Re-estimation of the vertical sensible heat flux by determining the environmental temperature on a single-point tower measurement
- 1Key Laboratory of Science and Technology on Operational Oceanography, South China Sea Institute of Oceanology, Chinese Academy of Sciences, Guangzhou, China
- 2State Key Laboratory of Tropical Oceanography, South China Sea Institute of Oceanology, Chinese Academy of Sciences, Guangzhou, China
- 3State Key Laboratory of Atmospheric Boundary Layer Physics and Atmospheric Chemistry, Institute of Atmospheric Physics, Chinese Academy of Sciences, Beijing, China
- 4Guangzhou Institute of Tropical and Marine Meteorology, China Meteorological Administration, Guangzhou, China
Surface energy balance has always been a goal of those studying the Earth’s climate system. However, many studies have demonstrated that turbulent heat fluxes are usually underestimated by eddy covariance (EC) measurements, such that the energy balance is not closed. This study proposes a new perspective on calculating sensible heat flux based on the environmental temperature using EC. Using this approach, additional sensible heat fluxes were detected as outcomes of the vertical transportation of thermal structures in the atmospheric surface layer (ASL). For data obtained over a 40-day period over a grassland in Southern China, additional sensible heat flux observations exceeding 50 W m−2 were measured for 8 of the 40 days; smaller but still significant contributions were captured for another 11 days. In the proposed model, the difference between the mean and environmental temperature (
1 Introduction
The eddy covariance (EC) method has been widely used for decades to estimate the vertical fluxes of momentum, heat, and gases between the Earth’s surface and the atmospheric surface layer (ASL). The EC method assumes that all signals of small-scale motions are included to calculate the results from the covariance between measurement scalars and vertical velocity fluctuations. However, it is known that the conventional EC method often underestimates the vertical energy flux, which is one of the main reasons for the widespread “energy imbalance problem” in the ASL. Analysis from FLUXNET sites shows that average turbulent energy fluxes underestimate available energy by 20% at most sites (Wilson et al., 2002). Instrumental errors (Richardson et al., 2012; Mauder and Zeeman, 2018), data processing errors (Kaimal and Finnigan, 1994; Leuning et al., 2012), additional sources of energy (Mauder et al., 2013; Garcia-Santos et al., 2019), and sub-mesoscale transport processes (Mauder et al., 2010, 2020) are supposedly the underlying reasons for the surface energy imbalance. In the past 25 years, although a great deal of research has attempted to address the energy imbalance in the ASL, the results have not been satisfactory. Thus, energy imbalance remains an ongoing issue. Based on the above reasons, some have taken a closer look at the theoretical foundations of the EC method.
The turbulent heat flux in the ASL
As such, some have shifted their attention to determining environmental temperature and new aspects of the total heat flux. Shang et al. (2003, 2004) used the most probable temperature as the reference temperature for studying the local convective heat flux in turbulent Rayleigh–Bénard convection; their results showed that non-isotropic coherent structures in the convection cell can carry much more heat than that specified using the conventional EC method.
Mauder et al. (2008) treated the time–space-averaged temperature as the environmental temperature and determined that the contribution of additional sensible heat flux was significant. Specifically, they designed a 3.5 km × 3.5 km ground-based experimental set-up to study the sensible eddy heat flux. The additional flux, with the contribution of large, organized structures captured by the spatial EC method, exceeded 50 Wm−2.
However, neither Shang et al. (2004) nor Mauder et al. (2008) provided a physical basis for their treatments of the environmental temperature. A more accurate determination of the environmental temperature and its impact on the turbulent heat flux in the ASL is necessary, allowing for a re-estimation of the vertical sensible eddy heat flux from single-point tower measurements. A new calculation of sensible heat flux based on determination of the environmental temperature is here proposed. Even for average measuring times of 30 min, our results from single-point tower measurements show that the total vertical sensible eddy heat flux is considerably underestimated. The addition of the sensible heat flux, which is not the same as the spatial averaging method derived from Mauder’s study (Mauder et al., 2008), can also be measured by single-point tower measurements and is associated with anisotropic thermal structures in the ASL that are ignored by the conventional EC method. This new calculation of sensible heat flux may provide an inspection of the energy imbalance problem in the ASL.
In this study, we re-estimated the vertical sensible heat flux using the new calculation of sensible heat flux and single-point tower measurements over a 40-day period in a grassland region of southern China. Additional sensible heat fluxes were detected that were considered outcomes of the vertical transportation of thermal structures in the ASL. In our research, three 30-min runs with different stability conditions were chosen to shed light on the environmental temperature and its diurnal fluctuations. Long-term flux measurement data were included in the re-estimation of the vertical sensible heat flux. In the following, we describe our experimental setup and explain the theoretical basis of our approach.
2 Materials and methods
2.1 Data resource
Detailed descriptions of the study site and long-term flux measurement system can be found in Bi et al. (2007). Only a brief account is provided here.
Preliminary measurements were carried out in May 2004 over a flat, homogeneous, grassland site (area: 300 m × 400 m) in southern China (Bi et al., 2007; Qi et al., 2015). The site was 12.5 m above sea level and was located in the tropical monsoon region (22.43°N, 113.25°E). The air temperature and wind velocity components (
Following Foken et al. (2004), post-field data quality control processes were applied. In particular, noise and various kinds of interference from 30-min measurements of turbulence using a criterion of
The study period was 40 days from 1 June 2004 to 10 July 2004 to re-estimate the sensible heat flux. Three 30-min runs were conducted daily to obtain data under different stability conditions: 3:00–3:30 for stable conditions, 11:00–11:30 for unstable conditions, and 19:00–19:30 for neutral conditions. The mean meteorological conditions for the three runs are shown in Table 1, where the friction velocity
where
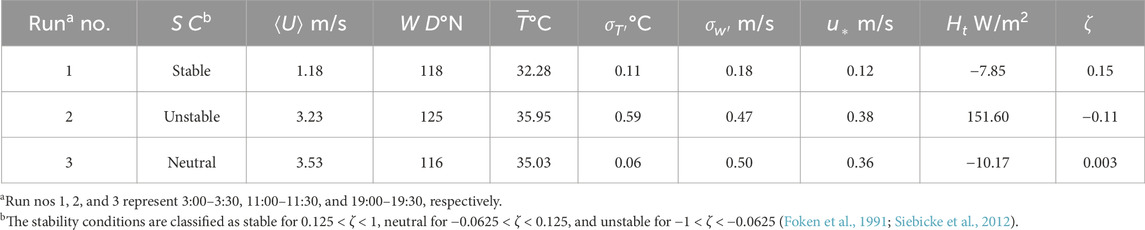
Table 1. Summary of the mean meteorological conditions for the three 30-min runs: friction velocity (
2.2 Theoretical considerations
The heat transferred by eddies cross a horizontal surface in the atmosphere, at any height z, is given by Priestley and Swinbank (1947):
where
where
In the conventional approach, the first term on the right side of Eq. 3 is usually neglected as small values of
First, consider the two terms on the right side of Eq. 3 denoted as
2.2.1 Environmental temperature
An air parcel is heated or cooled as it moves between different layers at different heights. For instance, heat is taken away by the parcels ejected upward, and new parcels with lower temperatures sweep in to supply the removed air. Ramps are commonly observed in temperature traces when high-frequency measurements of temperature are taken for the vertical transportation of these thermal structures (Gao et al., 1989; Chu et al., 1996). A sample of temperature traces under various stability conditions is shown in Figure 1 for 23 June 2004 at 11:00. The ramp-like structures occur under both stable and unstable conditions. In this regard, the surface renewal ramp model was first described by Snyder et al. (1996) and further modified by Chen et al. (1997) as a more realistic ramp model. Figure 2 shows the ramp-like model and information to be extracted, as summarized thus:
• The total ramp duration is characterized by the time over which the air temperature changes, Lr (s), and the time over which the air temperature remains unchanged (i.e., quiescent), Lq (s). The thermal structures in the ramp period Lr are responsible for heat, momentum, water vapor, and other gaseous transportation. During the quiescent period Lq between the falling ramp and the formation of the next ramp, the flux exchange is scarce (Chen et al., 1997).
