Characterization of Whole Biomasses in Pyridine Based Ionic Liquid at Low Temperature by 31P NMR: An Approach to Quantitatively Measure Hydroxyl Groups in Biomass As Their Original Structures
- 1Key Laboratory of Energy Thermal Conversion and Control of Ministry of Education, Nanjing, China
- 2School of Energy and Environment, Southeast University, Nanjing, China
- 3Laboratory of New Fiber Materials and Modern Textile (The Growing Base for State Key Laboratory), Qingdao, China
- 4College of Textiles, Qingdao University, Qingdao, China
- 5Biosciences Division, Oak Ridge National Laboratory, Oak Ridge, TN, United States
- 6Department of Chemical and Biomolecular Engineering, University of Tennessee, Knoxville, TN, United States
- 7Department of Forestry, Wildlife, and Fisheries, University of Tennessee, Knoxville, TN, United States
In this study, the dissolution of biomass components—cellulose, hemicellulose, and lignin, and two whole biomasses—switchgrass and poplar in a pyridine based ionic liquid at a low temperature—50°C has been examined, which will provide an opportunity to explore the original structures of biomass components. The following phosphitylation, and 31P NMR measurement could provide quantitative results for various hydroxyl groups, including aliphatic, condensed phenolic, guaiacyl phenolic, p-hydroxyl phenyl and carboxylic hydroxyl groups in the biomass components, and whole biomass. By employing various biomass model compounds (glucose, cellotriose, and cellohexose), artificial mixtures of biomass components (cellulose, hemicellulose, and lignin), and computational simulation for the assignments by using density functional theory calculation in Gaussian, reliability and accuracy of this method have been examined as well, which indicated that this method is a reliable and accurate way to quantitatively characterize five different types of hydroxyl groups in biomass and its components.
Introduction
Biomass is a renewable resource for the sustainable production of fuels and chemicals that, to date, have been made primarily from fossil resources. Because of its carbon neutrality, relative abundance and non-food competition (David and Ragauskas, 2010), the use of biofuels and biochemicals could increase economic growth and provide environmental benefits. Lignocellulosic biomass contains three major constituents: cellulose, hemicelluloses, and lignin. Several reviews have summarized the distribution of these three major biopolymers in several hardwoods, softwoods, and agricultural residue species (Ragauskas et al., 2006; Huang et al., 2011).
Although lignocellulosic resources are readily becoming available for bioethanol production, their processing requires an aggressive pretreatment step to overcome their natural recalcitrance toward biological deconstruction to simple sugars. Clearly, the characterization of plant cell wall structure and its three major components has become a crucial research topic (Foston and Ragauskas, 2012). The traditional methods for the characterization of lignin, cellulose, and hemicellulose in the whole biomass always involve the isolation of the individual components by separation procedures followed by spectroscopic techniques (Hallac et al., 2009; Hu et al., 2010; Huang et al., 2011; Ben and Ragauskas, 2012). Furthermore, the traditional methods may change the original chemical structures of biomass components due to the relatively high-temperature treatment, oxidation, and hydrolysis. All of these limitations for the traditional methods are calling for new ways to quantitatively characterize the biomass without separation procedures, such as NMR characterization especially performed in a whole biomass solution (Ben and Ferrell Iii, 2016; Hao et al., 2016; Yoo et al., 2016; Li et al., 2018). A solvent system, which has the ability to dissolve whole biomass and could keep all the original chemical structures intact, appears to be very pragmatic. A DMSO-based system reported in the literature is a promising approach (Lu and Ralph, 2003). Most recently, some researchers used ionic liquid as a tool for dissolution and pretreatment of whole biomass (da Costa Lopes et al., 2013a,b) and employed various analytical methods including FT-IR and NMR to characterize the biomass. Argyropoulos’ group (Kilpeläinen et al., 2007; Xie et al., 2007; King et al., 2008, 2009, 2010; Sadeghifar et al., 2014) has developed various imidazole-based ionic liquid to dissolve and characterize whole biomass and biomass components. Characterization of biomass