–8. doi: 10.1016/j.tcb.2021.11.002
38. Zhang W, Sun J, Cao H, Tian R, Cai L, Ding W, et al. Post-translational modifications are enriched within protein functional groups important to bacterial adaptation within a deep-sea hydrothermal vent environment. Microbiome. (2016) 4:49. doi: 10.1186/s40168-016-0194-x
39. Mueller TM, Meador-Woodruff JH. Post-translational protein modifications in schizophrenia. NPJ Schizophr. (2020) 6:5. doi: 10.1038/s41537-020-0093-9
40. Sostaric N, van Noort V. Molecular dynamics shows complex interplay and long-range effects of post-translational modifications in yeast protein interactions. PLoS Comput Biol. (2021) 17:e1008988. doi: 10.1371/journal.pcbi.1008988
41. Mishra A, Mohammad G, Norboo T, Newman JH, Pasha MA. Lungs at high-altitude: genomic insights into hypoxic responses. J Appl Physiol. (2015) 119:1–15. doi: 10.1152/japplphysiol.00513.2014
42. Tsai SH, Huang PH, Tsai HY, Hsu YJ, Chen YW, Wang JC, et al. Roles of the hypoximir microRNA-424/322 in acute hypoxia and hypoxia-induced pulmonary vascular leakage. FASEB J. (2019) 33:12565–75. doi: 10.1096/fj.201900564RR
43. Dada LA, Chandel NS, Ridge KM, Pedemonte C, Bertorello AM, Sznajder JI. Hypoxia-induced endocytosis of Na, K-ATPase in alveolar epithelial cells is mediated by mitochondrial reactive oxygen species and PKC-zeta. J Clin Invest. (2003) 111:1057–64. doi: 10.1172/JCI16826
44. Qin Y, Qiao Y, Li L, Luo E, Wang D, Yao Y, et al. The m(6)A methyltransferase METTL3 promotes hypoxic pulmonary arterial hypertension. Life Sci. (2021) 274:119366. doi: 10.1016/j.lfs.2021.119366
45. Dassanayaka S, Jones SP. O-GlcNAc and the cardiovascular system. Pharmacol Ther. (2014) 142:62–71.
46. Yang Q, Lu Z, Ramchandran R, Longo LD, Raj JU. Pulmonary artery smooth muscle cell proliferation and migration in fetal lambs acclimatized to high-altitude long-term hypoxia: role of histone acetylation. Am J Physiol Lung Cell Mol Physiol. (2012) 303:L1001–10. doi: 10.1152/ajplung.00092.2012
47. Sartori C, Rimoldi SF, Rexhaj E, Allemann Y, Scherrer U. Epigenetics in cardiovascular regulation. Adv Exp Med Biol. (2016) 903:55–62. doi: 10.1007/978-1-4899-7678-9_4
48. Reyes RV, Diaz M, Ebensperger G, Herrera EA, Quezada SA, Hernandez I, et al. The role of nitric oxide in the cardiopulmonary response to hypoxia in highland and lowland newborn llamas. J Physiol. (2018) 596:5907–23. doi: 10.1113/JP274340
49. Gao Y, Portugal AD, Negash S, Zhou W, Longo LD, Usha Raj J. Role of Rho kinases in PKG-mediated relaxation of pulmonary arteries of fetal lambs exposed to chronic high altitude hypoxia. Am J Physiol Lung Cell Mol Physiol. (2007) 292:L678–84. doi: 10.1152/ajplung.00178.2006
50. Panzhinskiy E, Zawada WM, Stenmark KR, Das M. Hypoxia induces unique proliferative response in adventitial fibroblasts by activating PDGFbeta receptor-JNK1 signalling. Cardiovasc Res. (2012) 95:356–65. doi: 10.1093/cvr/cvs194
51. Pooja Ghosh D, Bhargava K, Sethy NK. Post-translational modifications of eNOS augment nitric oxide availability and facilitates hypoxia adaptation in Ladakhi women. Nitric Oxide. (2018) 78:103–12. doi: 10.1016/j.niox.2018.06.003
52. Semenza GL. The genomics and genetics of oxygen homeostasis. Annu Rev Genomics Hum Genet. (2020) 21:183–204. doi: 10.1146/annurev-genom-111119-073356
53. Zhou XL, Huang FJ, Li Y, Huang H, Wu QC. SEDT2/METTL14-mediated m6A methylation awakening contributes to hypoxia-induced pulmonary arterial hypertension in mice. Aging. (2021) 13:7538–48. doi: 10.18632/aging.202616
54. Suzuki YJ, Shults NV. Redox signaling in the right ventricle. Adv Exp Med Biol. (2017) 967:315–23. doi: 10.1007/978-3-319-63245-2_19
55. Espinosa L, Chouabe C, Morales A, Lachuer J, Georges B, Fatemi M, et al. Increased sodium-calcium exchange current in right ventricular cell hypertrophy induced by simulated high altitude in adult rats. J Mol Cell Cardiol. (2000) 32:639–53. doi: 10.1006/jmcc.2000.1106
56. Park AM, Wong CM, Jelinkova L, Liu L, Nagase H, Suzuki YJ. Pulmonary hypertension-induced GATA4 activation in the right ventricle. Hypertension. (2010) 56:1145–51. doi: 10.1161/HYPERTENSIONAHA.110.160515
57. Ning XH, Chen SH. Mild hypothermic cross adaptation resists hypoxic injury in hearts: a brief review. Chin J Physiol. (2006) 49:213–22.
