Carbon Isotope Chemostratigraphy Across the Permian-Triassic Boundary at Chaotian, China: Implications for the Global Methane Cycle in the Aftermath of the Extinction
- 1Institut des Sciences de la Terre, Université de Lausanne, Lausanne, Switzerland
- 2Research and Development (R&D) Center for Submarine Resources, Japan Agency for Marine-Earth Science and Technology (JAMSTEC), Yokosuka, Japan
- 3Earth-Life Science Institute, Tokyo Institute of Technology, Tokyo, Japan
- 4Department of Earth Science and Astronomy, The University of Tokyo, Tokyo, Japan
During the end-Permian extinction, a substantial amount of methane (CH4) was likely released into the ocean-atmosphere system associated with the Siberian Traps volcanism, although fluctuations in the global CH4 cycle in the aftermath of the extinction remain poorly understood. The carbon (C) isotopic composition of carbonate (δ13Ccarb) across the Permian-Triassic boundary (P-TB) was analyzed at Chaotian, South China. The δ13Ccarb values decrease from ca. +1 to –2‰ across the P-TB, possibly caused by a collapse of primary productivity associated with the shallow-marine extinction. The frequent intercalation of felsic tuff layers around the P-TB suggests that a volcanogenic carbon dioxide (CO2) input to the surface oceans may also have contributed to the δ13Ccarb decline. The magnitude of the δ13Ccarb decrease (∼3‰) is substantially smaller than the magnitude of a decrease in C isotopic composition of organic matter (δ13Corg) in the same P-TB interval (∼7‰). This apparent δ13Ccarb-δ13Corg decoupling could be explained by proliferation of methanogen (“methanogenic burst”) in the sediments. A global δ13C compilation shows a large variation in marine δ13Corg records, implying that the “methanogenic burst” according to the Siberian Traps volcanism may have contributed, at least in part, to the δ13Corg variability and to the elevated CH4 levels in the atmosphere. The present and previous observations allow us to infer that the global CH4 cycle may have fluctuated substantially in the aftermath of the extinction.
Introduction
The end-Permian extinction was one of the largest biodiversity crises in the Phanerozoic (e.g., Erwin, 2006; Alroy, 2010; Shen et al., 2011a; Stanley, 2016), and many geologic events around the Permian-Triassic boundary (P-TB) have been proposed as the cause of the extinction, including a bolide impact (e.g., Xu et al., 1985; Becker et al., 2004), Siberian Traps volcanism (e.g., Renne and Basu, 1991; Campbell et al., 1992; Kamo et al., 2003; Reichow et al., 2009; Burgess and Bowring, 2015) and the associated massive release of thermogenic gases (e.g., Wignall, 2001; Retallack and Jahren, 2008; Svensen et al., 2009; Shen et al., 2012; Polozov et al., 2016) and resulting global warming (e.g., Hallam and Wignall, 1997; Kidder and Worsley, 2004; Brand et al., 2012; Joachimski et al., 2012), oceanic anoxia (e.g., Wignall and Hallam, 1992; Wignall and Twitchett, 1996; Isozaki, 1997; Algeo et al., 2008; Shen et al., 2011c; Schobben et al., 2015) accompanied by H2S poisoning (e.g., Grice et al., 2005; Kump et al., 2005; Zhang et al., 2017; Zhou et al., 2017) and hypercapnia (Knoll et al., 1996, 2007), and oceanic acidification (e.g., Heydari et al., 2003; Payne et al., 2007; Clapham and Payne, 2011; Clarkson et al., 2015; Baresel et al., 2017; Garbelli et al., 2017; Jurikova et al., 2020). However, the ultimate trigger mechanisms of the extinction remain a topic of discussion (e.g., Payne and Clapham, 2012; Isozaki, 2019; Racki, 2020).
The global carbon (C) cycle was likely perturbed during the Permian-Triassic transition in association with those geologic events that potentially contributed to the extinction (e.g., Kump and Arthur, 1999). Stable C isotope geochemistry is useful to correlate sections in different regions and to reveal the changes in the global C cycle (e.g., Hayes et al., 1999). Plenty of studies analyzed the C isotopic composition of carbonate (δ13Ccarb) of P-TB rocks and documented a negative δ13Ccarb shift by ∼5‰ during the extinction in various sections around the world, including South China, Iran, Armenia, northern Italy, Austria, Slovenia, and Pakistan (e.g., Magaritz et al., 1988; Baud et al., 1989; Holser et al., 1989; Jin et al., 2000; Richoz, 2006; Horacek et al., 2007a; Horacek et al., 2007b; Korte and Kozur, 2010 and references therein; Shen et al., 2013; Schobben et al., 2016; Joachimski et al., 2020). Although several studies pointed out a substantial diagenetic overprint and/or erosional unconformity on the P-TB δ13Ccarb records (e.g., Heydari et al., 2001; Heydari and Hassanzadeh, 2003; Grasby and Beauchamp, 2008; Yin et al., 2014; Schobben et al., 2016; Li and Jones, 2017), the widely recognized P-TB δ13Ccarb decrease has been generally regarded as an original isotopic signal of seawater (Bagherpour et al., 2019).
Considering a simple box model of the surface-ocean C pool, two principle mechanisms could explain the P-TB δ13C decrease in marine carbonates: 1) a decrease in the output and 2) an increase in the input of 13C-depleted C (e.g., Korte and Kozur, 2010). A collapse in primary productivity and reduction in biological pump (“strangelove oceans”) corresponds to the former mechanism (e.g., Kump, 1991), although it may have produced only a ∼3‰ negative δ13Ccarb shift (Rampino and Caldeira, 2005) and might not be sufficient to explain larger P-TB δ13C declines commonly observed around the world. The increase in the input of 13C-depleted C to the surface oceans seems to be more important (e.g., Payne and Clapham, 2012), and several geologic events have been proposed for the C injection, including volcanogenic CO2 emission involved in the Siberian Traps volcanism (e.g. Renne et al., 1995; Hansen, 2006), methane (CH4) release during destabilization of submarine and permafrost clathrates or thermal alteration of coal by volcanic intrusion (e.g. Erwin, 1993; Morante, 1996; Krull and Retallack, 2000; Berner, 2002; Sarkar et al., 2003; Retallack and Jahren, 2008), enhanced erosion and reoxidation of sedimentary organic matter or terrestrial soil (e.g. Baud et al., 1989; Holser et al., 1989; Ward et al., 2000; Sephton et al., 2005), and the oceanic overturn or shoaling of deep-water (e.g., Kajiwara et al., 1994; Knoll et al., 1996; Algeo et al., 2007a). Korte and Kozur (2010) comprehensively correlated the P-TB δ13Ccarb records of shelf carbonates on a global scale. Based on a gradually decreasing trend toward the P-TB, the authors suggested that the δ13Ccarb decrease was caused by a combination of the Siberian Traps volcanism and a shoaling of anoxic deep-waters onto shelves.
A negative shift of the C isotopic composition of organic C (δ13Corg) across the P-TB has been reported in marine strata in many sections such as Arctic Canada (e.g., Grasby and Beauchamp, 2008; Algeo et al., 2012), Greenland (e.g., Twitchett et al., 2001), Spitsbergen (e.g., Wignall et al., 1998; Zuchuat et al., 2020), South China (e.g., Cao et al., 2002), Japan (e.g., Takahashi et al., 2010), and Australia (e.g., Morante, 1996). The marine δ13Corg decrease has been correlated normally with the negative δ13Ccarb shift in shelf carbonates by assuming that the δ13Ccarb and δ13Corg trends were parallel and the difference between the δ13Ccarb and δ13Ccarb values (∆13C) was consistent during the Permian-Triassic transition, although the ∆13C value is generally controlled by several factors such as carbon dioxide (CO2)-fixing enzymes and atmospheric CO2 levels (pCO2) (e.g., Hayes et al., 1999). A parallel δ13Ccarb and δ13Corg decline has been reported in the P-TB intervals in Austria (Magaritz et al., 1992), Italy (Sephton et al., 2002), mid-Panthalassa (Musashi et al., 2001), and Kashmir (Algeo et al., 2007b). However, some studies pointed out a δ13Ccarb-δ13Corg decoupling across the P-TB. The ∆13C values apparently decrease in some sections in South China, Slovenia, and Iran (Riccardi et al., 2007), and increase in some other Chinese sections (e.g., Shen et al., 2012).
The δ13Corg decrease in a terrestrial P-TB succession has also been reported in South China (e.g., Shen et al., 2011a), South Africa (e.g., Ward et al., 2005; Gastaldo et al., 2020), Antarctica (e.g., Krull and Retallack, 2000), Australia (Morante, 1996), Madagascar (de Wit et al., 2002), and Germany (Scholze et al., 2017). However, the apparent δ13Corg trends on land are generally variable compared to those of marine sediments, and the P-TB δ13Corg decline is not clearly recognized in many terrestrial sections (e.g., Retallack et al., 2005; Fielding et al., 2019). Moreover, the P-TB is generally not well assigned in the terrestrial successions due to their poorer biostratigraphic constraints. Under these circumstances, it is still difficult to correlate the terrestrial δ13Corg records well with the marine δ13Corg and δ13Ccarb records.
Previous studies particularly suggested that a substantial amount of CH4 was released into the ocean/atmosphere during the Siberian Traps volcanism via several processes, including volcanic intrusion into coal (e.g., Retallack and Jahren, 2008; Shen et al., 2012; Rampino et al., 2017), destabilization of submarine and permafrost clathrates (e.g., Krull et al., 2000; Krull et al., 2004; Brand et al., 2016), and enhanced microbial methanogenesis (“methanogenic burst”) (Rothman et al., 2014). As CH4 is a potent greenhouse gas, the huge CH4 input may have contributed to climate warming in the aftermath of the extinction (e.g., Hallam and Wignall, 1997; Joachimski et al., 2012; Sun et al., 2012; Cui and Kump, 2015). However, the global CH4 cycle during the Permian-Triassic transition has been poorly examined and constrained. In this study, we analyzed the δ13Ccarb records of the P-TB carbonates at Chaotian in northern Sichuan, South China. Together with the previously reported δ13Corg records of the same interval, we examined the sedimentary C cycle in eastern Paleotethys during the Permian-Triassic transition. Moreover, we compiled the δ13Ccarb and δ13Corg records of P-TB successions in various marine and terrestrial environments around the world, to examine whether the isotopic signal detected at Chaotian was a global one. Based on those results, we suggest fluctuations in the global CH4 cycle in the aftermath of the end-Permian extinction.
Geological Setting and Stratigraphy
During the Permian to early Triassic, South China was isolated from other continental blocks and located at low latitudes on the eastern side of Pangea (Figure 1B; Muttoni et al., 2009). Shallow-marine carbonates and terrigenous clastics with diverse fossils accumulated extensively on its platform (e.g., Zhao et al., 1981; Yang et al., 1987; Jin et al., 1998). In northern Sichuan along the northwestern edge of South China, carbonates and mudstones of deep-water facies accumulated on a slope/basin that faced on the eastern Paleotethys (Figure 1C; Zhu et al., 1999; Wang and Jin, 2000). The Chaotian section is located ca. 20 km to the north of Guangyuan city in northern Sichuan (Figure 1; 32°37′N, 105°51′E; Isozaki et al. 2004). At Chaotian, Guadalupian (middle Permian) to lowermost Triassic carbonates are continuously exposed along the bank of the Jialingjiang River in a narrow gorge called Mingyuexia. We mapped the eastern bank of the gorge on the southern limb of an E-W trending anticline.
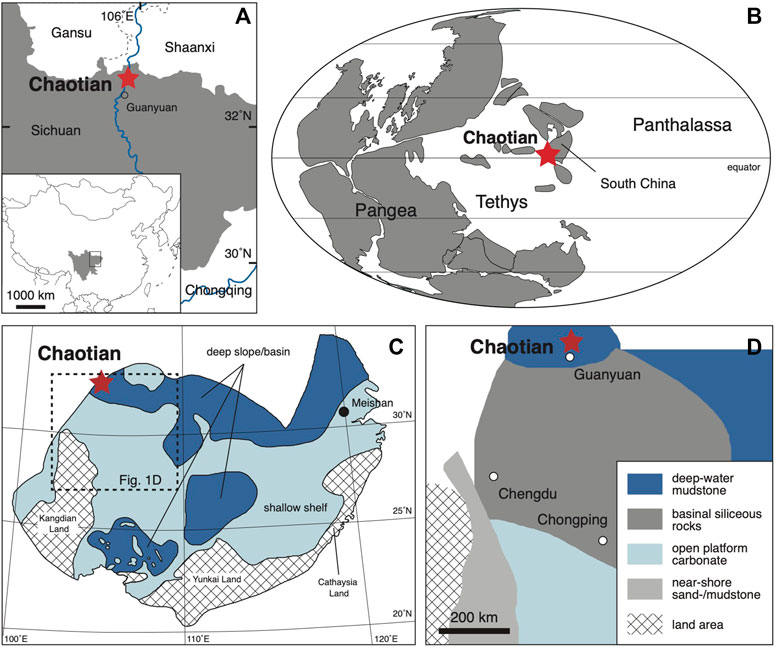
FIGURE 1. Locality and paleogeographic maps of Chaotian. (A) Location map of Chaotian. (B) Global paleogeographic map around the P-TB (modified from Muttoni et al., 2009). (C) Sedimentary facies distribution in South China in the late Changhsingian (late Late Permian) (modified from Wang and Jin, 2000). (D) Detailed sedimentary facies in the square in C (modified from Zhu et al., 1999).
The Permo-Triassic rocks at Chaotian (>300 m thick in total) are composed of the Guadalupian Maokou Formation, the Lopingian Wujiaping and Dalong formations, and the lowermost Triassic Feixianguan Formation, in ascending order (Figure 2; Isozaki et al., 2004; Isozaki et al., 2007; Saitoh et al., 2013a; Saitoh et al., 2013b; Saitoh et al., 2014a). The Maokou Formation (>150 m thick) is composed mainly of massive dark gray bioclastic limestone with diverse shallow-marine fossils, such as calcareous algae, brachiopods, ostracodes, crinoids, rugosa corals, and fusulines. However, the uppermost part (∼11 m thick) of the Maokou Formation is composed of thinly bedded black calcareous mudstone and black chert/siliceous mudstone with abundant radiolarians, conodonts, and ammonoids. The Wujiaping Formation (∼70 m thick) is composed mainly of massive dark gray bioclastic limestone with black chert nodules/lenses, containing shallow-marine fossils such as fusulines, smaller foraminifer, rugosa corals, calcareous algae and brachiopods. At the base of the Wujiaping Formation, a ca. 2 m thick tuffaceous Wangpo bed occurs. The Dalong Formation (∼25 m thick) is composed mainly of thinly bedded black mudstone, black siliceous mudstone and dark gray limestone with radiolarians, ammonoids, bivalves, and brachiopods. The Feixianguan Formation (>30 m thick) is composed mainly of thinly bedded light gray micritic limestone containing few conodonts, ammonoids, and brachiopods.
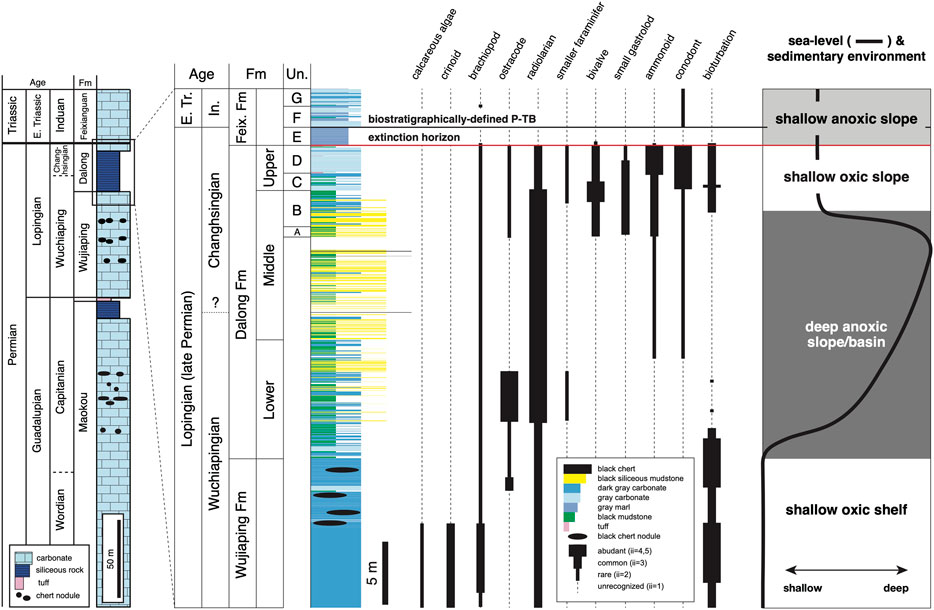
FIGURE 2. The analyzed P-TB interval at Chaotian with ranges of fossil groups (modified from Saitoh et al., 2014a). Un.: unit in Isozaki et al. (2007), E. Tr.: Early Triassic, In.: Induan, Feix.: Feixianguan, ii: ichnofabric index.