• The amplitude
• Both the warm plumes found in unstable conditions, and the cool plumes that occur under stable conditions in the low ASL are usually driven by instabilities in the velocity layer (Gao et al., 1989; Belmonte and Libchaber, 1996), thus creating the slopes of the ramp-like structures.
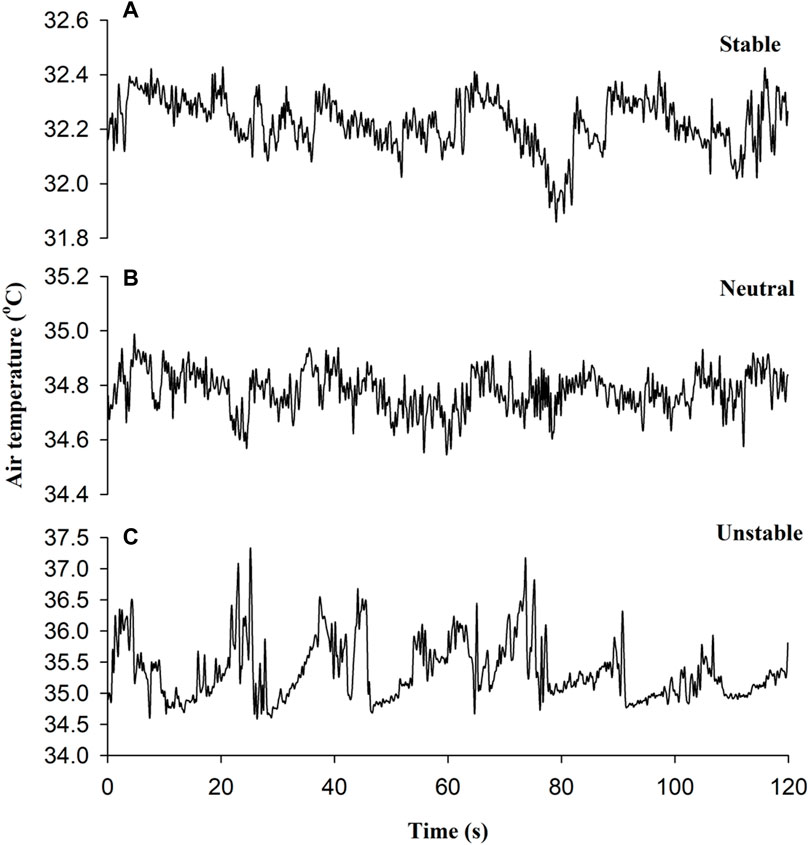
Figure 1. Air temperature (°C) fluctuations observed in a 10-Hz sample for stable (03:00, (A)), neutral (19:00, (B)), and unstable conditions (11:00, (C)) on 23 June 2004.
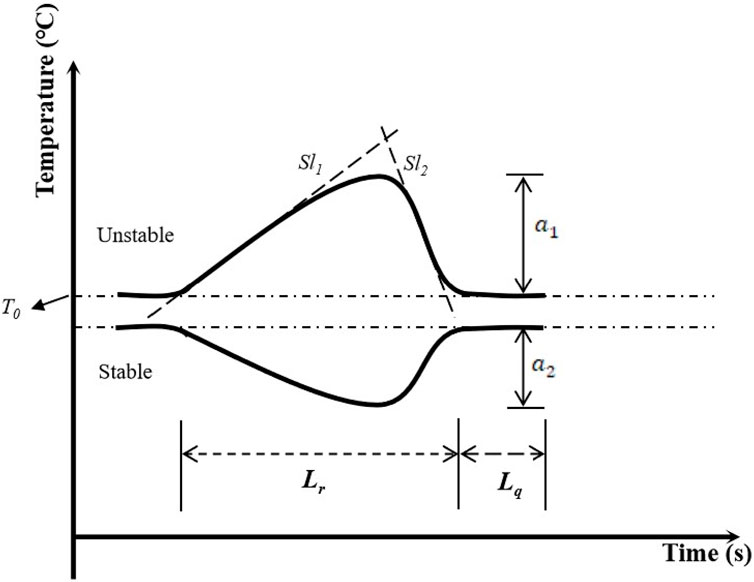
Figure 2. Analysis of the ramp-like model for temperature fluctuations in the atmospheric surface layer (ASL), where
Here, we take the temperature within the quiescent period Lq as the environmental temperature
Webb et al. (1980) noted that
The environmental temperature
1) Calculate the temperature fluctuations
and
Here, the width of window (
2) Evaluate the PDF, denoted as
3) Identify temperature
4) Obtain the environmental temperature
5) Calculate the difference between the mean and environmental temperature using Eq. 7:
3 Results
3.1 for single-point tower measurements
To more accurately illustrate
Figure 3 shows the PDFs for the temperature fluctuations of the three runs under the different stability conditions. To contrast them more fully, all maximums of the PDFs were normalized to the value of 1. Distinct peaks were found in the PDF distributions for all stability conditions. For the three runs, the differences between the mean and environmental temperatures (
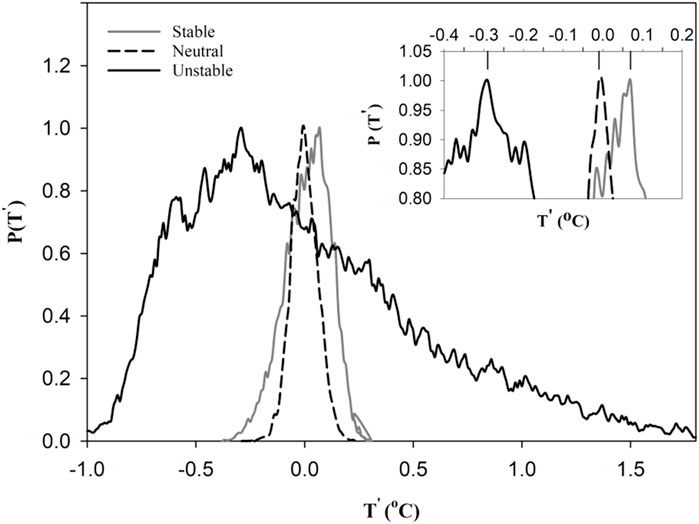
Figure 3. Probability density functions of the temperature fluctuations for the three runs under different stability conditions.