58. Liu H, Bian X, Xu M, Ma X, Zhang C, Jiang J, et al. HIF1-alpha protein SUMOylation is an important protective mechanism of action of hypothermia in hypoxic cardiomyocytes. Mol Med Rep. (2021) 23:476. doi: 10.3892/mmr.2021.12115
59. Qin J, Guo N, Tong J, Wang Z. Function of histone methylation and acetylation modifiers in cardiac hypertrophy. J Mol Cell Cardiol. (2021) 159:120–9. doi: 10.1016/j.yjmcc.2021.06.011
60. Drazic A, Myklebust LM, Ree R, Arnesen T. The world of protein acetylation. Biochim Biophys Acta. (2016) 1864:1372–401. doi: 10.1016/j.bbapap.2016.06.007
61. Hao L, Park J, Jang HY, Bae EJ, Park BH. Inhibiting protein kinase activity of pyruvate kinase M2 by SIRT2 deacetylase attenuates psoriasis. J Invest Dermatol. (2021) 141:355–363.e6. doi: 10.1016/j.jid.2020.06.024
62. Lin Q, Zuo W, Liu Y, Wu K, Liu Q. NAD(+) and cardiovascular diseases. Clin Chim Acta. (2021) 515:104–10.
63. Winnik S, Auwerx J, Sinclair DA, Matter CM. Protective effects of sirtuins in cardiovascular diseases: from bench to bedside. Eur Heart J. (2015) 36:3404–12. doi: 10.1093/eurheartj/ehv290
64. Xu S, Bai P, Jin ZG. Sirtuins in cardiovascular health and diseases. Trends Endocrinol Metab. (2016) 27:677–8. doi: 10.1016/j.tem.2016.07.004
65. Yu L, Tu Y, Jia X, Fang K, Liu L, Wan L, et al. Resveratrol protects against pulmonary arterial hypertension in rats via activation of silent information regulator 1. Cell Physiol Biochem. (2017) 42:55–67. doi: 10.1159/000477115
66. Xi L, Ruan L, Yao X, Zhang D, Yuan H, Li Q, et al. SIRT1 promotes pulmonary artery endothelial cell proliferation by targeting the Akt signaling pathway. Exp Ther Med. (2020) 20:179. doi: 10.3892/etm.2020.9309
67. Kononova VA, Vtiurin BV. [Functional morphology of myocardial ultrastructure during prolonged adaptation to pressure chamber hypoxia]. Biull Eksp Biol Med. (1980) 90:616–9.