in ionic liquid solutions have been reported including the use of 1H, 31P NMR, and FT-IR. Kishimoto’s group (Qu et al., 2012, 2013) has investigated imidazole based ionic liquid to dissolve several hardwood, softwood, and bamboo samples, and HSQC NMR was also employed to characterize biomass solutions. Similarly, Muhammad (Muhammad et al., 2011) and Sun’s groups (Wen et al., 2012; Yang et al., 2013) also used the imidazole family ionic liquid to dissolve various biomasses and biomass components, including lignin, cellulose, hemicellulose, and holocellulose. FT-IR, 13C, and HSQC NMR have been used to characterize these ionic liquid solutions. All the imidazole type ionic liquids reported (Kilpeläinen et al., 2007; Xie et al., 2007; King et al., 2008, 2009, 2010; Muhammad et al., 2011; Qu et al., 2012, 2013; Wen et al., 2012; Yang et al., 2013) in the literature need a relatively high temperature (~100°C) to dissolve biomass. However, on the basis of reported TGA results (Yang et al., 2007), some biomass components, such as cellulose and hemicellulose, could be slightly decomposed even at ~80°C (Figure S3 in Supplementary Material). Ragauskas’ group (Jiang et al., 2009, 2010; Samuel et al., 2011; Foston et al., 2012) has developed various pyridine ionic liquids and employed various NMR analytical methods, including 1H, 13C, and HSQC NMR, to characterize the chemical structures of biomass. Based on the screening of various pyridine-based ionic liquids (with different side chains), 1-allyl-3-butylpyridinium chloride is the most potential one, which could dissolve the whole biomass and biomass components at a relatively low temperature (50°C). To preserve as much as original chemical structures of biomass, this study will report a dissolution of biomass and its components at 50°C. Normally, 1H and HSQC NMR characterizations of ionic liquid solutions require deuterated ionic liquids, which are relatively expensive. Nevertheless, the phosphitylation of hydroxyl groups using 2-chloro-4,4,5,5-tetramethyl-1,3,2-dioxaphospholane (TMDP) has been developed to quantitatively determine hydroxyl functional groups in various substrates including coal pyrolysis condensates (Wroblewski et al., 1988), coal extracts (Wroblewski et al., 1991), lignin, and pyrolysis oils (Ben and Ragauskas, 2011; Kosa et al., 2011). These hydroxyl functional groups which include aliphatic, condensed phenolic, guaiacyl phenolic, p-hydroxyl phenyl, and carboxylic OH groups are very important to understand the chemical structures of biomass and will also affect the following conversion process. In this study, 31P NMR will be employed to analyze biomass solutions, which could provide quantitative structure information for various hydroxyl groups and will not require deuterated ionic liquids.
Experimental
Synthesis of 1-Allyl-3-Butylpyridinium Chloride
Allyl chloride (4.23 g, 55 mmol), 3-butylpyridine (6.75 g, 50 mmol), and anhydrous toluene (4.0 g) was added to a 25-ml vial. The mixture was heated at 110°C for 24 h under nitrogen. After decanting the toluene, the mixture was placed under direct vacuum at 80°C for 48 h. Figure 1 shows the synthesis pathway and 13C NMR spectrum (see Figure S1 in Supplementary Material for the detailed assignments. Based on 31P NMR analysis, the water content for the ionic liquid is <0.1 wt%).
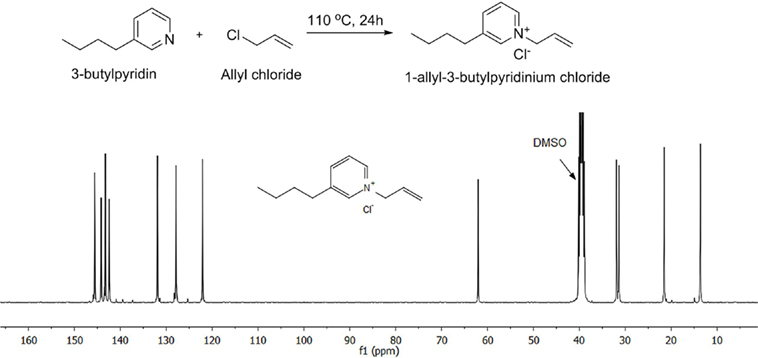
Figure 1. Synthesis pathway and 13 C NMR result for the 1-allyl-3-butylpyridinium chloride ionic liquid.
Biomass Components and Biomasses Preparation
Holocellulose was isolated from extractives-free Poplar by treatment with NaClO2 and acetic acid at 70°C for 2 h (see Supplementary Material for detail). The process was repeated twice to ensure maximum lignin removal.
Ball-milled lignin was isolated by ball-mill extractive-free Poplar in a porcelain jar with ceramic balls using a rotatory ball-mill running at 96 rpm for 14 days under N2. The ball-milled cell wall powder was then extracted with p-dioxane/water (96:4, v/v) and concentrated by evaporation and freeze–dried (Huang et al., 2011).
Two different types of hemicellulose are used in this study, one was commercially purchased (Sigma Aldrich) hemicellulose (xylan) produced from oak wood (hemicellulose #1) and the other was isolated by KOH extraction from Poplar holocellulose (hemicellulose #2). The differences from the natural and isolation process can lead to some different chemical structures for hemicellulose #1 and #2. Glucose, cellotriose, cellohexose, and cellulose were commercially purchased.