Table 2. Summary of skewness (S) of temperature and vertical velocity, skewness (S’) of the temperature derivative, the environmental temperature (
Figure 4 shows the variations in
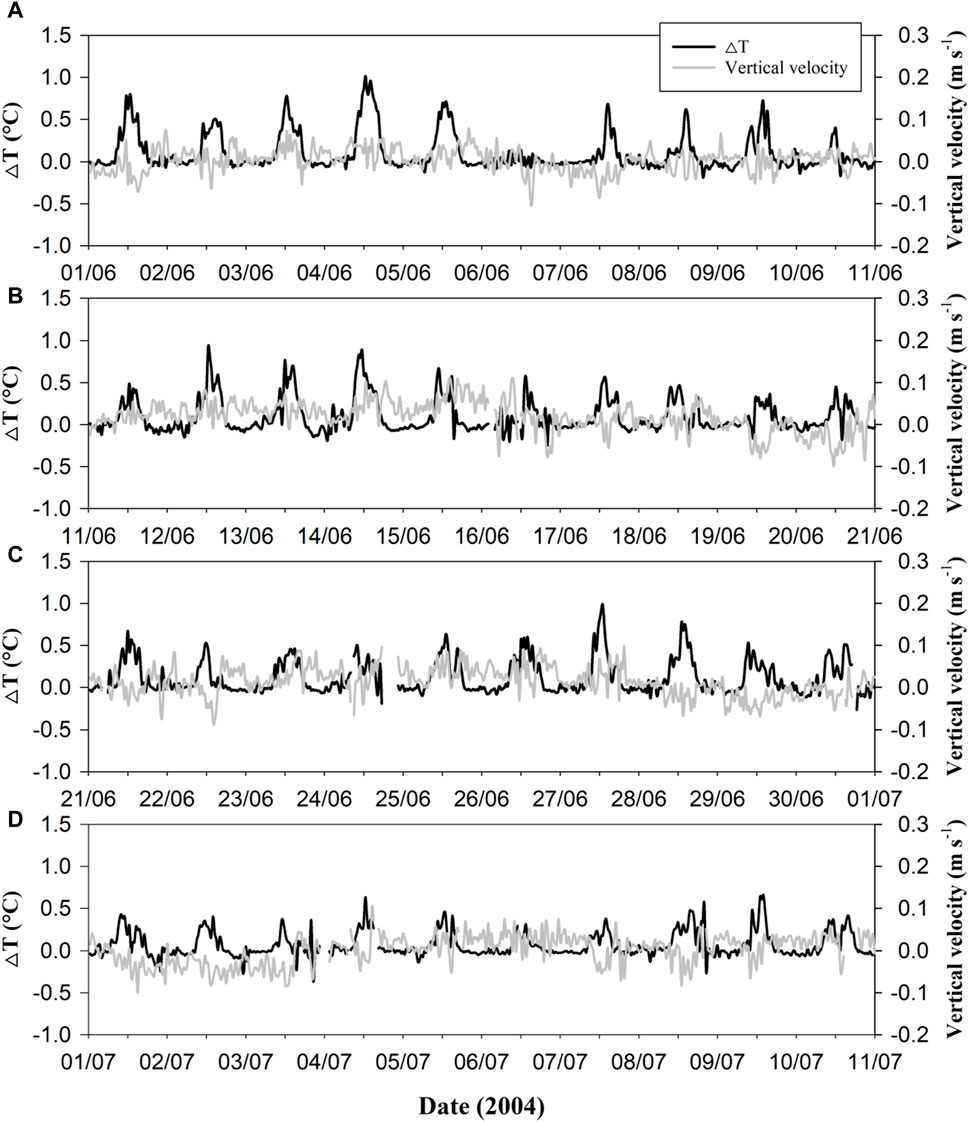
Figure 4. Vertical wind velocities (m s−1) averaged over 30 min and ∆T (°C) for the period (A) 01 June to 10 June 2004, (B) 11 June to 20 June 2004, (C) 21 June to 30 June 2004, and (D) 01 July to 10 July 2004.
