Preparation and Electrochemical Performance of a Graphene Cathode Catalyst Decorated With Pt by Prolysis
- 1Key Laboratory of Materials Modification by Laser, Ion and Electron Beams (Ministry of Education), School of Materials Science and Engineering, Dalian University of Technology, Dalian, China
- 2School of Materials Science and Engineering, Henan University of Technology, Zhengzhou, China
- 3Fuel Cell System and Engineering Laboratory, Key Laboratory of Fuel Cell and Hybrid Power Sources, Dalian Institute of Chemical Physics, Chinese Academy of Sciences, Dalian, China
For fuel cells, to produce high-quality and low-platinum catalyst is a pressing technical problem. In this study, graphene cathode catalysts with controllable platinum content were decorated by pyrolyzing chloroplatinic acid under various process parameters to obtain a high catalytic activity and durability. The results show that platinum particles generated by pyrolyzing chloroplatinic acid are uniformly loaded on graphene without agglomeration. The average particle size of platinum particles is about 2.12 nm. The oxygen reduction reaction catalytic activity of catalyst samples first increases, then decreases with increasing platinum loading in cyclic voltammetry and LSV. Compared with the commercial Pt/C (20 wt% Pt) catalyst, the initial potential and the current density retention rate of the catalyst decorated with 8% platinum are 55 mV and 23.7% higher, respectively. From i-t curves, it was found that the stability of the catalyst prepared in this paper was improved compared with the commercial Pt/C catalyst. The catalysts prepared in the present research exhibits superior catalytic activity and stability.
Introduction
Fuel cells are of worldwide interest due to their advantages of emitting less pollution and their high energy-conversion rate; they are recognized as a green, efficient, clean power-generation technology. The chemical energy in fuels and oxidants is directly converted into electric energy through catalysts without limiting the efficiency of the Carnot cycle (Steele and Heinzel, 2001; Wang et al., 2020). Catalytic materials are one of the important components of such fuel cells. The performance of a fuel cell is more affected by the oxygen reduction reaction (ORR) due to the slower multi-electron reaction at the cathode, which is why cathode catalysts are the focus of most research into fuel cells (Ioroi et al., 2019; Zaman et al., 2021). In the past few decades, many investigations have been undertaken on non-platinum-based cathode catalysts. Commercial Pt/C catalysts are often used as reference catalysts to measure the performance of self-made catalysts (Hu et al., 2018; Luo et al., 2021; Yang et al., 2021). For example, transition metal nitrogen-carbon catalysts have the advantages of low cost and high catalytic activity, however, the catalysts also show the disadvantages of poor stability. Ru-based compounds are the most extensively studied cathode catalytic materials among transition metal chalcogenides, however, as a precious metal, Ru is not only scarce but expensive. In addition, Co-based transition metal chalcogenides are considered to be a potential material, unfortunately, it is difficult to meet requirements imposed on both high activity and good stability under the working conditions of a PEMFC(Tran et al., 2020; Zhong et al., 2020). Although non-metallic heteroatoms doped with carbon catalysts have certain advantages in terms of cost and performance, they fail to meet the requirements for commercialization (Yang et al., 2013). In fact, highly dispersed platinum and its alloys supported on carbon materials have proven to be the most effective catalysts for oxygen reduction in commercial proton exchange membrane fuel cells. Low-platinum catalyst has the characteristics of low price and high catalytic activity, it is the research and development hotspot of fuel cell cathode catalyst. Therefore, how to produce high-quality and low-platinum catalyst is a pressing technical problem.
It has been shown that the carrier material of the catalyst should have good conductivity, a large specific surface area, high electrochemical corrosion resistance, and an appropriate porosity and porous structure (Wang et al., 2016). Honeycomb hexagonal-lattice graphene with sp2 hybridized orbitals is a two-dimensional carbon nanomaterial with a much higher specific surface area than carbon nanotubes, Vulcan XC-72, and graphite. Besides, the graphene has a high coefficient of thermal conductivity and good electrical conductivity. Many catalysts supported by graphene can not only extend the surface area of the active materials, reduce the amount of precious metals, but also improve the activity and stability of such catalysts (Begum and Kim, 2019; Bae et al., 2020; Das et al., 2020). Generally, the preparation of graphene-supported metal catalysts entails chemical reduction, electrochemical deposition, sol-gel, coprecipitation, hydrothermal, and solvothermal methods. The chemical reduction method is commonly used to prepare graphene-based catalysts, however, the activity of the interface between graphene and nanoparticles could be reduced by using reducing agents and organic solvents, leading to the poorer properties of the resulting composite materials (Zhou et al., 2015).