Baseline populous (Populous trichocarpa × deltodies) and lowland cultivar Alamo switchgrass (Panicum virgatum) samples were first Wiley-milled to pass 0.13-cm screen and then ball-milled with a Retsch MM 200 Mixer Mill equipped with a 10-ml stainless steel jar and two 7-mm stainless steel grinding balls, and milled for 20 min × 6 times at 25 s−1 frequency. The detailed information about the component analyses of feedstock has been reported in the literature (Foston and Ragauskas, 2010).
Dissolution and 31P NMR Analysis of Biomass and Biomass Components in Ionic Liquid
Biomass and biomass components (5 mg, which is the maximum loading amount for the whole biomasses. For biomass components, the maximum dissolved amount could be even higher than 20 mg, for lignin it can be ~100 mg, however, to be consistent with the whole biomass samples, all the reported solution in this study have the similar concentrations) were dissolved in 1-allyl-3-butylpyridinium chloride ionic liquid (300 mg) at 50°C under nitrogen for 48 h. With 100–200 µl anhydrous pyridine added into the biomass solution, 80 µl 2-chloro-4, 4, 5, 5-tetramethyl-1,3,2-dioxaphospholane was carefully added to the solution. For whole biomass and cellulose samples, anhydrous 1-methyl-2-pyrrolidinone (NMP) and anhydrous N,N-dimethylformamide (DMF, 200 µl, each) were added to improve the dissolution of phosphitylation products. After fully dissolution and phosphitylation, an internal standard (50 µl, 20 mg/ml, cyclohexanol/CDCl3) and relaxation reagent [50 µl, 20 mg/ml Cr(acac)3/CDCl3] were added into the final solution. (Note: The normally used internal standard—endo-N-hydroxy-5-norbornene-2,3-dicarboximide will be decomposed in this type of ionic liquid.)
31P NMR was recorded at room temperature with a 5-mm BBO probe in the Bruker Avance/DMX 400 MHz NMR spectrometer and used an inverse gated decoupling pulse sequence, 90° pulse angle, 25 s pulse delay [optimized based on literature report (Zawadzki and Ragauskas, 2001; Ben and Ragauskas, 2011; Pu et al., 2011)], and 512 scans with a LB of 4.0 Hz. Spectral width is 200 ppm, and the acquisition time is 0.98 s. The cyclohexanol peak signal at 145.1 ppm was used as reference.
Results and Discussion
It has been reported (Pinkert et al., 2009) that the mechanism for dissolution of biomass and its components in ionic liquid involves the disrupture of hydrogen bonds between biopolymers and replacing these hydrogen bonds between ionic liquid and biopolymer. By employing the new developed pyridine-based ionic liquids, the biomass and its components can be dissolved at a low temperature (50°C) very well, which indicate the original hydrogen bond in the biomass has been destroyed and formed a new equilibrium with the ionic liquid. However, after phosphitylation (see Figure S2 in Supplementary Material for reactions) of biomass solutions, some precipitate particles may appear, which may be due to the TMDP reacted with all the OH groups in the biomass and broke the equilibrium between ionic liquid and biomass. It is also evident that TMDP can react with all the OH groups in the biomass ionic liquid solution, which will also change the polarity for the biomass and lead to precipitate. It has been reported (Rinaldi, 2011) that NMP could improve the dissolution of cellulose, and DMF (Pu et al., 2011) was used to dissolve lignin samples. Therefore, to regain the balance and equilibrium, two extra solvents such as NMP and DMF were added into the solution to improve the dissolution. The result indicated that NMP will work perfectly for cellulose sample but not for whole biomass samples. DMF is a necessary step for the total dissolution of whole biomass, but DMF will not work as a single enhancer even for cellulose sample. The final solution is transparent with an amber color. Figure 2 shows the possible dissolution and phosphitylation pathways of biomass in ionic liquid. It has been found the commonly used internal standard NHND (endo-N-hydroxy-5-norbornene-2,3-dicarboximide) is not stable in this type of ionic liquid, with the peak for this internal standard disappeared after 24 h. The similar phenomena have been reported in the literature as well (Ben and Ferrell Iii, 2016); however, the peak for NHND still remained 80% intensity after 14 days, which means this type of ionic liquid can accelerate the decomposition process for NHND. Therefore, for a precise and accurate data, in this study, cyclohexanol has been used as internal standard. By employing the same ionic liquid system, the obtained 31P NMR spectrums for cellulose, hemicellulose, lignin, and poplar whole biomass are shown in Figure 3. Aliphatic OH, condensed phenolic OH (combined C5 substituted/condensed phenolic hydroxyl groups including β-5, syringyl, 4-O-5, and 5-5 structures. Table S1 for detailed assignment ranges and structures), guaiacyl phenolic OH, p-hydroxyl phenol, and carboxylic acid OH can be quantitatively characterized by this method.