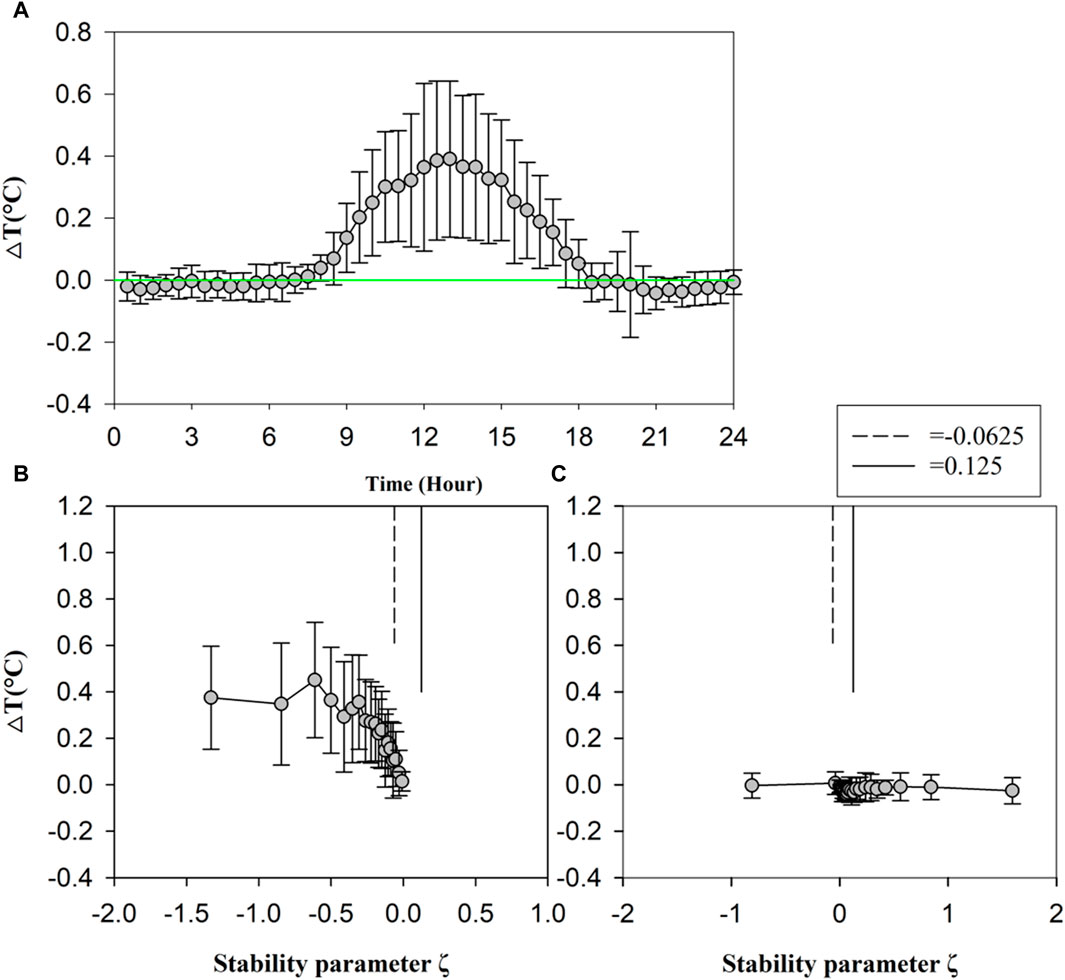
Figure 5. (A) Diurnal variations in the difference between the mean and environmental temperatures (
3.2 Vertical velocity
Wind data from sonic anemometers are usually transformed into a mean streamline parallel coordinate system to correct for non-zero
where:
The wind data for 27 to 30 June were selected to determine the linear regression coefficients (
Figure 4 shows relatively small changes in the vertical velocity (
There was a linear relationship between the daily maximum of
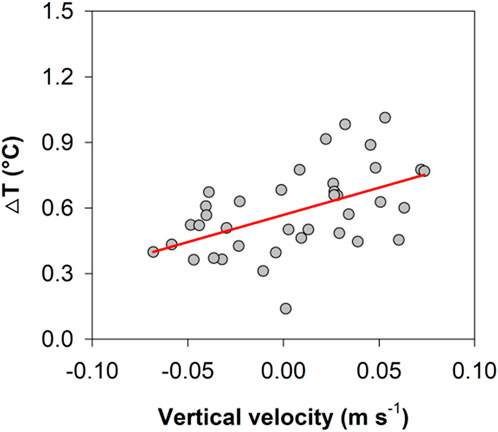
Figure 6. Plot of the maximum of
3.3 Sensible heat flux
The measurement location was in the tropic monsoon region of southern China. Because measurement commenced in June, the air temperature was relatively high and the diurnal variation in the maximum temperature ranged between 26
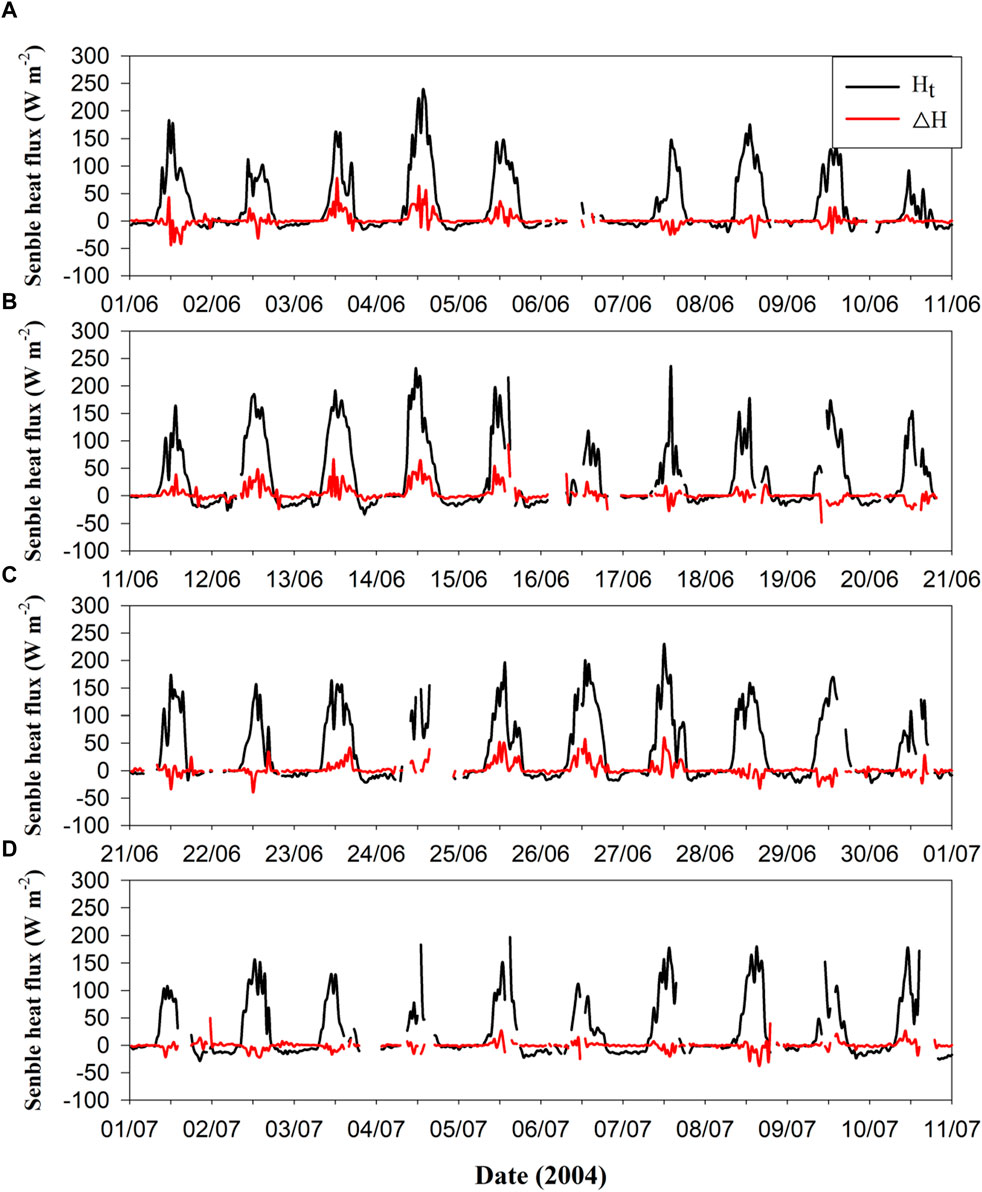
Figure 7. Sensible heat flux estimates for the additional flux ΔH (W m−2) and the conventional estimated flux Ht (W m−2) for the period 01 June to 10 July 2004. The averaging time is 30 min for the period (A) 01 June to 10 June 2004, (B) 11 June to 20 June 2004, (C) 21 June to 30 June 2004, and (D) 01 July to 10 July 2004.