In the present work, chloroplatinic acid was pyrolyzed and reduced in an argon atmosphere. Differing from the existing literature, this method does not use reducing agents or organic solvents and is easy to realize the controllable preparation of platinum-loaded catalysts. It is simple, safe, and reliable. The catalysts were characterized by XRD, Raman spectroscopy, SEM, and TEM. The effects of platinum loading on the catalytic activity of oxygen reduction reaction were investigated by cyclic voltammetry (CV) and linear sweep voltammetry (LSV), and the durability of the catalysts was evaluated by chronoamperometry.
Experimental Methods
Six 50-mg specimens of graphene were added to 10 ml H2PtCl6•H2O solution at 0.625, 1.25, 2.5, 5, 8, and 16 g L−1 respectively and ultrasonicated for 2 h at 30 °C. The dispersed solution was lyophilized, dried, and powdered. The dried powder specimens were placed in crucibles, heated to 480°C at a rate of 5°C/min in a tubular furnace, and held at that temperature for 1 h. Argon was used as a protective gas. During the heat-preservation process, pumping and injecting were conducted at 20 min intervals. The resulting catalyst specimens have a theoretical platinum content of 4, 8, 16, 27, 38, and 55%, respectively.
The phase composition of the materials was analyzed by X-ray diffractometer (XRD, Empyrean, Panaco Company, Netherlands). The carbon structure was characterized by Raman spectrometer (HORIBA JOBIN YVON SAS, France). The elemental composition was determined by energy dispersive spectrometer (EDS). The morphology of the catalyst was observed, and the particle size was measured using a scanning electron microscope (SEM, Zeiss, SUPARR 55) and transmission electron microscope (TEM, Jem-2100, Japan Electronics Co., Ltd.).
For electrochemical analysis, 2 mg catalyst and 0.5 ml ethanol solution were ultrasonicated for 1 h to obtain the sample ink. The glassy carbon (GC) electrode (with a diameter d of 3 mm) was polished with alumina slurry on a rayon cloth and cleaned with distilled water. 5 μL catalyst ink was removed with a pipette to the surface of the polished electrode and dried for 1 h; 6 μL 0.5% Nafion solution was then added to the surface of the electrode and dried to form a thin film protecting the catalyst and separating it from the electrode (Shao et al., 2010). The solution system used in the test was a 0.1 M KOH electrolyte of saturated oxygen or nitrogen, and 60 sccm nitrogen was injected into the solution for 1 h before each test to remove gaseous impurities in the solution. Electrochemical measurement was performed using an electrochemical workstation (Shanghai Chenhua, CHI-760E) and rotating disk electrode (RDE) with a diameter of 3 mm. A three-electrode system was used with a platinum wire as the auxiliary electrode, an Ag/AgCl reference electrode, and a modified glassy carbon electrode as the working electrode. Oxygen reduction catalytic activity was characterized by cyclic voltammetry (CV) and linear scanning voltammetry (LSV) curves. The scanning rate and range of the CV test were 50 mV/s and -0.8–0.4 V, respectively. The scanning rate used in the LSV test was 10 mV/s under rotation at 400–2,400 rpm. The stability was obtained by running the test for 15,000 s at an onset potential of -1 V and a rate of rotation of 1,600 rpm by means of chronoamperometry. The aforementioned electrochemical measurements were conducted at room temperature (25°C).
Results and Discussion
The crystalline structure of the samples was analyzed by XRD (Figure 1A). The characteristic peaks of graphene at 2θ values of 26.3° and 54.5° correspond to the (002) and (004) crystal planes of the C standard card (PDF#41–1,487). The peaks at 39.8, 46.3, 67.7, and 81.3 correspond to the (111), (200), (220), and 311) crystal planes of FCC Pt (PDF # 04–0,802). this indicates that chloroplatinic acid is pyrolyzed and reduced at 480 °C to form platinum. With the increase of platinum content, the diffraction peak is stronger, and the peak position is not changed to any significant extent. The relative content of Pt (111) crystal plane of catalyst samples is between 58.60 and 60.55% according to calculations. Pt (111) is an ORR-sensitive crystal plane. A higher Pt (111) crystal plane content can promote oxygen dissociation and adsorption, thus improving the electrocatalytic activity of ORR (Xiong et al., 2020).