Zhao et al. (1978) and Yang et al. (1987) originally described the overall biostratigraphy of the Chaotian section based on fusulines, conodonts, and ammonoids. Isozaki et al. (2004) and Isozaki et al. (2007) re-examined the stratigraphy of the section that spans across the two mass extinction intervals; i.e. the Guadalupian-Lopingian boundary (G-LB) and the P-TB in higher resolution. More analyses on the litho-, bio-, and chemo-stratigraphy of the Chaotian section added further information (Isozaki et al., 2008; Lai et al., 2008; Saitoh et al., 2013a; Saitoh et al., 2013b; Jost et al., 2014; Saitoh et al., 2014b; Saitoh et al., 2015; Saitoh et al., 2017). Isozaki et al. (2007) and Ji et al. (2007) constructed the detailed lithostratigraphy and conodont zonation for the ∼12 m thick interval across the P-TB, and suggested that intermittent felsic volcanism may have contributed to the extinction. Cao et al. (2010) analyzed the δ13Ccarb values around the P-TB and found a negative δ13C excursion around the extinction horizon. Saitoh et al. (2014a) analyzed the nitrogen and organic C isotopic composition of the ∼40 m thick P-TB interval at Chaotian and suggested enhanced nitrogen fixation in the anoxic oceans throughout the Changhsingian.
In the present study, we focused on the ∼40 m thick P-TB interval at Chaotian (Figure 2). This interval is identical to that analyzed in Saitoh et al. (2014a), which contains the ∼12 m thick carbonates analyzed in Isozaki et al. (2007). Fresh rock samples, collected from outcrops and from core samples by scientific drilling to a depth of >30 m, were prepared as polished slabs and thin sections for describing microtextures by petrographical observations. The analyzed P-TB interval is composed of three stratigraphic units: 1) the upper Wujiaping Formation, 2) the Dalong Formation, and 3) the lowermost Feixianguan Formation, in ascending order. The upper Wujiaping Formation (∼10 m thick) is composed mainly of massive dark gray limestone (lime mudstone/wackestone) with some sandy/muddy limestones (Figure 3D). Black chert nodules (<10 cm in diameter) occur in the uppermost part of the Wujiaping Formation. The upper Wujiaping limestones contain diverse shallow-marine fossils such as calcareous algae, crinoids, brachiopods, radiolarians, and ostracodes. Burrows frequently occur in the upper Wujiaping limestones.
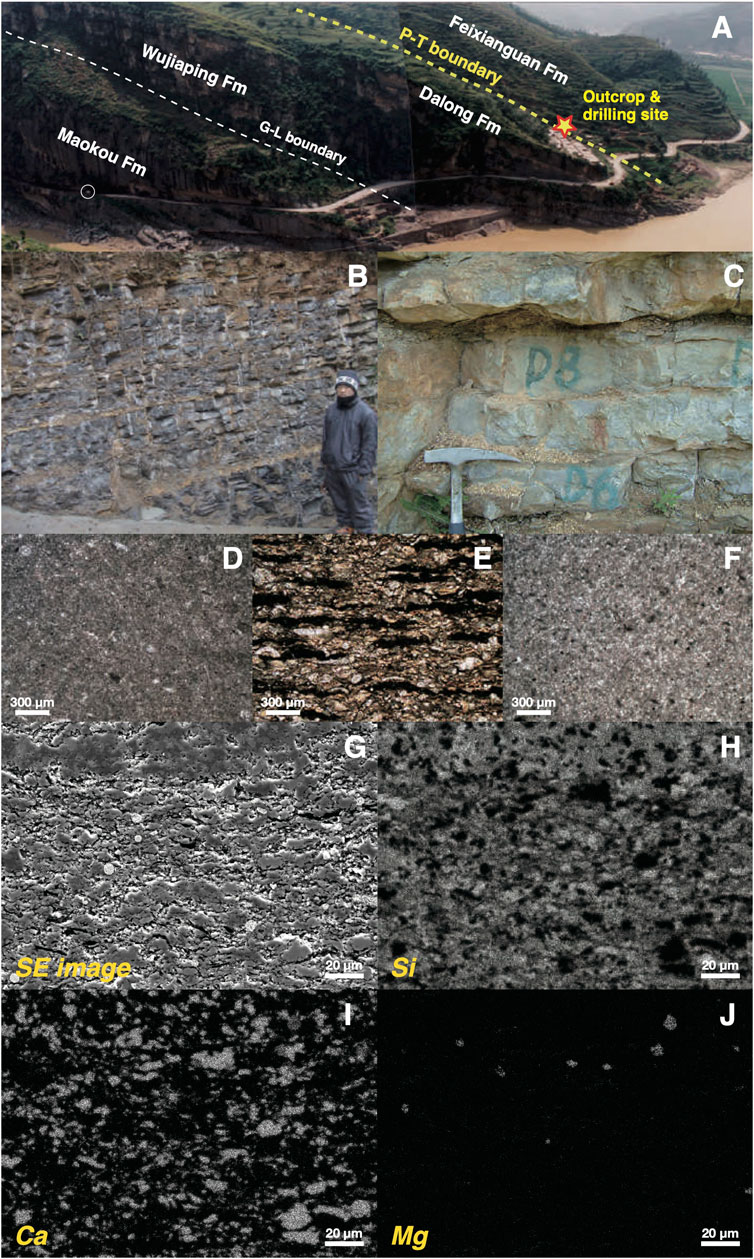
FIGURE 3. A distant view and photographs of the P-TB interval at Chaotian. (A) A distant view of Chaotian (circled car for scale). (B,C) Outcrops of the uppermost Dalong limestones. (D–F) Thin sections of bioclastic limestone in the upper Wujiaping Formation (D), calcareous mudstone in the Dalong Formation (E), and lowermost Feixianguan limestone (F). (G-J) Secondary electron (SE) image (G) and element maps (H–J) of calcareous mudstone in the Dalong Formation. Carbonates in the mudstones are mainly finely-fragmented bioclasts with few secondary dolomites.
The Dalong Formation (∼25 m thick) is composed mainly of thinly bedded black calcareous mudstone, black siliceous mudstone, dark gray muddy limestone, and bedded gray limestone (lime mudstone/wackestone) (Figures 2, 3). The Dalong Formation contains abundant radiolarians with a minor amount of ostracodes, brachiopods, ammonoids, and conodonts, bivalves and small foraminifers. The uppermost (∼3.5 m thick) part of the Dalong Formation mostly consists of bedded gray limestone (lime mudstone/wackestone) (‘Unit C and D’ in Isozaki et al., 2007). Thin (<10 cm thick) acidic tuff layers frequently occur in these limestones. Burrows are observed in the lower and upper Dalong Formation, although bioturbation is generally absent in the middle Dalong Formation. Small pyrite framboids (mostly 3–7 µm in diameter) occur abundantly throughout the Dalong Formation. The lowermost Feixianguan Formation (∼5 m thick) is composed of thinly bedded gray marl and light gray micritic limestone with some sandy/muddy limestones. In particular, the lowermost (1.4 m thick) part (‘Unit E’ in Isozaki et al., 2007) is composed of gray marl. This marl unit is almost barren of fossil, although few ammonoids and bivalves occur from the basal part. The upper part of the lowermost Feixianguan Formation consists of light gray micritic limestone containing few conodonts and brachiopods. Few trace fossils are recognized in the lowermost Feixianguan Formation.
Based on index fossils (fusulines, conodonts, ammonoids, corals, and brachiopods), the analyzed P-TB interval at Chaotian is dated as follows (Figure 2; Zhao et al., 1978; Yang et al., 1987; Isozaki et al., 2004; Isozaki et al., 2007): The upper Wujiaping Formation is correlated with the Wuchiapingian (early Lopingian). The lower Dalong Formation is correlated with the late Wuchiapingian, whereas the middle Dalong Formation belongs to the late Wuchiapingian to early Changhsingian. The upper Dalong Formation is correlated with the late Changhsingian. The lowermost Feixianguan Formation is correlated with the latest Changhsingian to early Induan (early Early Triassic). The main extinction horizon is assigned at the base of the Feixianguan Formation (‘Unit D/E boundary’ in Isozaki et al., 2007), whereas the biostratigraphically defined P-TB is assigned at the base of the overlying micritic limestones (‘Unit E/F boundary’ in Isozaki et al., 2007). Based on the litho- and bio-facies, the sedimentary environments of the three stratigraphic units of the analyzed P-TB interval were reconstructed (Figure 2; Saitoh et al., 2014a). The upper Wujiaping limestones were deposited on the shallow euphotic shelf under oxic conditions. In contrast, the lower and middle Dalong Formation was deposited on the relatively deep slope/basin under anoxic conditions. The upper Dalong limestones were deposited on the relatively shallow slope below the storm wave base under oxic conditions. The lowermost Feixianguan formations were deposited on a relatively shallow slope under anoxic conditions.
Analytical Methods
Fresh rock samples were carefully chosen based on detailed observations of polished slabs and thin sections. Powdered sample was reacted with 100% phosphoric acid at 28°C for 24 h using a GasBench (Thermo Fisher Scientific). The extracted CO2 was separated in a chromatography line with a helium flow, and the carbon and oxygen isotope ratios were measured with a DELTA V PLUS mass spectrometer. The carbonate carbon and oxygen isotopic compositions are presented using the delta notation δ13C = ((13C/12C)sample/(13C/12C)standard−1) and δ18O = ((18O/16O)sample/(18O/16O)standard−1), respectively. The δ13C and δ18O values are reported in ‰ relative to the Vienna Peedee Belemnite (V-PDB) standard. The analytical reproducibility of the δ13Ccarb and δ18Ocarb values was better than 0.1 and 0.1‰, respectively.
Results
Table 1 lists all the measured δ13Ccarb and δ18Ocarb values of the P-TB interval. Figure 4 shows chemostratigraphic profiles of the δ13Ccarb, δ18Ocarb, δ13Corg, and ∆13C values and TOC contents. The δ13Corg values and TOC contents were previously reported in Saitoh et al. (2014a). Figure 5 shows a δ13Ccarb-δ18Ocarb cross plot. The δ13Ccarb values range from −6.9 to +4.3‰, with an average value of ca. 0‰. The δ18Ocarb values range from −7.9 to −2.9‰, with an average value of ca. −5.9‰.
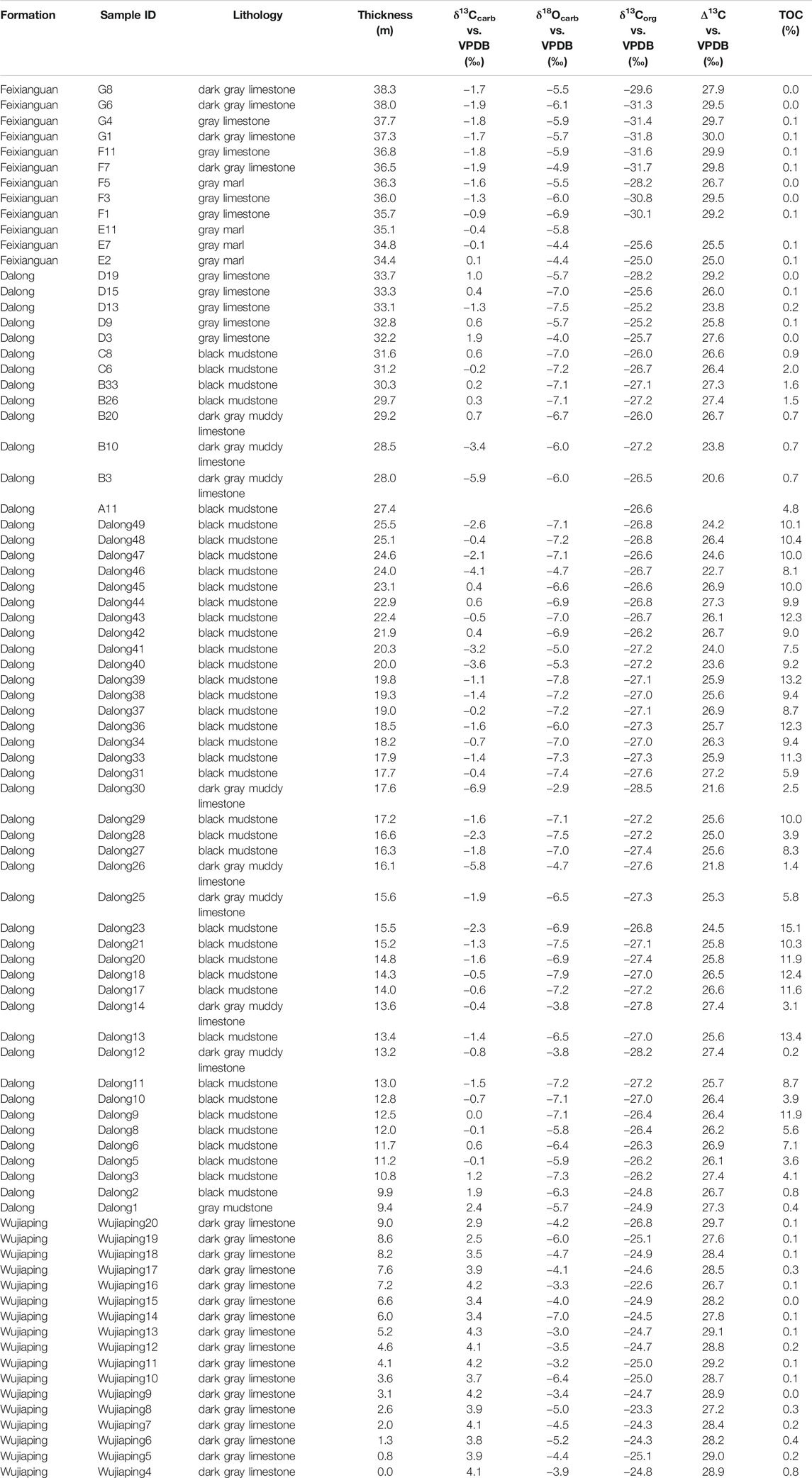
TABLE 1. C and O isotopic composition of the analyzed P-TB interval at Chaotian. The δ13Corg values and TOC contents were reported in Saitoh et al. (2014a).
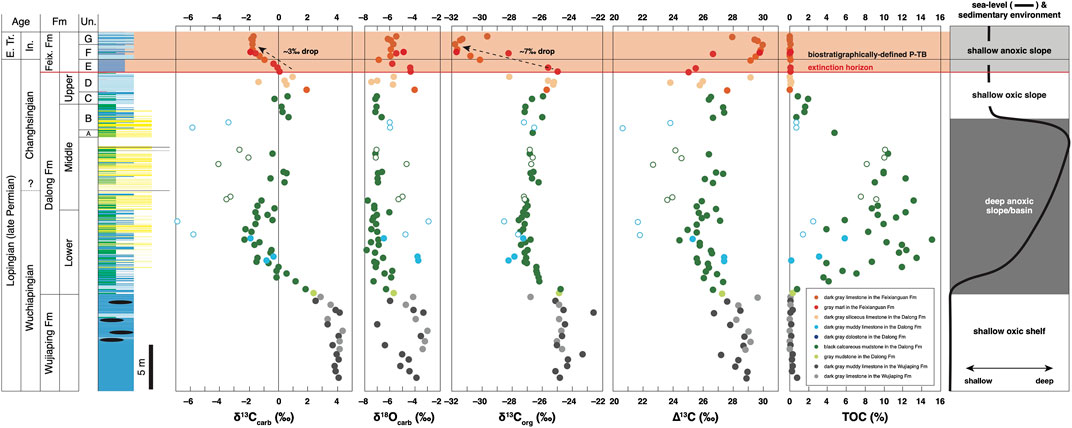
FIGURE 4. Chemostratigraphy of the analyzed P-TB interval at Chaotian. The δ13Corg values and TOC contents were previously reported in Saitoh et al. (2014a). Open symbols represent samples with anomalously low δ13Ccarb values in the Dalong Formation.
