Temperature and Light Modulation of Herbicide Toxicity on Algal and Cyanobacterial Physiology
- 1Ecotoxicology of Aquatic Microorganisms Laboratory, Département des Sciences Biologiques – GRIL – TOXEN, Université du Québec à Montréal, Montréal, QC, Canada
- 2Laboratório de Fisiologia Vegetal, Departamento de Botânica, Instituto de Ciências Biológicas, Universidade Federal de Minas Gerais, Belo Horizonte, Brazil
HIGHLIGHTS
• We reviewed the interaction between light, temperature and herbicides on algal and cyanobacterial physiology.
• Temperature is the main factor affecting herbicide toxicity to algae and cyanobacteria.
• Changes in light environment may modulate the effects of photosynthesis-targeting herbicides.
Important interactions between climatic parameters and herbicide toxicity have been discussed in the literature. As climate changes are expected to influence the growth conditions of aquatic photosynthetic organisms over the next century by modifying the physicochemical parameters of the environment (such as temperature and incident light characteristics), the following questions arise: How will variations in climatic conditions influence herbicide toxicity in algae and cyanobacteria? Are these coupled effects on aquatic photosynthetic organism physiology antagonistic, additive, or synergistic? We discuss here the physiological responses of algae and cyanobacteria to the combined effects of environmental changes (temperature and light) and herbicide exposure. Both temperature and light are proposed to influence herbicide toxicity through acclimation processes that are mainly related to cell size and photosynthesis. Algal and cyanobacterial responses to interactions between light, temperature, and herbicides are species-specific, making it difficult today to establish a single model of how climate changes will affect toxicity of herbicides. Acclimation processes could assure the maintenance of primary production but total biodiversity should decrease in communities exposed to herbicides under changing temperature and light conditions. The inclusion of considerations on the impacts of environmental changes on toxicity of herbicides in water quality guidelines directed toward protecting aquatic life is now urgently needed.
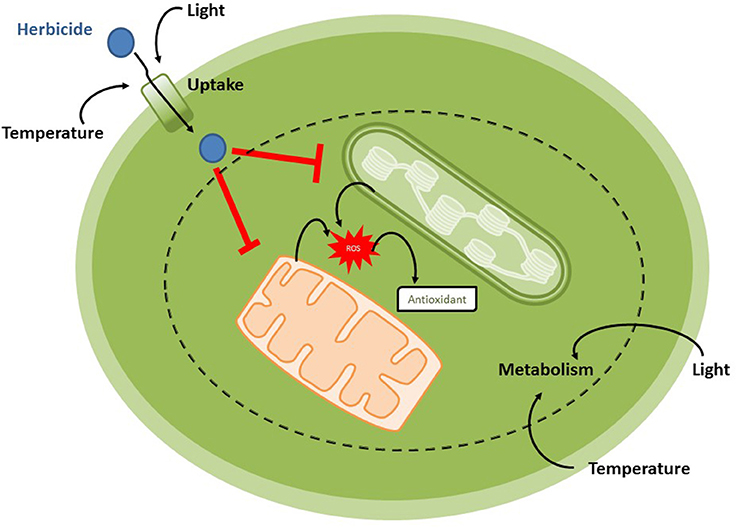
Graphical Abstract. Changes in temperature and light conditions in water systems over the next century will drive physiological responses of algal and cyanobacterial community to herbicides, by antagonistic, additive, or synergic effects with these pollutants.
Introduction
Climatic changes are expected to influence aquatic photosynthetic organism growth conditions over the next century by modifying physicochemical parameters of the environment such as temperature, precipitation, and incident light characteristics (Finkel et al., 2010). Changes in water temperature, pH, UV-B in the upper water column (due to ozone depletion), and the introduction of pollutants into waterways induce stress on algal and cyanobacterial communities (Finkel et al., 2010), affecting their physiological processes. Due to the central role of aquatic photosynthetic organisms for global primary production, the identification of their physiological responses to these changes is essential to our understanding of how these natural and anthropogenic alterations of the aquatic ecosystems will affect the carbon-climate system at a global scale.
Parameters linked to the photosynthetic activity of plants have been shown to be useful to study responses to stress conditions (Juneau et al., 2007), mainly because photosynthesis is directly associated with growth and primary production. Several studies have examined the effects of climatic factors (such as temperature and irradiance) on photosynthesis of aquatic organisms (Huner et al., 2003; Necchi Jr, 2004). Temperature, for example, has been observed to modulate algal and cyanobacterial photosynthesis by modifying pigment and lipid compositions (and thus membrane fluidity) and enzyme activities (Huner et al., 2003; Necchi Jr, 2004; Chalifour et al., 2014). Although photoacclimation processes allow aquatic organisms to adjust their photosynthetic apparatus in response to changing light environments, prolonged exposure to high light intensities can induce photoinhibition and photodamage (Huner et al., 2003).
The development of intensive agriculture in areas near aquatic ecosystems had led to increased agricultural waste load entering these waterways (Costanzoi and Dennison, 2003). Among the agricultural wastes, nutrient inputs, such as phosphates and nitrates, are known to increase eutrophication of lakes, rivers and coastlines (Costanzoi and Dennison, 2003), and these nutrients are rarely limiting in these ecosystems. In most laboratorial studies on algal physiological responses, media used contain enough nutrients (like in agricultural area aquatic ecosystems) to allow maximum growth rate, which therefore, are not problematic for physiological processes associated to growth and photosynthesis. Therefore, in this review, the effects of agricultural nutrient inputs will not be considered. In contrast, anthropogenic additions of hazardous compounds to waterways can significantly affect photosynthesis of aquatic organisms (DeLorenzo et al., 2001), and agrotoxics (mainly herbicides) heavily used in agricultural and industrial practices are notorious for their effects on algal and cyanobacterial physiology and growth (Bérard et al., 1999; Chalifour and Juneau, 2011). Photosynthesis-targeting herbicides, such as atrazine, simazine and terbuthylazine, act primarily on photosynthetic light reactions and inhibit carbon assimilation (Jursinic and Stemler, 1983). On the other hand, even if photosynthesis and respiration are not the primary target of some herbicides (such as glyphosate), these metabolic pathways may be also affected by these substances (Qiu et al., 2013; Gomes and Juneau, 2016; Gomes et al., 2016). Although it has been banned or restricted in several countries, PSII inhibitors such as atrazine, simazine and terbuthylazine can be detected in the majority of surface waters of agricultural areas (Comber, 1999). Together with glyphosate, the most used herbicide worldwide, photosynthesis-targeting herbicides are important contaminants of waters, and therefore, the present review focused on the interaction between environmental factors and these herbicides. Table 1 shows some examples of maximal concentrations of herbicides found in waters around the world. Although light is essential to perform photosynthesis, adequate levels (those that do not induce photoinhibition) are needed otherwise reactive oxygen species (ROS) accumulation and photosynthetic activity decrease will occur (Waring et al., 2010; McGinty et al., 2012). Similarly, temperature needs to be optimal in order to maintain maximal enzyme activity (Huner et al., 2003; Necchi Jr, 2004), including antioxidant enzymes necessary to keep ROS under non-harmful physiological levels. The deleterious effects of some herbicides on photosynthesis have been shown to be associated with cell oxidative bursts due to ROS accumulation (Jursinic and Stemler, 1983; Cuypers et al., 2001; Romero et al., 2011; Gomes et al., 2016) which promotes lipid peroxidation, leading to the destruction of cell membrane systems such as, chloroplast thylakoids (Li et al., 2006; Hao et al., 2010). In this context, a physiologically interconnected interpretation of the environmental condition (natural and anthropogenic factors) impacts on aquatic organism's primary production is needed.
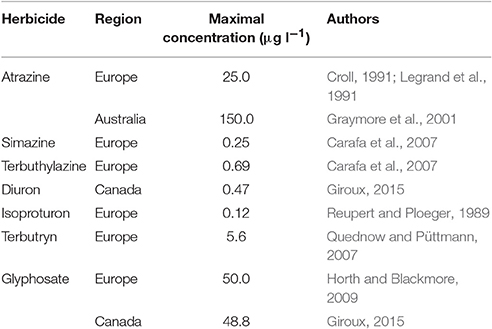
Table 1. Examples of maximal concentrations of herbicides (PSII-inhibitors and glyphosate) in waters of different regions.
The impacts of some herbicides such as the bipyridinium (i.e., diquat and paraquat) and fluometuron are closely linked to climatic factors such as light. Indeed, in presence of light, bipyridinium herbicides inhibit photosynthesis by intercepting electrons from the reducing side of PSI, forming relatively stable free radicals; in the presence of oxygen, the bipyridinium free radical is then oxidized, which leads to the formation of a highly activated oxygen species that promote cellular oxidative bursts (DeLorenzo et al., 2001). Fluometuron, in turn, on the top of its action on PSII electron transport (Wilkinson et al., 2015) inhibits carotene biosynthesis and deprives cells of their photo-oxidative carotene shield—leading to chlorophyll degradation and decreases in photosynthetic rates (DeLorenzo et al., 2001). In the absence of light, however, neither of these herbicides is effective (Sikka and Pramer, 1968; Corbett et al., 1984). Similarly, temperature has been shown to modulate atrazine toxicity in microalgae (Chalifour and Juneau, 2011). These results indicate the existence of important interactions between climatic parameters and the toxicity of herbicides. In this context, the following questions arise: How do variations in climatic conditions influence herbicide toxicity in algae and cyanobacteria? Are their coupled effects on photosynthetic aquatic organism's physiology antagonistic, additive, or synergistic?
We discuss here the physiological responses of algae and cyanobacteria (both phytoplanktonic and periphytic since their physiological processes are similar) to the combined effects of environmental changes (temperature and light) and herbicide exposure. Firstly, physiological processes associated to aquatic photosynthetic organism acclimation to changes in temperature and light conditions are presented. Then, the interactive effects of changes in temperature or light conditions and herbicide toxicity to algae and cyanobacteria are discussed. In addition to contributing to the field of plant physiology, this review provides unique perspectives for better understanding natural aquatic photosynthetic organism community responses to herbicides in the context of climate change.
Acclimation
Acclimation is the process by which organisms can adjust to environmental changes and maintain their performance levels under new environmental conditions. By modifying their physiological responses, aquatic photosynthetic organism communities can deal with different conditions of temperature, light (Davison, 1991), and contaminant exposure. Although acclimation processes may initiate within just few hours (Davison, 1991), acclimation/adaptation times reported for microalgae can vary from hours to many weeks (Davison, 1991; Chalifour and Juneau, 2011; Larras et al., 2013)—an important aspect that must be considered in studies evaluating algal and cyanobacterial acclimation in response to new environmental pressures.
Thermal-Acclimation
Temperature is an important regulating factor for primary production in aquatic environments as it controls fundamental organism functional properties such as photosynthesis, respiration, and nutrient uptake (Staehr and Sand-Jensen, 2006). Photochemical processes are influenced by temperature since membrane fluidity and diffusion of electron carriers are modulated by temperature changes (Los and Murata, 2004). Optimum temperatures for photosynthesis, when carbon assimilation is the highest, are species-specific. In general, however, at low temperatures, the viscosity of the membranes is increased, preventing the transport of associated components into the membranes, while the reverse is observed at higher temperatures (up to a limit where degradation of the membranes and proteins is observed) (Barber et al., 1984). Moreover, temperature modulates intermolecular collision processes of electron carriers (such as plastoquinone and plastocyanin) that independently of any effects on membrane fluidity per se, increases the oxidation-reduction turnover times of these components under low temperatures (Falkowski and Raven, 2013). Low temperatures also induce decreased Rubisco activity (Staehr and Sand-Jensen, 2006). This constraint gradually vanishes, however, as temperature increases across the optimum temperature range, stimulating carbon fixation (Staehr and Sand-Jensen, 2006). The Calvin cycle enzyme activity also represents a point of temperature modulation of plant photochemistry. In this context, the maximum rate of electron transport is lower under low temperatures and accelerated under higher temperatures (Pimentel et al., 2007).