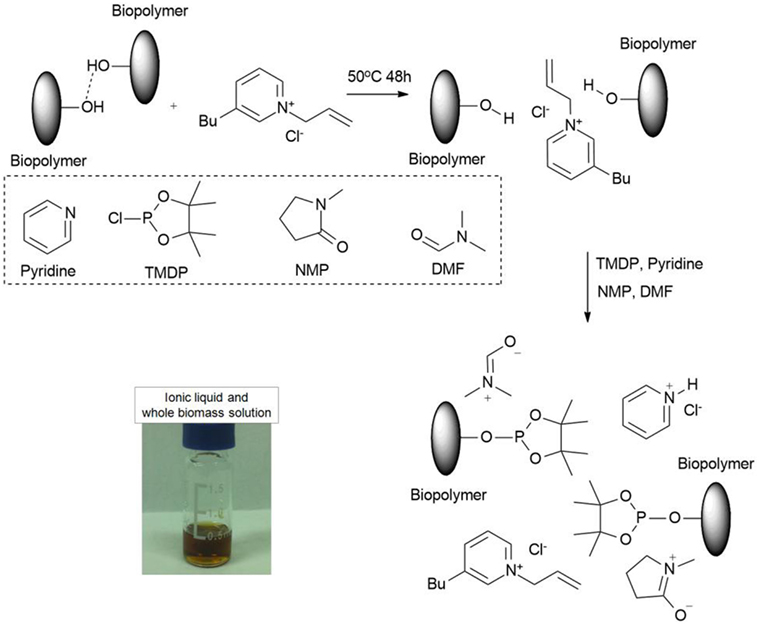
Figure 2. Possible dissolution and phosphitylation of biomass components and whole biomass in the ionic liquid.
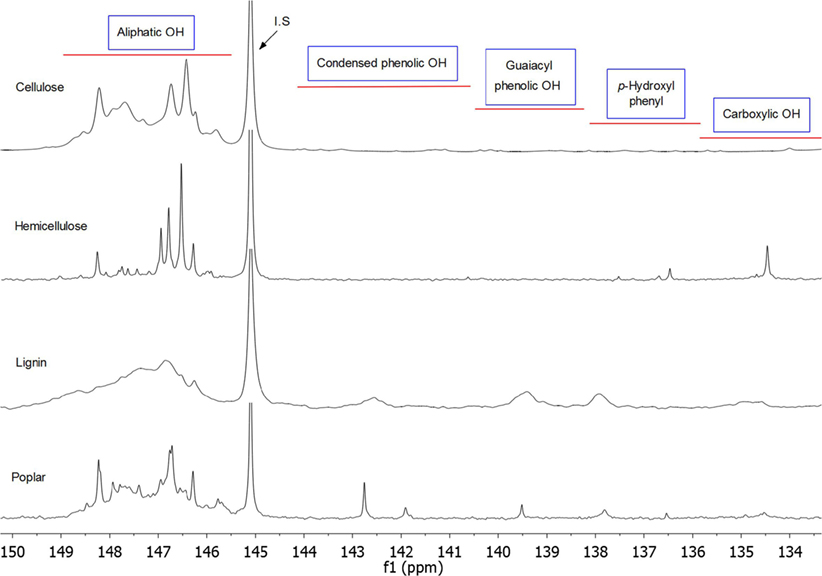
Figure 3. 31P NMR spectra for cellulose, hemicellulose, lignin, and poplar whole biomass after dissolution and phosphitylation with TMDP in ionic liquid.
To further evaluate the accuracy of this method, various oligomers of glucose and cellulose have been examined. Figure S2 in Supplementary Material demonstrates the dissolution and phosphitylation of aliphatic hydroxyl bonds with TMDP in ionic liquid, and Figure 4 shows the correlation between experimental and theoretical data of hydroxyl groups in cellulose and oligomers of glucose, which is the evidence that this method is a reliable way to provide quantitative data for the contents of hydroxyl group for cellulose.