The δ13Ccarb values are consistently ca. +4‰ in the upper Wujiaping Formation and decrease from ca. +4‰ to −2‰ across the Wujiaping/Dalong formation boundary. In the Dalong Formation, the δ13Ccarb values increase slightly from ca. −2 to +1‰ upward except for some anomalously low (<−3‰) values (open symbols in Figure 4). The δ13Ccarb values decrease from ca. +1‰ to −2‰ across the Dalong/Feixianguan formation boundary (i.e., the P-TB) and are consistent around −2‰ in the lowermost Feixianguan carbonates. The present δ13Ccarb results around the P-TB are generally identical to the results in Cao et al. (2010). The δ18Ocarb values are somewhat variable in in the upper Wujiaping limestones and are mainly around −7‰ in the overlying Dalong Formation. Above the P-TB, the δ18Ocarb values are mostly around −6‰ in the lowermost Feixianguan Formation. The ∆13C values are mainly between 27 and 29‰ in the upper Wujiaping Formation and decrease slightly to ca. 26‰ in the Dalong Formation. The ∆13C values increase to ca. 30‰ in the overlying lowermost Feixianguan carbonates.
No linear correlation is observed between the δ13Ccarb and δ18Ocarb values (Figure 5). It indicates that secondary overprinting on the Chaotian carbonates is not significant (Knauth and Kennedy, 2009). In the chemostratigraphic profile, however, some δ13Ccarb values in the Dalong Formation are anomalously low (<−3‰; open symbols in Figure 4) and these samples were likely affected at least partly by the secondary addition of 13C-depleted C. Except for these anomalous values, the present δ13Ccarb results represent a smooth chemostratigraphic trend in the analyzed interval. This trend may record secular changes in the δ13C value of dissolved inorganic carbon (DIC) in seawater (δ13CDIC) in the Lopingian to earliest Triassic. Based on the C isotopic analyses of the P-TB carbonates in Iran, Schobben et al. (2016) suggested that the first-order δ13Ccarb trend in the bulk carbonates is robust, although small-scale isotopic fluctuations may be due to secondary alteration. In the following discussion, we focus solely on the first-order δ13Ccarb trend in the analyzed interval at Chaotian.
Discussion
δ13C Stratigraphy at Chaotian
δ13Ccarb Decrease Around the Wuchiapingian-Changhsingian Boundary
The δ13Ccarb values unidirectionally decrease from ca. +4 to −2‰ across the Wujiaping/Dalong formation boundary at Chaotian (Figure 4). Although the timing of this negative δ13Ccarb shift is not well constrained because of the poor occurrence of index fossils, the δ13Ccarb shift likely occurred around the Wuchiapingian-Changhsingian boundary. This δ13Ccarb decrease apparently coincides with the deepening of the sedimentary setting from a shallow shelf to relatively deep slope/basin floor. However, we emphasize that carbonates in the Dalong Formation of deep-water facies comprise mainly finely fragmented bioclasts and few secondary dolomite (Figures 3E, G–J). Thus, regardless of the lithofacies change, this prominent δ13Ccarb decrease likely records the Lopingian secular change in the δ13CDIC value in the surface oceans in the eastern Paleotethys.
Two possible mechanisms could explain the δ13Ccarb decrease: 1) collapse of primary productivity in the surface oceans and 2) addition of isotopically light C into the DIC pool of the surface oceans. As certain shallow-marine taxa went extinct across the Wuchiapingian-Changhsingian boundary (e.g., Knoll et al., 1996; Bambach, 2006), extinction-related productivity deficiency may have contributed to the δ13Ccarb decrease at Chaotian. However, the magnitude of the δ13Ccarb drop (∼6‰) seems to be too large to be caused solely by the collapse of primary productivity (e.g., Berner, 2002); thus the addition of 13C-depleted C to the DIC pool in the surface oceans likely contributed to the δ13Ccarb drop. We emphasize that the sedimentary setting deepened from oxic shelf to anoxic slope/basin floor. The ubiquitous occurrence of small pyrite framboids in the Dalong Formation suggests the dominance of sulfate reduction in an anoxic deep-water mass on the slope/basin. The DIC in the deep-water mass would have become enriched in 12C due to the anaerobic respiration; therefore, the injection of 13C-depleted C into the surface oceans via shoaling of the deep-water might have contributed to the negative δ13Ccarb shift. It is noteworthy that no eruption of a large igneous province or substantial sea-level change occurred on a global scale around the Wuchiapingian-Changhsingian boundary (e.g., Haq and Schutter, 2008; Bond and Wignall, 2014). An input of volcanogenic excess CO2 and/or reoxidized sedimentary organic C during a eustatic sea-level fall is unlikely for the cause of the δ13Ccarb drop at Chaotian.
Regional and global correlations would be useful to constrain the cause of the δ13Ccarb decrease at Chaotian. Around the Wuchiapingian-Changhsingian boundary, a ca. 6‰ negative δ13Ccarb shift was reported at Shangsi, ca. 60 km southwest of Chaotian, in northern Sichuan (Bai et al., 2008; Shen et al. 2013), which is almost identical in magnitude to that at Chaotian. Therefore, the deep-water carbonates in northwestern South China probably record the common δ13CDIC decrease in eastern Paleotethys. Similar δ13Ccarb declines were also reported from other sections in South China, including Dukou in Sichuan, Shiligou in Chongqing, Zhuqiao in Hubei, and Heshan and Matan in Guangxi (Shao et al., 2000; Shen et al., 2013; Yang et al., 2019); nonetheless, the magnitude of the δ13Ccarb decreases (∼2–5‰) is variable and smaller than that at Chaotian. At Abadeh in central Iran, Richoz (2006) found a negative δ13Ccarb shift in the corresponding interval in the Hambast Formation, which was later re-confirmed by Liu et al. (2013). A similar negative δ13Ccarb excursion (∼4‰) was also reported from the equivalent horizons of Julfa beds/Alibashi Formation boundary at Kuh-e-Ali Bashi in northwestern Iran (Shen et al., 2013). These almost co-eval negative δ13Ccarb shifts around the Wuchiapingian-Changhsingian boundary in Iran are comparable to that at Chaotian. Moreover, a large negative δ13Ccarb excursion (∼7‰) was demonstrated in the Bellerophon Formation at the Reppwand section in the Carnic Alps, Austria (Buggisch et al., 2015), which is also correlative to the negative shift at Chaotian.
A negative δ13Corg shift, as well as the δ13Ccarb drop, around the Wuchiapingian-Changhsingian boundary has been documented in several sections in South China and Arctic Canada (e.g., Bai et al., 2008; Beauchamp et al., 2009; Wei et al., 2015; Liao et al., 2016). These δ13Corg decreases may have likewise recorded fluctuations in the global C cycle; however, a negative δ13Ccarb/δ13Corg shift around the Wuchiapingian-Changhsingian boundary is not clear in the rest of the Permian world (e.g., Baud et al., 1996; Korte et al., 2004; Richoz, 2006; Baud et al., 2012). Even at the above-mentioned sections in which a negative δ13C shift is recognized, the magnitude of and the trend in the δ13C decrease vary substantially (Shen et al., 2013). Under the circumstances, we infer that the C cycle in the surface oceans around the Wuchiapingian-Changhsingian boundary was not globally uniform but rather widely variable possibly owing to local factors, such as primary productivity and oceanic circulation along the continental margins. More studies with high chemo- and bio-stratigraphic resolutions in various sections around the world are necessary to reveal the fluctuations in the global C cycle around the Wuchiapingian-Changhsingian boundary.
δ13Ccarb Decrease Across the P-TB
The δ13Ccarb values decrease from ca. +1 to −2‰ across the Dalong/Feixianguan formation boundary (P-TB) at Chaotian (Figure 4). This negative δ13Ccarb shift is identical to that previously reported at Chaotian in Cao et al. (2010). Likewise, two possible mechanisms should be considered for the cause of this δ13Ccarb decrease: 1) collapse of primary productivity in the surface oceans and 2) addition of 13C-depleted C to the DIC pool in the surface oceans. The negative δ13Ccarb shift occurs across the major extinction horizon, suggesting that the shift was driven by the collapse of primary productivity during the extinction. The addition of isotopically light C to the shallow-marine DIC pool is another plausible candidate for the cause of the δ13Ccarb decrease. Isozaki et al. (2007) found frequent intercalations of thin felsic tuff layers across the extinction horizon at Chaotian and suggested volcanic stress on the shallow-marine biota during the extinction. An excess input of volcanogenic CO2 (δ13C: ∼−5‰) may also have contributed to the δ13Ccarb decrease. Weathering of sedimentary organic matter due to a large regression is another possible mechanism for the δ13Ccarb decrease (e.g., Yin et al., 2014). However, the sea-level drop occurred significantly before the δ13Ccarb shift at Chaotian (Isozaki et al., 2007; Saitoh et al., 2014a), without any evidence for shallowing across the extinction horizon (Figure 4). This suggests that a contribution of reoxidized organic C to the δ13Ccarb drop was negligible. Song et al. (2012a) proposed the existence of a large vertical δ13Ccarb gradient in the end-Permian oceans, along which a deepening of the sedimentary setting possibly caused the observed δ13Ccarb decrease. At Chaotian, however, the upper Dalong and lowermost Feixianguan carbonates across the P-TB were deposited on a relatively shallow slope without abrupt sea-level changes. Thus the δ13Ccarb decrease cannot be attributed to the assumed vertical δ13Ccarb gradient in the water column.
∆13C Increase Across the P-TB
Saitoh et al. (2014a) reported the δ13Corg chemostratigraphy of the P-TB interval at Chaotian and revealed that the δ13Corg values drop abruptly by 7‰ (from −25 to −32‰) immediately above the extinction horizon (Figure 4). This clear δ13Corg decrease is consistent apparently with the δ13Ccarb shift documented in the present study, although the magnitude of the δ13Corg decrease (∼7‰) is substantially larger than that of the δ13Ccarb decrease (∼3‰). The ∆13C (=δ13Ccarb−δ13Corg) values increase by 4‰ from +26‰ to +30‰ in the aftermath of the extinction at Chaotian. Two possible mechanisms may explain this ∆13C increase: 1) an increase in C isotopic fractionation of biological C fixation and 2) additional input of 13C-depleted C to the sedimentary organic matter. The high aqueous CO2 concentration ([CO2 (aq)]) generally promotes the large C isotopic fractionation during photosynthetic C fixation (εp) in the surface oceans (e.g., Rau et al., 1992, Rau et al., 1997; Kump and Arthur, 1999). The ∆13C values are ∼26‰ in the middle to upper Dalong Formation at Chaotian, which are consistent with the typical Calvin cycle (e.g., Schidlowski et al., 1983), although the values increase substantially to ca. 30‰ above the P-TB (Figure 4). It should be noted that a substantial amount of CO2 was released during the Siberian Traps volcanism around and mostly after the P-TB (Supplementary Information) (e.g. Renne et al., 1995; Hansen, 2006; Cui et al., 2013; Cui and Kump, 2015). The increased [CO2 (aq)] may have promoted greater εp (e.g., Kump and Arthur, 1999).
The previous estimates of the amount of emitted CO2 and of the elevated pCO2 during the Permian-Triassic transition are useful to constrain the influence of εp changes on the δ13C records. Cui and Kump (2015) estimated that pCO2 rose from 500 to 4,000 ppm to ∼8,000 ppm during the extinction (Supplementary Information). Kump and Arthur (1999) derived a simplified relationship between pCO2 and εp from isotopic data of modern marine algae, with some assumptions: εp ≈ 25–2,301/pCO2. According to this relationship, εp with pCO2 of 500, 4,000, and 8,000 ppm is calculated apparently to be ∼20.4, ∼24.4, and ∼24.7‰, respectively. Thus, the relatively large δ13Corg decline (∼7‰) compared to the δ13Ccarb decrease (∼3‰) at Chaotian can be explained by the enlarged εp according to the elevated pCO2. However, it is obviously difficult to extrapolate the relationship formula between pCO2 and εp in Kump and Arthur (1999) directly to the end-Permian system, due to several reasons. First, several assumptions with large uncertainties are included in this simplified formula, such as the dissolved phosphate concentration in the surface seawater. Moreover, εp is controlled, not only by pCO2, but also by temperature and the growth rate of phytoplankton, in general (e.g., Rau et al., 1997; Kump and Arthur, 1999). Second, this relationship formula was obtained empirically on the basis of data of modern haptophyte algae and seawater (Bidigare et al., 1997). Different coefficients on the formula would be more appropriate for the end-Permian phytoplankton communities with unknown physiology. Third, the relationship was obtained on the basis of observations of modern environments with relatively low [CO2 (aq)] (calculated pCO2 < 850 ppm, assuming equilibration according to Henry’s Law at 25°C). It is uncertain whether the formula can be extrapolated to environments with substantially high [CO2 (aq)], which are particularly supposed in the aftermath of the end-Permian extinction (Cui et al., 2013; Cui and Kump, 2015).
Nonetheless, the low sensitivity of εp to pCO2 under high-pCO2 conditions is probably essential (Rau et al., 1997; Kump and Arthur, 1999). Because the reciprocal dependence of εp on pCO2 is attributed theoretically to isotopic discrimination by diffusion of CO2 from the ambient seawater to the phytoplankton cell (Laws et al., 1995). It is also suggested that the sensitivity of εp to pCO2 of land plants is lower than that of marine phytoplankton (e.g., Popp et al., 1989). The estimated high pCO2 up to 4,000 ppm before the end-Permian extinction (Brand et al., 2012; Cui et al., 2013; Cui and Kump, 2015) allows us to postulate that the influence of εp change on the Chaotian δ13C records was not significant.
Additional input of 13C-depleted C to the sedimentary organic C pool is the other possible mechanism for the Chaotian P-TB ∆13C increase. Rothman et al. (2014) suggested that increased Ni input to the ocean/atmosphere during the Siberian Traps volcanism promoted methanogen proliferation in the oceans (“methanogenic burst”). Methanogens generally fix C via the reductive acetyl-CoA pathway, which can produce greater C isotopic fractionation than the Calvin cycle (e.g., Preuss et al., 1989). The organic C from methanogenic biomass may have contributed, at least partly, to the ∆13C increase. Terrestrial plants are another possible C source for sedimentary organic matter in the Chaotian carbonates. In general, the δ13Corg value of Permian terrestrial plant is thought to be higher than the value of marine phytoplankton (e.g., Faure et al., 1995; Foster et al., 1997; Korte et al., 2001). Thus, if the terrestrial C flux decreased across the P-TB, the bulk δ13Corg and ∆13C values of the sediments would have decreased and increased, respectively. However, two lines of evidence exclude the possibility of the decreased terrestrial flux at Chaotian. First, as discussed above, the water depth of depositional site did not change substantially across the P-TB, and a large change in terrestrial flux owing to sea-level changes is unlikely. Second, previous studies suggested enhanced chemical weathering and increased continental flux during the Permian-Triassic transition (Algeo and Twitchett, 2010; Algeo et al., 2011; Cao et al., 2019). At Chaotian, the enhanced chemical weathering is supported by the increased supply of clay minerals/micas around the P-TB based on bulk nitrogen isotope records (Saitoh et al., 2014a). The terrestrial flux may have increased (rather than decreased) in the aftermath of the extinction, and thus the observed ∆13C increase cannot be attributed to the decreased terrestrial C flux. Also, the putative vertical δ13Corg gradient (Luo et al., 2014) cannot explain the ∆13C increase because the water depth of depositional site did not change substantially across the P-TB at Chaotian.
In summary, the P-TB ∆13C increase at Chaotian was most likely due to the proliferation of methanogen in the sediments during the Siberian Traps volcanism (Figure 4), corresponding to the “methanogenic burst” event at a local scale (Rothman et al., 2014).
Global δ13C Correlations Around the P-TB
Regional δ13C Differences
The δ13C profile across the P-TB at Chaotian is here relatively perceived through global correlation, in the context of global C cycle during the Permian-Triassic transition. We focused particularly on the apparent magnitude of the δ13C decrease across the P-TB in previous studies and examined its frequency distribution on a global scale (Figures 6, 7). All the reference sections in the current compilation are summarized in Table 2. We categorize the compiled sections geographically into seven realms; i.e., Boreal, eastern Paleotethys, western Paleotethys, western Pangea, mid-Panthalassa, Neotethys, and Gondwana (Figure 6).