Similarly to Calvin cycle, respiratory processes are less active under lower temperatures and activated at higher temperatures, demonstrating that temperature can also modulate mitochondrial activities (Atkin and Tjoelker, 2003). There is an optimal temperature for photosynthesis; beyond that temperature, the anabolism of carbon skeleton molecules (by respiration, for instance) are greater than their production (by photosynthesis) which leads to decreasing growth rates (and increases in light requirements for survival). Moreover, if temperatures continue to rise, higher membrane fluidity and enhanced protein and enzyme degradation rates (Daniel et al., 1996; Los and Murata, 2004) will decrease aquatic photosynthetic organism performances. It is also important to note that increased metabolism also increases nutrient demands (Rhee and Gothan, 1981). Nutritional status is directly linked to pigment and enzyme concentrations and, as nutrient availability will vary among different aquatic systems and seasons, it can markedly influence metabolic processes in response to temperature changes (Raven and Geider, 1988). Similarly, increased membrane fluidity can result in increased cell permeability to water pollutants, which will also modulate algal and cyanobacterial responses to temperature changes.
Algal and cyanobacterial species have the ability to acclimate to changes in water temperatures and can alter their physiological processes within just a few generations (Vona et al., 2004). At low temperatures, chlorophyll/cell ratio tends to become reduced, while carboxylation activity tends to increase (through increasing Rubisco levels) (Davison, 1991). These alterations lead to the reduction in light absorption capacity to avoid photoinhibition, while increasing carbon assimilation. Thus, aquatic photosynthetic organism adaptations to low temperatures are basically through acclimation to high photosystem II excitation pressure (Maxwell et al., 1995). In contrast, under high temperature conditions, thermal acclimation assures a balance between photosynthesis and respiration, and the temperatures required for optimal photosynthesis rates are often lower than those of respiration (Raven and Geider, 1988). This means that high temperatures will constrain photosynthesis more than respiration, resulting in higher consumption rates of ATP and carbohydrates than production rates (Raven and Geider, 1988), so that mechanisms allowing acclimation of the photosynthetic apparatus will be crucial in determining algal and cyanobacterial survival. Thermal acclimation modulates also antioxidant system activities that protect proteins and membranes from oxidative stress induced, for example, by low temperatures (Suzuki and Mittler, 2006). Changes in lipid composition is also a common response of thermal acclimation (mainly to low temperatures) to adjust membrane fluidity (Falkowski and Raven, 2013), thereby maintaining PSII stability (Hölzl and Dörmann, 2007) while allowing the movement of components in the electron transport chain (Sarcina et al., 2001).
The influence of temperature on algal and cyanobacterial pigment contents, especially on chlorophyll a (which is generally used to estimate the biomasses of photosynthetic microorganisms), has also received substantial attention—but inconsistent results have been reported in the literature. Increased chlorophyll content at 10°C compared to 25°C have been observed in the mesophilic species Scenedesmus obliquus (Chalifour and Juneau, 2011). Similarly, chlorophyll and carotenoid contents were lower in the green algae Chlamydomonas reinhardtii grown under 15°C compared to 25°C (Chalifour et al., 2014). Chen et al. (2010), in turns, observed an increased chlorophyll content in the cyanobacteria Microcystis aeruginosa grown at 25°C in relation to 10°C. On the other hand, chlorophyll content was not significantly affected by temperature in the diatom Navicula pelliculosa (at 10, 15, and 25°C) (Chalifour and Juneau, 2011) or in the cyanobacteria Merismopedia tenuissima and Oscillatoria sp. (at 15, 20, 25, and 30°C) (Coles and Jones, 2000). Temperature effects on pigment content may therefore vary depending on the algal and cyanobacterial species, the growth temperature, and light intensity. The unimodal relationship between functional properties and temperature can be altered by acclimation processes (Staehr and Sand-Jensen, 2006). Since aquatic photosynthetic organisms can tolerate relatively large changes in environmental temperatures through the adjustment into their cellular physiology (Staehr and Birkeland, 2006), the effects of global warming on algal and cyanobacterial productivity could be less dramatic than predicted on studies based on models using non-acclimated species (Atkin and Tjoelker, 2003).
Photo-Acclimation
Aquatic organisms are exposed to variable light intensities throughout the growing season due to daily and seasonal annual changes in light availability, or to their position in the water column (Dubinsky and Stambler, 2009). These modifications in light intensity are amplified by the physical properties of the water and by the presence of suspended particles and dissolved chemicals that attenuate light, creating highly variable light environments depending on the habitats (Smith and Mobley, 2008). High light levels during sunny days can be sufficient to induce photoinhibition, while light attenuation by the water column may impose restraints on photosynthetic organisms just a few meters below the surface (Smith and Mobley, 2008). Within this context, photoacclimation processes aid photosynthetic aquatic organism's survival under changing light conditions.
Morphological traits such as interspecific cell size variations, the formation of colonies, filaments, or cell clusters, and cell motility will affect light use efficiency in phytoplankton (Deblois et al., 2013). Colonial organization is an ecological gain since it provides grazing protection; however, it stablish a condition of lower light availability for cells, once this kind of organization increases density (and thus the sinking rate) at the same time that promotes self-shading (Falkowski and Raven, 2013). Flagella or vacuoles allow phytoplankton to move in the water column and optimize light harvesting (Falkowski and Raven, 2013). Pigment variability (chlorophyll, carotenoids, and phycobiliproteins) is also other source of variation in phytoplankton light harvesting capacity (Falkowski and Raven, 2013). It is also known that the production of ultraviolet-radiation absorbing micosporne-like amino acids (MLAAs) in cyanobacteria varies with the intensity of photosynthetically active radiation (PAR) (Torres et al., 2016). Together, these characters can influence photoacclimation processes and help to explain the different photoacclimation strategies observed among different algal and cyanobacterial species.
Photoacclimation mechanisms tend to equilibrate light energy harvested and the energy used in metabolism in order to maximize adenosine triphosphate (ATP) and nicotinamide adenine dinucleotide phosphate (NADPH) production in accordance with cellular activities (Falkowski and Raven, 2013). Some important photoacclimation mechanisms involve modifications in: the sizes of light harvesting complexes (LHC); pigment compositions and concentrations; and the stoichiometry of PSI and PSII, and the components of the electron transport chain (MacIntyre et al., 2002). Changes in LHC size allow phytoplankton to increase or decrease the energy delivered to their photosystems (Behrenfeld et al., 2004). Similarly, modifications of chlorophyll contents modulate photon harvesting—increasing or decreasing the energy processed in the photosynthetic apparatus (Deblois et al., 2013). Additionally, as chlorophyll is an important component of photosynthetic reaction centers (RC), modulation of chlorophyll contents will induce changes in RC stoichiometry (Mauzerall and Greenbaum, 1989). In addition to the protective roles of photoprotective pigments (i.e., carotenoids), changes in their concentrations also help control the amounts of energy directed to the PS during light harvesting by increasing or decreasing light dissipation as heat (Kirilovsky, 2007). The non-photochemical processes were also shown to play a critical role in protection against high light when diatoms are exposed to a combination of environmental factors (Juneau et al., 2015). Finally, changes in PS stoichiometry can increase or decrease PSI to PSII ratios, equilibrating the flow of energy from PSII to PSI and reducing light pressure on the photosynthetic apparatus (Sonoike et al., 2001). Under low light (LL) conditions, phytoplankton adjust their cellular constituents to increase their light absorption efficiency, which involves down-regulation or de novo synthesis of photosynthetic (chlorophyll) and photoprotective pigments (carotenoids), PS, Rubisco, and ultrastructural cell alterations (Herzig and Dubinsky, 1992). Under high light (HL) conditions, in turns, photoacclimation allows phytoplankton to avoid photoinhibition and oxidative damage by decreasing their chlorophyll and increasing their carotenoid contents—which are associated with decreases in the numbers of PS and changes in LHC size (MacIntyre et al., 2002).
Photoinhibition and oxidative stress are closely related. ROS are continuously produced during chloroplast activity: the triplet chlorophyll, the electron transport chain, and the oxygen evolution complex are all ROS production sites (Gomes and Garcia, 2013), and the over-excitation of PS by HL induces ROS production in phytoplankton (Herzig and Dubinsky, 1992). Within this context, the activity of antioxidant systems (i.e., antioxidant-enzymes) is another site of modulation of the photoacclimation process (MacIntyre et al., 2002). Little attention, however, has been given to this important physiological aspect that may be intrinsically related to algal and cyanobacterial survival under light changing environments.
Although acclimation processes may assure their survival under environmental changes in temperature and light conditions, algae and cyanobacteria in aquatic systems are also exposed to hazardous compounds, such as herbicides, and their ability to cope with these compounds may be influenced by the physiological responses to changes in temperature and light conditions. In the following section, the interaction between temperature/light herbicides is discussed.
Temperature and Herbicides in Phytoplankton and Periphyton
General Concern
Anthropogenic disturbances that occurred over the past century have driven relatively rapid changes in environmental temperatures resulting in global warming, affecting both land and aquatic habitats (Knutti et al., 2016). As temperature is a major determinant of the broad-scale distribution of organisms (Haidekker and Hering, 2008), one important factor of biological responses to climate change will be the extent of those temperature changes (Deutsch et al., 2008).
Fluctuations in growth temperatures are known to alter the effects of chemical stressors (Folt et al., 1999; Chalifour and Juneau, 2011), but the implication of these interactive effects has not been yet addressed in standard toxicity test protocols (Folt et al., 1999). The interactions of combined chemical and physical stressors may create effects that are either greater than, or lesser than, those of each stressor alone (Crain et al., 2008). Consequently, it is essential to understand when natural variations in environmental variables modify the toxicity of water pollutants and to incorporate this knowledge into environmental regulation and risk evaluation and mitigation strategies (Chen et al., 2008).
Pollutants and temperature are two environmental factors that can affect algal and cyanobacterial photosynthesis and growth in natural environments. Pollutants often inhibit photosynthesis and growth, which are also affected by changes in temperature. Temperature modulates the enzymatic activities involved in repair processes (including the activation, synthesis, or repair of damaged molecules) (Sobrino and Neale, 2007) needed to counteract the damages induced by exposure to pollutants. Temperature can also interact additively or synergistically with pollutants, accentuating their detrimental effects on cellular mechanisms (Bicalho et al., 2017).
Numerous studies have demonstrated the increasing frequency of chronic or acute pollution of waterways with high levels of pesticide (especially herbicide) residues (IFEN, 2006; Sullivan et al., 2009; Giroux, 2015). Aquatic environments near agricultural fields receive direct and indirect herbicide inputs that may impact the primary production of algae and cyanobacteria communities (and therefore other trophic levels) (DeLorenzo et al., 2001). In the following sections the interaction between temperature and some herbicides are reviewed.