Large differences (>50 Wm−2) between the new method used to estimate the total sensible heat flux
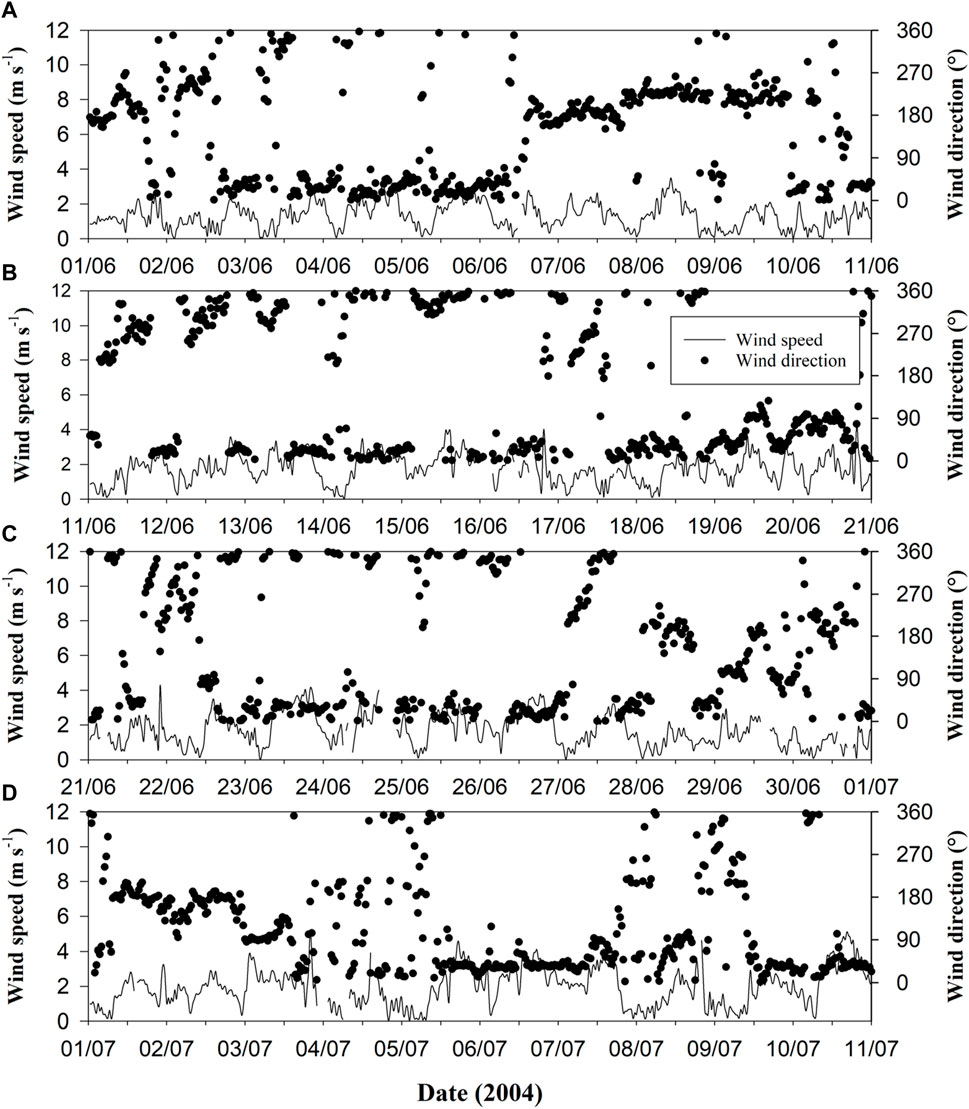
Figure 8. Wind speed (m s−1) and wind direction (°) averaged over 30 min for the period 01 June to 10 July 2004 for the period (A) 01 June to 10 June 2004, (B) 11 June to 20 June 2004, (C) 21 June to 30 June 2004, and (D) 01 July to 10 July 2004.
In addition to the large positive additional flux observed, there were also relatively large negative additional flux values (<−30 Wm−2) for some of the other days: −44.0, −32.0, −30.1, −48.2, −33.4, −39.4, −54.9, and −34.3 Wm−2 for 01, 02, 08, 19, 21, 22, and 28 June, and 08 July, respectively. As with the positive flux, all of the negative flux occurred at noon, with
Mauder and Foken (2006) indicated an uncertainty of 5% or 10 Wm−2 from sonic anemometer measurements, using the conventional EC method to estimate the sensible heat flux. The large discrepancies described in sensible heat flux calculated from the conventional EC method and the new EC method at noon in our observations seem plausible as the thermal structures were active at this time of day. The presence of small local vertical velocities resolves these large discrepancies.
4 Discussion
4.1 Reason for the additional flux
The conventional EC method was developed under the condition of homogeneous and isotropic turbulence flow, and it was thought able to capture the heat flux contributed by all signals of small-scale motions. The visible contradiction between the prerequisite of the conventional EC method and the widespread anisotropic turbulence in the ASL was the first indication that the conventional EC method may fail to estimate the correct heat flux. The identified thermal structures were responsible for the majority of the vertical heat, momentum, and mass fluxes in the ASL (Gao et al., 1989; Chen et al., 1997). Behind these thermal structures are anisotropic flows. The ability of an anisotropic flow to transport heat differs considerably from that of an isotropic flow.