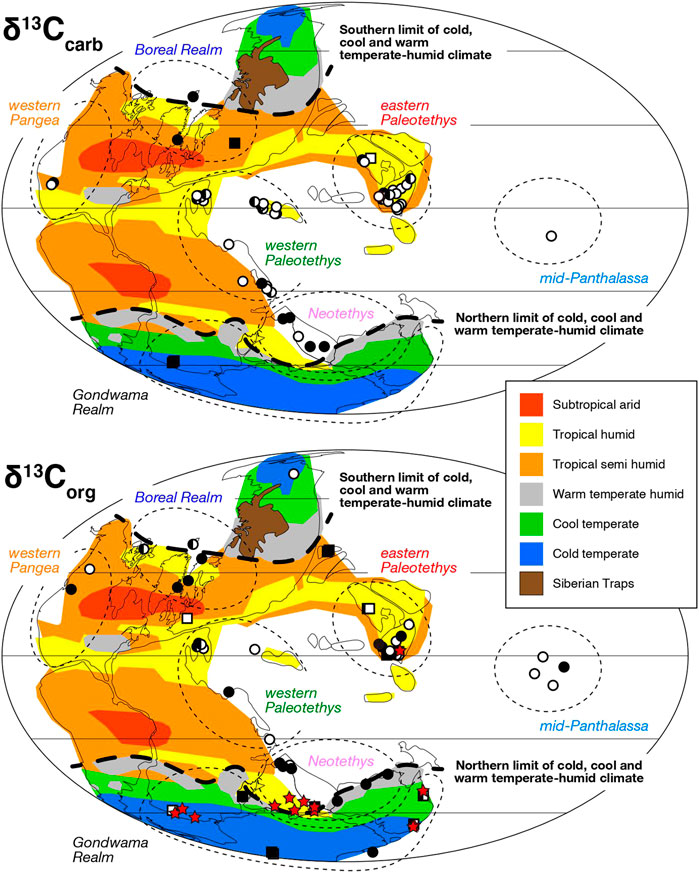
FIGURE 6. Paleolocation map of the P-TB sections examined in inorganic (upper) and organic (lower) carbon isotope studies. Marine and terrestrial sections are shown as circles and squares, respectively. Filled and opened symbols represent sections in which the reported magnitude of the P-TB δ13Ccarb decrease is large (>5‰) and small (<5‰), respectively. A half-filled symbol represents a section in which both large and small δ13C decreases were reported. A terrestrial section at which the P-TB δ13Corg decrease is not clearly recognized is shown as a red star. The paleolocation of mid-Panthalassan sections is not precisely specified. Paleoclimate classification is after Schneebeli-Hermann (2012) and Benton and Newell (2014).
Marine Records
Previous δ13Ccarb studies are concentrated mostly in eastern (China) and western Tethys realms (Iran to Italy) (Figures 6, 7; Table 2), where extensive carbonate platforms developed under warm tropical climate. These two realms share almost the same frequency distribution of the magnitude of the P-TB δ13Ccarb decrease (Figure 7), suggesting a common δ13CDIC decline throughout Paleotethys during the Permian-Triassic transition. The magnitude of the δ13Ccarb decrease ranges mostly between 3 and 6‰ in those tropical regions. In the Boreal realm, the magnitude of the P-TB δ13Ccarb decrease in few marine records is substantially large (to 19‰), which indicates a diagenetic overprint onto the original isotopic signal from seawater (Mii et al., 1997). In contrast, the magnitude of the P-TB δ13Corg decrease varies substantially around the world (Figures 6, 7). In particular, a relatively large (∼7‰) δ13Corg decline has been reported in several marine sections in high latitudes such as Greenland, Spitsbergen, Australia, and Antarctica (e.g., Retallack and Jahren, 2008; Nabbefeld et al., 2010). It is consistent with the previous notion that the magnitude of the δ13Corg decrease in high latitudes was substantially larger than that of the δ13Ccarb decrease in equatorial regions (e.g., Krull et al., 2000; Krull et al., 2004; Korte and Kozur, 2010; Payne and Clapham, 2012; Saltzman and Sedlacek, 2013; Yuan et al., 2015).
Terrestrial Records
The present δ13C compilation also demonstrates that the P-TB δ13Corg decrease is not clearly recognized in a number of terrestrial sections, especially around the Neotethys and in Gondwana realms (Figure 6). The δ13Corg decline is not clearly recognized in previous studies at 14 out of 49 terrestrial sections (Table 2). In these sections, the δ13Corg values are sometimes substantially scattered and no smooth δ13Corg trend is observed (e.g., de Wit et al., 2002; Retallack et al., 2005; Coney et al., 2007). In other Gondwanan sections, the δ13Corg decline is recognized and the apparent magnitude of the δ13Corg decrease is mostly not large (Figure 7). However, the δ13Corg decline is obscured frequently by sharp δ13Corg drops to −45‰ (e.g., Krull and Retallack, 2000; Retallack et al., 2005).
Variable ∆13C Records
Magaritz et al. (1992) originally proposed a parallel δ13Ccarb and δ13Corg trend on the basis of the P-TB isotope profile of the Gartnerkofel Core from the Carnic Alps, Austria, and later studies confirmed similar parallel trends not only in the Tethyan but also in the Panthalassan realms (e.g., Musashi et al., 2001; Algeo et al., 2007b; Luo et al., 2014). However, other studies pointed out a decoupling between the δ13Ccarb and δ13Corg trends around the P-TB. For example, Riccardi et al. (2007) analyzed the δ13Ccarb and δ13Corg values of the P-TB carbonates at Meishan and Shangsi in South China, and compiled the ∆13C changes during the extinction in Iran, Slovenia, Japan, Austria, and South China. They found a ∆13C decrease across the extinction horizon in these regions and suggested that it was due to proliferation of green sulfur bacteria with photic zone euxinia (e.g., Grice et al., 2005; Zhang et al., 2017). Kaiho et al. (2009) confirmed the ∆13C decrease at Meishan. In contrast, the apparent ∆13C increase was reported at several sections in Italy (Siegert et al., 2011), South China (Shen et al., 2012; this study), and Pakistan (Schneebeli-Hermann et al., 2013). It is difficult to reconstruct the ∆13C changes in high latitudes as carbonates are generally scarce in those regions. Nonetheless, the present compilation illustrates that the δ13Corg records are substantially variable on a global scale particularly in high latitudes, in marked contrast to the rather consistent δ13Ccarb records in equatorial Paleotethys (Figures 6, 7).
Potential Causes of the Marine δ13Corg Variability
Several possible mechanisms may explain the observed δ13Corg variability in marine records on a global scale during the Permian-Triassic transition: 1) eustatic sea-level changes, 2) intense continental weathering, 3) proliferation of green sulfur bacteria, 4) elevated pCO2, and 5) “methanogenic burst”.
Eustatic Sea-Level Changes
The δ13Corg value of terrestrial plant was generally thought to be higher than the value of marine phytoplankton in the Permian (e.g., Faure et al., 1995; Foster et al., 1997; Korte et al., 2001). Eustatic sea-level changes during the Permian-Triassic transition may have been responsible for the δ13Corg variability of shallow-marine records, controlling the mixing ratio of 13C-enriched terrestrial and 13C-depleted marine C in the shelf sediments. The Permian-Triassic transition is marked by a major transgression on a global scale (e.g., Hallam and Wignall, 1999; Erwin et al., 2002; but also see; Yin et al., 2014). It is therefore likely that shelf sediments of proximal facies shifted to be of more distal facies during the transgression, and that the terrestrial C flux to the depositional setting reduced, which resulted in the apparent δ13Corg decrease in the bulk sediments. Although the eustatic sea-level changes could have exerted a first-order control on the global δ13Corg variability of shallow-marine records, they do not fully explain the regionally variable δ13Corg records. For example, the δ13Corg records of terrestrial to marine transitional sections in western South China do not record a simple mixing of terrestrial and marine C, despite of a regional transgression, but rather the atmospheric C isotopic signal (Cui et al., 2017). Moreover, the P-TB δ13Corg drop and ∆13C increase at the present Chaotian cannot be attributed to the sea-level changes, because the water depth of depositional site did not change substantially across the P-TB, as discussed above (Figure 4).
Intense Continental Weathering and Increased Terrestrial C Flux
The Permian-Triassic transition is characterized by extensive vegetation collapse on lands (e.g., Retallack et al., 1996; Benton and Newell, 2014), massive soil erosion (e.g., Retallack, 2005; Sephton et al., 2005), and intense continental weathering and increased continental flux to the oceans on a global scale (e.g., Algeo and Twitchett, 2010; Algeo et al., 2011; Cao et al., 2019). An increased flux of terrestrial plant C (with relatively high δ13Corg value) to the shelf sediments via the vegetation collapse and intense weathering may have increased the bulk δ13Corg values of sediments. However, this process generally decreases the bulk ∆13C value of shelf carbonates and cannot explain the P-TB ∆13C increase observed in several sections, including Chaotian, as discussed in the former section (Figure 4). Aged refractory organic matters may also have been derived into the sediments via the enhanced continental weathering and/or soil erosion (Hayes et al., 1989), though it is difficult to constrain their δ13Corg values. On the other hand, it is most likely that a terrestrial nutrient flux to the oceans also increased simultaneously via the intense continental weathering, which stimulated eutrophication and algal blooms in the coastal oceans (e.g., Xie et al., 2007; Algeo et al., 2011; Kaiho et al., 2016). The algal blooms may have resulted in an increased flux of marine C (with relatively low δ13Corg value) to the shelf sediments, offsetting the influence of increased terrestrial C flux on the bulk δ13Corg value of the sediments. Because of this offset effect, the net influence of the intense continental weathering on the marine δ13Corg records is highly uncertain.
Proliferation of Green Sulfur Bacteria With Photic Zone Euxinia
Characteristic green sulfur bacteria (GSB) may have proliferated under photic-zone euxinic conditions during the Permian-Triassic transition (e.g., Grice et al., 2005). They generally fix C via the reverse tricarboxylic acid (TCA) cycle, which can produce smaller C isotopic fractionation (∼12.5‰; van Breugel et al., 2005), than the Calvin cycle (variable but mostly 25–35‰) (e.g., Schidlowski et al., 1983). Thus, the proliferation of GSB and an increased contribution of GSB biomass to the bulk organic-C pool in the shelf sediments would have decreased the ∆13C value of the sediments. Riccardi et al. (2007) reported the P-TB ∆13C decrease in various sections in the peri-Tethyan realm and attributed it to the GSB proliferation. Nonetheless, the proliferation of GSB cannot explain the apparent ∆13C increase observed in several sections, including Chaotian (Figure 4).
Enlarged C Isotopic Fractionation During Photosynthesis Under the Elevated pCO2
The increased [CO2 (aq)] and εp in the surface oceans is another potential mechanism for the observed δ13Ccarb-δ13Corg decoupling (e.g., Rau et al., 1992, Rau et al., 1997). However, as discussed above for the Chaotian record, the influence of εp change on the P-TB δ13C records may not have been significant.
Methanogenic Burst
In addition to the several potential mechanisms discussed above, we emphasize here that the “methanogenic burst” may also have contributed to the variable δ13Corg records on a global scale (Figures 6, 7). A substantial amount of Ni was presumably released into the atmosphere during the Siberian Traps volcanism (Le Vaillant et al., 2017; Rampino et al., 2017). Because Ni is a key element for microbial methanogenesis (e.g., Diekert et al., 1981), the temporary input of excess Ni was likely favorable for methanogens (e.g., Basiliko and Yavitt, 2001) and presumably enhanced microbial methanogenesis (“methanogenic burst”) on a global scale (Rothman et al., 2014).
Methanogen generally fixes C via the reductive acetyl-CoA pathway, which can produce larger C isotopic fractionation up to 40‰ (e.g., Preuss et al., 1989), compared to the Calvin cycle (e.g., Schidlowski et al., 1983). Thus, according to the “methanogenic burst”, the increased organic-C flux from expanded methanogen biomass to the bulk organic-C pool in the local sediments may have caused the large δ13Corg decrease (Figures 6, 7). However, the activity of methanogen is generally regulated, not only by the Ni availability, but also by a number of environmental factors, such as temperature, CO2 levels, and availability of organic substrates (Supplementary Information; e.g., Singh et al., 2010; Nazaries et al., 2013). Although the excess Ni input during the Siberian Traps volcanism likely promoted methanogenesis, the variable activity of methanogen in the local sediments may have been responsible for the observed δ13Corg variability on a global scale. The elevated temperature and pCO2 may also have stimulated the “methanogenic burst”, because these factors generally increase the biogenic CH4 emissions in various environments (Supplementary Information; e.g., van Groenigen et al., 2011; Yvon-Durocher et al., 2014; Aben et al., 2017). The “methanogenic burst” may have occurred not only in marine sediments but also in terrestrial wetlands (Figure 8). Nonetheless, it is still difficult to estimate the total amount of released Ni during the Siberian Traps volcanism (Le Vaillant et al., 2017), and to evaluate the influence of the “methanogenic burst” on the global δ13Corg records quantitatively.
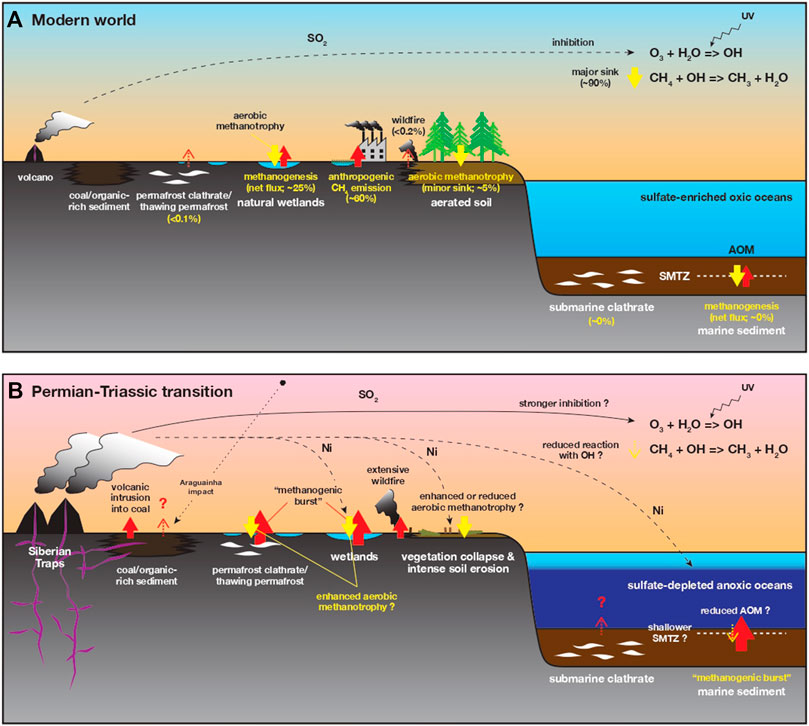
FIGURE 8. Schematic diagram of the global CH4 cycle in the modern world (A) and during the Permian-Triassic transition (B). CH4 influx to and efflux from the atmosphere are shown as red and yellow arrows, respectively. In the modern system, the global atmospheric CH4 budget is 0.4–0.5 Gt C/year (Supplementary Information; e.g., Conrad, 2009; Nazaries et al., 2013). During the Permian-Triassic transition, several processes may have contributed to the elevated pCH4 and climate warming, although terrestrial responses to the elevated pCH4 were probably complex. The elevated pCH4 enhanced aerobic methanotrophy in local soils whereas the intense soil erosion decreased a net CH4 efflux in the global budget. AOM: anaerobic oxidation of methane; SMTZ: sulfate-methane transition zone. See Supplementary Information for details.
In summary, the P-TB δ13Corg variability in marine records on a global scale may have been attributed to several potential mechanisms, including the eustatic sea-level changes, intense continental weathering, and GSB proliferation. We infer that the “methanogenic burst” was also involved, at least in part, in the substantial δ13Corg decrease in several sections, including the present Chaotian (Figure 4).