Case of PSII Inhibitors and Temperature
Atrazine (6-chloro-N-ethyl-N′-(1-methylethyl)-1,3,5-triazine-2,4,diamine) is the most frequently detected herbicide in aquatic systems (Rebich et al., 2004; Giroux, 2015). Atrazine concentrations can exceed the general quality standard for surface and drinking water (5 μg l−1) (Giroux, 2015). Values ranging from 9 to 25 μg l−1 in Europe (Croll, 1991; Legrand et al., 1991), 87 to 100 μg l−1 in North America (Steinheimer, 1993; Graymore et al., 2001), and up to 150 μg l−1 in Australia (Graymore et al., 2001) have been reported. Although it has been proposed an acute toxicity threshold value of 20 μg atrazine l−1, (Diana et al., 2000), under concentrations lower than 20 μg l−1, atrazine was reported to cause sub-lethal effects in various aquatic organisms such as unicellular algae (DeLorenzo et al., 2001). This herbicide interacts with the Qb-binding site on the D1 protein of PSII, resulting in the inhibition of photosynthetic electron transport with consequent ROS formation (Jursinic and Stemler, 1983). ROS accumulation ultimately induces cellular oxidative bursts that damage pigments and proteins, and the lipids of the photosynthetic apparatus (Gonzáles-Barreiro et al., 2004). Atrazine and temperature are therefore both environmental factors that affect algal and cyanobacterial photochemistry, and their interactive effects have been previously studied (Bérard et al., 1999; Debenest et al., 2010; Chalifour and Juneau, 2011; Larras et al., 2013). Since atrazine is mainly applied when water temperatures are still low (<20°C) in the Northern hemisphere, its interaction with temperature is essential to take into account. The 96-h EC50 values for growth rate in the green algal species Raphidocelis subcapitata exposed to atrazine (0–250 μg l−1) were diminished with decreases in temperature (20 to 5°C), indicating a higher toxicity at lower temperature (Baxter et al., 2016). In an in vivo experiment, Bérard et al. (1999) observed that the cyanobacteria Oscillatoria limneltica was twice as sensitive to atrazine (10 μg l−1) at low temperature (13°C) compared to higher temperature (20°C). The EC50 for O. limnetica was 24.2 μg l−1 of atrazine at 13 °C, and 52.3 μg l−1 of atrazine at 20 °C. This temperature-sensitivity response was attributed to the low turnover rates of D1 proteins, the specific target site of this herbicide, at low temperature (Bérard et al., 1999). Since D1 protein turnover is less effective at low temperatures (Ross and Vincent, 1988), low temperatures contribute to the deleterious effects of atrazine on the photosynthetic apparatus by decreasing PSII activity recovery after atrazine binding. It is important to note here that cyanobacteria are more characteristic of aquatic systems at warmer temperatures (Butterwick et al., 2004). Therefore, the increased sensitivity of cyanobacteria species to atrazine under low temperatures could be also accentuated by the sensitivity of these species to low temperatures (Elliott, 2010)—a relevant aspect to be considered in interaction studies.
Chalifour and Juneau (2011) attributed the toxic effects of atrazine on phytoplankton growing at low temperatures to their capacity for cell thermal energy dissipation (non-photochemical energy dissipation processes). They also shown that atrazine (0.1 μM = 21.56 μg l−1) reduced the electron transport rate (ETR) and kept the plastoquinone pool in an oxidized state (similarly to light limitation effect) in the green algae S. obliquus and in the cyanobacteria M. aeruginosa. Light limitation of the PSII is known to up-regulate LHCII polypeptide and chlorophyll a accumulation (Wilson and Huner, 2000), increasing light absorbing capacity of the cells. Due to the blockage of electron transport by atrazine, increased light absorption will therefore result in increased photo-oxidative damage. Additionally, decreases in ETR due to atrazine exposure decreased xanthophyll-cycle-dependent non-photochemical quenching in the green algal cells (Chalifour and Juneau, 2011) that is dependent on the trans-thylakoid ΔpH (Gilmore, 1997). Decreased non-photochemical quenching (NPQ) was reported for green algae exposed to 0.1 μM atrazine at 10 and 15°C (Chalifour and Juneau, 2011). These authors also noted that a toxic M. aeruginosa strain had greater reduction in NPQ at 15°C than at higher temperatures and was more sensitive to atrazine than the non-toxic strain. Thus, we may advance that atrazine-sensitive populations showed reduced abilities to cope with excess energy at low temperatures. On the other hand, these same authors observed increased effective dissipation in active reaction centers (DI0/RC) in the diatom N. pelliculosa at low temperatures (10°C) when exposed to atrazine. This increase in the efficiency of excess energy dissipation was related to the high atrazine tolerance of this diatom (as compared to the other species studied). In their study on Anabaena circinalis populations acclimated to increased photon flux densities (PFD - 50, 130, and 230 μmol·m−2·s−1 of PAR), Millie et al. (1992) observed that decreasing photosynthetic activity and pigment content (chlorophyll a and carotenoid) coincided with increasing PFD. Interestingly, increased sensitivity to simazine (another triazine herbicide that also blocks PSII electron transport) was also seen with increased PFD. These authors concluded that sensitivity to PSII inhibitors was influenced by alterations in the pigment content. High light and low temperature acclimation are similar conditions in terms of light excitation pressure (Maxwell et al., 1995) and, in this context, the acclimatization of photosynthetic aquatic organisms sensitive to atrazine is related to their pigment contents and their thermal energy dissipation abilities. The important role of carotenoids as ROS scavengers and in protecting photosystems from oxidative bursts by quenching the deleterious effects of triplet chlorophyll and singlet oxygen is well-established (Boussiba, 2000). Additionally, since β-carotene is involved in the xanthophyll biosynthetic pathway in algal cells (Bouvier et al., 1996), modulation of carotenoid contents could directly affect their NPQ ability.
In several countries where atrazine was banned for agricultural usage, triazine (PSII-inhibitors), such as terbuthylazine (TBA) and simazine (SIM), has been used as substitutes, and significant concentrations (from 0.57 to 694.32 ng l−1 and 1.45 ng l−1 to 25.96 ng l−1 for TBA and SIM, respectively) of these herbicides and their byproducts were observed in aquatic environments (Carafa et al., 2007). It was found that concentrations of these herbicides in aquatic environments followed a clear seasonal pattern, with higher concentrations being detected during spring periods (Carafa et al., 2007). Studying the effects of temperature increase (from 15°C to, 25°C) on cellular responses of the marine diatom Skeletonema marinoi to environmental to sub-lethal levels (1, 5, 10, 20, and 30 μg l−1) of TBA, Fiori and Pistocchi (2014), observed that this species was quite resistant to TBA exposure at 15°C. However, enhanced temperature increased the algal sensitivity to this herbicide. According to the authors, the low division rates associated with the high nutrient uptake rates at the lowest temperature may contribute with the gradual adaptation of the algal photosynthetic apparatus to the PSII inhibition caused by TBA. Moreover, it is shown that diatoms were more sensitive to triazine herbicides than other species (Bérard et al., 1999; Bérard and Benninghoff, 2001; Chalifour and Juneau, 2011) including harmful flagellate species, specially under rising temperatures. Therefore, the shift in photosynthetic aquatic community composition due to herbicide run off in coastal areas associated with increasing temperatures could led to losses in some essential species for global primary production such as S. marinoi.
Other important PSII inhibitor herbicides observed in aquatic systems are diuron, isoproturon and terbutryn, with concentrations up to 0.47 μg l−1 (Giroux, 2015), 0.12 μg l−1 (Reupert and Ploeger, 1989) and 5.60 μg l−1, (Quednow and Püttmann, 2007), respectively, being reported. By evaluating the effects of temperature (18, 21, 24 and 28 °C) and two concentrations of an equitoxic mixture of diuron (5.36 or 32.63 μg l−1), isoproturon (4.74 or 28.87 μg l−1), atrazine (4.96 or 30.19 μg l−1), and terbutryn (5.55 or 33.78 μg l−1) on freshwater periphytic algae, Larras et al. (2013) noted lower sensitivities of the diatoms when grown at higher temperatures. Similarly, Tasmin et al. (2014a,b) observed that rising water temperatures (from 10 to 30°C) reduced the toxicity of diuron (up to 32 μg l−1) on the green algae Pseudokirchneriella subcapitata. This could be due to the smaller biovolumes of these organisms compared to the other species and to their decreased herbicide uptake rates (Tang et al., 1998; Larras et al., 2013). As seen with herbicides, decreased biovolumes will also limit nutrient uptake in diatoms—so that additional tolerance mechanisms involving better nutrient recycling, or specialized mechanisms of nutrient uptake with herbicide avoidance, may also be involved. In this context, it is important to note that temperature modulation of lipid composition is necessary to maintain membrane fluidity. This acclimation is important to assure efficient photosynthetic electron transport (which is dependent upon membrane-protein interactions) (Morgan-Kiss and Dolhi, 2012). Changes in membrane composition, however, can also drive changes in membrane permeability, reducing or increasing the penetration of pollutants into the cells (Tuckey et al., 2002), thus modulating their inhibitory effects on photosynthetic aquatic organisms.
Case of Glyphosate and Temperature
The introduction of glyphosate-resistant (GR) plants (Coupe et al., 2012) has resulted in glyphosate [N-(phosphonomethyl)glycine) becoming the most widely used herbicide worldwide. Due to its high water solubility and extensive use, glyphosate exposure of non-target aquatic organisms is a growing concern (Tsui and Chu, 2003). While glyphosate has been detected in many aquatic systems it rarely exceeds a few micrograms per liter (Pesce et al., 2008, 2009), which could be related to its rapid degradation to its major metabolite, aminomethylphosphonic acid (AMPA) (Kolpin et al., 2006; Borggaard and Gimsing, 2008). In surface waters, concentrations ranging from 1 to 50 μg glyphosate l−1 and from 4 to 48.9 μg AMPA l−1 were reported in Europe (Horth and Blackmore, 2009), while in Canada, concentrations observed were ≤ 40.8 and ≤ 1.1 μg l−1 for glyphosate and AMPA, respectively (Giroux, 2015). AMPA has been identified as toxic to higher plants (Reddy et al., 2004), and was shown to disrupt chlorophyll biosynthesis (Gomes et al., 2016); its effects on algae and cyanobacteria, however, have not been thoroughly investigated.
Algal and cyanobacterial tolerance to glyphosate can vary among the species, but phytoplankton are generally considered tolerant to this herbicide (Wong, 2000; Tsui and Chu, 2003; Arunakumara et al., 2013; Wang et al., 2016). Glyphosate and AMPA have been shown to be degraded by cyanobacteria activity in aqueous environments (as reviewed by Arunakumara et al., 2013), and glyphosate mineralization could be related to the low glyphosate sensitivity of phytoplankton. Stachowski-Haberkorn et al. (2008) observed shifts in microbial diversity in in situ microcosms in a coastal ecosystem treated with low concentrations of Roundup (<10 μg l−1). Glyphosate (e.g., at 150 μg kg−1 of sediment dry weight) also affected freshwater sediment microbial communities (Widenfalk et al., 2008). Some toxic effects of a commercial formulation of glyphosate (Factor 540—at 50 μg l−1 of glyphosate) on photosynthesis and growth were recently demonstrated for various phytoplankton species (Smedbol et al., under revision). These observations suggest that glyphosate exposure may affect photosynthetic aquatic organism communities, possibly by a glyphosate herbicidal mode of action or through glyphosate mineralization. Additionally, the growth of species able to degrade glyphosate and AMPA may be stimulated, as glyphosate mineralization represents a potential source of nutritional phosphorus and nitrogen (Widenfalk et al., 2008; Wang et al., 2016).