68. Parodi-Rullan RM, Chapa-Dubocq XR, Javadov S. Acetylation of mitochondrial proteins in the heart: the role of SIRT3. Front Physiol. (2018) 9:1094. doi: 10.3389/fphys.2018.01094
69. Yang Y, Wang W, Xiong Z, Kong J, Qiu Y, Shen F, et al. Activation of SIRT3 attenuates triptolide-induced toxicity through closing mitochondrial permeability transition pore in cardiomyocytes. Toxicol In Vitro. (2016) 34:128–37. doi: 10.1016/j.tiv.2016.03.020
70. Sundaresan NR, Gupta M, Kim G, Rajamohan SB, Isbatan A, Gupta MP. Sirt3 blocks the cardiac hypertrophic response by augmenting Foxo3a-dependent antioxidant defense mechanisms in mice. J Clin Invest. (2009) 119:2758–71. doi: 10.1172/JCI39162
71. Ge RL, Ma RY, Bao HH, Zhao XP, Qi HN. Changes of cardiac structure and function in pediatric patients with high altitude pulmonary hypertension in Tibet. High Alt Med Biol. (2009) 10:247–52. doi: 10.1089/ham.2009.0001
72. Chouabe C, Espinosa L, Megas P, Chakir A, Rougier O, Freminet A, et al. Reduction of I(Ca,L) and I(to1) density in hypertrophied right ventricular cells by simulated high altitude in adult rats. J Mol Cell Cardiol. (1997) 29:193–206. doi: 10.1006/jmcc.1996.0264
73. Kee HJ, Kook H. Roles and targets of class I and IIa histone deacetylases in cardiac hypertrophy. J Biomed Biotechnol. (2011) 2011:928326. doi: 10.1155/2011/928326
74. Yoon S, Kim M, Min HK, Lee YU, Kwon DH, Lee M, et al. Inhibition of heat shock protein 70 blocks the development of cardiac hypertrophy by modulating the phosphorylation of histone deacetylase 2. Cardiovasc Res. (2019) 115:1850–60. doi: 10.1093/cvr/cvy317
75. Zhao L, Chen CN, Hajji N, Oliver E, Cotroneo E, Wharton J, et al. Histone deacetylation inhibition in pulmonary hypertension: therapeutic potential of valproic acid and suberoylanilide hydroxamic acid. Circulation. (2012) 126:455–67.
76. Seok SH. Structural insights into protein regulation by phosphorylation and substrate recognition of protein kinases/phosphatases. Life. (2021) 11:957. doi: 10.3390/life11090957
77. Humphrey SJ, James DE, Mann M. Protein phosphorylation: a major switch mechanism for metabolic regulation. Trends Endocrinol Metab. (2015) 26:676–87. doi: 10.1016/j.tem.2015.09.013
78. Hirschler V. Cardiometabolic risk factors in native populations living at high altitudes. Int J Clin Pract. (2016) 70:113–8. doi: 10.1111/ijcp.12756
79. Downes NL, Laham-Karam N, Kaikkonen MU, Yla-Herttuala S. Differential but complementary HIF1alpha and HIF2alpha transcriptional regulation. Mol Ther. (2018) 26:1735–45.
80. Hopfl G, Ogunshola O, Gassmann M. Hypoxia and high altitude. The molecular response. Adv Exp Med Biol. (2003) 543:89–115. doi: 10.1007/978-1-4419-8997-0_7
81. Zagorska A, Dulak J. HIF-1: the knowns and unknowns of hypoxia sensing. Acta Biochim Pol. (2004) 51:563–85. doi: 10.18388/abp.2004_3545
82. Zhang L, Wang Y, Wu G, Rao L, Wei Y, Yue H, et al. Blockade of JAK2 protects mice against hypoxia-induced pulmonary arterial hypertension by repressing pulmonary arterial smooth muscle cell proliferation. Cell Prolif. (2020) 53:e12742. doi: 10.1111/cpr.12742
83. Zhang J, Dong J, Martin M, He M, Gongol B, Marin TL, et al. AMP-activated protein kinase phosphorylation of angiotensin-converting enzyme 2 in endothelium mitigates pulmonary hypertension. Am J Respir Crit Care Med. (2018) 198:509–20.
84. Tiebe M, Lutz M, Levy D, Teleman AA. Phenotypic characterization of SETD3 knockout Drosophila. PLoS One. (2018) 13:e0201609. doi: 10.1371/journal.pone.0201609
85. Liu X, Chen Z, Xu C, Leng X, Cao H, Ouyang G, et al. Repression of hypoxia-inducible factor alpha signaling by Set7-mediated methylation. Nucleic Acids Res. (2015) 43:5081–98. doi: 10.1093/nar/gkv379
86. Xiong X, Fu M, Lan D, Li J, Zi X, Zhong J. Yak response to high-altitude hypoxic stress by altering mRNA expression and DNA methylation of hypoxia-inducible factors. Anim Biotechnol. (2015) 26:222–9. doi: 10.1080/10495398.2014.1002563
87. Childebayeva A, Jones TR, Goodrich JM, Leon-Velarde F, Rivera-Chira M, Kiyamu M, et al. LINE-1 and EPAS1 DNA methylation associations with high-altitude exposure. Epigenetics. (2019) 14:1–15.