Implications for the Global CH4 Cycle in the Aftermath of the Extinction
A substantial amount of CH4 was presumably released into the atmosphere during the Siberian Traps volcanism (Figure 8B), via volcanic intrusion into coal (e.g., Retallack and Krull, 2006; Retallack and Jahren, 2008; Grasby et al., 2011; Shen et al., 2012; Rampino et al., 2017; Elkins-Tanton et al., 2020), and via destabilization of submarine and permafrost clathrates (e.g., Krull et al., 2000; Krull et al., 2004). Pyrogenic CH4 was also produced by the incomplete combustion of organic carbon (e.g., Kirschke et al., 2013) and emitted during extensive wildfire events around the P-TB both in the northern and southern hemispheres (e.g., Shen et al., 2011b; Hudspith et al., 2014; Vajda et al., 2020). The “methanogenic burst” likely contributed to the elevated pCH4 (Rothman et al., 2014). The claimed Araguainha impact event in Brazil (Tohver et al., 2013) may also have contributed to the CH4 accumulation in the atmosphere, though its timing, magnitude, and effective time span for the global CH4 cycle are not well-constrained. Together with other greenhouse gases like CO2, the elevated pCH4 may have contributed to the climate warming during the earliest Triassic (e.g., Hallam and Wignall, 1997; Joachimski et al., 2012; Sun et al., 2012; Cui and Kump, 2015), although the long-term warming may have been disturbed intermittently by short-term SO2-induced cooling (Black et al., 2018).
Although there still remains large uncertainty, we infer fluctuations in the global CH4 cycle in the aftermath of the extinction based on the present and previous observations (Figure 8B). Firstly, aerobic methanotrophy may have prevailed in aerated terrestrial soils in the Gondwana and peri-Gondwanan realms (Figure 8B; Krull and Retallack, 2000). The present compilation illustrates that the P-TB δ13Corg decrease is not clearly recognized in a number of terrestrial sections, especially around the Neotethys and in Gondwana realms (Figure 6; Table 2). The highly scattered and variable δ13Corg records in the terrestrial successions were likely due to local-scale and short-term organic C dynamics in soils, including vegetation, selective microbial decomposition of sedimentary organic matters with C isotopic fractionation, and addition of microbial biomass to the sedimentary C pool (e.g., Krull and Retallack, 2000; Korte and Kozur, 2010). The locally enhanced methanotrophy might have been involved, at least in part, in the soil C dynamics and in the scattered δ13Corg records in the Gondwana and peri-Gondwanan realms (Krull and Retallack, 2000), possibly according to the warming and permafrost thaw (Supplementary Information; e.g., Oh et al., 2020).
Secondly, the oceanic sediments may have been a significantly large source for atmospheric CH4 at that time. In the modern oceans enriched in sulfate (the SO42– concentration = 28 mM), almost all CH4, produced in deeper sediments, is consumed by anaerobic oxidation of methane (AOM) in the sulfate-methane transition zone (SMTZ) (Figure 8; Supplementary Information; e.g., Reeburgh, 2007). However, AOM would be substantially suppressed when the sulfate concentration is <0.5 mM (Knittel and Boetius, 2009). The Permian-Triassic transition interval is characterized by the substantially low sulfate concentration in the oceans (0.6–2.8 mM) (Luo et al., 2010; Schobben et al., 2017; Stebbins et al., 2019). This estimated SO42– range is slightly higher than the threshold of the AOM rate. Nonetheless, the porewater sulfate concentration in the sediments may have quickly become <0.5 mM at a very shallow depth, due to sulfate consumption via decomposition of other organic substrates. The sedimentary AOM was consequently suppressed and the oceanic sediments might have been a larger CH4 source compared to in the modern oceans, further contributing to the elevated pCH4. The impact of “methanogenic burst” on the enhanced CH4 emissions should have been significant under such sulfate-depleted conditions with less AOM. Prevailing oceanic anoxia (e.g., Wignall and Hallam, 1992; Isozaki, 1997; Song et al., 2012b) also helped CH4 to escape from the oceans to the atmosphere (e.g., Ryskin, 2003).
Finally, the global CH4 budgets may have been disturbed by the terrestrial devastation and intense continental weathering (e.g., Algeo and Twitchett, 2010; Cao et al., 2019). The Permian-Triassic transition is characterized by the extensive vegetation collapse on lands and massive soil erosion on a global scale (Figure 8B; e.g., Retallack, 2005; Sephton et al., 2005; Benton and Newell, 2014). The destruction of terrestrial ecosystems and the decay of land plants in the aftermath of the extinction was claimed to bring the well-known Early Triassic “coal gap” (Retallack et al., 1996). Although the release of substantial amounts of Ni into the ocean-atmosphere during the Siberian Traps volcanism may have caused the “methanogenic burst”, it may also have contributed to the vegetation collapse because excess Ni is generally toxic to plants (Fielding et al., 2019). The vegetation collapse could have stimulated the destabilization of permafrost and the CH4 emissions in high latitudes according to the warming (Nauta et al., 2015), whereas the massive soil erosion might also have contributed to the atmospheric CH4 accumulation as aerated terrestrial soils are a CH4 sink (Supplementary Information; Figure 8B).
Conclusions
The carbon isotopic composition of carbonate (δ13Ccarb) across the Permian-Triassic boundary (P-TB) was analyzed at Chaotian, Sichuan, South China, and was correlated to the chemostratigraphy of the carbon isotopic composition of organic carbon (δ13Corg) of the same interval. The δ13Ccarb and δ13Corg records at Chaotian were further integrated into the records from various marine and terrestrial environments all around the world, to examine fluctuations in the global methane (CH4) cycle during the Permian-Triassic transition. The following results were obtained:
(1) The δ13Ccarb values decrease from ca. +1 to −2‰ across the P-TB, possibly reflecting the shallow-marine extinction and the collapse of primary productivity in the oceans. The frequent intercalation of felsic tuff layers around the extinction horizon suggests that volcanic activity also contributed to the δ13Ccarb decrease.
(2) The magnitude of the δ13Ccarb decline (∼3‰) is substantially smaller than the magnitude of the δ13Corg decrease (∼7‰) across the P-TB. This δ13Ccarb-δ13Corg decoupling could be explained by an elevated CO2 concentration in the ocean/atmosphere and/or proliferation of methanogen (“methanogenic burst”) in the sediments, according to the Siberian Traps volcanism.
(3) A global P-TB δ13C compilation shows a large variation in marine δ13Corg records, which could be attributed to several potential mechanisms including eustatic sea-level changes and proliferation of green sulfur bacteria. We infer that the “methanogenic burst” may also have contributed, at least in part, to the δ13Corg variability. The global CH4 cycle might have fluctuated substantially in the aftermath of the extinction.
Data Availability Statement
The original contributions presented in the study are included in the article/Supplementary Material, further inquiries can be directed to the corresponding author.
Author Contributions
MS designed the study. YI provided the samples for the analyses. MS and YI conducted the lithofacies description. MS conducted the isotopic analyses. MS and YI wrote the manuscript.
Funding
This study was supported by JSPS KAKENHI (16204040, 20224012, 26610159, and 15H03740).
Conflict of Interest
The authors declare that the research was conducted in the absence of any commercial or financial relationships that could be construed as a potential conflict of interest.
Acknowledgments
Naomi Takahashi assisted with the isotopic analyses. Shuzhong Shen and two anonymous reviewers provided constructive comments that improved the article.
Supplementary Material
The Supplementary Material for this article can be found online at: https://www.frontiersin.org/articles/10.3389/feart.2020.596178/full#supplementary-material.
References
Aben, R. C. H., Barros, N., van Donk, E., Frenken, T., Hilt, S., Kazanjian, G., et al. (2017). Cross continental increase in methane ebullition under climate change. Nat. Commun. 8, 1682. doi:10.1038/s41467-017-01535-y
Algeo, T. J., Chen, Z. Q., Fraiser, M. L., and Twitchett, R. J. (2011). Terrestrial–marine teleconnections in the collapse and rebuilding of Early Triassic marine ecosystems. Palaeogeogr. Palaeoclimatol. Palaeoecol. 308, 1–11. doi:10.1016/j.palaeo.2011.01.011
Algeo, T. J., Ellwood, B., Nguyen, T. K. T., Rowe, H., and Maynard, J. B. (2007a). The Permian– Triassic boundary at Nhi Tao, Vietnam: evidence for recurrent influx of sulfidic watermasses to a shallow-marine carbonate platform. Palaeogeogr. Palaeoclimatol. Palaeoecol. 252, 304–327. doi:10.1016/j.palaeo.2006.11.055
Algeo, T. J., Hannigan, R., Rowe, H., Brookfield, M., Baud, A., Krystyn, L., et al. (2007b). Sequencing events across the permian–triassic boundary, guryul ravine (Kashmir, India). Palaeogeogr. Palaeoclimatol. Palaeoecol. 252, 328–346. doi:10.1016/j.palaeo.2006.11.050
Algeo, T. J., Henderson, C. M., Ellwood, B., Rowe, H., Elswick, E., Bates, S., et al. (2012). Evidence for a diachronous Late Permian marine crisis from the Canadian Arctic region. GSA Bull. 124, 1424–1448. doi:10.1130/B30505.1
Algeo, T. J., and Maynard, B. (2004). Trace-element behavior and redox facies in core shales of Upper Pennsylvanian Kansas-type cyclothems. Chem. Geol. 206, 289–318. doi:10.1016/j.chemgeo.2003.12.009
Algeo, T. J., Shen, Y., Zhang, T. G., Lyons, T. W., Bates, S., Rowe, H., et al. (2008). Association of 34S-depleted pyrite layers with negative carbonate δ13C excursions at the Permian-Triassic boundary: evidence for upwelling of sulfidic deep-ocean water masses. Geochem. Geophys. Geosyst. 9, Q04025. doi:10.1029/2007GC001823
Algeo, T. J., and Twitchett, R. J. (2010). Anomalous Early Triassic sediment fluxes due to elevated weathering rates and their biological consequences. Geology. 38, 1023–1026. doi:10.1130/G31203.1
Alroy, J. (2010). The shifting balance of diversity among major marine animal groups. Science 276, 235–238. doi:10.1126/science.1189910
Bagherpour, B., Bucher, H., Vennemann, T., Schneebeli-Hermann, E., Yuan, D. X., Leu, M., et al. (2019). Are Late Permian carbon isotope excursions of local or of global significance? GSA Bull. 132, 521–544. doi:10.1130/B31996.1
Bai, X., Luo, G. M., Wu, X., Wang, Y. Z., Huang, J. H., and Wang, X. J. (2008). Carbon isotope records indicative of paleoceanographical events at the latest permian Dalong formation at Shangsi, northeast sichuan, China. J. Chin. Univ. Geosci. 19, 481–487. doi:10.1016/S1002-0705(08)60053-9
Bambach, R. K. (2006). Phanerozoic biodiversity mass extinctions. Ann. Rev. Earth Planet. Sci. 34, 127–155. doi:10.1146/annurev.earth.33.092203.122654
Baresel, B., Bucher, H., Bagherpour, B., Brosse, M., Guodun, K., and Schaltegger, U. (2017). Timing of global regression and microbial bloom linked with the Permian-Triassic boundary mass extinction: implications for driving mechanisms. Sci. Rep. 7:43630. doi:10.1038/srep43630
Basiliko, N., and Yavitt, J. B. (2001). Influence of Ni, Co, Fe, and Na additions on methane production in Sphagnum-dominated Northern American peatlands. Biogeochemistry 52, 133–153. doi:10.1023/A:1006461803585
Baud, A., Atudorei, V., and Sharp, Z. (1996). Late Permian and Early Triassic evolution of the Northern Indian margin: carbon isotope and sequence stratigraphy. Geodinam. Acta 9, 57–77. doi:10.1080/09853111.1996.11105278
Baud, A., Magaritz, M., and Holser, W. T. (1989). Permian–Triassic of the Tethys: carbon isotope studies. Geol. Rundsch. 78, 649–677.
Baud, A., Richoz, S., Beauchamp, B., Cordey, F., Grasby, S., Henderson, C. M., et al. (2012). The buday’ah formation, sultanate of Oman: a middle permian to early triassic oceanic record of the Neotethys and the late induan microsphere bloom. J. Asian Earth Sci. 43, 130–144. doi:10.1016/j.jseaes.2011.08.016
Beauchamp, B., Henderson, C. M., Grasby, S. E., Gates, L. T., Beatty, T. W., Utting, J., et al. (2009). Late permian sedimentation in the Sverdrup Basin, Canadian Arctic: the lindström and black stripe formations. Bull. Can. Petrol. Geol. 57, 167–191. doi:10.2113/gscpgbull.57.2.167
Becker, L., Poreda, R. J., Basu, A. R., Pope, K. O., Harrison, T. M., Nicholson, C., et al. (2004). Bedout: a possible end-Permian impact crater offshore of northwestern Australia. Science 304, 1469–1476. doi:10.1126/science.1093925
Benton, M. J., and Newell, A. J. (2014). Impacts of global warming on Permo-Triassic terrestrial ecosystems. Gondwana Res. 25, 1308–1337. doi:10.1016/j.gr.2012.12.010
Berner, R. A. (2002). Examination of hypotheses for the Permo–Triassic boundary extinction by carbon cycle modeling. Proc. Natl. Acad. Sci. USA 99, 4172–4177. doi:10.1073/pnas.032095199
Bidigare, R. R., Fluegge, A., Freeman, K. H., Hanson, K. L., Hayes, J. M., Hollander, D., et al. (1997). Consistent fractionation of 13C in nature and in the laboratory: growth-rate effects in some haptophyte algae. Glob. Biogeochem. Cycles 11, 279–292. doi:10.1029/96gb03939
Black, B. A., Neely, R. R., Lamarque, J., Elkins-Tanton, L. T., Kiehl, J. T., Shields, C. A., et al. (2018). Systemic swings in end-Permian climate from Siberian Traps carbon and sulfur outgassing. Nat. Geosci. 11, 949–954. doi:10.1038/s41561-018-0261-y
Bond, D. P. G., and Wignall, P. B. (2014). “Large igneous provinces and mass extinctions: an update.” in Volcanism, impacts, and mass extinctions: causes and effects. Spec. Pap., Editors G. Keller, and A. C. Kerr, Geol. Soc. Am. Vol 505, 29–55. doi:10.1130/2014.2505(02)
Brand, U., Blamey, N., Garbelli, C., Griesshaber, E., Posenato, R., Angiolini, L., et al. (2016). Methane Hydrate: killer cause of Earth’s greatest mass extinction. Palaeoworld 25, 496–507. doi:10.1016/j.palwor.2016.06.002
Brand, U., Posenato, R., Came, R., Affek, H., Angiolini, L., Azmy, K., et al. (2012). The end‐Permian mass extinction: a rapid volcanic CO2 and CH4‐climatic catastrophe. Chem. Geol. 322-323, 121–144. doi:10.1016/j.chemgeo.2012.06.015
Buggisch, W., Krainer, K., Schaffhauser, M., Joachimski, M., and Korte, C. (2015). Late Carboniferous to Late Permian carbon isotope stratigraphy: A new record from post-Variscan carbonates from the Southern Alps (Austria and Italy). Palaeogeogr. Palaeoclimatol. Palaeoecol. 433, 174–190. doi:10.1016/j.palaeo.2015.05.012
Burgess, S. D., and Bowring, S. A. (2015). High-precision geochronology confirms voluminous magmatism before, during, and after Earth’s most severe extinction. Sci. Adv. 1:e1500470. doi:10.1126/sciadv.1500470
Campbell, I. H., Czamanske, G. K., Fedorenko, V. A., Hill, R. I., and Stepanov, V. (1992). Synchronism of the siberian traps and the permian-triassic boundary. Science 258, 1760–1763. doi:10.1126/science.258.5089.1760
Cao, C. Q., Wang, W., and Jin, Y. G. (2002). Carbon isotope excursions across the permian-triassic boundary in the meishan section, zhejiang province, China. Chin. Sci. Bull. 47, 1125–1129.