The detrimental effects of glyphosate are due to its inhibition of the enzyme 5-enolpyruvylshikimate-3-phosphate (EPSP) synthase (an enzyme of the shikimate pathway), which prevents the biosynthesis of aromatic amino acids (Siehl, 1997), leading to the reduction of protein synthesis. It has also been reported that glyphosate has detrimental effects on photosynthetic processes that could be directly or indirectly linked to glyphosate-induced reductions of plastoquinone (PQ) biosynthesis (Cobb and Reade, 2010; Gomes et al., 2017b) and chlorophyll concentrations (Gomes et al., 2016). Reduction of PQ and chlorophyll contents will directly affect the chloroplast electron transport rate (ETR). Additionally, PQ is a co-factor of phytoene desaturase and ζ-carotene desaturase, during carotenoid synthesis (Sandmann et al., 2006), and decreased PQ content could therefore decrease cell carotenoid and xanthophyll contents [as β-carotene is known to be the precursor of the xanthophyll cycle (Bouvier et al., 1996)]. This would result in decreased thermal dissipation of excess energy, leading to ROS accumulation and oxidative bursts that would ultimately induce decreases in photosynthetic activity (Figure 1). Glyphosate was also proposed to affect photosynthesis by the overproduction of ROS in mitochondria due to its inhibitory effect on respiratory electron transport chain; once produced in mitochondria, ROS leave mitochondria, entering in chloroplast where they inducing oxidative damages to photosynthetic apparatus, decreasing photosynthesis (Gomes and Juneau, 2016).
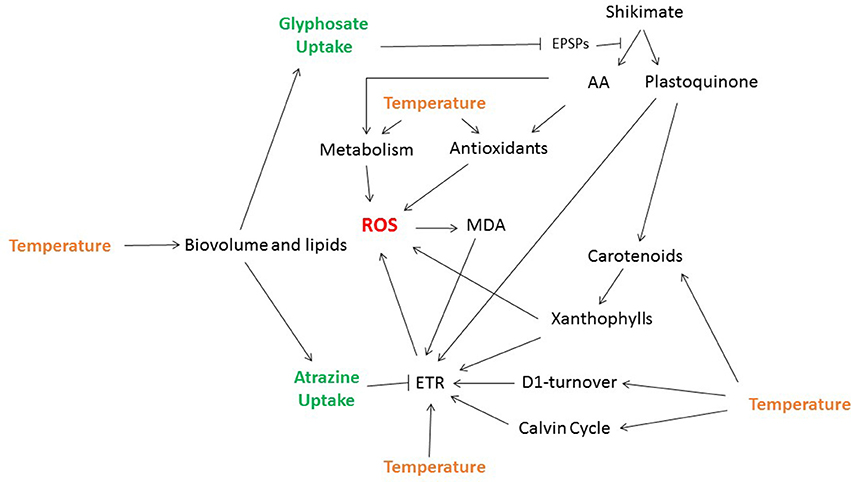
Figure 1. Effects of temperature on the toxic effects of glyphosate and atrazine on photosynthetic aquatic organisms. Both glyphosate and atrazine can induce reactive oxygen species (ROS) accumulation, which will increase lipid peroxidation (MDA) and lead to oxidative bursts and membrane destruction (thylakoids), thus contributing to decreases in photosynthetic rates (ETR). Temperature can modulate the deleterious effects of atrazine and glyphosate exposure by: (1) inducing changes in cell biovolumes and lipid composition, and therefore herbicide uptake; (2) modulating enzymatic activity, including antioxidant systems and cell respiratory metabolism (which are both related to ROS content), as well as D1-protein recovery and the Calvin Cycle (which are involved in ETR and photosynthetic rates); (3) modulating pigment contents, especially of carotenoids, which are linked to thermal dissipation capacity, ROS, and the synthesis of xanthophylls; and (4) modulating membrane fluidity and the diffusion of electron carriers (which are related to ETR).
As with other herbicides, glyphosate toxicity in the environment can be modulated by climatic conditions, especially by temperature (Pesce et al., 2009). However, studies on algae and cyanobacteria involving combined glyphosate and temperature effects are quite scarce. Pesce et al. (2009) demonstrated that glyphosate (10 μg l−1) effects on riverine microorganisms are seasonally dependent, with no difference in algal community genera distributions being observed between control and treated microcosms in the spring, although there were marked differences between the algal communities in the summer, with the disappearance of three algal genera (Asterionella, Cyclotella, and Oocystis) in glyphosate-treated microcosms. Although differences occurred in the species found between the two seasons, they noticed that glyphosate had no effect on chlorophyll a concentrations. While glyphosate did not affect total community biomass, it had inhibitory effects on the reproduction of Asterionella, Cyclotella, and Oocystis during the summer (Pesce et al., 2009), indicating that glyphosate-tolerant species became more representative in the community biomass. More recently, Baier et al. (2016) observed reduced algal diversity (Shannon- and Evenness-index) by glyphosate (1.5, 3, and 4 mg l−1) under increased temperatures. This suggests that responses of natural communities to glyphosate can vary with changing environmental conditions, and that temperature can modulate glyphosate toxicity in algae (Pesce et al., 2009).
As mentioned previously, glyphosate was proposed to impair respiration by inhibiting the mitochondrial electron transport chain, leading to direct ROS formation (Gomes and Juneau, 2016; Gomes et al., 2017a). As higher temperature stimulates respiration (Falkowski and Raven, 2013), decreasing metabolic rates under lower temperatures can lead to decreased ROS production, mainly through the slowing of respiratory pathways. It is important to note, however, that temperature might modulate the activity of antioxidant systems, which are directly related to the control of ROS amounts in cells. The decreased activity of enzymatic antioxidant systems under low temperatures can lead to a less efficient ROS scavenging system, leading to their accumulation and deleterious effects (Figure 1). In contrast, although the increase in respiration could lead to increased ROS formation in glyphosate-exposed cells (due to increased glyphosate uptake and respiration activity), the increased activity of antioxidant system under physiological higher temperatures could prevent increases in the oxidative status (Figures 1, 2).
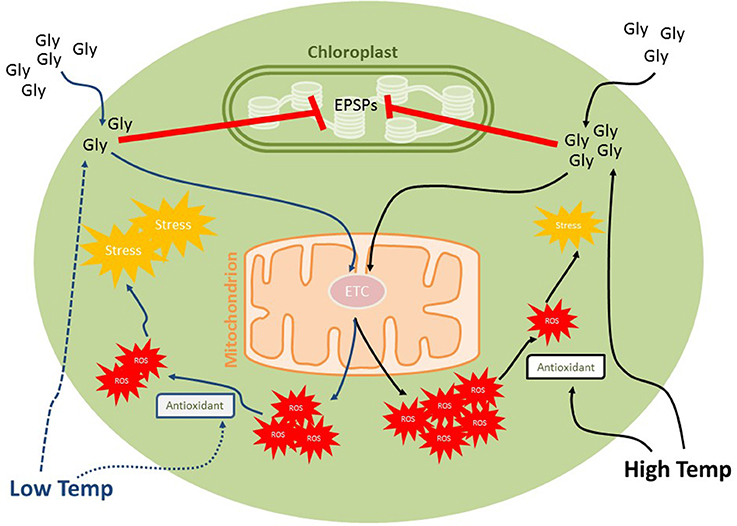
Figure 2. Effects of low temperature and high physiological temperatures on the toxic effects of glyphosate on phytoplankton. Glyphosate herbicidal effects are due the inhibition of 5-enolpyruvylshikimate-3-phosphate (EPSP) synthase activity in chloroplast but it is also known to block mitochondrial electron transport chain, inducing reactive oxygen species (ROS) formation. Low temperature decrease both glyphosate uptake (by modulating membrane permeability) and antioxidant activities, the contrast being expected under increased temperatures. Although increasing glyphosate uptake, respiration activity and ROS formation, higher temperatures can induce antioxidant activities, decreasing potential oxidative damages from ROS accumulation.
Herbicides such as atrazine (as well other photosynthesis-targeting herbicides) and glyphosate are known to induce oxidative stress in photosynthetic aquatic organisms (DeLorenzo et al., 2001; Romero et al., 2011). It was shown that different species have various susceptibility to oxidative stress and thermal acclimation ability of the antioxidant defenses (Choo et al., 2004; Lohrmann et al., 2004; Bouchard and Purdie, 2011). It is known that the cyanobacteria M. aeruginosa decreases its metabolic activity when temperature is increased to a level that is considered stressful (Jochem, 1999). This may explain the decreased oxidative stress (ROS cell−1) when M. aeruginosa cultures were transferred from 25 to 35°C (Bouchard and Purdie, 2011). In contrast, higher lipid peroxidation was observed at 15°C when compared to 26°C in the green algae Cladophora glomerata and Enteromorpha ahlneriana, which could be related to temperature modulation of their antioxidant enzymes since decreased ascorbate peroxidase (APX) activity was, in fact, observed when these algae were grown at 15°C (Choo et al., 2004). The modulation of antioxidant systems may therefore also be related to algal and cyanobacterial tolerance to herbicides under changing temperature regimes, although this important physiological process has not yet gained much attention, and inter-connected physiological evaluations of experimental results are missing. Xanthophylls, for example, is involved in the thermal dissipation of excess energy—while also contributing to ROS scavenging and increasing membrane stability by decreasing membrane susceptibility to lipid peroxidation (Havaux, 1998). In this context, pigment (carotenoid) content (as proposed by Millie et al., 1992), thermal dissipation (as proposed by Chalifour and Juneau, 2011), and oxidative stress could all be linked to the temperature modulation of herbicide toxicity in algae and cyanobacteria (Figure 2).
Temperature and herbicides could have both antagonistic and synergistic effects on photosynthetic aquatic organisms. Furthermore, temperature modulates uptake and metabolism of pollutants, which have important applied implications in respect to removal and fate of these pollutants in aquatic systems. Raising temperatures can significantly favor their removal by more tolerant algal and cyanobacterial species (which is of interest for reclaiming projects) but will drastically increase herbicide toxicity to sensitive species, leading to possible irreversible changes in photosynthetic aquatic community.
Light and Herbicides in Phytoplankton and Periphyton
General Concern
Phytoplankton success in aquatic environments is linked to their ability to convert light into biochemical energy that can be used in biomass production (Deblois et al., 2013). Photosynthesis is directly related to photon flux: at the light compensation point, CO2 input (carboxylation) is equal to CO2 release (respiration); above the light compensation point, increasing photon fluxes will increase photosynthetic rates in a linear relationship until reaching a saturating point at which factors other than incident light (i.e., the electron transport rate, Rubisco activity, or carbon metabolism) become limiting to photosynthesis. Therefore, photosynthetically active light can be a critical limiting resource for algal and cyanobacterial growth and reproduction.
Predictions of regional or global changes in light availabilities are currently difficult as (in addition to physical characteristics of water) light regimes will vary as a function of photosynthetic aquatic organism growth (Finkel et al., 2010). The new climatic scenario, however, is set to impose increasing light intensity and solar ultraviolet (UV) radiation on ecosystems due to ozone depletion (Caldwell et al., 2003) that can result in cellular damage and inhibitory effects on photosynthesis, at least in the upper water column (Finkel et al., 2010). Additionally, global warming will alter circulation patterns, water column stratification, and water layer mixing, resulting in algal and cyanobacterial exposure to high light and UV intensities (Finkel et al., 2010). In this context, predicted changes in light conditions will probably have an impact on the selection of species possessing strategies to cope with or avoid photoinhibition (Sunda et al., 2002).