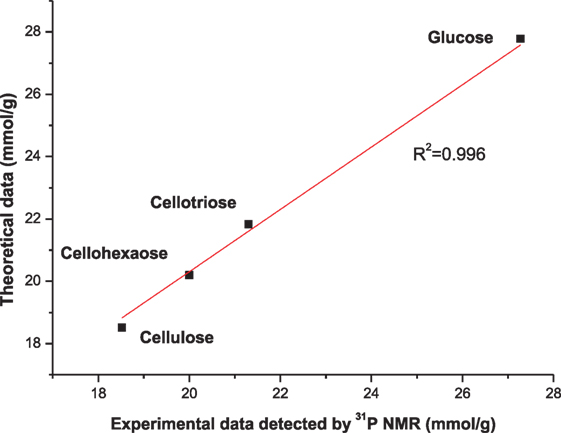
Figure 4. The correlation between experimental and theoretical data of hydroxyl group contents of cellulose and cellulose model compounds.
To explore more about this proposed ionic liquid system, two hemicellulose samples, lignin and holocellulose, were studied and the results are shown in Table 1. It was found that hemicellulose and lignin readily dissolved in this type of ionic liquid and will not precipitate after adding TMDP. Two cosolvents such as DMP and DMF were added for experimental consistency. As anticipated, the results show that there are no phenolic hydroxyl signal in cellulose and hemicellulose. The holocellulose sample contained a very small peak which can be assigned to guaiacyl and p-hydroxyl phenyl OH, which may be due to the residues of lignin. For lignin sample, the results of hydroxyl group contents between employing ionic liquid and routine 31P NMR solvent are virtually identical. Therefore, this method can also provide detailed information of hydroxyl groups for all the three major biomass components—cellulose, hemicellulose, and lignin. To further evaluate this methodology, a set of biomass mixtures were prepared and examined as summarized in Table 2. The artificial mixtures of lignin, hemicellulose, and cellulose have the similar contents for each biomass components in the whole biomass tested in this study to represent the real biomass. The only difference for these two mixtures is the difference for the types of hemicellulose. Since the OH groups contents for all of these three biomass components have been fully characterized (Table 1), the artificial mixtures have the theoretical results for these different OH groups. By using the proposed ionic liquid system, the experimental and theoretical results for these two artificial mixtures are consistent with each other, which indicated that the proposed system can provide quantitative data for complex mixtures, which is the preapproval for this system to perform precise analysis for the real whole biomass.
Having successfully characterized major biomass components and artificial mixtures of these components, this method has also been used to analyze two whole biomasses—switchgrass and poplar. The 31P NMR results are shown in Figure 5. The integration results indicated that compared to poplar, switchgrass has relatively less amount of aliphatic OH, but more aromatic and carboxylic OH. The carbohydrate and Klason lignin contents of these two biomasses (poplar: ~lignin 30%, ~cellulose 50%; switchgrass: ~lignin 28, ~cellulose 40%) also provide similar results (Foston and Ragauskas, 2010). Compare to the literature reported (Akim Leonid et al., 2001) lignin OH groups in the poplar, this study presents a relatively lower content. The reason for this difference is still unclear, which may be due to the benefit of low temperature treatment of biomass in this study or can be due to the differences between the biomass samples and characterization methods.
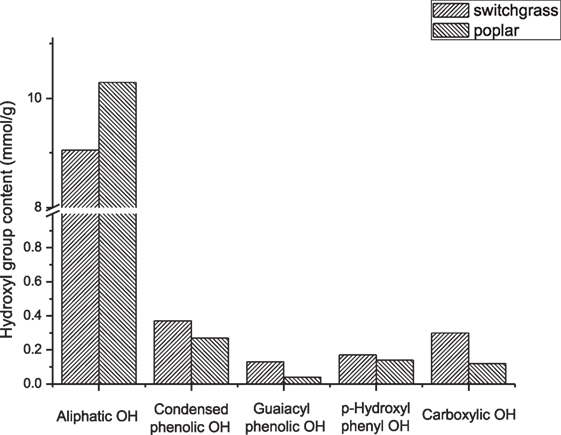
Figure 5. Hydroxyl group contents of switchgrass and poplar. Detected by 31P NMR after dissolution and phosphitylation with TMDP in ionic liquid. (Due to the possible overlap and shift, the content of syringyl type OH is only a preliminary number. There were ~65% of C5 substituted/condensed phenolic OH in poplar belong to syringyl OH, and there were ~40% of C5 substituted/condensed phenolic OH in switchgrass belong to syringyl OH.).