88. Zhang Z, Du H, Bai L, Yang C, Li Q, Li X, et al. Whole genome bisulfite sequencing reveals unique adaptations to high-altitude environments in Tibetan chickens. PLoS One. (2018) 13:e0193597. doi: 10.1371/journal.pone.0193597
89. Childebayeva A, Harman T, Weinstein J, Day T, Brutsaert TD, Bigham AW. Genome-wide DNA methylation changes associated with high-altitude acclimatization during an Everest base camp trek. Front Physiol. (2021) 12:660906. doi: 10.3389/fphys.2021.660906
90. Zhang B, Ban D, Gou X, Zhang Y, Yang L, Chamba Y, et al. Genome-wide DNA methylation profiles in Tibetan and Yorkshire pigs under high-altitude hypoxia. J Anim Sci Biotechnol. (2019) 10:25. doi: 10.1186/s40104-019-0316-y
91. van Kasteren SI, Kramer HB, Gamblin DP, Davis BG. Site-selective glycosylation of proteins: creating synthetic glycoproteins. Nat Protoc. (2007) 2:3185–94. doi: 10.1038/nprot.2007.430
92. Lira-Navarrete E, Hurtado-Guerrero R. A perspective on structural and mechanistic aspects of protein O-fucosylation. Acta Crystallogr F Struct Biol Commun. (2018) 74:443–50. doi: 10.1107/S2053230X18004788
93. Ma C, Qu J, Meisner J, Zhao X, Li X, Wu Z, et al. Convenient and precise strategy for mapping n-glycosylation sites using microwave-assisted acid hydrolysis and characteristic ions recognition. Anal Chem. (2015) 87:7833–9. doi: 10.1021/acs.analchem.5b02177
94. Lundby AK, Keiser S, Siebenmann C, Schaffer L, Lundby C. Kidney-synthesized erythropoietin is the main source for the hypoxia-induced increase in plasma erythropoietin in adult humans. Eur J Appl Physiol. (2014) 114:1107–11. doi: 10.1007/s00421-014-2844-7
95. Sharma D, Barhwal KK, Biswal SN, Srivastava AK, Bhardwaj P, Kumar A, et al. Hypoxia-mediated alteration in cholesterol oxidation and raft dynamics regulates BDNF signalling and neurodegeneration in hippocampus. J Neurochem. (2019) 148:238–51. doi: 10.1111/jnc.14609
96. Yang F, Wang Z, Zhang JH, Tang J, Liu X, Tan L, et al. Receptor for advanced glycation end-product antagonist reduces blood-brain barrier damage after intracerebral hemorrhage. Stroke. (2015) 46:1328–36. doi: 10.1161/STROKEAHA.114.008336
97. Zhong FY, Zhao YC, Zhao CX, Gu ZC, Lu XY, Jiang WL, et al. The role of CD147 in pathological cardiac hypertrophy is regulated by glycosylation. Oxid Med Cell Longev. (2022) 2022:6603296. doi: 10.1155/2022/6603296
98. Ufret-Vincenty CA, Baro DJ, Lederer WJ, Rockman HA, Quinones LE, Santana LF. Role of sodium channel deglycosylation in the genesis of cardiac arrhythmias in heart failure. J Biol Chem. (2001) 276:28197–203. doi: 10.1074/jbc.M102548200
99. Pan M, Han Y, Si R, Guo R, Desai A, Makino A. Hypoxia-induced pulmonary hypertension in type 2 diabetic mice. Pulm Circ. (2017) 7:175–85. doi: 10.1086/690206
100. Ngoh GA, Watson LJ, Facundo HT, Jones SP. Augmented O-GlcNAc signaling attenuates oxidative stress and calcium overload in cardiomyocytes. Amino Acids. (2011) 40:895–911. doi: 10.1007/s00726-010-0728-7
101. Eifler K, Vertegaal ACO. SUMOylation-mediated regulation of cell cycle progression and cancer. Trends Biochem Sci. (2015) 40:779–93. doi: 10.1016/j.tibs.2015.09.006
102. Rabellino A, Khanna KK. The implication of the SUMOylation pathway in breast cancer pathogenesis and treatment. Crit Rev Biochem Mol Biol. (2020) 55:54–70. doi: 10.1080/10409238.2020.1738332
103. Lee JS, Choi HJ, Baek SH. Sumoylation and Its Contribution to Cancer. Adv Exp Med Biol. (2017) 963:283–98. doi: 10.1007/978-3-319-50044-7_17
104. Yao Y, Li H, Da X, He Z, Tang B, Li Y, et al. SUMOylation of Vps34 by SUMO1 promotes phenotypic switching of vascular smooth muscle cells by activating autophagy in pulmonary arterial hypertension. Pulm Pharmacol Ther. (2019) 55:38–49. doi: 10.1016/j.pupt.2019.01.007
105. Jin JY, Wei XX, Zhi XL, Wang XH, Meng D. Drp1-dependent mitochondrial fission in cardiovascular disease. Acta Pharmacol Sin. (2021) 42:655–64. doi: 10.1038/s41401-020-00518-y
106. Qi Z, Huang Z, Xie F, Chen L. Dynamin-related protein 1: a critical protein in the pathogenesis of neural system dysfunctions and neurodegenerative diseases. J Cell Physiol. (2019) 234:10032–46. doi: 10.1002/jcp.27866
107. Xu H, Wu M, Ma X, Huang W, Xu Y. Function and mechanism of novel histone posttranslational modifications in health and disease. Biomed Res Int. (2021) 2021:6635225.