Cao, C. Q., Yang, Y. C., Shen, S. Z., Wang, W., Zheng, Q. F., and Summons, R. E. (2010). Pattern of δ13Ccarb and implications for geological events during the Permian-Triassic transition in South China. Geol. J. 45, 186–194. doi:10.1002/gj.1220
Cao, Y., Song, H., Algeo, T. J., Chu, D., Du, Y., Tian, L., et al. (2019). Intensified chemical weathering during the Permian-Triassic transition recorded in terrestrial and marine successions. Palaeogeogr. Palaeoclimatol. Palaeoecol. 519, 166–177. doi:10.1016/j.palaeo.2018.06.012
Clapham, M. E., and Payne, J. L. (2011). Acidification, anoxia, and extinction: a multiple logistic regression analysis of extinction selectivity during the Middle and Late Permian. Geology 39, 1059–1062. doi:10.1130/G32230.1
Clarkson, M. O., Kasemann, S. A., Wood, R. A., Lenton, T. M., Daines, S. J., Richoz, S., et al. (2015). Ocean acidification and the Permo-Triassic mass extinction. Science 348, 229–232. doi:10.1126/science.aaa0193
Coney, L., Reimold, W. U., Hancox, P. J., Mader, D., Koeberl, C., McDonald, I., Struck, U., Vajda, V., and Kamo, S. L. (2007). Geochemical and mineralogical investigation of the Permian‒Triassic boundary in the continental realm of the southern Karoo Basin, South Africa. Palaeoworld Rep. 16, 67–104.
Conrad, R. (2009). The global methane cycle: recent advances in understanding the microbial processes involved. Environ. Microbiol. Rep. 1, 285–292. doi:10.1111/j.1758-2229.2009.00038.x
Cui, Y., Bercovici, A., Yu, J. X., Kump, L. R., Freeman, K. H., Su, S. G., et al. (2017). Carbon cycle perturbation expressed in terrestrial Permian–Triassic boundary sections in South China. Glob. Planet. Change 148, 272–285. doi:10.1016/j.gloplacha.2015.10.018
Cui, Y., and Kump, L. R. (2015). Global warming and the end-Permian extinction event: proxy and modeling perspectives. Earth-Sci. Rev. 149, 5–22. doi:10.1016/j.earscirev.2014.04.007
Cui, Y., Kump, L. R., and Ridgwell, A. (2013). Initial assessment of the carbon emission rate and climatic consequences during the end-Permian mass extinction. Palaeogeogr. Palaeoclimatol. Palaeoecol. 389, 128–136. doi:10.1016/j.palaeo.2013.09.001
de Wit, M. J., Ghosh, J. G., de Villiers, S., Rakotosolofo, N., Alexander, J., Tripathi, A., et al. (2002). Multiple organic carbon isotope reversals across the Permian– Triassic boundary of terrestrial Gondwana Sequences: clues to extinction patterns and delayed ecosystem recovery. J. Geol. 110, 227–240.
Diekert, G., Konheiser, U., Piechulla, K., and Thauer, R. K. (1981). Nickel requirement and factor F430 content of methanogenic bacteria. J. Bacteriol. 148, 459–464.
Elkins-Tanton, L. T., Grasby, S. E., Black, B. A., Veselovskiy, R. V., Ardakani, O. H., and Goodarzi, F. (2020). Field evidence for coal combustion links the 252 Ma Siberian Traps with global carbon disruption. Geology 48, 986–991. doi:10.1130/G47365.1
Erwin, D. H., Bowring, S. A., and Yugan, J. (2002). “End-Permian mass extinctions: a review.” in Catastrophic events and mass extinctions: impacts and beyond: boulder, Colorado. Editors C. Koeberl, and K. G. MacLeod, (Boulder: Geol. Soc. Am. Spec. Pap.) 356, pp. 363–383.
Erwin, D. H. (2006). Extinction; how life on Earth nearly ended 250 million years ago. New Jersey, United States: Princeton Univ. Press, 296 p.
Erwin, D. H. (1993). The great paleozoic crisis. New York, United States: Columbia Univ. Press, 327 p.
Faure, K., de Wit, M. J., and Willis, J. P. (1995). Late Permian global coal hiatus linked to 13C-depleted CO2 flux into the atmosphere during the final consolidation of Pangea. Geology 23, 507–510.
Fielding, C. R., Frank, T. D., McLoughlin, S., Vajda, V., Mays, C., Tevyaw, A. P., et al. (2019). Age and pattern of the southern high-latitude continental end-Permian extinction constrained by multiproxy analysis. Nat. Commun. 10:385. doi:10.1038/s41467-018-07934-z
Foster, C. B., Logan, G. A., Summons, R. E., Gorter, J. D., and Edwards, D. S. (1997). Carbon isotopes, kerogen types and the Permian–Triassic boundary in Australia: implications for exploration. Aus. Petrol. Product. Explor. Assoc. J. 37, 472–489.
Garbelli, C., Angiolini, L., and Shen, S. Z. (2017). Biomineralization and global change: a new perspective for understanding the end-Permian extinction. Geology 45, 19–22. doi:10.1130/G38430.1
Gastaldo, R. A., Kamo, S. L., Neveling, J., Geissman, J. W., Looy, C. V., and Martini, A. M. (2020). The base of the lystrosaurus assemblage zone, karoo basin, predates the end-permian marine extinction. Nat. Commun. 11, 1428. doi:10.1038/s41467-020-15243-7
Grasby, S. E., and Beauchamp, B. (2008). Intrabasin variability of the carbon-isotope record across the permian–triassic transition, sverdrup basin, arctic Canada. Chem. Geol. 253, 141–150. doi:10.1016/j.chemgeo.2008.05.005
Grasby, S. E., Sanei, H., and Beauchamp, B. (2011). Catastrophic dispersion of coal fly ash into oceans during the latest Permian extinction. Nat. Geosci. 4, 104–107. doi:10.1038/ngeo1069
Grice, K., Cao, C. Q., Love, G. D., Böttcher, M. E., Twitchett, R. J., Grosjean, E., et al. (2005). Photic zone euxinia during the Permian-Triassic superanoxic event. Science. 307, 706–709. doi:10.1126/science.1104323
Hallam, A., and Wignall, P. B. (1999). Mass Extinctions and sea-level changes. Earth-Sci. Rev. 48, 217–250. doi:10.1016/S0012-8252(99)00055-0
Hallam, A., and Wignall, P. B. (1997). Mass extinctions and their aftermath. Oxford, UK: Oxford Univ. Press, 320 p.
Hansen, H. J. (2006). Stable isotopes of carbon from basaltic rocks and their possible relation to atmospheric isotope excursions. Lithos 92, 105–116. doi:10.1016/j.lithos.2006.03.029
Haq, B. U., and Schutter, S. R. (2008). A chronology of paleozoic sea-level changes. Science 322, 64–68. doi:10.1126/science.1161648
Hayes, J. M., Popp, B. N., Takigiku, R., and Johnson, M. W. (1989). An isotopic study of biogeochemical relationships between carbonates and organic carbon in the Greenhorn Formation. Geochim. Cosmochim. Acta 53, 2961–2972.
Hayes, J. M., Strauss, H., and Kaufman, A. J. (1999). The abundance of 13C in marine organic matter and isotopic fractionation in the global biogeochemical cycle of carbon during the past 800 Ma. Chem. Geol. 161, 103–125. doi:10.1016/S0009-2541(99)00083-2
Heydari, E., and Hassanzadeh, J. (2003). Deev Jahi Model of the Permian–Triassic boundary mass extinction: a case for gas hydrates as the main cause of biological crisis on Earth. Sed. Geol. 163, 147–163. doi:10.1016/j.sedgeo.2003.08.002
Heydari, E., Wade, W. J., and Hassanzadeh, J. (2001). Diagenetic origin of carbon and oxygen isotope compositions of Permian–Triassic boundary strata. Sed. Geol. 143, 191–197. doi:10.1016/S0037-0738(01)00095-1
Holser, W. T., Schönlaub, H.-P., Attrep, M., Boeckelmann, K., Klein, P., Magaritz, M., et al. (1989). A unique geochemical record at the Permian/Triassic boundary. Nature 337, 39–44.
Horacek, M., Brandner, R., and Abart, R. (2007b). Carbon isotope record of the P/T boundary and the Lower Triassic in the Southern Alps: evidence for rapid changes in storage of organic carbon. Palaeogeogr. Palaeoclimatol. Palaeoecol. 252, 347–354. doi:10.1016/j.palaeo.2006.11.049
Horacek, M., Richoz, S., Brandner, R., Krystyn, L., and Spötl, C. (2007a). Evidence for recurrent changes in Lower Triassic oceanic circulation of the Tethys: the δ13C record from marine sections in Iran. Palaeogeogr. Palaeoclimatol. Palaeoecol. 252, 355–369. doi:10.1016/j.palaeo.2006.11.052
Hudspith, V. A., Rimmer, S. M., and Belcher, C. M. (2014). Latest Permian chars may derive from wildfires, not coal combustion. Geology 42, 879–882. doi:10.1130/G35920.1
Isozaki, Y. (2019). “End-paleozoic mass extinction: hierarchy of causes and a new cosmoclimatological perspective for the largest crisis.” in Astrobiology. Editors A. Yamagishi, T. Kakegawa, and T. Usui. (Singapore: Springer), 273–301. doi:10.1007/978-981-13-3639-3_18
Isozaki, Y. (1997). Permo-Triassic boundary Superanoxia and stratified superocean: records from lost deep-sea. Science 276, 235–238. doi:10.1126/science.276.5310.235
Isozaki, Y., Shimizu, N., Yao, J. X., Ji, Z. S., and Matsuda, T. (2007). End-Permian extinction and volcanism-induced environmental stress: the Permian-Triassic boundary interval of lower-slope facies at Chaotian, South China. Palaeogeogr. Palaeoclimatol. Palaeoecol. 252, 218–238. doi:10.1016/j.palaeo.2006.11.051
Isozaki, Y., Yao, J. X., Ji, Z. S., Saitoh, M., Kobayashi, N., and Sakai, H. (2008). Rapid sea-level change in the late guadalupian (permian) on the tethyan side of South China: litho- and biostratigraphy of the chaotian section in sichuan. Proc. Jpn. Acad. Ser. B. 84, 344–353. doi:10.2183/pjab/84.344
Isozaki, Y., Yao, J. X., Matsuda, T., Sakai, H., Ji, Z. S., Shimizu, N., et al. (2004). Stratigraphy of the middle-upper permian and lowermost triassic at chaotian, sichuan, China. –Record of late permian double mass extinction event–. Proc. Jpn. Acad. Ser. B. 80, 10–16. doi:10.2183/pjab.80.10
Ji, Z. S., Yao, J. X., Isozaki, Y., Matsuda, T., and Wu, G. C. (2007). Conodont biostratigraphy across the permian-triassic boundary at chaotian, in northern sichuan, China. Palaeogeogr. Palaeoclimatol. Palaeoecol. 252, 39–55. doi:10.1016/j.palaeo.2006.11.033
Jin, Y. G., Mei, S. L., Wang, W., Wang, X. D., Shen, S. Z., Shang, Q. H., et al. (1998). On the Lopingian series of the Permian system. Palaeoworld 9, 1–18.
Jin, Y. G., Wang, Y., Wang, W., Shang, Q. H., Cao, C. Q., and Erwin, D. H. (2000). Pattern of marine mass extinction near the Permian–Triassic boundary in South China. Science 289, 432–436. doi:10.1126/science.289.5478.432
Joachimski, M. M., Alekseev, A. S., Grigoryan, A., and Gatovsky, Y. A. (2020). Siberian Trap volcanism, global warming and the Permian-Triassic mass extinction: new insights from Armenian Permian-Triassic sections. GSA Bull. 132, 427–443. doi:10.1130/B35108.1
Joachimski, M. M., Lai, X. L., Shen, S. Z., Jiang, H. S., Luo, G. M., Chen, B., et al. (2012). Climate warming in the latest Permian and the Permian-Triassic mass extinction. Geology 40, 195–198. doi:10.1130/G32707.1
Jost, A. B., Mundil, R., He, B., Brown, S. T., Altiner, D., Sun, Y. D., et al. (2014). Constraining the cause of the end-Guadalupian extinction with coupled records of carbon and calcium isotopes. Earth Planet Sci. Lett. 396, 201–212. doi:10.1016/j.epsl.2014.04.014
Jurikova, H., Gutjahr, M., Wallmann, K., Flögel, S., Liebetrau, V., Posenato, R., et al. (2020). Permian–Triassic mass extinction pulses driven by major marine carbon cycle perturbations. Nat. Geosci. 13, 745–750. doi:10.1038/s41561-020-00646-4
Kaiho, K., Chen, Z. Q., and Sawada, K. (2009). Possible causes for a negative shift in the stable carbon isotope ratio before, during and after the end-Permian mass extinction in Meishan, South China. Aus. J. Earth Sci. 56, 799–808. doi:10.1080/08120090903002615
Kaiho, K., Saito, R., Ito, K., Miyaji, T., Biswas, R., Tian, L., et al. (2016). Effects of soil erosion and anoxic–euxinic ocean in the Permian–Triassic marine crisis. Heliyon 2, e00137. doi:10.1016/j.heliyon.2016.e00137
Kajiwara, Y., Yamakita, S., Ishida, K., Ishiga, H., and Imai, A. (1994). Development of a largely anoxic stratified ocean and its temporary massive mixing at the Permian/Triassic boundary supported by the sulfur isotopic record. Palaeogeogr. Palaeoclimatol. Palaeoecol. 111, 367–379. doi:10.1016/0031-0182(94)90072-8
Kamo, S. L., Czamanske, G. K., Amelin, Y., Fedorenko, V. A., Davis, D. W., and Trofimov, V. R. (2003). Rapid eruption of Siberian flood-volcanic rocks and evidence for coincidence with the Permian–Triassic boundary and mass extinction at 251 Ma. Earth Planet Sci. Lett. 214 (1–2), 75–91. doi:10.1016/S0012-821X(03)00347-9
Kidder, D. L., and Worsley, T. R. (2004). Causes and consequences of extreme Permo-Triassic warming to globally equable climate and relation to the Permo-Triassic extinction and recovery. Palaeogeogr. Palaeoclimatol. Palaeoecol. 203, 207–237. doi:10.1016/S0031-0182(03)00667-9
Kirschke, S., Bousquet, P., Ciais, P., Saunois, M., Canadell, J. G., Dlugokencky, E. J., et al. (2013). Three decades of global methane sources and sinks. Nat. Geosci. 6, 813–823. doi:10.1038/ngeo1955
Knauth, L. P., and Kennedy, M. J. (2009). The late Precambrian greening of the Earth. Nature 460, 728–732. doi:10.1038/nature08213
Knittel, K., and Boetius, A. (2009). Anaerobic oxidation of methane: progress with an unknown process. Ann. Rev. Microbiol. 63, 311–334. doi:10.1146/annurev.micro.61.080706.093130
Knoll, A. H., Bambach, R. K., Canfield, D. E., and Grotzinger, J. P. (1996). Comparative earth history and late permian mass extinction. Science 273, 452–457. doi:10.1126/science.273.5274.452
Knoll, A. H., Bambach, R. K., Payne, J. L., Pruss, S., and Fischer, W. W. (2007). Paleophysiology and end-Permian mass extinction. Earth Planet Sci. Lett. 256, 295–313. doi:10.1016/j.epsl.2007.02.018
Korte, C., and Kozur, H. W. (2010). Carbon-isotope stratigraphy across the Permian-Triassic boundary: a review. J. Asian Earth Sci. 39, 215–235. doi:10.1016/j.jseaes.2010.01.005
Korte, C., Kozur, H. W., and Mohtat-Aghai, P. (2004). Dzhulfian to lowermost Triassic δ13C record at the Permian/Triassic boundary section at Shahreza, Central Iran. Hallesch. Jahr. Geowissensch. 18, 73–78.
Korte, C., Veizer, J., Leythaeuser, D., Below, R., and Schwark, L. (2001). Evolution of Permian and Lower Triassic δ13C in marine and terrigenous organic material. Terra Nostra 2001(4), 30–34.