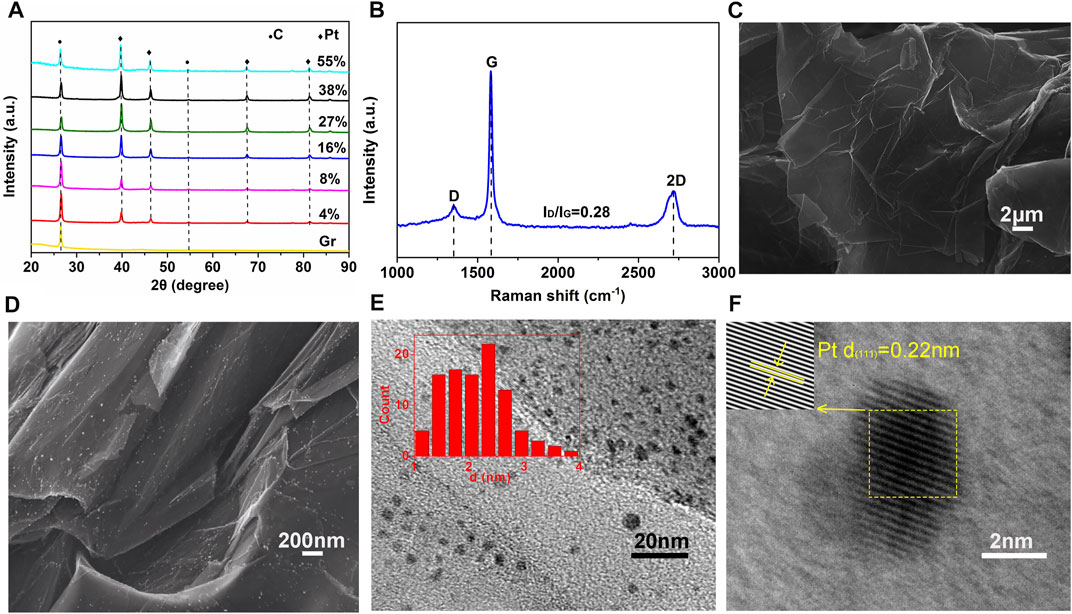
FIGURE 1. (A) XRD patterns of graphene and catalyst samples with a platinum content of 55, 38, 27, 16, 8, and 4 wt% and (B) Raman spectrum of the graphene decorated with 8 wt% Pt (C) (D) SEM (E) TEM (inset: particle size distribution histogram) and (F) HR-TEM images of graphene decorated with 8 wt% Pt.
The most effective and powerful non-destructive method of analysis used to determine the degree of disorder and defect structure of carbon materials is Raman spectroscopy. A typical Raman spectrum of the graphene decorated with 8 wt% Pt is shown in Figure 1B. The narrow and pointed peaks at 1,581.1 cm−1 and 1,347.7 cm−1 are called “ordered” graphite G and the “disordered” D band, respectively. The degree of graphitization can be obtained from the ratio ID/IG, and a lower value of 0.28 represents a high degree of graphitization. It could be concluded that there are far fewer structural defects in the catalyst with an almost perfect ordered graphite structure, which is resistant to electrochemical corrosion.
The SEM morphology of catalyst samples with a platinum content of 8% is shown in Figures 1C,D. It can be inferred that platinum particles generated by pyrolysis of chloroplatinic acid are uniformly loaded on graphene without agglomeration. The elemental content was assayed by EDS. TEM was used to observe the distribution of platinum, and Nano Measurer software was employed to estimate the average particle size of platinum particles. Figure 1E shows the TEM image with the histogram for of the particle size distribution. The average particle size of platinum particles is about 2.12 nm. The large specific surface area of graphene improves the rate of utilization of platinum and increases the number of active sites thereon. The lattice stripe with a crystal plane spacing of 0.22 nm is illustrated in Figure 1F, which corresponds to the highly-active Pt (111) crystal plane.