108. Giles JT, Fert-Bober J, Park JK, Bingham CO III, Andrade F, Fox-Talbot K, et al. Myocardial citrullination in rheumatoid arthritis: a correlative histopathologic study. Arthritis Res Ther. (2012) 14:R39. doi: 10.1186/ar3752
109. Yuzhalin AE. Citrullination in cancer. Cancer Res. (2019) 79:1274–84. doi: 10.1158/0008-5472.CAN-18-2797
110. Darrah E, Andrade F. Rheumatoid arthritis and citrullination. Curr Opin Rheumatol. (2018) 30:72–8. doi: 10.1097/BOR.0000000000000452
111. Zhu D, Zhang Y, Wang S. Histone citrullination: a new target for tumors. Mol Cancer. (2021) 20:90. doi: 10.1186/s12943-021-01373-z
112. Zhang Z, Tan M, Xie Z, Dai L, Chen Y, Zhao Y. Identification of lysine succinylation as a new post-translational modification. Nat Chem Biol. (2011) 7:58–63. doi: 10.1038/nchembio.495
113. Weinert BT, Scholz C, Wagner SA, Iesmantavicius V, Su D, Daniel JA, et al. Lysine succinylation is a frequently occurring modification in prokaryotes and eukaryotes and extensively overlaps with acetylation. Cell Rep. (2013) 4:842–51. doi: 10.1016/j.celrep.2013.07.024
114. Di Pierro E, Granata F. Nutrients and porphyria: an intriguing crosstalk. Int J Mol Sci. (2020) 21:3462. doi: 10.3390/ijms21103462
115. Zhao Y, Han Y, Sun Y, Wei Z, Chen J, Niu X, et al. Comprehensive succinylome profiling reveals the pivotal role of lysine succinylation in energy metabolism and quorum sensing of Staphylococcus epidermidis. Front Microbiol. (2020) 11:632367. doi: 10.3389/fmicb.2020.632367
116. Yang L, Miao S, Zhang J, Wang P, Liu G, Wang J. The growing landscape of succinylation links metabolism and heart disease. Epigenomics. (2021) 13:319–33. doi: 10.2217/epi-2020-0273
117. Fukushima A, Alrob OA, Zhang L, Wagg CS, Altamimi T, Rawat S, et al. Acetylation and succinylation contribute to maturational alterations in energy metabolism in the newborn heart. Am J Physiol Heart Circ Physiol. (2016) 311:H347–63. doi: 10.1152/ajpheart.00900.2015
118. Sadhukhan S, Liu X, Ryu D, Nelson OD, Stupinski JA, Li Z, et al. Metabolomics-assisted proteomics identifies succinylation and SIRT5 as important regulators of cardiac function. Proc Natl Acad Sci USA. (2016) 113:4320–5. doi: 10.1073/pnas.1519858113
119. Notarangelo G, Haigis MC. Sweet temptation: from sugar metabolism to gene regulation. Immunity. (2019) 51:980–1. doi: 10.1016/j.immuni.2019.11.008
120. Zhang D, Tang Z, Huang H, Zhou G, Cui C, Weng Y, et al. Metabolic regulation of gene expression by histone lactylation. Nature. (2019) 574:575–80. doi: 10.1038/s41586-019-1678-1
121. Hagihara H, Shoji H, Otabi H, Toyoda A, Katoh K, Namihira M, et al. Protein lactylation induced by neural excitation. Cell Rep. (2021) 37:109820. doi: 10.1016/j.celrep.2021.109820
122. Tan M, Luo H, Lee S, Jin F, Yang JS, Montellier E, et al. Identification of 67 histone marks and histone lysine crotonylation as a new type of histone modification. Cell. (2011) 146:1016–28. doi: 10.1016/j.cell.2011.08.008
123. Wei W, Mao A, Tang B, Zeng Q, Gao S, Liu X, et al. Large-scale identification of protein crotonylation reveals its role in multiple cellular functions. J Proteome Res. (2017) 16:1743–52. doi: 10.1021/acs.jproteome.7b00012