We obtained the local environmental temperature from the ramp-like structures in our model (Figure 2). If warm (cool) structures occupy the space, then the time mean temperature will be higher (lower) than the environmental temperature. The time scales of the thermal structures are much less than 30 min; thus, they can be captured by the 30-min average operator. Additionally, their spatial scales are not very large, such that the results represent the local heat flux. To measure the additional heat flux contributed by large-scale organized structures, Mauder et al. (2008) used the time–space-averaged temperature as the environmental temperature. However, this may be a limitation of their theory in practice.
When using the most probable temperature within a 30-min period as the environmental temperature, an additional flux due to the transportation of thermal structures could be detected. The additional vertical sensible eddy heat flux
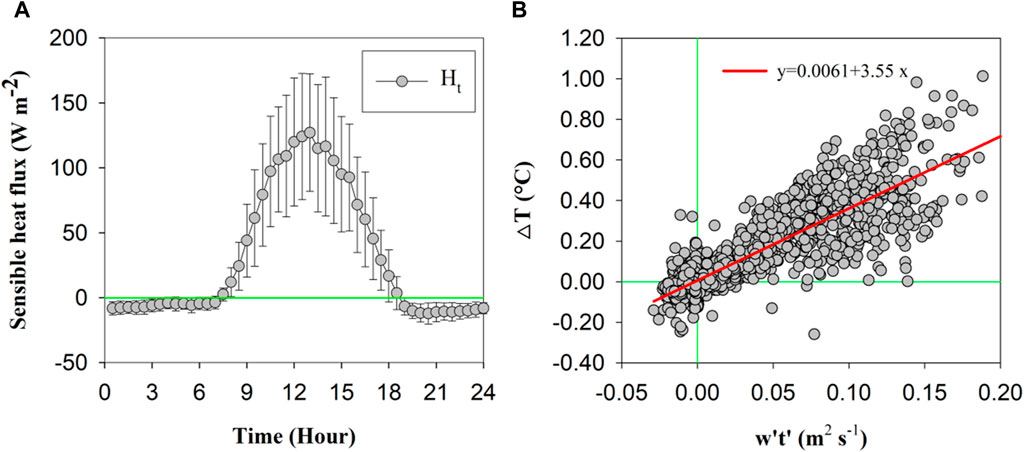
Figure 9. (A) Diurnal variations in the conventional EC method to calculate sensible heat flux (
The linear regression relationship from Figure 9 provides a modeled estimation of
where
Substituting Eq. 9 into Eq. 3 leads to the following relationship:
Based on Eq. 10, it is clear that, once the coefficient is known, the additional flux coinciding with local vertical convection can be resolved. For example, an additional flux of 35.5% of
4.2 The role of vertical convection
The additional vertical sensible eddy heat flux
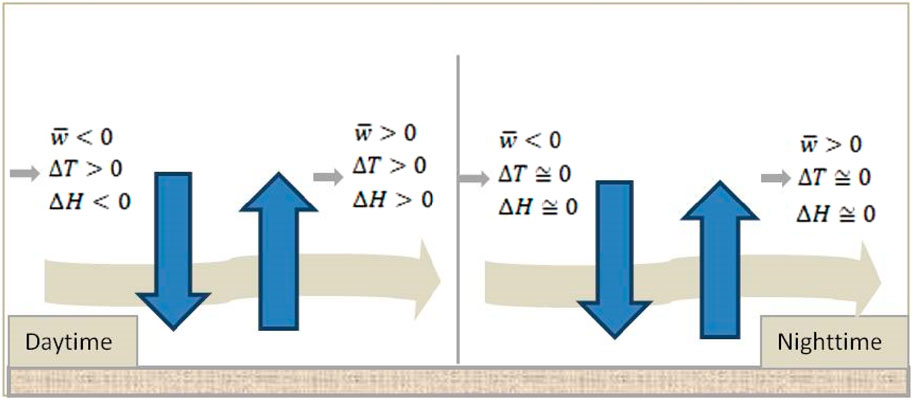
Figure 10. Schematic diagram showing the underestimation or overestimation of total vertical sensible heat flux caused by the thermal structures interacting with local vertical convection in the ASL when using the conventional EC method in Eq. 3.
4.3 The probable role of the method in the energy imbalance problem
It is known that the majority of vertical heat, momentum, and mass fluxes in the ASL are due to the movements of the identified thermal or coherent structures (Qiu et al., 1995; Chen et al., 1997). The creation of these thermal structures is dynamically dominated by the vertical shear of wall-bounded turbulent flows (Gao et al., 1989), and their thermodynamics are dominated by the temperature difference between the upper and bottom boundaries of Earth’s surface or the Rayleigh–Bénard convection (Shang et al., 2004). The structures are usually more pronounced under unstable conditions (Figure 1). Similar ramp patterns of humidity and CO2 can also be found, and they are both shaped by the turbulence structures in the ASL. Therefore, additional heat flux in the form of latent heat and additional CO2 transport due to this turbulence should also be considered.
In this study, both the conventional EC estimate and the additional contribution are fluxes transported by the turbulence in the surface layer; that is, the additional contribution is a component of heat transported by the thermal structures but cannot be identified by the conventional EC method. A more intuitive understanding comes from a laboratory experiment of Shang et al. (2004), who compared the heat transports calculated by the EC method and those injected by heater in a turbulent Rayleigh–Bénard convection system. They concluded that the conventional EC estimates can significantly underestimate the injected heat flux under convective conditions, and the underestimated heat transport was captured by the new method (Eq. (3)). The results revealed by Shang et al. (2004) may be inspiration for addressing the energy imbalance problem in the terrestrial surface. The energy imbalance problem was widespread at FLUXNET sites and was most apparent during the day (Wilson et al., 2002). According to Wilson et al. (2002), the difference between the available energy and turbulent energy fluxes reaches its maximum value at noon. Foken et al. (2010) also reported an imbalance of 20%–30% during the daytime in the LITFASS-2003 experiment. Based on the results of our study, the additional contribution
The new estimate considers heat transport by thermal structures. These are not unique to the underlying surface of grassland types but are rather a common feature shared by all types of underlying surfaces, indicating the universality of this research. The contribution of thermal structures generated by plant canopies such as grasslands, forests, and crops to heat transport and their related boundary layer energy imbalance problem have received widespread attention (Chen et al., 1997; Castellvi et al., 2008; Holwerda et al., 2021; Cely-Toro et al., 2023). As they are all based on the conventional EC results to study the contribution of thermal structures to heat flux, there is also the inevitable issue of the energy imbalance problem.