Conclusion
The dissolution of biomass components—cellulose, hemicellulose, and lignin, and two whole biomasses—switchgrass and poplar in 1-allyl-3-butylpyridinium chloride—was examined. The phosphitylation and 31P NMR measurement provided quantitative results for various hydroxyl groups, including aliphatic OH, condensed phenolic OH, guaiacyl phenolic OH, p-hydroxyl phenyl OH, and carboxylic OH in the biomass components and whole biomass. Several evaluations of this process including the use of cellulose and various oligomers of glucose, and artificial mixtures of major biomass components have been examined. All the results show that this method is a reliable and accuracy way to quantitatively characterize hydroxyl groups in biomass and its components. The 31P NMR results for the whole biomasses are also consistent with the traditional analysis method. Nevertheless, the established method in this work opens up a new way to dissolve whole biomass and biomass components at a low temperature (50°C), which provide an opportunity to explore the original structures of biomass (Figure S3 in Supplementary Material). The ongoing research in our group involves detailed assignments for cellulose (Figures S4 and S5 in Supplementary Material) and hemicellulose and evaluation of various pretreatment processes by this method.
Supporting Information
Experimental details, 31P NMR assignments and spectrums, computational simulations are available free of charge via the Internet at http://pubs.acs.org.
Author Contributions
HB conducted all the major experiments and wrote the manuscript. GH provided the NMR facility in Qingdao University to finish some additional tests from China. YS did the sample preparation and helped with the manuscript preparation. WJ did some NMR tests and helped with the manuscript preparation. YP did the sample preparation, NMR tests in the US, and helped with the manuscript preparation. AR provided very valuable information on the whole idea and manuscript writing.
Conflict of Interest Statement
The authors declare that the research was conducted in the absence of any commercial or financial relationships that could be construed as a potential conflict of interest.
Funding
The authors would like to acknowledge the financial support from the National Science Foundation of China (51706044), the Natural Science Foundation of the Jiangsu of China (BK20170666), and the Recruitment Program for Young Professionals in China.
Supplementary Material
The Supplementary Material for this article can be found online at https://www.frontiersin.org/articles/10.3389/fenrg.2018.00013/full#supplementary-material.
References
Akim Leonid, G., Argyropoulos Dimitris, S., Jouanin, L., Leplé, J.-C., Pilate, G., Pollet, B., et al. (2001). Quantitative 31P NMR Spectroscopy of Lignins from Transgenic Poplars. Bordeaux: Holzforschung, 386.
Ben, H., and Ferrell Iii, J. R. (2016). In-depth investigation on quantitative characterization of pyrolysis oil by 31P NMR. RSC Adv. 6, 17567. doi: 10.1039/C5RA23939G
Ben, H., and Ragauskas, A. J. (2011). NMR characterization of pyrolysis oils from Kraft lignin. Energy Fuels 25, 2322. doi:10.1021/ef2001162
Ben, H., and Ragauskas, A. J. (2012). Torrefaction of Loblolly pine. Green Chem. 14, 72. doi:10.1039/C1GC15570A
da Costa Lopes, A. M., João, K. G., Morais, A. R. C., Bogel-Łukasik, E., and Bogel-Łukasik, R. (2013a). Ionic liquids as a tool for lignocellulosic biomass fractionation. Sustain. Chem. Process. 1, 1. doi:10.1186/2043-7129-1-3
da Costa Lopes, A. M., Joao, K. G., Rubik, D. F., Bogel-Lukasik, E., Duarte, L. C., Andreaus, J., et al. (2013b). Pre-treatment of lignocellulosic biomass using ionic liquids: wheat straw fractionation. Bioresour. Technol. 142, 198. doi:10.1016/j.biortech.2013.05.032
David, K., and Ragauskas, A. J. (2010). Switchgrass as an energy crop for biofuel production: a review of its ligno-cellulosic chemical properties. Energy Environ. Sci. 3, 1182. doi:10.1039/b926617h
Foston, M., and Ragauskas, A. J. (2010). Changes in lignocellulosic supramolecular and ultrastructure during dilute acid pretreatment of Populus and switchgrass. Biomass Bioenergy 34, 1885. doi:10.1016/j.biombioe.2010.07.023