To study the catalytic activity of ORR, a three-electrode system was used to obtain CV curves of catalyst materials with different platinum contents. The CV curve was measured in N2 and O2-saturated 0.1-M KOH electrolyte at a scanning speed of 50 mV/s. Figure 2A shows the absence of an oxygen-reduction peak in the potential range of -0.8–0.4 V in N2-saturated conditions, while there is evidence of catalytic activity (as shown in Figure 2B) in O2-saturated conditions. The change in potential and current density at the oxygen-reduction peak is shown in Figure 2C. With the increase of platinum content, the oxygen-reduction peak first moves positively, then negatively; the peak current density first increases, then decreases, both reaching the extreme value at 38% (at a peak potential of -0.04 V. Ag/AgCl and a peak current density of 2.01 mA/cm2). Generally, the higher the platinum content, the better the catalytic activity of the ORR, however, it may lead to the aggregation of platinum particles and a decrease in catalytic activity if the platinum content is excessive.
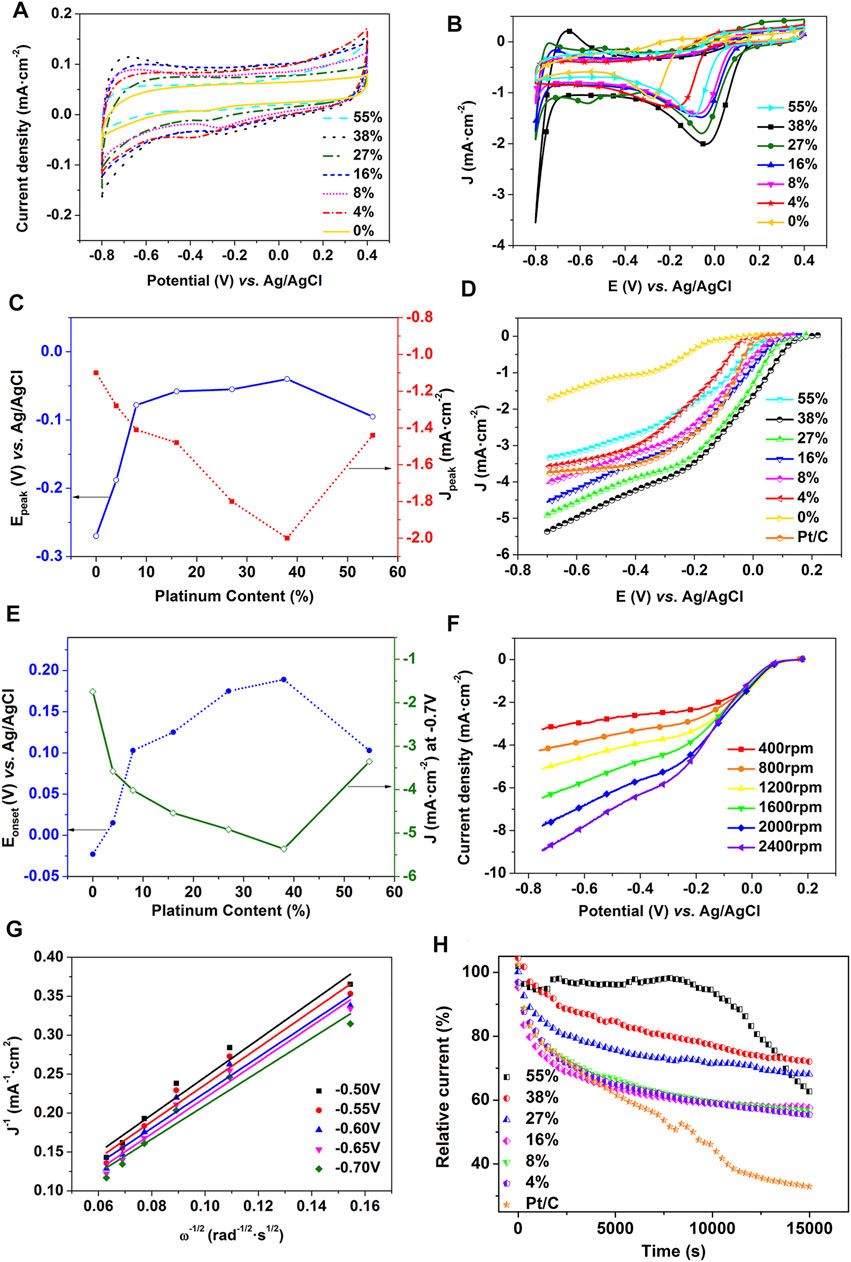
FIGURE 2. CVs of catalysts decorated with different amounts of Pt in (A) N2-saturated and (B) O2-saturated 0.1-M KOH(C) relationships between platinum content and peak current density and peak potential of CV curves (D) LSVs of Pt/C and catalysts with different platinum contents in O2-saturated 0.1-M KOH electrolyte at a rate of rotation of 1,200 rpm (E) relationships between platinum content and initial potential and current density (at -0.7 V) of LSV (F) LSVs (at different rates of rotation) (G) K-L curves (at a potential of -0.50 V to -0.70 V) of the specimen decorated with 27 wt% Pt (H) i-t curves of commercial Pt/C and samples with different platinum contents.