Using the conventional EC method, the large-eddy simulation study of the energy imbalance problem by Steinfeld et al. (2007) showed that the imbalances are relatively small (∼5%) at a height of 20 m. However, reports from field experiments indicated a larger imbalance (10%–30%) at lower measurement heights closer to the surface (Wilson et al., 2002; Oncley et al., 2007; Foken et al., 2010). No viable reasons were offered to explain the imbalance difference between the different measured heights.
We describe the presence of thermal structures in the ASL that determine the existing
5 Summary
The conventional temporal EC method (
The additional sensible heat flux usually peaked at noon, when solar forcing was strong and the convective boundary was developed. Large underestimations of the sensible heat flux exceeding 50 Wm−2 were found for 8 of the 40 days of the observational period. For the eight days, large differences between the mean and environmental temperatures (>0.39°C) and small vertical velocities (<0.11 ms−1) were evident. Underestimations which, although smaller, were still significant in the order of 30–50 Wm−2 were found for 11 other days, as well as a relatively large negative additional flux (<−30 Wm−2). A simple model of the interaction between the thermal structures and local vertical convection was developed. The underestimation or overestimation of vertical sensible heat flux was decided by the vertical wind direction in the daytime, while the errors in sensible heat flux were small at night.
The additional flux can be determined once the difference between the mean and environmental temperatures (
In general, additional sensible heat flux cannot be ignored during the daytime. This may thus be an important reason for the widespread energy imbalance, which is more apparent at noon. In addition,
Data availability statement
The raw data supporting the conclusion of this article will be made available by the authors, without undue reservation.
Author contributions
YQ: methodology, visualization, and writing–original draft. XS: conceptualization, funding acquisition, supervision, and writing–review and editing. GC: conceptualization and writing–original draft. ZG: validation and writing–review and editing. XB: investigation and writing–review and editing. LY: formal analysis and writing–review and editing. HM: formal analysis and writing–review and editing.
Funding
The author(s) declare that financial support was received for the research, authorship, and/or publication of this article. The study was mainly supported by the National Key R&D Plan of China under contract nos 2021YFC3101301, 2021YFC2803104, and 2022YFC3104403.
Acknowledgments
The authors are grateful to the Guangzhou Institute of Tropical and Marine Meteorology, CMA, Guangzhou, China, for the available data used in this study.
Conflict of interest
The authors declare that the research was conducted in the absence of any commercial or financial relationships that could be construed as a potential conflict of interest.
Publisher’s note
All claims expressed in this article are solely those of the authors and do not necessarily represent those of their affiliated organizations or those of the publisher, the editors, and the reviewers. Any product that may be evaluated in this article, or claim that may be made by its manufacturer, is not guaranteed or endorsed by the publisher.
References
Belmonte, A., and Libchaber, A. (1996). Thermal signature of plumes in turbulent convection: the skewness of the derivative. Phys. Rev. E 53, 4893–4898. doi:10.1103/physreve.53.4893
Bi, X., Gao, Z., Deng, X., Wu, D., Liang, J., Zhang, H., et al. (2007). Seasonal and diurnal variations in moisture, heat, and CO2 fluxes over grassland in the tropical monsoon region of southern China. J. Geophys. Res. 112, D10106. doi:10.1029/2006JD007889
Castellvi, F., Snyder, R. L., and Baldocchi, D. D. (2008). Surface energy-balance closure over rangeland grass using the eddy covariance method and surface renewal analysis. Agric. For. Meteorol. 6-7 (148), 1147–1160. doi:10.1016/j.agrformet.2008.02.012
Cely-Toro, I. M., Mortarini, L., Dias-Junior, C. D., Giostra, U., Buligo, L., Degrazia, G. A., et al. (2023). Coherent structures detection within a dense Alpine forest. Agric. For. Meteorol. 343, 109767. doi:10.2139/ssrn.4357159
Chen, W., Novak, M. D., Black, T. A., and Lee, X. (1997). Coherent eddies and temperature structure functions for three contrasting surfaces. Part I: ramp model with finite microfront time. Boundary-Layer Meteorol. 84, 99–124. doi:10.1023/a:1000338817250
Chu, C. R., Parlange, M. B., Katul, G. G., and Albertson, J. D. (1996). Probability density functions of turbulent velocity and temperature in the atmospheric surface layer. Water Resour. Res. 32, 1681–1688. doi:10.1029/96wr00287
Dellwik, E., Mann, J., and Larsen, K. S. (2010). Flow tilt angles near forest edges-part 1: sonic anemometry. Biogeosciences 7, 1745–1757. doi:10.5194/bg-7-1745-2010
Finnigan, J. J., Clement, R., Malhi, Y., Leuning, R., and Cleugh, H. (2003). A re-evaluation of long-term flux measurement techniques part I: averaging and coordinate rotation. Boundary-Layer Meteorol. 107, 1–48. doi:10.1023/a:1021554900225
Foken, T., Mauder, M., Liebethal, C., Wimmer, F. B. F., Leps, J. P., Raasch, S., et al. (2010). Energy balance closure for the LITFASS-2003 experiment. Appl. Climatol. 101, 149–160. doi:10.1007/s00704-009-0216-8
Foken, T., Skeib, G., and Ricter, S. H. (1991). Dependence of the integral turbulence characteristics on the stability of stratification and their use of Doppler-Sodar measurements. Z. Meteorol. 41, 311–315.
Foken, T. M., Gockede, M., Mauder, L. M., Amiro, B., and Munger, W. (2004). “Post-field data quality control,” in Handbook of micrometeorology: a guide for surface flux measurement and analysis. Editors X. Lee, W. J. Massman, and B. Law (Dordrecht: Kluwer Academic Publishers), 182–208.
Gao, W., Shaw, R. H., and Paw U, K. T. (1989). Observation of organized structure in turbulent flow within and above a forest canopy. Boundary-Layer Meteorol. 47, 349–377. doi:10.1007/bf00122339
Garcia-Santos, V., Cuxart, J., Jimenez, M. A., Martinez-Villagrasa, D., Simo, G., Picos, R., et al. (2019). Study of temperature heterogeneities at sub-kilometric scales and influence on surface-atmosphere energy interactions. IEEE Trans. Geosci. Remote Sens. 57, 640–654. doi:10.1109/tgrs.2018.2859182
Holwerda, F., Guerrero-Medina, O., and Meesters, A. G. C. A. (2021). Evaluating surface renewal models for estimating sensible heat flux above and within a coffee agroforestry system. Agric. For. Meteorol. 308-309, 108598. doi:10.1016/j.agrformet.2021.108598
Kaimal, J. C. C., and Finnigan, J. J. (1994). Atmospheric boundary layer flows: their structure and measurement. New York: Oxford University Press.