Foston, M., and Ragauskas, A. J. (2012). Biomass characterization: recent progress in understanding biomass recalcitrance. Ind. Biotechnol. 8, 191. doi:10.1089/ind.2012.0015
Foston, M. B., McGaughey, J., O’Neill, H., Evans, B. R., and Ragauskas, A. (2012). Deuterium incorporation in biomass cell wall components by NMR analysis. Analyst 137, 1090. doi:10.1039/c2an16025k
Hallac, B. B., Sannigrahi, P., Pu, Y., Ray, M., Murphy, R. J., and Ragauskas, A. J. (2009). Biomass characterization of Buddleja davidii a potential feedstock for biofuel production. J. Agric. Food Chem. 57, 1275. doi:10.1021/jf8030277
Hao, N., Ben, H., Yoo, C. G., Adhikari, S., and Ragauskas, A. J. (2016). Review of NMR characterization of pyrolysis oils. Energy Fuels 30, 6863. doi:10.1021/acs.energyfuels.6b01002
Hu, Z., Sykes, R., Davis, M. F., Charles Brummer, E., and Ragauskas, A. J. (2010). Chemical profiles of switchgrass. Bioresour. Technol. 101, 3253. doi:10.1016/j.biortech.2009.12.033
Huang, F., Singh, P. M., and Ragauskas, A. J. (2011). Characterization of Milled Wood Lignin (MWL) in Loblolly Pine Stem Wood, Residue, and Bark. J. Agric. Food Chem. 59, 12910. doi:10.1021/jf202701b
Jiang, N., Pu, Y., and Ragauskas, A. J. (2010). Rapid determination of lignin content via direct dissolution and 1H NMR analysis of plant cell walls. ChemSusChem 3, 1285. doi:10.1002/cssc.201000120
Jiang, N., Pu, Y., Samuel, R., and Ragauskas, A. J. (2009). Perdeuterated pyridinium molten salt (ionic liquid) for direct dissolution and NMR analysis of plant cell walls. Green Chem. 11, 1762. doi:10.1039/b913609f
Kilpeläinen, I., Xie, H., King, A., Granstrom, M., Heikkinen, S., and Argyropoulos, D. S. (2007). Dissolution of wood in ionic liquids. J. Agric. Food Chem. 55, 9142. doi:10.1021/jf071692e
King, A. W., Zoia, L., Filpponen, I., Olszewska, A., Xie, H., Kilpelainen, I., et al. (2009). In situ determination of lignin phenolics and wood solubility in imidazolium chlorides using (31)P NMR. J. Agric. Food Chem. 57, 8236. doi:10.1021/jf901095w
King, A. W. T., Jalomaki, J., Granstrom, M., Argyropoulos, D. S., Heikkinen, S., and Kilpelainen, I. (2010). A new method for rapid degree of substitution and purity determination of chloroform-soluble cellulose esters, using 31P NMR. Anal. Methods 2, 1499. doi:10.1039/c0ay00336k
King, A. W. T., Kilpeläinen, I., Heikkinen, S., Ja¨rvi, P., and Argyropoulos, D. S. (2008). Hydrophobic interactions determining functionalized lignocellulose solubility in dialkylimidazolium chlorides, as probed by 31P NMR. Biomacromolecules 10, 458. doi:10.1021/bm8010159
Kosa, M., Ben, H., Theliander, H., and Ragauskas, A. J. (2011). Pyrolysis oils from CO2 precipitated Kraft lignin. Green Chem. 13, 3196. doi:10.1039/c1gc15818j
Li, M., Yoo, C. G., Pu, Y., and Ragauskas, A. J. (2018). 31P NMR chemical shifts of solvents and products impurities in biomass pretreatments. ACS Sustain. Chem. Eng. 6, 1265. doi:10.1021/acssuschemeng.7b03602
Lu, F., and Ralph, J. (2003). Non-degradative dissolution and acetylation of ball-milled plant cell walls: high-resolution solution-state NMR. Plant J. 35, 535. doi:10.1046/j.1365-313X.2003.01817.x
Muhammad, N., Man, Z., Bustam, M., Mutalib, M. I. A., Wilfred, C., and Rafiq, S. (2011). Dissolution and delignification of bamboo biomass using amino acid-based ionic liquid. Appl. Biochem. Biotechnol. 165, 998. doi:10.1007/s12010-011-9315-y
Pinkert, A., Marsh, K. N., Pang, S., and Staiger, M. P. (2009). Ionic liquids and their interaction with cellulose. Chem. Rev. 109, 6712. doi:10.1021/cr9001947
Pu, Y., Cao, S., and Ragauskas, A. J. (2011). Application of quantitative 31P NMR in biomass lignin and biofuel precursors characterization. Energy Environ. Sci. 4, 3154. doi:10.1039/c1ee01201k
Qu, C., Kishimoto, T., Hamada, M., and Nakajima, N. (2013). Dissolution and acetylation of ball-milled lignocellulosic biomass in ionic liquids at room temperature: application to nuclear magnetic resonance analysis of cell-wall components. Holzforschung 67, 25. doi:10.1515/hf-2012-0037