Agricultural wastes increase water turbidity and decrease light availability for photosynthetic aquatic community (Tilman et al., 2001). Additionally, wind episodes may cause photosynthetic organisms to be exposed to strong light intensity variations during the day (Dubinsky and Stambler, 2009). In these conditions, algae and cyanobacteria may alter their cellular metabolism and invest more resources to produce UV absorbing compounds (to shield UV-sensitive cellular components) and to increase the metabolic repair processes of PSII after photoinactivation (Finkel et al., 2010; Falkowski and Raven, 2013). Photoacclimation processes can also alter algal and cyanobacterial cell sizes, as light-limiting environments often result in decreased cell sizes (which help to assure less internal light shading and thus higher light absorption efficiency), where under higher light conditions larger cells are observed (Finkel et al., 2010). Changes in cellular sizes can also be related to the uptake of pollutants by aquatic organisms, with decreased cell sizes being directly related to decreased contaminant uptake. Additionally, and at same time, once exposed to higher light conditions, small cells are more prone to photoinhibition (Falkowski and Raven, 2013)—making studies of the effects of water contaminants on algae and cyanobacteria under changing light environments essential.
Case of PSII Inhibitors and Light
Light stress targets the photosynthetic apparatus, as do some herbicides, and photoacclimation processes may therefore directly influence herbicide toxicity in aquatic photosynthetic organisms. The first studies examining the relationships between light and herbicides date from the 1980s (O'Neal and Lembi, 1983; Mayasich et al., 1986). O'Neal and Lembi (1983) observed that the effectiveness of the herbicide simazine (a triazine herbicide often used to control algal growth in USA and Europe) was influenced by light intensity. These authors found that the inhibitory effects of simazine (0.76 μg l−1) on C. glomerata growth (a filamentous algae) increased with increasing light intensity. Similar results were also reported by Millie et al. (1992), who observed that the deleterious effects of simazine (20, 40, 80, 160, and 320 μg l−1) on photosynthesis in A. circinalis became even greater in photoacclimated populations as photon flux density increased (50, 130, 230 μmol m−2 s−1). In this context, the algaecide efficiency of simazine should increase under higher light conditions but be more limited in shaded environments. Millie et al. (1992) demonstrated that increases in simazine-toxicity for high light photoacclimated A. circinalis were correlated with reductions in pigment contents (chlorophyll a, c-phycocianin, and carotenoids), leading the authors to suggest that algal sensitivity to PSII inhibitors is affected by alterations in their pigment contents. In their study of the interactive effects of light (0.208, 0.780, and 1.352 mW/cm2) and atrazine (also a triazine herbicide—0.05 and 0.10 μg l−1) on the marine algae Phaeodactylum tricornutum, Mayasich et al. (1986) observed that the inhibitory effects of this herbicide were greater at low light intensities. These authors hypothesized that cells pre-acclimated to higher light acquired protective mechanisms of sufficient capacity to dissipate atrazine-induced photo-oxidative stress by diverting the excitation energy from PSII to PSI and/or through quenching by carotenoid pigments. Although increases in accessory pigments have been observed in low-light photoacclimated cells (Guasch and Sabater, 1998), these authors did not quantify their pigment contents to confirm that hypothesis. It is important to note that other mechanisms besides pigment modulation (i.e., increased antioxidant system efficiency) could be involved in the influence of light on photosynthesis-inhibiting herbicides.
In their studies (using series of eight concentrations from 0 to 4.31 mg l−1) of changes in atrazine toxicity during succession in stream periphyton communities, Guasch et al. (1997) observed that communities photoacclimated to higher irradiance levels (150 as opposed to 50 μmol m−2 s−1) showed a lower photosynthetic EC50 (atrazine concentrations inhibiting 50% of the photosynthetic rate)—suggesting a relationship between light conditions during growth and atrazine toxicity in which aquatic photosynthetic organism life histories have a central role in their responses to atrazine. Guasch and Sabater (1998) confirmed this hypothesis in subsequent short-term concentration-response test studies, reporting that periphyton communities that colonized shaded sites showed higher percentages of accessory pigments and were less sensitive (higher photosynthetic EC50) to atrazine than communities from open sites (photoacclimated to higher light intensities), indicating that this herbicide was more toxic to high light-photoacclimated algae.
The importance of the light histories of phytoplankton to their responses to atrazine (from 0.005 to 2.156 mg l−1) was also tested by Deblois et al. (2013) who examined the combined effects of different light intensities and atrazine concentrations on 10 phytoplankton species (chlorophytes, baccilariophytes, and cyanophytes) adapted or not to low [LL – 76 μmol photons (PAR) m−2 s−1] or high [HL – 583 μmol photons (PAR) m−2 s−1] light intensities. Firstly, these authors observed that cyanophytes were more sensitive to atrazine, indicating a species-dependent response to the herbicide. Additionally, evaluations of photosynthetic parameters indicated a positive relationship between atrazine toxicity and increased irradiance for non-adapted phytoplankton and these authors argued that this relationship could be related to changes affecting electron transport rates due to rapid photoregulatory processes. The availability of light and electron transporters (such as quinones) were advance to be the limiting factors under LL and HL situations, respectively. Indeed, under LL condition and fixed atrazine concentration only a small fraction of the quinone pool needed for photosynthesis would therefore be blocked, attenuating the deleterious effects of the herbicide. In contrast, under HL conditions the entire quinone pool is needed for effective electron transport to avoid photoinhibition, and atrazine would therefore have a more deleterious effect by further limiting the electron transport (and increasing photoinhibitory effect). These authors also observed that for HL-adapted organisms atrazine has less effect than for the LL-adapted phytoplankton. Deblois et al. (2013) noted that HL-adaptation often increases the size of the electron transport pool (including quinones), increasing the quantity of target sites of atrazine and thus diluting its hazardous effects (Figure 3). The results of Deblois et al. (2013) on adapted algae and cyanobacteria, therefore, seem to contrast with those of Guasch and Sabater (1998). However, it is important to note that these authors were working with different levels of biological complexity: while Guasch and Sabater (1998) evaluated the atrazine responses of a periphyton community, Deblois et al. (2013) investigated herbicide effects at the level of individual phytoplankton species. Furthermore, when we take into account that the HL-acclimated (and high atrazine sensitive) community studied by Guasch and Sabater (1998) was predominantly composed of chlorophytes and cyanophytes, the results of Deblois et al. (2013) showing that cyanophytes were more sensitive to atrazine than other phytoplankton groups, are in accordance with the community level study.
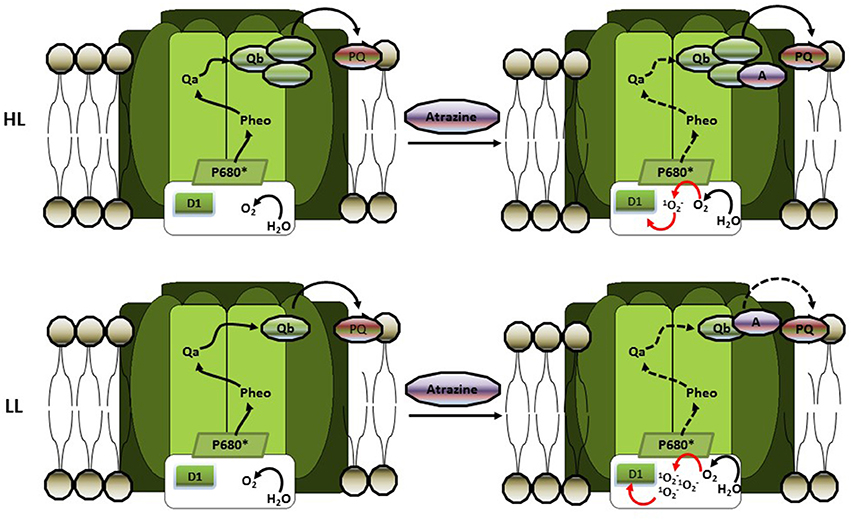
Figure 3. The influence of high light (HL) and low light (LL) acclimation on atrazine toxicity in phytoplankton (according to Deblois et al., 2013). HL-adaptation increases the quinone pool, thus increasing the number of atrazine targets. This dilution effect decreases reactive oxygen production (ROS) due to atrazine-induced photoinhibition, thus decreasing the hazardous effects of that herbicide. In LL adapted cells, on the other hand, the lack of sufficient quinone to maintain electron transport will drastically increase (ROS) production and oxidative damage.
Case of Lipid Synthesis Inhibitor and Light
Molinate (a thiocarbamate herbicide) is widely used in China in rice plantations in both pre- and post-emergence applications (Yan et al., 1997). Yan et al. (1997) evaluated the effects of molinate (5, 25, and 50 μg l−1) on Anabaena sphaerica, a filamentous nitrogen-fixing cyanobacterium, and reported that its toxicity was higher under high light conditions (3000 lux compared to 300 lux). Interestingly, molinate was observed to stimulate chlorophyll a biosynthesis in A. sphaerica, although the stimulatory effect was lower under lower light conditions (300 lux) than at 3000 lux. On the other hand, molinate interfered with A. sphaerica protein metabolism, inhibiting the biosynthesis of biliproteins that are important light-harvesting components in cyanobacteria, and molinate toxicity was greater under high light intensity conditions due to decreased organic carbon assimilation (which is known to chelate the herbicide to form an inactive complex, thus diminishing its toxicity).
Case of Glyphosate and Light
In spite of the environmental importance of glyphosate, to our knowledge, only one study examining light effects on its toxicity in photosynthetic aquatic organisms was published. In their study, Wood et al. (2016) examined the effects of changing light intensities (20 and 100 μmol m−2 s−1) on glyphosate (50, 200, and 500 μg l−1) toxicity to natural benthic communities collected from 4 different rivers in the Great Barrier Reef World Heritage Area (Australia). For the majority of the evaluated taxa (22 of the 26) and for the entire community, no significant interaction between light and herbicide effects at the community levels was found. It is important to note, however, that the response of diatoms to herbicide exposure at the community levels was dependent on prior exposure histories of the sites and can be influenced by intra-specific interactions between taxa (Wood et al., 2016).
Under a physiological point of view, interaction effects between glyphosate and light conditions can be expected since light modulates the quantities of electron transport components such as plastoquinones that are produced in the shikimate pathway—the target of the herbicide glyphosate. Shick et al. (1999) demonstrated that both UV-A and B radiation stimulate the shikimate pathway, and they evaluated the mycosporine-like amino acid (MAA) contents of the algal partners of colonies of Stylophorapistillata coral that are produced via the shikimate pathway (Bandaranayake, 1998). Exposure to UV was found to induce MAA accumulation, indicating that the shikimate pathway was stimulated by UV irradiation (Bandaranayake, 1998). In this context, increased environmental UV could result in either increased or decreased glyphosate toxicity: (1) by inhibiting EPSPs, as glyphosate induces unregulated carbon flux through shikimate pathway, thus disturbing carbon metabolism and several cellular metabolic pathways (Gomes et al., 2014). Stimulation of the shikimate pathway by UV exposure could therefore increase the negative impact of glyphosate on cell metabolism; (2) by stimulating the shikimate pathway, as previous exposure to UV can increase aromatic amino acid production (the first physiological glyphosate target) and plastoquinone (the secondary physiological glyphosate target) contents of the cells, decreasing the detrimental effects of glyphosate on protein biosynthesis and photosynthesis respectively (for more details see Figure 1). Since studies on the interactions between light and glyphosate are at their infancy, additional studies are definitively needed.