To assess the ORR catalytic activity of samples further, LSV curves of the catalysts with different platinum contents were tested in O2-saturated 0.1-M KOH solution using a rotating disk electrode. The scanning and rotating speeds during LSVs were 10 mV/s and 1,200 rpm, respectively.
As shown in Figure 2D, there are two current platforms in the graphene catalyst, and only one in the catalysts decorated with platinum. One current platform ensures that the oxygen-reduction process is dominated by four electrons (Wu et al., 2017). It can be seen from Figure 2E that, for the catalyst specimens with platinum contents of 0, 4, 8, 15, 27, 38, and 55%, the onset potentials were about -0.023, 0.015, 0.103, 0.125, 0.175, 0.189, and 0.103 V, and the current densities at -0.7 V were -1.745, -3.577, -4.017, -4.540, -4.918, -5.365, and -3.301 mA/cm2, respectively. The greater the onset potential, the greater the limiting current density, and the better the ORR catalytic activity. Changes in the LSV were consistent with those of the CV: both reached their extreme value at 38%. In addition, the LSV of commercial Pt/C (20 wt% Pt) was measured under the same experimental conditions. The results showed that the initial potential of commercial Pt/C was 0.048 V, which was lower than the initial potential of the prepared catalyst sample containing 8% platinum (this can be attributed to the good conductivity, the appropriate porosity of graphene, and the interaction between graphene and platinum).
The limiting current density increases with the increase in the rate of rotation because the diffusion distance of oxygen molecules is shortened at higher rotational speeds (Figure 2F). The oxygen-reduction reaction is dependent on the properties of the catalyst materials and electrolyte. The path of the ORR can be obtained by calculating the electron transfer number (n) of the catalytic materials. The catalyst has a high selectivity of four and two electron reaction paths when n is close to four and two, respectively. The ORR path is a mixture of two and four electrons when n is between two and four. The product of the two-electron reaction (HO2-) has a strong ability of be oxidized and high corrosivity. The four-electron reaction path will provide a higher voltage for fuel cells, so the two-electron reaction should be avoided as far as possible. The number of transferred electrons n can be calculated according to the Koutecky–Levich (K-L) equation (Eqs. 1 and (2)) (Suo et al., 2019) as follows:
Where J is the measured current density, JK represents the kinetic current density, JL is the limiting diffusion current density, n is the number of transferred electrons per oxygen molecule in the ORR, F denotes the Faraday constant (96,485 C mol−1), c0 is the oxygen concentration in the volume (1.2 × 10−3 mol L−1), B is the slope of the linear best-fit to the K-L equation,
Stability is also one of the important factors used to evaluate the performance of fuel cell catalysts. The stability of the catalyst was tested in O2-saturated 0.1-M KOH electrolyte by chronoamperometry (i-t) with a constant potential of -1 V and a rate of rotation of 1,600 rpm (Figure 2H): the current decline ratio of graphene catalysts modified with different platinum contents is within 50% after cycling for 15,000 s. The attenuation rate of i-t curve was shown in Table 1. With the increase of platinum content, the current decline ratio first decreases, then increases, and the graphene decorated with 38 wt% Pt has the best durability due to its lowest current decline ratio (29%). In contrast, the current through a commercial Pt/C (20 wt% Pt) catalyst is decreased by 67% under the same conditions. This indicates that the durability of catalyst specimens prepared by pyrolysis was significantly higher than that of the commercial Pt/C catalyst. This is due to the high resistance of graphene to electrochemical corrosion.