Qu, C., Kishimoto, T., Ogita, S., Hamada, M., and Nakajima, N. (2012). Dissolution and acetylation of ball-milled birch (Betula platyphylla) and bamboo (Phyllostachys nigra) in the ionic liquid [Bmim]Cl for HSQC NMR analysis. Holzforschung 66, 607. doi:10.1515/hf.2011.186
Ragauskas, A. J., Nagy, M., Kim, D. H., Eckert, C. A., Hallett, J. P., and Liotta, C. L. (2006). From wood to fuels: integrating biofuels and pulp production. Ind. Biotechnol. 2, 55. doi:10.1089/ind.2006.2.55
Rinaldi, R. (2011). Instantaneous dissolution of cellulose in organic electrolyte solutions. Chem. Commun. 47, 511. doi:10.1039/C0CC02421J
Sadeghifar, H., Dickerson, J. P., and Argyropoulos, D. S. (2014). Quantitative 31P NMR analysis of solid wood offers an insight into the acetylation of its components. Carbohydr. Polym. 113, 552. doi:10.1016/j.carbpol.2014.07.046
Samuel, R., Foston, M., Jaing, N., Cao, S., Allison, L., Studer, M., et al. (2011). HSQC (heteronuclear single quantum coherence) 13C-1H correlation spectra of whole biomass in perdeuterated pyridinium chloride–DMSO system: an effective tool for evaluating pretreatment. Fuel 90, 2836. doi:10.1016/j.fuel.2011.04.021
Wen, J.-L., Sun, S.-L., Xue, B.-L., and Sun, R.-C. (2012). Quantitative structures and thermal properties of birch lignins after ionic liquid pretreatment. J. Agric. Food Chem. 61, 635. doi:10.1021/jf3051939
Wroblewski, A. E., Lensink, C., Markuszewski, R., and Verkade, J. G. (1988). Phosphorus-31 NMR spectroscopic analysis of coal pyrolysis condensates and extracts for heteroatom functionalities possessing labile hydrogen. Energy Fuels 2, 765. doi:10.1021/ef00012a008
Wroblewski, A. E., Reinartz, K., and Verkade, J. G. (1991). Moisture determination of Argonne Premium coal extracts by phosphorus-31NMR spectroscopy. Energy Fuels 5, 786. doi:10.1021/ef00030a003
Xie, H., King, A., Kilpelainen, I., Granstrom, M., and Argyropoulos, D. S. (2007). Thorough chemical modification of wood-based lignocellulosic materials in ionic liquids. Biomacromolecules 8, 3740. doi:10.1021/bm700679s
Yang, D., Zhong, L.-X., Yuan, T.-Q., Peng, X.-W., and Sun, R.-C. (2013). Studies on the structural characterization of lignin, hemicelluloses and cellulose fractionated by ionic liquid followed by alkaline extraction from bamboo. Ind. Crops Prod. 43, 141. doi:10.1016/j.indcrop.2012.07.024
Yang, H., Yan, R., Chen, H., Lee, D., and Zheng, C. (2007). Characteristics of hemicellulose, cellulose and lignin pyrolysis. Fuel 86, 1781. doi:10.1016/j.fuel.2006.12.013
Yoo, C. G., Pu, Y., Li, M., and Ragauskas, A. J. (2016). Elucidating structural characteristics of biomass using solution-state 2 D NMR with a mixture of deuterated dimethylsulfoxide and hexamethylphosphoramide. ChemSusChem 9, 1090. doi:10.1002/cssc.201600135
Keywords: ionic liquid, biomass, lignin, cellulose, hemicellulose, 31P NMR
Citation: Ben H, Chen X, Han G, Shao Y, Jiang W, Pu Y and Ragauskas AJ (2018) Characterization of Whole Biomasses in Pyridine Based Ionic Liquid at Low Temperature by 31P NMR: An Approach to Quantitatively Measure Hydroxyl Groups in Biomass As Their Original Structures. Front. Energy Res. 6:13. doi: 10.3389/fenrg.2018.00013
Received: 01 November 2017; Accepted: 28 February 2018;
Published: 26 March 2018
Edited by:
Uwe Schröder, Technische Universitat Braunschweig, GermanyReviewed by:
Kwang Ho Kim, Sandia National Laboratories, United StatesTianju Chen, Qingdao Institute of Bioenergy and Bioprocess Technology (CAS), China
Copyright: © 2018 Ben, Chen, Han, Shao, Jiang, Pu and Ragauskas. This is an open-access article distributed under the terms of the Creative Commons Attribution License (CC BY). The use, distribution or reproduction in other forums is permitted, provided the original author(s) and the copyright owner are credited and that the original publication in this journal is cited, in accordance with accepted academic practice. No use, distribution or reproduction is permitted which does not comply with these terms.
*Correspondence: Haoxi Ben, benhaoxi@gmail.com
†These authors have contributed equally to this work.