Changing light environments can act antagonistically or synergistically with herbicides effects on phytoplankton physiology. Although light-limiting conditions could decreased chemical uptake by reducing cell sizes, it may favor negative effect of photosynthetic-target herbicides in aquatic organisms (when compared to cells photoacclimated to higher light intensities) due to less effective protective mechanisms associated to pigment contents. In this context, we can expect that the increase in light intensities could favor the presence of algal and cyanobacterial species less sensitive to PSII inhibitors (such as atrazine) due to the presence of species having more efficient photoacclimation mechanisms.
Future Perspectives and Conclusions
Changes in temperature and light regimes exert profound effects on phytoplankton metabolic processes as well as on the environment. Therefore, the physiological responses of phytoplankton to aquatic pollutants would be expected to be modulated by environmental changes. Guidelines for the protection of aquatic life have largely been restricted until now to studies carried out at temperatures between 20 and 30°C (USEPA, 2004; MDDEP, 2008). New environmental pressures (mainly anthropological) are currently driving changes in environmental conditions, however, especially in terms of temperature and light conditions, studies directed toward the influence of environmental factors on aquatic pollutant toxicity are urgently needed. The use of herbicides has greatly increased over the last few decades, and herbicide contamination, especially of aquatic systems, is a growing concern. Most herbicides (such as atrazine) accumulate in aquatic ecosystems; others (such as glyphosate) are quickly degraded, although the toxicities of their by-products have not been extensively studied. The glyphosate by-product AMPA, for example, has been considered toxic to plants (Gomes et al., 2016), but no studies of its effects on phytoplankton have been undertaken yet. This presents a double blind spot in the literature: (1) our lack of knowledge concerning the toxicities of pollutants (including some herbicides and large numbers of herbicide by-products) and, (2) our lack of knowledge about how environmental changes can affect herbicide toxicities in aquatic ecosystems.
In attempting to address these questions, a number of authors have conducted studies examining the influences of temperature and light on herbicide toxicity in phytoplankton, but their results have not been consistent or conclusive. Firstly, the duration of phytoplankton acclimation processes have varied among the studies (from a few hours to several days) and no study has compared temperature or light effects on herbicide toxicity over short- vs. long-term timescales. Acclimation processes are of great importance to phytoplankton metabolism, and acclimation histories are central to understand phytoplanktonic responses to herbicides (Guasch and Sabater, 1998). The duration of phytoplankton acclimation can therefore influence their responses to waterborne contaminants, and this point must be considered when comparing new studies to established models. Secondly, studies have often only used the metrics of growth and photosynthesis to evaluate phytoplankton responses to herbicide exposure under changing environmental conditions. Despite their obvious importance, growth and photosynthesis are not the only physiological aspects that can or should be considered. Many herbicides can induce oxidative stress, although oxidative mechanisms (such as the activities of antioxidant systems, ROS accumulation, and lipid peroxidation) have not been widely used as toxicity markers. Oxidative events are central features in metabolism, as ROS can induce cell damage but also act as cellular signaling molecules (Gomes and Garcia, 2013). Investigations of these processes can help us to elucidate herbicide toxicity and identify the effects of herbicides on phytoplankton metabolism in an interconnected manner.
It is difficult to predict the effects of environmental changes on herbicide toxicity to phytoplankton as temperature and light can modulate phytoplankton responses to herbicides through acclimation processes. The changes induced at the metabolic machinery level by these climatic factors can either increase or decrease phytoplankton tolerance (even in a species-specific manner). These responses are highly specific and can vary between strains of the same species (Chalifour and Juneau, 2011). Temperature could possibly modulate the deleterious effects of herbicides such as atrazine and glyphosate by: (1) inducing changes in cell biovolumes and their lipid compositions, and therefore herbicide uptake; (2) modulating enzymatic activities, including those of antioxidant systems and of cell respiratory metabolism (which are both related to ROS content), as well as D1-protein recovery and the Calvin Cycle (which are involved in ETR and photosynthetic rates); (3) modulating the pigment contents of the cells, especially carotenoids, which are linked to thermal energy dissipation capacity, ROS scavenging, and synthesis of xanthophylls; and (4) modulating membrane fluidity and the diffusion of electron carriers (which are related to ETR). On the other hand, light appears to influence algal and cyanobacterial responses to herbicides (at least those that target photosynthesis) by modulating the numbers of electron transporters (such as quinones) and by increasing or decreasing the concentrations of herbicide binding sites (Deblois et al., 2013). By modulating metabolic changes (acclimation), both temperature and light can drive herbicide tolerance and will be important factors in selecting species able to survive to herbicide contamination. In this context, total primary production may not be affected by the presence of herbicides in aquatic environments although phytoplankton biodiversity will certainly be altered (as observed by Pesce et al., 2009). In addition to reducing biodiversity, these interactions can induce eutrophication processes by stimulating herbicide mineralization, for example, or by increasing the nutrient contents of waterways (Stachowski-Haberkorn et al., 2008).
Among the studied environmental factors, temperature seems to be the most important in driving phytoplankton responses to herbicide contamination. Considering the present literature, we can advise that the season of herbicide application might influence the phytoplankton community in the following sense: at low temperatures, herbicide application may reduce cyanobacterial community, since these species are quite sensitive to low temperature (Butterwick et al., 2004). On the other hand, at warmer temperatures, cyanobacteria may have higher tolerance to the hazardous effects of herbicides, and their growth may be favored compared to other groups of algae and cyanobacteria. Since many cyanobacteria can produce harmful toxins, their dominance induce, on top of herbicide presence, another environmental problem. In this context, the use of herbicide during the summer (or in equatorial and tropical regions) might represent an additional ecological constrain, as it can induce hazardous cyanobacterial blooms. In turns, light conditions appear to be more linked to herbicide efficiency, as found for the algaecide efficiency of simazine (Millie et al., 1992). However, it is important to consider that ozone depletion will induce increased UV radiation, which influences phytoplankton performance. However, few studies have been conducted regarding the interaction between UV and herbicides.
As an overview, the published literature emphasizes the importance of light regimes and temperatures on herbicide toxicity to algae and cyanobacteria—although it is difficult today to establish species-specific models of how climate change will affect phytoplanktonic and periphytonic responses to herbicides. Acclimation processes will affect the selection of algal and cyanobacterial species able to survive under conditions of herbicide contamination, assuring sustained primary production, although this process will necessarily decrease biodiversity. More investigations are needed to determine how these interactions will influence the success of organisms of different taxa, and it will be important to include environmental changes as variables in any new guidelines proposed for the protection of aquatic life forms. Finally, guidelines should be re-evaluated based on field studies, instead of being developed based on only in situ evaluations.
Author Contributions
All authors listed have made a substantial, direct and intellectual contribution to the work, and approved it for publication.
Funding
This research was supported by the Natural Science and Engineering Research Council of Canada (NSERC). MPG received a Ph.D. scholarship from Fond de Recherche du Quebec–Nature et Technologies (FRQNT).
Conflict of Interest Statement
The authors declare that the research was conducted in the absence of any commercial or financial relationships that could be construed as a potential conflict of interest.
References
Arunakumara, K. K. I. U., Walpola, B. C., and Yoon, M. (2013). Metabolism and degradation of glyphosate in aquatic cyanobacteria : a review. Afr. J. Microbiol. Res. 7, 4084–4090. doi: 10.5897/AJMR12.2302
Atkin, O. K., and Tjoelker, M. G. (2003). Thermal acclimation and the dynamic response of plant respiration to temperature. Trends Plant Sci. 8, 343–351. doi: 10.1016/S1360-1385(03)00136-5
Baier, F., Gruber, E., Hein, T., Bondar-kunze, E., Ivankovic, M., and Mentler, A. (2016). Non-target effects of a glyphosate-based herbicide on Common Toad larvae (Bufo bufo, Amphibia) and associated algae are altered by temperature. PeerJ 4:e2641. doi: 10.7717/peerj.2641
Bandaranayake, W. M. (1998). Mycosporines: Are they nature's sunscreens? Nat. Prod. Rep. 15, 159–172. doi: 10.1039/a815159y
Barber, J., Ford, R. C., Mitchell, R. A. C., and Millner, P. A. (1984). Chloroplast thylakoid membrane fluidity and its sensitivity to temperature. Planta 161, 375–380. doi: 10.1007/BF00398729
Baxter, L., Brain, R. A., Lissemore, L., Solomon, K. R., Hanson, M. L., and Prosser, R. S. (2016). Influence of light, nutrients, and temperature on the toxicity of atrazine to the algal species Raphidocelis subcapitata: implications for the risk assessment of herbicides. Ecotoxicol. Environ. Saf. 132, 250–259. doi: 10.1016/j.ecoenv.2016.06.022
Behrenfeld, M. J., Prasil, O., Babin, M., and Bruyant, F. (2004). In search of a physiological basis for covariations in light-limited and light saturated photosynthesis. J. Phycol. 40, 4–25. doi: 10.1046/j.1529-8817.2004.03083.x
Bérard, A., and Benninghoff, C. (2001). Pollution-induced community tolerance (PICT) and seasonal variations in the sensitivity of phytoplankton to atrazine in nanocosms. Chemosphere 45, 427–437. doi: 10.1016/S0045-6535(01)00063-7
Bérard, A., Leboulanger, C., and Pelte, T. (1999). Tolerance of oscillatoria limnetica lemmermann to atrazine in natural phytoplankton populations and in pure culture : influence of season and temperature. Arch. Environ. Contam. Toxicol. 479, 472–479. doi: 10.1007/s002449900541
Bicalho, E. M., Gomes, M. P., Rodrigues, A. G. Jr., Oliveira, T. G. S., de Almeida Gonçalves, C., Fonseca, M. B., et al. (2017). Integrative effects of zinc and temperature on germination in Dimorphandra wilsonii rizz.: implications of climate changes. Environ. Toxicol. Chem. 9999, 1–7. doi: 10.1002/etc.3729
Borggaard, O. K., and Gimsing, A. L. (2008). Fate of glyphosate in soil and the possibility of leaching to ground and surface waters: a review. Pest Manag. Sci. 456, 441–456. doi: 10.1002/ps.1512
Bouchard, J. N., and Purdie, D. A. (2011). Effect of elevated temperature, darkness, and hydrogen peroxide treatment on oxidative stress and cell death in the bloom-forming toxic cyanobacterium Microcystis aeruginosa 1. J. Phycol. 47, 1316–1325. doi: 10.1111/j.1529-8817.2011.01074.x
Boussiba, S. (2000). Carotenogenesis in the green alga Haematococcus pluvialis: cellular physiology and stress response. Physiol. Plant. 108, 111–117. doi: 10.1034/j.1399-3054.2000.108002111.x
Bouvier, F., D'Harlingues, A., Hugueney, P., Marin, E., Marion-Poll, A., and Camara, B. (1996). Xanthophyll biosynthesis. Cloning, expression, functional reconstitution, and regulation of beta -cyclohexenyl carotenoid epoxidase from pepper (Capsicum annuum). J. Biol. Chem. 271, 28861–28867.