Conclusion
Graphene cathode catalysts modified with different amounts of platinum were prepared. According to the CV and LSV tests, the ORR catalytic activity of catalyst samples first increases, then decreases with increasing platinum loading. Compared with the commercial Pt/C (20 wt% Pt) catalyst, the catalyst decorated with 8 wt% Pt has a better ORR catalytic activity and durability. This means that the preparation method of pyrolyzing chloroplatinic acid on graphene can improve the catalytic activity and stability while requiring less platinum.
Data Availability Statement
The raw data supporting the conclusion of this article will be made available by the authors, without undue reservation.
Author Contributions
All authors listed have made a substantial, direct, and intellectual contribution to the work and approved it for publication.
Funding
This work was financially supported by Cultivation Programme for Young Backbone Teachers in Henan University of Technology (No. 21420117).
Conflict of Interest
The authors declare that the research was conducted in the absence of any commercial or financial relationships that could be construed as a potential conflict of interest.
Publisher’s Note
All claims expressed in this article are solely those of the authors and do not necessarily represent those of their affiliated organizations, or those of the publisher, the editors and the reviewers. Any product that may be evaluated in this article, or claim that may be made by its manufacturer, is not guaranteed or endorsed by the publisher.
Supplementary Material
The Supplementary Material for this article can be found online at: https://www.frontiersin.org/articles/10.3389/fenrg.2021.824733/full#supplementary-material
References
Bae, J. H., Park, J. H., Seo, M. K., and Kim, S. (2020). Synthesis and Electrochemical Performances of Platinum Decorated Polydopamine-Coated Carbon Nanotubes/graphene Composites as Fuel Cell Catalysts. J. Alloys Comp. 822, 153586. doi:10.1016/j.jallcom.2019.153586
Begum, H., and Kim, Y. B. (2019). Improvement of Catalytic Activity of Platinum Nanoparticles Decorated Carbon Graphene Composite on Oxygen Electroreduction for Fuel Cells. Processes 7 (9), 586. doi:10.3390/pr7090586
Das, E., Gursel, S. A., and Yurtcan, A. B. (2020). Pt-Alloy Decorated Graphene as an Efficient Electrocatalyst for PEM Fuel Cell Reactions. J. Supercrit. Fluids 165, 104962. doi:10.1016/j.supflu.2020.104962
Hu, C., Xiao, Y., Zou, Y., and Dai, L. (2018). Carbon-Based Metal-Free Electrocatalysis for Energy Conversion, Energy Storage, and Environmental Protection. Electrochem. Energ. Rev. 1 (1), 84–112. doi:10.1007/s41918-018-0003-2
Ioroi, T., Siroma, Z., Yamazaki, S., and Yasuda, K. (2019). Electrocatalysts for PEM Fuel Cells. Adv. Energ. Mater. 9 (23), 1801284. doi:10.1002/aenm.201801284
Luo, E., Chu, Y., Liu, J., Shi, Z., Zhu, S., Gong, L., et al. (2021). Pyrolyzed M–Nx Catalysts for Oxygen Reduction Reaction: Progress and Prospects. Energ. Environ. Sci. 14 (4), 2158–2185. doi:10.1039/d1ee00142f
Shao, Y., Zhang, S., Wang, C., Nie, Z., Liu, J., Wang, Y., et al. (2010). Highly Durable Graphene Nanoplatelets Supported Pt Nanocatalysts for Oxygen Reduction. J. Power Sourc. 195 (15), 4600–4605. doi:10.1016/j.jpowsour.2010.02.044
Steele, B. C. H., and Heinzel, A. (2001). Materials for Fuel-Cell Technologies. Nature 414 (6861), 345–352. doi:10.1038/35104620
Suo, N., Wu, A., Huang, H., Cao, G., and Zhang, G. (2019). Electrocatalytic Oxygen Reduction Reaction Activity of KOH Etched Carbon Films as Metal-free Cathodic Catalysts for Fuel Cells. RSC Adv. 9 (5), 2803–2811. doi:10.1039/c8ra08629j