Krull, E. S., Lehrmann, D. J., Druke, D., Kessel, B., Yu, Y. Y., and Li, R. X. (2004). Stable carbon isotope stratigraphy across the Permian–Triassic boundary in shallow marine carbonate platforms, Nanpanjiang Basin, south China. Palaeogeogr. Palaeoclimatol. Palaeoecol. 204, 297–315. doi:10.1016/S0031-0182(03)00732-6
Krull, E. S., Retallack, G. J., Campbell, H. J., and Lyon, G. L. (2000a). δ13Corg chemostratigraphy of the Permian–Triassic boundary in the Maitai Group, New Zealand: evidence for high-latitudinal methane release. New Zealand J. Geol. Geophys. 43, 21–32. doi:10.1080/00288306.2000.9514868
Krull, E. S., Retallack, G. J., Campbell, H. J., and Lyon, G. L. (2000b). δ13C depth profiles from paleosols across the Permian–Triassic boundary: evidence for methane release. GSA Bull. 112, 1459–1472. doi:10.1130/0016-7606(2000)112
Kump, L. R., and Arthur, M. A. (1999). Interpreting carbon-isotope excursions: carbonates and organic matter. Chem. Geol. 161, 181–198. doi:10.1016/S0009-2541(99)00086-8
Kump, L. R. (1991). Interpreting carbon-isotope excursion: strangelove oceans. Geology 19, 299–302. doi:10.1130/0091-7613(1991)019
Kump, L. R., Pavlov, A., and Arthur, M. A. (2005). Massive release of hydrogen sulfide to the surface ocean and atmosphere during intervals of oceanic anoxia. Geology 33, 397–400. doi:10.1130/G21295.1
Lai, X. L., Wang, W., Wignall, P. B., Bond, D. P. G., Jiang, H. S., Ali, J. R., et al. (2008). Palaeoenvironmental change during the end-Guadalupian (Permian) mass extinction in Sichuan, China. Palaeogeogr. Palaeoclimatol. Palaeoecol. 269, 78–93. doi:10.1016/j.palaeo.2008.08.005
Laws, E. A., Popp, B. N., Bidigare, R. R., Kennicutt, M. C., and Macko, S. A. (1995). Dependence of phytoplankton carbon isotopic composition on growth rate and [CO2]aq:theoretical considerations and experimental results. Geochim. Cosmochim. Acta 59, l l31-1138.
Le Vaillant, M., Barnes, S. J., Mungall, J. E., and Mungall, E. L. (2017). Role of degassing of the Noril’sk nickel deposits in the Permian–Triassic mass extinction event. Proc. Natl. Acad. Sci. USA 114, 2485–2490. doi:10.1073/pnas.1611086114
Li, R., and Jones, B. (2017). Diagenetic overprint on negative δ13C excursions across the Permian/Triassic boundary: a case study from Meishan section, China. Palaeogeogr. Palaeoclimatol. Palaeoecol. 299, 70–82. doi:10.1016/j.palaeo.2016.11.044
Liao, Z. W., Hu, W. X., Cao, J., Wang, X. L., Yao, S., and Wan, Y. (2016). Permian–Triassic boundary (PTB) in the Lower Yangtze Region, southeastern China: a new discovery of deep-water archive based on organic carbon isotopic and U–Pb geochronological studies. Palaeogeogr. Palaeoclimatol. Palaeoecol. 451, 124–139. doi:10.1016/j.palaeo.2016.03.004
Liu, X. C., Wang, W., Shen, S. Z., Gorgij, M. N., Ye, F. C., Zhang, Y. C., et al. (2013). Late Guadalupian to Lopingian (Permian) carbon and strontium isotopic chemostratigraphy in the Abadeh section, central Iran. Gondwana Res. 24, 222–232. doi:10.1016/j.gr.2012.10.012
Luo, G. M., Algeo, T. J., Huang, J. H., Zhou, W. F., Wang, Y. B., Yang, H., et al. (2014). Vertical δ13Corg gradients record changes in planktonic microbial community composition during the end-Permian mass extinction. Palaeogeogr. Palaeoclimatol. Palaeoecol. 396, 119–131. doi:10.1016/j.palaeo.2014.01.006
Luo, G. M., Kump, L. R., Wang, Y. B., Tong, J., Arthur, M. A., Yang, H., et al. (2010). Isotopic evidence for an anomalously low oceanic sulfate concentration following end-Permian mass extinction. Earth Planet Sci. Lett. 300, 101–111. doi:10.1016/j.epsl.2010.09.041
Magaritz, M., Bär, R., Baud, A., and Holser, W. T. (1988). The carbon-isotope shift at the Permian/Triassic boundary in the Southern Alps is gradual. Nature 331, 337–339.
Magaritz, M., Krishnamurthy, R. V., and Holser, W. T. (1992). Parallel trends in organic and inorganic carbon isotopes across the Permian/Triassic boundary. Am. J. Sci. 292, 727–739. doi:10.2475/ajs.292.10.727
Mii, H. S., Grossman, E. L., and Yancey, T. E. (1997). Stable carbon and oxygen isotope shifts in Permian seas of West Spitsbergen – global change or diagenetic artifact? Geology 25, 227–230. doi:10.1130/0091-7613(1997)025<0227:SCAOIS>2.3.CO;2
Morante, R.. (1996). Permian and Early Triassic isotopic records of carbon and strontium in Australia and a scenario of events about the Permian-Triassic boundary. Hist. Biol. 11, 289–310. doi:10.1080/10292389609380546
Musashi, M., Isozaki, Y., Koike, T., and Kreulen, R. (2001). Stable carbon isotope signature in mid-Panthalassa shallow-water carbonates across the Permo-Triassic boundary: evidence for 13C-depleted superocean. Earth Planet Sci. Lett. 191, 9–20. doi:10.1016/S0012-821X(01)00398-3
Muttoni, G., Gaetani, M., Kent, D. V., Sciunnach, D., Angiolini, L., Berra, F., et al. (2009). Opening of the neo-tethys ocean and the Pangea B to Pangea A transformation during the permian. GeoArabia 14, 17–48.
Nabbefeld, B., Grice, K., Schimmelmann, A., Sauer, P. E., Böttcher, M. E., and Twitchett, R. (2010). Significance of δDkerogen, δ13Ckerogen and δ34Spyrite from several Permian/Triassic (P/Tr) sections. Earth Planet Sci. Lett. 295, 21–29. doi:10.1016/j.epsl.2010.03.015
Nauta, A., Heijmans, M., Blok, D., Limpens, J., Elberling, B., Gallagher, A., et al. (2015). Permafrost collapse after shrub removal shifts tundra ecosystem to a methane source. Nat. Clim. Change 5, 67–70. doi:10.1038/nclimate2446
Nazaries, L., Murrell, J. C., Millard, P., Baggs, L., and Singh, B. K. (2013). Methane, microbes and models: fundamental understanding of the soil methane cycle for future predictions. Environ. Microbiol. 15, 2395–2417. doi:10.1111/1462-2920.12149
Ogden, D. E., and Sleep, N. H. (2012). Explosive eruption of coal and basalt and the end-Permian mass extinction. Proc. Natl. Acad. Sci. USA 109, 59–62. doi:10.1073/pnas.1118675109
Oh, Y., Zhuang, Q., Liu, L., Welp, L. R., Lau, M. C. Y., Onstott, T. C., et al. (2020). Reduced net methane emissions due to microbial methane oxidation in a warmer Arctic. Nat. Clim. Chang. 10, 317–321. doi:10.1038/s41558-020-0734-z
Payne, J. L., and Clapham, M. E. (2012). End-Permian mass extinction in the oceans: an ancient analog for the twenty-first century? Ann. Rev. Earth Planet. Sci. 40, 89–111. doi:10.1146/annurev-earth-042711-105329
Payne, J. L., Lehrmann, D. J., Follett, D., Seibel, M., Kump, L. R., Riccardi, A., et al. (2007). Erosional truncation of uppermost Permian shallow-marine carbonates and implications for Permian-Triassic boundary events. GSA Bull. 119, 771–784. doi:10.1130/B26091.1
Polozov, A. G., Svensen, H. H., Planke, S., Grishina, S. N., Fristad, K. E., and Jerram, D. A. (2016). The basalt pipes of the Tunguska Basin (Siberia, Russia): high temperature processes and volatile degassing into the end-Permian atmosphere. Palaeogeogr. Palaeoclimatol. Palaeoecol. 441, 51–64. doi:10.1016/j.palaeo.2015.06.035
Popp, B. N., Takigiku, R., Hayes, J. M., Louda, J. W., and Baker, E. W. (1989). The post-Paleozoic Chronology and Mechanism of 13C Depletion in primary marine organic matter. Am. J. Sci. 289, 436–454.
Preuss, A., Schauder, R., Fuchs, G., and Stichler, W. (1989). Carbon isotope fractionation by autotrophic bacteria with three different CO2 fixation pathways. Z. Naturforsch. 44c, 397–402.
Racki, G. (2020). “Big 5 mass extinctions.” in Encyclopedia of geology. 2nd Edition, Editors S. Elias, and D. D. Alderton, (Newyork, United States: Elsevier), pp. 1–14. doi:10.1016/B978-0-12-409548-9.12028.7
Rampino, M. R., and Caldeira, K. (2005). Major perturbation of ocean chemistry and a ‘Strangelove Ocean’ after the end-Permian mass extinction. Terra. Nova 17, 554–559. doi:10.1111/j.1365-3121.2005.00648.x
Rampino, M. R., Rodriguez, S., Baransky, E., and Cai, Y. (2017). Global nickel anomaly links Siberian Traps eruptions and the latest Permian mass extinction. Sci. Rep. 7, 12416. doi:10.1038/s41598-017-12759-9
Rau, G. H., Riebesell, U., and Wolf-Gladrow, D. (1997). CO2,aq-dependent photosynthetic 13C fractionation in the ocean: A model versus measurements. Global Biogeochem. Cycles 11, 267–278. doi:10.1029/97GB00328
Rau, G. H., Takahaski, T., Des Marais, D. J., Repeta, D. J., and Martin, J. H. (1992). The relationship between δ13C of organic matter and [CO2,aq] in ocean surface water: data from a JGOFS site in the northeast Atlantic Ocean and a model. Geochim. Cosmochim. Acta 56, 1413–1419. doi:10.1016/0016-7037(92)90073-R
Reeburgh, W. S. (2007). Oceanic methane biogeochemistry. Chem. Rev. 107, 486–513. doi:10.1021/cr050362v
Reichow, M. K., Pringle, M. S., Al'Mukhamedov, A. I., Allen, M. B., Andreichev, V. L., Buslov, M. M., et al. (2009). The timing and extent of the eruption of the Siberian Traps large igneous province: implications for the end-Permian environmental crisis. Earth Planet Sci. Lett. 277, 9–20. doi:10.1016/j.epsl.2008.09.030
Renne, P. R., and Basu, A. R. (1991). Rapid eruption of the Siberian traps flood basalts at the Permian-Triassic boundary. Science 253, 176–179. doi:10.1126/science.253.5016.176
Renne, P. R., Zichao, Z., Richards, M. A., Black, M., and Basu, A. R. (1995). Synchrony and causal relations between Permian-Triassic boundary crises and Siberian flood volcanism. Science 269, 1413–1416. doi:10.1126/science.269.5229.1413
Retallack, G. J. (2005). Earliest triassic claystone breccias and soil-erosion crisis. J. Sed. Res. 75, 679–695. doi:10.2110/jsr.2005.055
Retallack, G. J., and Jahren, A. H. (2008). Methane release from igneous intrusion of coal during Late Permian extinction events. J. Geol. 116, 1–20. doi:10.1086/524120
Retallack, G. J., Jahren, A. H., Sheldon, N. D., Chakrabarti, R., Metzger, C. A., and Smith, R. M. H. (2005). The permian–triassic boundary in Antarctica. Antarctic Sci. 17, 241–258. doi:10.1017/S0954102005002658
Retallack, G. J., and Krull, E. S. (2006). “Carbon isotopic evidence for terminal-Permian methane outbursts and their role in extinctions of animals, plants, coral reefs, and peat swamps.” in Wetlands through time. Editors S. F. Greb, and W. A. DiMichele, (Boulder: Geol. Soc. Am. Spec. Pap.) 399, 249–268. doi:10.1130/2006.2399(12
Retallack, G. J., Veevers, J. J., and Morante, R. (1996). Global coal gap between Permian–Triassic extinction and Middle Triassic recovery of peat-forming plants. GSA Bull. 108, 195–207. doi:10.1130/0016-7606(1996)108<0195:GCGBPT>2.3.CO;2
Riccardi, A., Kump, L. R., Arthur, M. A., and D’Hondt, S. (2007). Carbon isotopic evidence for chemocline upward excursions during the end-Permian event. Palaeogeogr. Palaeoclimatol. Palaeoecol. 248, 73–81. doi:10.1016/j.palaeo.2006.11.010
Richoz, S. (2006). Stratigraphie et variations isotopiques du carbone dans le Permien supérieur et le Trias inférieur de la Néotéthys (Turquie, Oman et Iran). Mem. Géol. 46, 251.
Rothman, D. H., Fournier, G. P., French, K. L., Alm, E. J., Boyle, E. A., Cao, C. Q., et al. (2014). Methanogenic burst in the end-Permian carbon cycle. Proc. Natl. Acad. Sci. USA 111, 5462–5467. doi:10.1073/pnas.1318106111
Ryskin, G. (2003). Methane-driven oceanic eruptions and mass extinctions. Geology 31, 741–744. doi:10.1130/G19518.1
Saitoh, M., Isozaki, Y., Ueno, Y., Yoshida, N., Yao, J. X., and Ji, Z. S. (2013b). Middle-Upper Permian carbon isotope stratigraphy at Chaotian, South China: pre-extinction multiple upwelling of oxygen-depleted water onto continental shelf. J. Asian Earth Sci. 67-68, 51–62. doi:10.1016/j.jseaes.2013.02.009
Saitoh, M., Isozaki, Y., Yao, J. X., Ji, Z. S., Ueno, Y., and Yoshida, N. (2013a). The appearance of an oxygen-depleted condition on the Capitanian disphotic slope/basin in South China: middle-Upper Permian stratigraphy at Chaotian in northern Sichuan. Glob. Planet. Change 105, 180–192. doi:10.1016/j.gloplacha.2012.01.002
Saitoh, M., Ueno, Y., Isozaki, Y., Nishizawa, M., Shozugawa, K., Kawamura, T., et al. (2014b). Isotopic evidence for water-column denitrification and sulfate reduction at the end-Guadalupian (Middle Permian). Glob. Planet. Change 123, 110–120. doi:10.1016/j.jseaes.2014.06.026
Saitoh, M., Ueno, Y., Isozaki, Y., Shibuya, T., Yao, J. X., Ji, Z. S., et al. (2015). Authigenic carbonate precipitation at the end-Guadalupian (Middle Permian) in China: implications for the carbon cycle in ancient anoxic oceans. Prog. Earth Planet. Sci. 2:41. doi:10.1186/s40645-015-0073-2
Saitoh, M., Ueno, Y., Matsu'ura, F., Kawamura, T., Isozaki, Y., Yao, J. X., et al. (2017). Multiple sulfur isotope records at the end-Guadalupian (Permian) at Chaotian, China: implications for a role of bioturbation in the Phanerozoic sulfur cycle. J. Asian Earth Sci. 135, 70–79. doi:10.1016/j.jseaes.2016.12.009
Saitoh, M., Ueno, Y., Nishizawa, M., Isozaki, Y., Takai, K., Yao, J. X., et al. (2014a). Nitrogen isotope chemostratigraphy across the permian-triassic boundary at chaotian, sichuan, south China. J. Asian Earth Sci. 93, 113–128. doi:10.1016/j.gloplacha.2014.10.014
Saltzman, M. R., and Sedlacek, A. R. C. (2013). Chemostratigraphy indicates a relatively complete Late Permian to Early Triassic sequence in the western United States. Geology 41, 399–402. doi:10.1130/G33906.1
Sarkar, A., Yoshioka, H., Ebihara, M., and Naraoka, H. (2003). Geochemical and organic carbon isotope studies across the continental Permo–Triassic boundary of Raniganj Basin, eastern India. Palaeogeogr. Palaeoclimatol. Palaeoecol. 191, 1–14. doi:10.1016/S0031-0182(02)00636-3
Saunders, A., and Reichow, M. (2009). The Siberian Traps and the End-Permian mass extinction: a critical review. Chin. Sci. Bull. 54, 20–37. doi:10.1007/s11434-008-0543-7
Schidlowski, M., Hayes, J. M., and Kaplan, I. R. (1983). “Isotopic inferences of ancient biochemistries: carbon, sulfur, hydrogen, and nitrogen.” in Earth's earliest biosphere. Editor J. W. Schopf (Princeton, NJ: Princeton University Press), 149–186.