Butterwick, C., Heaney, S. I., and Talling, J. F. (2004). Diversity in the influence of temperature on the growth rates of freshwater algae, and its ecological relevance. Freshw. Biol. 50, 291–300. doi: 10.1111/j.1365-2427.2004.01317.x
Caldwell, M. M., Ballaré, C. L., Bornman, J. F., Flint, S. D., Bjorn, L. O., Teramura, A. H., et al. (2003). Terrestrial ecosystems, increased solar ultraviolet radiation and interactions with other climatic change factors. Photochem. Photobiol. Sci. 2, 252–266. doi: 10.1039/B211159B
Carafa, R., Wollgast, J., Canuti, E., Ligthart, J., Dueri, S., Hanke, G., et al. (2007). Seasonal variations of selected herbicides and related metabolites in water, sediment, seaweed, and clams in the Sacca di Gorocoastal lagoon (Northern Adriatic). Chemosphere 69, 1625–1637. doi: 10.1016/j.chemosphere.2007.05.060
Chalifour, A., Arts, M. T., Kainz, M. J., and Juneau, P. (2014). Combined effect of temperature and bleaching herbicides on photosynthesis, pigment and fatty acid composition of Chlamydomonas reinhardtii. Eur. J. Phycol. 49, 508–515. doi: 10.1080/09670262.2014.977962
Chalifour, A., and Juneau, P. (2011). Temperature-dependent sensitivity of growth and photosynthesis of Scenedesmus obliquus, Navicula pelliculosa and two strains of Microcystis aeruginosa to the herbicide atrazine. Aquat. Toxicol. 103, 9–17. doi: 10.1016/j.aquatox.2011.01.016
Chen, C. Y., Hathaway, K. M., Thompson, D. G., and Folt, C. L. (2008). Multiple stressor effects of herbicide, pH, and food on wetland zooplankton and a larval amphibian. Ecotoxicol. Environ. Saf. 71, 209–218. doi: 10.1016/j.ecoenv.2007.08.007
Chen, M., Li, J., Dai, X., Sun, Y., and Chen, F. (2010). Effect of phosphorus and temperature on chlorophyll a contents and cell sizes of Scenedesmus obliquus and Microcystis aeruginosa. Limnology 12, 187–192. doi: 10.1007/s10201-010-0336-y
Choo, K., Snoeijs, P., and Pedersén, M. (2004). Oxidative stress tolerance in the filamentous green algae Cladophora glomerata and Enteromorpha ahlneriana. J. Exp. Mar. Bio. Ecol. 298, 111–123. doi: 10.1016/j.jembe.2003.08.007
Cobb, A. H., and Reade, J. P. (2010). “The inhibition of amino acid byosynthesis,” in Herbicides and Plant Physiology, eds A. H. Cobb and J. P. Reade (Wiley-Blackwell), 176–197.
Coles, J. F., and Jones, R. C. (2000). Effect of temperature on photosynthesis-light response and growth of four phytoplankton species isolated from a tidal freshwater river. J. Phycol. 16, 7–16. doi: 10.1046/j.1529-8817.2000.98219.x
Comber, S. D. W. (1999). Abiotic persistence of atrazine and simazine in water. Pestic. Sci. 55, 696–702.
Corbett, J. R., Wright, K., and Baillie, A. C. (1984). The Biochemical Mode of Action of Pesticides. London: Academic.
Costanzoi, S. D., and Dennison, W. C. (2003). Assessing the seasonal influence of sewage and agricultural nutrient inputs in a subtropical river estuary. Estuaries 26, 857–865. doi: 10.1007/BF02803344
Coupe, R., Kalkhoff, S., Capel, P., and Gregoire, C. (2012). Factors affecting the fate and transport of glyphosate and AMPA into surface waters of agricultural watersheds in the United States and Europe. Geophys. Res. Abstr. 14:5877.
Crain, C. M., Kroeker, K., and Halpern, B. S. (2008). Interactive and cumulative effects of multiple human stressors in marine systems. Ecol. Lett. 11, 1304–1315. doi: 10.1111/j.1461-0248.2008.01253.x
Croll, B. (1991). Pesticides in surface and underground waters. Water Environ. J. 5, 389–395. doi: 10.1111/j.1747-6593.1991.tb00635.x
Cuypers, A., Vangronsveld, J., and Clijsters, H. (2001). The redox status of plant cells (AsA and GSH) is sensitive to zinc imposed oxidative stress in roots and primary leaves of Phaseolus vulgaris. Plant Physiol. Biochem. 39, 657–664. doi: 10.1016/S0981-9428(01)01276-1
Daniel, R. M., Dines, M., and Peatach, H. H. (1996). The denaturation and degradation of stable enzymes at high temperatures. Biochem. J. 317, 1–11. doi: 10.1042/bj3170001
Davison, I. R. (1991). Environmental effects on algal photosynthesis: temperature. J. Phycol. 27, 2–8. doi: 10.1111/j.0022-3646.1991.00002.x
Debenest, T., Silvestre, J., Coste, M., and Pinelli, E. (2010). Effects of pesticides on freshwater diatoms. Rev. Environ. Contam. Toxicol. 203, 87–103. doi: 10.1007/978-1-4419-1352-4_2
Deblois, C. P., Dufresne, K., and Juneau, P. (2013). Response to variable light intensity in photoacclimated algae and cyanobacteria exposed to atrazine. Aquat. Toxicol. 126, 77–84. doi: 10.1016/j.aquatox.2012.09.005
DeLorenzo, M. E., Cott, G. I., and Ross, P. (2001). Toxicity of pesticides to aquatic microorganisms: a review. Environ. Toxicol. Chem. 20, 84–98. doi: 10.1002/etc.5620200108
Deutsch, C. A., Tewksbury, J. J., Huey, R. B., Sheldon, K. S., Ghalambor, C. K., Haak, D. C., et al. (2008). Impacts of climate warming on terrestrial ectotherms across latitude Thermal Safety margin. Proc. Natl. Acad. Sci. U.S.A. 105, 6668–6672. doi: 10.1073/pnas.0709472105
Diana, S. G., Resetarits, W. J., Schaeffer, D. J., Beckmen, K. B., and Beasley, V. R. (2000). Effects of atrazine on amphibian growth and survival in artificial aquatic communities. Environ. Toxicol. Chem. 19, 2961–2967. doi: 10.1002/etc.5620191217
Dubinsky, Z., and Stambler, N. (2009). Photoacclimation processes in phytoplankton: mechanisms, consequences, and applications. Aquat. Microb. Ecol. 56, 163–176. doi: 10.3354/ame01345
Elliott, J. A. (2010). The seasonal sensitivity of Cyanobacteria and other phytoplankton to changes in flushing rate and water temperature. Glob. Chang. Biol. 16, 864–876. doi: 10.1111/j.1365-2486.2009.01998.x
Falkowski, P. G., and Raven, J. A. (2013). Aquatic Photosynthesis. 2nd Edn., Princeton, NJ: Princeton University Press.
Finkel, Z. V., Beardall, J., Flynn, K. J., Quigg, A., Rees, T. A. V., and Raven, J. A. (2010). Phytoplankton in a changing world: cell size and elemental stoichiometry. J. Plankton Res. 32, 119–137. doi: 10.1093/plankt/fbp098
Fiori, E., and Pistocchi, R. (2014). Skeletonema marinoi (Bacillariophyceae) sensitivity to herbicides and effects of temperature increase on cellular responses to terbuthylazine exposure. Aquat. Toxicol. 147, 112–120. doi: 10.1016/j.aquatox.2013.12.014
Folt, C. L., Chen, C. Y., Moore, M., and Burnaford, J. (1999). Synergism and antagonism among multiple stressors. Limnol. Oceanogr. 44, 854–877. doi: 10.4319/lo.1999.44.3_part_2.0864
Gilmore, A. M. (1997). Mechanistic aspects of xanthophyll cycle-dependent photoprotection in higher plant chloroplasts and leaves. Physiol. Plant. 99, 197–209. doi: 10.1111/j.1399-3054.1997.tb03449.x
Giroux, I. (2015). Présence de Pesticides dans l'eau au Québec : Portrait et Tendances Dans les Zones de maïs et de soya – 2011 à 2014, Ministère du Développement Durable, de l'Environnement et de la Lutte contre les changements climatiques.
Gomes, M. P., Cruz, F. V. S., Bicalho, E. M., Borges, F. V., Fonseca, M. B., Juneau, P., et al. (2017a). Effects of glyphosate acid and the glyphosate-commercial formulation (Roundup) on Dimorphandra wilsonii seed germination: interference of seed respiratory metabolism. Environ. Pollut. 220, 452–459. doi: 10.1016/j.crvi.2005.08.004
Gomes, M. P., and Garcia, Q. S. (2013). Reactive oxygen species and seed germination. Biologia 68, 351–357. doi: 10.2478/s11756-013-0161-y
Gomes, M. P., and Juneau, P. (2016). Oxidative stress in duckweed (Lemna minor L.) induced by glyphosate: is the mitochondrial electron transport chain a target of this herbicide? Environ. Pollut. 218, 402–409. doi: 10.1016/j.envpol.2016.07.019
Gomes, M. P., Le Manac'h, S. G., Hénault-Éthier, L., Labrecque, M., Lucotte, M., and Juneau, P. (2017b). Glyphosate-dependent inhibition of photosynthesis in willow. Front. Plant Sci. 8:207. doi: 10.3389/fpls.2017.00207
Gomes, M. P., Le Manac'h, S. G., Maccario, S., Labrecque, M., Lucotte, M., and Juneau, P. (2016). Differential effects of glyphosate and aminomethylphosphonic acid (AMPA) on photosynthesis and chlorophyll metabolism in willow plants. Pestic. Biochem. Physiol. 130, 65–70. doi: 10.1016/j.pestbp.2015.11.010
Gomes, M. P., Smedbol, E., Chalifour, A., Hénault-Ethier, L., Labrecque, M., Lepage, L., et al. (2014). Alteration of plant physiology by glyphosate and its by-product aminomethylphosphonic acid (AMPA), an overview. J. Exp. Bot. 65, 4691–4703. doi: 10.1093/jxb/eru269
Gonzáles-Barreiro, Ó., Rioboo, C., Cid, A., and Herrero, C. (2004). Atrazine-induces chlorosis in Syechococcus elongatus cells. Arch. Environ. Contam. Toxicol. 46, 301–307. doi: 10.1007/s00244-003-2149-z
Graymore, M., Stagnitti, F., and Allinson, G. (2001). Impacts of atrazine in aquatic ecosystems. Environ. Int. 26, 483–495. doi: 10.1016/S0160-4120(01)00031-9
Guasch, H., Mu, I., and Sabater, S. (1997). Changes in atrazine toxicity throughout succession of stream periphyton communities. J. Appl. Phycol. 9, 137–146. doi: 10.1023/A:1007970211549
Guasch, H., and Sabater, S. (1998). Light history influences the sensitivity to atrazine in periphytic algae. J. Phycol. 241, 233–241. doi: 10.1046/j.1529-8817.1998.340233.x
Haidekker, A., and Hering, D. (2008). Relationship between benthic insects (Ephemeroptera, Plecoptera, Coleoptera, Trichoptera) and temperature in small and medium-sized streams in Germany: a multivariate study. Aquat. Ecol. 42, 463–481. doi: 10.1007/s10452-007-9097-z
Hao, Y. B., Liu, H. L., Ci, X. K., Dong, S. T., Zhang, J. W., and Liu, P. (2010). Effects of arsenic on maize growth, antioxidant system, and ion distribution. J. Appl. Ecol. 21, 3183–3190.
Havaux, M. (1998). Carotenoids as membrane stabilizers in chloroplasts. Trends Plant Sci. 3, 147–151. doi: 10.1016/S1360-1385(98)01200-X
Herzig, R., and Dubinsky, Z. (1992). Photoacclimation, photosynthesis, and growth in phytoplankton. Isr. J. Bot. 41, 199–212.