Lee, X. (1998). On micrometeorological observations of surface-air exchange over tall vegetation. Agric. For. Meteorol. 91, 39–49. doi:10.1016/s0168-1923(98)00071-9
Leuning, R., van Gorsel, E., Massman, W. J., and Isaac, P. R. (2012). Reflections on the surface energy imbalance problem. Agric. For. Meteorol. 156, 65–74. doi:10.1016/j.agrformet.2011.12.002
Mauder, M., Cuntz, M., Drüe, C., Graf, A., Rebmann, C., Schmid, H. P., et al. (2013). A strategy for quality and uncertainty assessment of long-term eddy-covariance measurements. Agric Meteorol 169, 122–135. doi:10.1016/j.agrformet.2012.09.006
Mauder, M., Desjardins, R. L., Pattey, E., Gao, Z., and van Haarlem, R. (2008). Measurement of the sensible eddy heat flux based on spatial averaging of continuous ground-based observations. Boundary-Layer Meteorol. 128, 151–172. doi:10.1007/s10546-008-9279-9
Mauder, M., Desjardins, R. L., Pattey, E., and Worth, D. (2010). An attempt to close the daytime surface energy balance using spatially-averaged flux measurements. Boundary-Layer Meteorol. 136, 175–191. doi:10.1007/s10546-010-9497-9
Mauder, M., and Foken, T. (2006). Impact of post-field data processing on eddy covariance flux estimates and energy balance closure. Meteorol. Z. 15, 597–609. doi:10.1127/0941-2948/2006/0167
Mauder, M., Foken, T., and Cuxart, J. (2020). Surface-energy-balance closure over land: a review. Boundary-Layer Meteorol. 177, 395–426. doi:10.1007/s10546-020-00529-6
Mauder, M., and Zeeman, M. J. (2018). Field intercomparison of prevailing sonic anemometers. Atmos. Meas. Tech. 11, 249–263. doi:10.5194/amt-11-249-2018
McNaughton, K. G. (2004). Turbulence structure of the unstable atmospheric surface layer and transition to the outer layer. Boundary-Layer Meteorol. 112, 199–221. doi:10.1023/b:boun.0000027906.28627.49
Oncley, S. P., Foken, T., Vogt, R., Kohsiek, W., DeBruin, H. A. R., Bernhofer, C., et al. (2007). The energy balance experiment EBEX-2000. Part I: overview and energy balance. Boundary-Layer Meteorol. 123, 1–28. doi:10.1007/s10546-007-9161-1
Priestley, C. H. B., and Swinbank, W. C. (1947). Vertical transport of heat by turbulence in the atmosphere. Proc. R. Soc. A 189, 543–561.
Qi, Y., Shang, X., Chen, G., Gao, Z., and Bi, X. (2015). Using the cross-correlation function to evaluate the quality of eddy-covariance data. Boundary-Layer Meteorol. 157, 173–189. doi:10.1007/s10546-015-0060-6
Richardson, A. D., Aubinet, M., Barr, A. G., Hollinger, D. Y., Ibrom, A., Lasslop, G., et al. (2012). “Uncertainty quantification,” in Eddy covariance: a practical guide to measurement and data analysis. Editors M. Aubinet, T. Vesala, and D. Papale (Dordrecht: Springer), 173–210.
Shang, X.-D., Qiu, X.-L., Tong, P., and Xia, K.-Q. (2003). Measured local heat transport in turbulent Rayleigh-Bénard convection. Phys. Rev. Lett. 90, 074501. doi:10.1103/physrevlett.90.074501
Shang, X.-D., Qiu, X.-L., Tong, P., and Xia, K.-Q. (2004). Measurements of the local convective heat flux in turbulent Rayleigh-Bénard convection. Phys. Rev. E 70, 026308. doi:10.1103/physreve.70.026308
Siebicke, L., Hunner, M., and Foken, T. (2012). Aspects of CO2 advection measurements. Theor. Appl. Climatol. 109, 109–131. doi:10.1007/s00704-011-0552-3
Snyder, R. L., Spano, D., and Paw U, K. T. (1996). Surface renewal analysis for sensible and latent heat flux density. Bound. Layer. Meteorol. 77, 249–266. doi:10.1007/bf00123527
Steinfeld, G., Letzel, M. O., Raasch, S., Kanda, M., and Inagaki, A. (2007). Spatial representativeness of single tower measurements and the imbalance problem with eddy-covariance fluxes: results of a large-eddy simulation study. Boundary-Layer Meteorol. 123, 77–98. doi:10.1007/s10546-006-9133-x
Webb, E. K. (1982). On the correction of flux measurements for effects of heat and water vapour transfer. Boundary-Layer Meteorol. 23, 251–254. doi:10.1007/bf00123301
Webb, E. K., Pearman, G. I., and Leuning, R. (1980). Correction of the flux measurements for density effects due to heat and water vapour transfer. Quart. J. R. Met. Soc. 106, 85–100.
Wilczak, J. M., Oncley, S. P., and Stage, S. A. (2001). Sonic anemometer tilt correction algorithms. Boundary-Layer Meteorol. 99, 127–150. doi:10.1023/a:1018966204465
Williams, A. G., and Hacker, J. M. (1992). The composite shape and structure of coherent eddies in the convective boundary-layer. Boundary-Layer Meteorol. 61, 213–245. doi:10.1007/bf02042933
Keywords: atmospheric surface layer, environmental temperature, sensible heat flux, single-point tower measurement, thermal structure
Citation: Qi Y, Shang X, Chen G, Gao Z, Bi X, Yu L and Mao H (2024) Re-estimation of the vertical sensible heat flux by determining the environmental temperature on a single-point tower measurement. Front. Earth Sci. 12:1269252. doi: 10.3389/feart.2024.1269252
Received: 29 July 2023; Accepted: 27 February 2024;
Published: 26 March 2024.
Edited by:
Shenming Fu, Institute of Atmospheric Physics, Chinese Academy of Sciences (CAS), ChinaReviewed by:
Chunsong Lu, Nanjing University of Information Science and Technology, ChinaMing Chang, Jinan University, China
Copyright © 2024 Qi, Shang, Chen, Gao, Bi, Yu and Mao. This is an open-access article distributed under the terms of the Creative Commons Attribution License (CC BY). The use, distribution or reproduction in other forums is permitted, provided the original author(s) and the copyright owner(s) are credited and that the original publication in this journal is cited, in accordance with accepted academic practice. No use, distribution or reproduction is permitted which does not comply with these terms.
*Correspondence: Xiaodong Shang, xdshang@scsio.ac.cn