Schneebeli-Hermann, E. (2012). Extinguishing a permian world. Geology 40, 287–288. doi:10.1130/focus032012.1
Schneebeli-Hermann, E., Kürschner, W. M., Hochuli, P. A., Ware, D., Weissert, H., Bernasconi, S. M., et al. (2013). Evidence for atmospheric carbon injection during the end-Permian extinction. Geology. 41, 579–582. doi:10.1130/G34047.1
Schobben, M., Stebbins, A., Algeo, T. J., Strauss, H., Leda, L., Haas, J., et al. (2017). Volatile earliest Triassic sulfur cycle: a consequence of persistent low seawater sulfate concentrations and a high sulfur cycle turnover rate? Palaeogeogr. Palaeoclimatol. Palaeoecol. 486, 74–85. doi:10.1016/j.palaeo.2017.02.025
Schobben, M., Stebbins, A., Ghaderi, A., Strauss, H., Korn, D., and Korte, C. (2015). Flourishing ocean drives the end-Permian marine mass extinction. Proc. Natl. Acad. Sci. USA. 112, 10298–10303. doi:10.1073/pnas.1503755112
Schobben, M., Ullmann, C. V., Leda, L., Korn, D., Struck, U., Reimold, W. U., et al. (2016). Discerning primary versus diagenetic signals in carbonate carbon and oxygen isotope records: an example from the Permian–Triassic boundary of Iran. Chem. Geol. 422, 94–107. doi:10.1016/j.chemgeo.2015.12.013
Scholze, F., Wang, X., Kirscher, U., Kraft, J., Schneider, J. W., Götz, A. E., et al. (2017). A multistratigraphic approach to pinpoint the Permian-Triassic boundary in continental deposits: the Zechstein–Lower Buntsandstein transition in Germany. Global Planet. Change. 152, 129–151. doi:10.1016/j.gloplacha.2017.03.004
Semrau, J. D., DiSpirito, A. A., and Yoon, S. (2010). Methanotrophs and copper. FEMS Microbiol. Rev. 34, 496–531. doi:10.1111/j.1574-6976.2010.00212.x
Sephton, M. A., Looy, C. V., Brinkhuis, H., Wignall, P. B., de Leeuw, J. W., and Visscher, H. (2005). Catastrophic soil erosion during the end-Permian biotic crisis. Geology. 33, 941–944. doi:10.1130/G21784.1
Sephton, M. A., Looy, C. V., Veefkind, R. J., Brinkhuis, H., de Leeuw, J. W., and Visscher, H. (2002). “Synchronous record of δ13C shifts in the oceans and atmosphere at the end of the Permian.” in Catastrophic events and mass extinctions: impacts and beyond., Editors C. Koeberl, and K. G. MacLeod (Boulder: Geol. Soc. Am. Spec. Pap.) Vol. 356, pp. 455–462.
Shao, L., Zhang, P. F., Dou, J. W., and Shen, S. Z. (2000). Carbon isotope compositions of the Late Permian carbonate rocks in southern China: their variations between the Wujiaping and Changxing formations. Palaeogeogr. Palaeoclimatol. Palaeoecol. 161, 179–192. doi:10.1016/S0031-0182(00)00122-X
Shen, J., Algeo, T. J., Hu, Q., Zhang, N., Zhou, L., Xia, W. C., et al. (2012). Negative C-isotope excursions at the Permian-Triassic boundary linked to volcanism. Geology 40, 963–966. doi:10.1130/G33329.1
Shen, S. Z., Cao, C. Q., Zhang, H., Bowring, S. A., Henderson, C. M., Payne, J. L., et al. (2013). High- resolution δ13Ccarb chemostratigraphy from latest Guadalupian through earliest Triassic in South China and Iran. Earth Planet Sci. Lett. 375, 156–165. doi:10.1016/j.epsl.2013.05.020
Shen, S. Z., Crowley, J. L., Wang, Y., Bowring, S. A., Erwin, D. H., Sadler, P. M., et al. (2011a). Calibrating the end-Permian mass extinction. Science 334, 1367–1372. doi:10.1126/science.1213454
Shen, W. J., Sun, Y. G., Lin, Y. T., Liu, D. H., and Chai, P. X. (2011b). Evidence for wildfire in the Meishan section and implications for Permian–Triassic events. Geochim. Cosmochim. Acta 75, 1992–2006. doi:10.1016/j.gca.2011.01.027
Shen, Y., Farquhar, J., Zhang, H., Masterson, A., Zhang, T. G., and Wing, B. A. (2011c). Multiple S-isotopic evidence for episodic shoaling of anoxic water during Late Permian mass extinction. Nat. Commun. 2, 210. doi:10.1038/ncomms1217
Siegert, S., Kraus, S. H., Mette, W., Struck, U., and Korte, C. (2011). Organic carbon isotope values from the Late Permian Seis/Siusi succession (Dolomites, Italy): implications for palaeoenvironmental changes. Fossil Record 14, 207–217. doi:10.1002/mmng.201100008
Singh, B. K., Bardgett, R. D., Smith, P., and Reay, D. S. (2010). Microorganisms and climate change: terrestrial feedbacks and mitigation options. Nat. Rev. Microbiol. 8, 779–790. doi:10.1038/nrmicro2439
Song, H. J., Tong, J. N., Xiong, Y. L., Sun, D. Y., Tian, L., and Song, H. Y. (2012a). The large increase of 13Ccarb-depth gradient and the end-Permian mass extinction. Sci. Chin. Earth Sci. 55, 1101–1109. doi:10.1007/s11430-012-4416-1
Song, H. J., Wignall, P. B., Tong, J., Bond, D. P. G., Song, H. Y., Lai, X. L., et al. (2012b). Geochemical evidence from bio-apatite for multiple oceanic anoxic events during Permian–Triassic transition and the link with end-Permian extinction and recovery. Earth Planet Sci. Lett. 353-354, 12–21. doi:10.1016/j.epsl.2012.07.005
Stanley, S. M.. (2016). Estimates of the magnitudes of major marine mass extinctions in earth history. Proc. Natl. Acad. Sci. USA 113, E6325–E6334. doi:10.1073/pnas.1613094113
Stebbins, A., Algeo, T. J., Olsen, C., Sano, H., Rowe, H., and Hannigan, R. (2019). Sulfur-isotope evidence for recovery of seawater sulfate concentrations from a PTB minimum by the Smithian-Spathian transition. Earth-Sci. Rev. 195, 83–95. doi:10.1016/j.earscirev.2018.08.010
Stocker, T. F., Qin, D., Plattner, G.-K., Tignor, M., Allen, S. K., Boschung, J., et al. (2014). Climate change 2013: the physical science basis. Cambridge, United Kingdom and New York, United States: Cambridge Univ. Press, 1535 pp.
Sun, Y., Joachimski, M. M., Wignall, P. B., Yan, C., Chen, Y., Jiang, H., et al. (2012). Lethally hot temperatures during the Early Triassic greenhouse. Science 338, 366–370. doi:10.1126/science.1224126
Svensen, H., Planke, S., Polozov, A. G., Schmidbauer, N., Corfu, F., Podladchikov, Y. Y., et al. (2009). Siberian gas venting and the end-Permian environmental crisis. Earth Planet Sci. Lett. 277, 490–500. doi:10.1016/j.epsl.2008.11.015
Takahashi, S., Kaiho, K., Oba, M., and Kakegawa, T. (2010). A smooth negative shift of organic carbon isotope ratios at an end-Permian mass extinction horizon in central pelagic Panthalassa. Palaeogeogr. Palaeoclimatol. Palaeoecol. 292, 532–539. doi:10.1016/j.palaeo.2010.04.025
Tohver, E., Cawood, P. A., Riccomini, C., Lana, C., and Trindade, R. I. F. (2013). Shaking a methane fizz: seismicity from the Araguainha impact event and the Permian–Triassic global carbon isotope record. Palaeogeogr. Palaeoclimatol. Palaeoecol. 387, 66–75. doi:10.1016/j.palaeo.2013.07.010
Tribovillard, N., Algeo, T. J., Lyons, T. W., and Riboulleau, A. (2006). Trace metals as paleoredox and paleoproductivity proxies: an update. Chem. Geol. 232, 12–32. doi:10.1016/j.chemgeo.2006.02.012
Twitchett, R. J., Looy, C. V., Morante, R., Visscher, H., and Wignall, P. B. (2001). Rapid and synchronous collapse of marine and terrestrial ecosystems during the end- Permian biotic crisis. Geology 29, 351–354. doi:10.1130/0091-7613(2001)029
Vajda, V., McLoughlin, S., Mays, C., Frank, T. D., Fielding, C. R., Tevyaw, A., et al. (2020). End-Permian (252 Mya) deforestation, wildfires and flooding–An ancient biotic crisis with lessons for the present. Earth Planet Sci. Lett. 529, 115875. doi:10.1016/j.epsl.2019.115875
van Breugel, Y., Schouten, S., Paetzel, M., Ossebaar, J., and Sinninghe Damsté, J. S. (2005). Reconstruction of δ13C of chemocline CO2 (aq) in past oceans and lakes using the δ13C of fossil isorenieratene. Earth Planet Sci. Lett. 235, 421–434. doi:10.1016/j.epsl.2005.04.017
van Groenigen, K., Osenberg, C., and Hungate, B. (2011). Increased soil emissions of potent greenhouse gases under increased atmospheric CO2. Nature 475, 214–216. doi:10.1038/nature10176
Wang, Y., and Jin, Y. (2000). Permian palaeogeographic evolution of the jiangnan basin, south China. Palaeogeogr. Palaeoclimatol. Palaeoecol. 160, 35–44. doi:10.1016/S0031-0182(00)00043-2
Ward, P. D., Botha, J., Buick, R., De Kock, M. O., Erwin, D. H., Garrison, G. H., et al. (2005). Abrupt and gradual extinction among Late Permian land vertebrates in the Karoo Basin, South Africa. Science 307, 709–714. doi:10.1126/science.1107068
Ward, P. D., Montgomery, D. R., and Smith, R. (2000). Altered river morphology in South Africa related to the Permian–Triassic extinction. Science 289, 1740–1743. doi:10.1126/science.289.5485.1740
Wei, H., Yu, H., Wang, J. G., Qiu, Z., Xiang, L., and Shi, G. (2015). Carbon isotopic shift and its cause at the Wuchiapingian–Changhsingian boundary in the Upper Permian at the Zhaojiaba section, South China: evidences from multiple geochemical proxies. J. Asian Earth Sci. 105, 270–285. doi:10.1016/j.jseaes.2015.01.011
Wignall, P. B., and Hallam, A. (1992). Anoxia as a cause of the Permian/Triassic mass extinction: facies evidence from northern Italy and the western United States. Palaeogeogr. Palaeoclimatol. Palaeoecol. 93, 21–46. doi:10.1016/0031-0182(92)90182-5
Wignall, P. B. (2001). Large igneous provinces and mass extinctions. Earth-Sci. Rev. 53, 1–33. doi:10.1016/S0012-8252(00)00037-4
Wignall, P. B., Morante, R., and Newton, R. (1998). The Permo–Triassic transition in Spitsbergen: δ13Corg chemostratigraphy, Fe and S geochemistry, facies, fauna and trace fossils. Geol. Mag. 135, 47–62. doi:10.1017/S0016756897008121
Wignall, P. B., and Twitchett, (1996). Oceanic anoxia and end Permian mass extinction. Science 272, 1155–1158. doi:10.1126/science.272.5265.1155
Xie, S. C., Pancost, R. D., Huang, J. H., Wignall, P. B., Yu, J. X., Tang, X. Y., et al. (2007). Changes in the global carbon cycle occurred as two episodes during the Permian–Triassic crisis. Geology 35, 1083–1086. doi:10.1130/G24224A.1
Xu, D. Y., Ma, S. L., Chai, Z. F., Mao, X. Y., Sun, Y. Y., Zhang, Q. W., et al. (1985). Abundance variation of iridium and trace elements at the Permian/Triassic boundary at Shangsi in China. Nature 314, 154–156.
Yang, B., Li, H. X., Wignall, P. B., Jiang, H. S., Niu, Z. J., Ye, Q., et al. (2019). Latest wuchiapingian to earliest triassic conodont zones and δ13Ccarb isotope excursions from deep-water sections in western Hubei province, south China. J. Earth Sci. 30, 1059–1074. doi:10.1007/s12583-019-1018-2
Yang, Z. Y., Yin, H. F., Wu, S. B., Yang, F. Q., Ding, M. H., and Xu, G. R. (1987). Permian-triassic boundary. Stratigraphy and faunas of South China. Ministry of geology and mineral resources. Geol. Mem. Ser. 26, 1–379 (in Chinese with English abstract).
Yin, H. F., Jiang, H. S., Xia, W. C., Feng, Q. L., Zhang, N., and Shen, J. (2014). The end-Permian regression in South China and its implication on mass extinction. Earth-Sci. Rev. 137, 19–33. doi:10.1016/j.earscirev.2013.06.003
Yuan, D. X., Chen, J., Zhang, Y. C., Zheng, Q. F., and Shen, S. Z. (2015). Changhsingian conodont succession and the end-Permian mass extinction event at the Daijiagou section in Chongqing, Southwest China. J. Asian Earth Sci. 105, 234–251. doi:10.1016/j.jseaes.2015.04.002
Yvon-Durocher, G., Allen, A., Bastviken, D., Conrad, R., Gudasz, C., St-Pierre, A., et al. (2014). Methane fluxes show consistent temperature dependence across microbial to ecosystem scales. Nature 507, 488–491. doi:10.1038/nature13164
Zhang, G. J., Zhang, X. L., Hu, D. P., Li, D. D., Algeo, T. J., Farquhar, J., et al. (2017). Redox chemistry changes in the Panthalassic ocean linked to the end-Permian mass extinction and delayed Early Triassic biotic recovery. Proc. Natl. Acad. Sci. USA 114, 1806–1810. doi:10.1073/pnas.1610931114
Zhao, J. K., Liang, X. L., and Zheng, Z. G. (1978). Late permian cephalopods of South China. Palaeontol. Sinica, N.S. 12, 1–194 (in Chinese with English abstract).
Zhao, J. K., Sheng, J. Z., Yao, Z. Q., Liang, X. L., Chen, C. Z., Rui, L., et al. (1981). The changhsingian and permian-triassic boundary of South China. Bull. Nanjing Inst. Geol. Paleontol. Acad. Sinica 2, 1–112 (in Chinese).
Zhou, W. F., Algeo, T. J., Ruan, X. Y., Luo, G. M., Chen, Z. Q., and Xie, S. C. (2017). Expansion of photic-zone euxinia during the Permian–Triassic biotic crisis and its causes: microbial biomarker records. Palaeogeogr. Palaeoclimatol. Palaeoecol. 474, 140–151. doi:10.1016/j.palaeo.2016.06.027
Zhu, T. X., Huang, Z. Y., and Hui, L. (1999). The geology of late permian period biohermal facies in upper yangtze tableland. Beijing, China: Geol. Pub. House, (in Chinese).
Zuchuat, V., Sleveland, A. R. N., Twitchett, R. J., Svensen, H. H., Turner, H., Augland, L. E., et al. (2020). A new high-resolution stratigraphic and palaeoenvironmental record spanning the End-Permian Mass Extinction and its aftermath in central Spitsbergen. Svalbard. Palaeogeogr. Palaeoclimatol. Palaeoecol. 554, 109732. doi:10.1016/j.palaeo.2020.109732
Keywords: end-Permian extinction, global CH4 cycle, methanogenesis, methanotrophy, anaerobic oxidation of methane (AOM), global warming, variable δ13Corg records
Citation: Saitoh M and Isozaki Y (2021) Carbon Isotope Chemostratigraphy Across the Permian-Triassic Boundary at Chaotian, China: Implications for the Global Methane Cycle in the Aftermath of the Extinction. Front. Earth Sci. 8:596178. doi: 10.3389/feart.2020.596178
Received: 19 August 2020; Accepted: 01 December 2020;
Published: 05 February 2021.
Edited by:
David Mark Hodgson, University of Leeds, United KingdomReviewed by:
Genming Luo, China University of Geosciences Wuhan, ChinaMike Rogerson, University of Hull, United Kingdom
Copyright © 2021 Saitoh and Isozaki. This is an open-access article distributed under the terms of the Creative Commons Attribution License (CC BY). The use, distribution or reproduction in other forums is permitted, provided the original author(s) and the copyright owner(s) are credited and that the original publication in this journal is cited, in accordance with accepted academic practice. No use, distribution or reproduction is permitted which does not comply with these terms.
*Correspondence: Masafumi Saitoh, saitoh.m.ab@gmail.com