Tran, T.-N., Lee, H.-Y., Park, J.-D., Kang, T.-H., Lee, B.-J., and Yu, J.-S. (2020). Synergistic CoN-Decorated Pt Catalyst on Two-Dimensional Porous Co-N-Doped Carbon Nanosheet for Enhanced Oxygen Reduction Activity and Durability. ACS Appl. Energ. Mater. 3 (7), 6310–6322. doi:10.1021/acsaem.0c00520
Wang, Y.-J., Fang, B., Li, H., Bi, X. T., and Wang, H. (2016). Progress in Modified Carbon Support Materials for Pt and Pt-alloy Cathode Catalysts in Polymer Electrolyte Membrane Fuel Cells. Prog. Mater. Sci. 82, 445–498. doi:10.1016/j.pmatsci.2016.06.002
Wang, Y., Ruiz Diaz, D. F., Chen, K. S., Wang, Z., and Adroher, X. C. (2020). Materials, Technological Status, and Fundamentals of PEM Fuel Cells - A Review. Mater. Today 32, 178–203. doi:10.1016/j.mattod.2019.06.005
Wu, B., Li, Q., Song, K., Zhu, X., Zhang, B., and Guo, J. (2017). Preparation and Catalytic Characterization of Pt/SiC with Less Platinum for Oxygen Reduction Reaction. J. Synth. Cryst. 46 (12), 2443–2450. doi:10.16553/j.cnki.issn1000-985x.2017.12.025
Xiong, Y., You, M., Liu, F., Wu, M., Cai, C., Ding, L., et al. (2020). Pt-Decorated, Nanocarbon-Intercalated, and N-Doped Graphene with Enhanced Activity and Stability for Oxygen Reduction Reaction. ACS Appl. Energ. Mater. 3 (3), 2490–2495. doi:10.1021/acsaem.9b02154
Yang, Z., Nie, H., Chen, X. a., Chen, X., and Huang, S. (2013). Recent Progress in Doped Carbon Nanomaterials as Effective Cathode Catalysts for Fuel Cell Oxygen Reduction Reaction. J. Power Sourc. 236, 238–249. doi:10.1016/j.jpowsour.2013.02.057
Yang, G., Zhu, J., Yuan, P., Hu, Y., Qu, G., Lu, B.-A., et al. (2021). Regulating Fe-Spin State by Atomically Dispersed Mn-N in Fe-N-C Catalysts with High Oxygen Reduction Activity. Nat. Commun. 12 (1), 1734. doi:10.1038/s41467-021-21919-5
Zaman, S., Huang, L., Douka, A. I., Yang, H., You, B., and Xia, B. Y. (2021). Oxygen Reduction Electrocatalysts toward Practical Fuel Cells: Progress and Perspectives. Angew. Chem. Int. Ed. 60, 17832–17852. doi:10.1002/anie.202016977
Zhong, J. P., Sun, M. L., Xiang, S., Fan, Y. J., Waqas, M., Huang, K. X., et al. (2020). Sulfonated Cobalt Phthalocyanine-Derived Co-N-S Tridoped Carbon Nanotubes as Platinum Catalyst Supports for Highly Efficient Methanol Electrooxidation. Appl. Surf. Sci. 511, 145519. doi:10.1016/j.apsusc.2020.145519
Keywords: fuel cell, cathode catalyst, oxygen reduction reaction, graphene, chloroplatinic acid
Citation: Wang X, Ren Y, Suo N and Zhang G (2022) Preparation and Electrochemical Performance of a Graphene Cathode Catalyst Decorated With Pt by Prolysis. Front. Energy Res. 9:824733. doi: 10.3389/fenrg.2021.824733
Received: 29 November 2021; Accepted: 16 December 2021;
Published: 12 January 2022.
Edited by:
Rongfang Wang, Qingdao University of Science and Technology, ChinaReviewed by:
Denghe Gao, Qingdao University of Science and Technology, ChinaXiao Xu, Qingdao University of Science and Technology, China
Copyright © 2022 Wang, Ren, Suo and Zhang. This is an open-access article distributed under the terms of the Creative Commons Attribution License (CC BY). The use, distribution or reproduction in other forums is permitted, provided the original author(s) and the copyright owner(s) are credited and that the original publication in this journal is cited, in accordance with accepted academic practice. No use, distribution or reproduction is permitted which does not comply with these terms.
*Correspondence: Ying Ren, ying_ren@haut.edu.cn; Guifeng Zhang, gfzhang@dlut.edu.cn
†These authors have contributed equally to this work and share first authorship