124. Sun H, Liu X, Li F, Li W, Zhang J, Xiao Z, et al. First comprehensive proteome analysis of lysine crotonylation in seedling leaves of Nicotiana tabacum. Sci Rep. (2017) 7:3013. doi: 10.1038/s41598-017-03369-6
125. Xu W, Wan J, Zhan J, Li X, He H, Shi Z, et al. Global profiling of crotonylation on non-histone proteins. Cell Res. (2017) 27:946–9. doi: 10.1038/cr.2017.60
126. Suzuki Y, Horikoshi N, Kato D, Kurumizaka H. Crystal structure of the nucleosome containing histone H3 with crotonylated lysine 122. Biochem Biophys Res Commun. (2016) 469:483–9. doi: 10.1016/j.bbrc.2015.12.041
127. Hou JY, Zhou L, Li JL, Wang DP, Cao JM. Emerging roles of non-histone protein crotonylation in biomedicine. Cell Biosci. (2021) 11:101. doi: 10.1186/s13578-021-00616-2
128. Sharma P, Bansal A, Sharma PC. RNA-seq-based transcriptome profiling reveals differential gene expression in the lungs of Sprague-Dawley rats during early-phase acute hypobaric hypoxia. Mol Genet Genomics. (2015) 290:2225–40. doi: 10.1007/s00438-015-1064-0
129. Feng S, Ma J, Long K, Zhang J, Qiu W, Li Y, et al. Comparative microRNA transcriptomes in domestic goats reveal acclimatization to high altitude. Front Genet. (2020) 11:809. doi: 10.3389/fgene.2020.00809
130. Gorski PA, Jang SP, Jeong D, Lee A, Lee P, Oh JG, et al. Role of SIRT1 in modulating acetylation of the sarco-endoplasmic reticulum Ca(2+)-ATPase in heart failure. Circ Res. (2019) 124:e63–80. doi: 10.1161/CIRCRESAHA.118.313865
131. Chelladurai P, Boucherat O, Stenmark K, Kracht M, Seeger W, Bauer UM, et al. Targeting histone acetylation in pulmonary hypertension and right ventricular hypertrophy. Br J Pharmacol. (2021) 178:54–71. doi: 10.1111/bph.14932
Keywords: protein post-translational modifications (PTMs), hypobaric hypoxia, cardiovascular complications adverse events, high-altitude pulmonary edema (HAPE), lactylation modification
Citation: Hou J, Wen X, Long P, Xiong S, Liu H, Cai L, Deng H and Zhang Z (2022) The role of post-translational modifications in driving abnormal cardiovascular complications at high altitude. Front. Cardiovasc. Med. 9:886300. doi: 10.3389/fcvm.2022.886300
Received: 28 February 2022; Accepted: 16 June 2022;
Published: 14 September 2022.
Edited by:
Lan Huang, Xinqiao Hospital, ChinaReviewed by:
Shi-Zhu Bian, Xinqiao Hospital, ChinaRahul Kumar, University of California, San Francisco, United States
Copyright © 2022 Hou, Wen, Long, Xiong, Liu, Cai, Deng and Zhang. This is an open-access article distributed under the terms of the Creative Commons Attribution License (CC BY). The use, distribution or reproduction in other forums is permitted, provided the original author(s) and the copyright owner(s) are credited and that the original publication in this journal is cited, in accordance with accepted academic practice. No use, distribution or reproduction is permitted which does not comply with these terms.
*Correspondence: Lin Cai, clin63@hotmail.com; Haoyu Deng, eric.deng@hli.ubc.ca; Zhen Zhang, peakhell@163.com
†These authors have contributed equally to this work