Hölzl, G., and Dörmann, P. (2007). Structure and function of glycoglycerolipids in plants and bacteria. Prog. Lipid Res. 46, 225–243. doi: 10.1016/j.plipres.2007.05.001
Horth, H., and Blackmore, K. (2009). Survey of Glyphosate and AMPA in Groundwaters and Surface Waters in Europe. Final Report. WRc Ref: UC8073.02. Monsanto, Wiltshire, UK.
Huner, N. A., Öquist, G., and Melis, A. (2003). “Photostasis in plants, green algae and cyanobacteria: The role of light harvesting antenna complexes,” in Light-Harvesting Antennas in Photosynthesis SE - 14 Advances in Photosynthesis and Respiration., eds B. Green and W. Parson (Dordrecht: Springer), 401–421.
Jochem, F. J. (1999). Dark survival strategies in marine phytoplankton assessed by cytometric measurement of metabolic activity with fluorescein diacetate. Mar. Biol. 135, 721–728. doi: 10.1007/s002270050673
Juneau, P., Barnett, A., Méléder, V., Dupuy, C., and Lavaud, J. (2015). Combined effect of high light and high salinity on the regulation of photosynthesis in three diatom species belonging to the main growth forms of intertidal flat inhabiting microphytobenthos. J. Exp. Mar. Bio. Ecol. 463, 95–104. doi: 10.1016/j.jembe.2014.11.003
Juneau, P., Qiu, B., and Deblois, C. P. (2007). Use of chlorophyll fluorescence as a tool for determination of herbicide toxic effect: review. Toxicol. Environ. Chem. 89, 609–625. doi: 10.1080/02772240701561569
Jursinic, P., and Stemler, A. (1983). Changes in [C]atrazine binding associated with the oxidation-reduction state of the secondary quinone acceptor of photosystem II. Plant Physiol. 73, 703–8. doi: 10.1104/pp.73.3.703
Kirilovsky, D. (2007). Photoprotection in cyanobacteria: the orange carotenoid protein (OCP)-related non-photochemical-quenching mecanism. Photosynth. Res. 93, 7–16. doi: 10.1007/s11120-007-9168-y
Knutti, R., Rogelj, J., Sedlacek, J., and Fischer, E. M. (2016). A scientific critique of the two-degree climate change target. Nat. Geosci 9, 13–18. doi: 10.1038/ngeo2595
Kolpin, D. W., Thurman, E. M., Lee, E. A., Meyer, M. T., Furlong, E. T., and Glassmeyer, S. T. (2006). Urban contributions of glyphosate and its degradate AMPA to streams in the United States. Sci. Total Environ. 354, 191–197. doi: 10.1016/j.scitotenv.2005.01.028
Larras, F., Lambert, A.-S., Pesce, S., Rimet, F., Bouchez, A., and Montuelle, B. (2013). The effect of temperature and a herbicide mixture on freshwater periphytic algae. Ecotoxicol. Environ. Saf. 98, 162–170. doi: 10.1016/j.ecoenv.2013.09.007
Legrand, M. F., Costentin, E., and Bruchet, A. (1991). Occurrence of 38 pesticides in various french surface and ground waters. Environ. Technol. 12, 985–996. doi: 10.1080/09593339109385097
Li, W.-X., Chen, T.-B., Huang, Z.-C., Lei, M., and Liao, X.-Y. (2006). Effect of arsenic on chloroplast ultrastructure and calcium distribution in arsenic hyperaccumulator Pteris vittata L. Chemosphere 62, 803–809. doi: 10.1016/j.chemosphere.2005.04.055
Lohrmann, N. L., Logan, B. A., and Johnson, A. S. (2004). Seasonal acclimatization of antioxidants and photosynthesis in Chondrus crispus and Mastocarpus stellatus, two co-occurring red algae with differing stress tolerances. Biol. Bull. 207, 225–232. doi: 10.2307/1543211
Los, D. A., and Murata, N. (2004). Membrane fluidity and its roles in the perception of environmental signals. Biochim. Biophys. Acta Biomembr. 1666, 142–157. doi: 10.1016/j.bbamem.2004.08.002
MacIntyre, H. L., Kana, T. M., Anning, T., and Geider, R. J. (2002). Review: Photoacclimation of photosynthesis irradiance response curves and photosynthetic pigments in microalgae and cyanobacteria. J. Phycol. 38, 17–38. doi: 10.1046/j.1529-8817.2002.00094.x
Mauzerall, D., and Greenbaum, N. L. (1989). The absolute size of a photosynthetic unit. Biochim. Biophys. Acta 974, 119–140. doi: 10.1016/S0005-2728(89)80365-2
Maxwell, D. P., Falk, S., and Huner, N. (1995). Growth at low temperature mimics high-light acclimation in Chlorella vulgaris. Plant Physiol. 107, 687–684. doi: 10.1104/pp.107.3.687
Mayasich, J. M., Karlander, E., and Terlizzi, J. (1986). Growth responses of Nannochloris oculata Droop and Phaeodactylum tricornutum Bohlin to the herbicide atrazine as influenced by light and temperature. Aquat. Toxicol. 8, 175–184. doi: 10.1016/0166-445X(86)90063-9
McGinty, E., Pieczonka, J., and Mydlarz, L. (2012). Variations in reactive oxygen release and antioxidant activity in multiple symbiodinium types in response to elevated temperature. Microb. Ecol. 64, 1000–1007. doi: 10.1007/s00248-012-0085-z
MDDEP (2008). Résultats de Cyanobactéries et Cyanotoxines à Sept Stations de Production d'eau Potable (2004–2006). Quebec Government, Ministère du Développement durable, Environnement et Parcs.
Millie, D. F., Hersh, C. M., and Dionigi, C. P. (1992). Simazine-induced inhibition in photoaclimated populations of Anabaena circinlis (cyanophyta). J. Phycol. 28, 19–26. doi: 10.1111/j.0022-3646.1992.00019.x
Morgan-Kiss, R., and Dolhi, J. (2012). “Microorganisms and plants: a photosynthetic perspective,” in Temperature Adaptation in a Changing Climate: Nature at Risk, eds K. Storey and K. Tanino (Cambridge: CABI), 24–44.
Necchi, O. Jr. (2004). Photosynthetic responses to temperature in tropical lotic macroalgae. Phycol. Res. 52, 140–148. doi: 10.1111/j.1440-1835.2004.tb00322.x
O'Neal, S. W., and Lembi, C. A. (1983). Effect of simazine on photosynthesis and growth of filamentous algae. Weed Sci. 31, 899–903.
Pesce, S., Batisson, I., Bardot, C., Fajon, C., Portelli, C., Montuelle, B., et al. (2009). Response of spring and summer riverine microbial communities following glyphosate exposure. Ecotoxicol. Environ. Saf. 72, 1905–1912. doi: 10.1016/j.ecoenv.2009.07.004
Pesce, S., Fajon, C., Bardot, C., Bonnemoy, F., Portelli, C., and Bohatier, J. (2008). Longitudinal changes in microbial planktonic communities of a French river in relation to pesticide and nutrient inputs. Aquat. Toxicol. 86, 352–360. doi: 10.1016/j.aquatox.2007.11.016
Pimentel, C., Bernacchi, C., and Long, S. (2007). Limitations to photosynthesis at different temperatures in the leaves of Citrus limon. Brazil. J. Plant Physiol. 19, 141–147. doi: 10.1590/S1677-04202007000200006
Qiu, H., Geng, J., Ren, H., Xia, X., Wang, X., and Yu, Y. (2013). Physiological and biochemical responses of Microcystis aeruginosa to glyphosate and its Roundup® formulation. J. Hazard. Mater. 248–249, 172–176. doi: 10.1016/j.jhazmat.2012.12.033
Quednow, K., and Püttmann, W. (2007). Monitoring terbutryn pollution in small rivers of Hesse, Germany. J. Environ. Monit. 9, 1337–1343. doi: 10.1039/b711854f
Raven, J. A., and Geider, R. J. (1988). Temperature and algal growth. New Phytol. 110, 441–461. doi: 10.1111/j.1469-8137.1988.tb00282.x
Rebich, R. A., Coupe, R. H., and Thurman, E. M. (2004). Herbicide concentrations in the Mississippi River Basin—the importance of chloroacetanilide herbicide degradates. Sci. Total Environ. 321, 189–199. doi: 10.1016/j.scitotenv.2003.09.006
Reddy, K. N., Rimando, A. M., and Duke, S. O. (2004). Aminomethylphosphonic acid, a metabolite of glyphosate, causes injury in glyphosate-treated, glyphosate-resistant soybean. J. Agric. Food Chem. 52, 5139–5143. doi: 10.1021/jf049605v
Reupert, R., and Ploeger, E. (1989). Determination of N-herbicides in groundwater, drinking-water and surface water: analytical method and results. Vom Wasser 72, 211–233.
Rhee, G. Y., and Gothan, I. J. (1981). The effects of environmental factors on phytoplankton growth: temperature and interactions of temperature with nutrient limitation. Limnol. Oceanogr. 26, 635–648. doi: 10.4319/lo.1981.26.4.0635
Romero, D. M., Ríos de Molina, M. C., and Juárez, A. B. (2011). Oxidative stress induced by a commercial glyphosate formulation in a tolerant strain of Chlorella kessleri. Ecotoxicol. Environ. Saf. 74, 741–747. doi: 10.1016/j.ecoenv.2010.10.034
Ross, J. C., and Vincent, W. F. (1988). Temperature dependence of UV radiation effects on Antartic cyanobacteria. J. Phycol. 34, 118–125. doi: 10.1046/j.1529-8817.1998.340118.x
Sandmann, G., Römer, S., and Fraser, P. D. (2006). Understanding carotenoid metabolism as a necessity for genetic engineering of crop plants. Metab. Eng. 8, 291–302. doi: 10.1016/j.ymben.2006.01.005
Sarcina, M., Tobin, M. J., and Mullineaux, C. W. (2001). Diffusion of phycobilisomes on the thylakoid membranes of the cyanobacterium Synechococcus 7942. J. Biol. Chem. 276, 46830–46834. doi: 10.1074/jbc.M107111200
Shick, J. M., Romaine-Lioud, S., Ferrier-Pagés, C., and Gattuso, J.-P. (1999). Ultraviolet-B radiation stimulates shikimate pathway-dependent accumulation of mycosporine-like amino acids in the coral Stylophora pistillata despite decreases in its population of symbiotic dinoflagellates. Limnol. Oceanogr. 44, 1667–1682. doi: 10.4319/lo.1999.44.7.1667
Siehl, D. (1997). “Inhibitors of EPSPS synthase, glutamine synthetase and histidine synthesis,” in Herbicide Activity: Toxicology, Biochemistry and Molecular Biology, eds R. Roe, J. Burton, and R. Kuhr (Amsterdam: IOS Press), 37–67.
Sikka, H. C., and Pramer, D. (1968). Physiological effects of fluometuron on some unicellular algae. Weed Sci. 16, 296–299.
Smith, R., and Mobley, C. (2008). “Underwater light,” in Photobiology SE - 7, ed L. Björn (New York, NY: Springer), 131–138.
Sobrino, C., and Neale, P. J. (2007). Short-Term and Long-Term Effects of Temperature on Photosynthesis in the Diatom Thalassiosira Pseudonana Under Uvr Exposures. J. Phycol. 43, 426–436. doi: 10.1111/j.1529-8817.2007.00344.x
Sonoike, K., Hihara, Y., and Ikeuchi, M. (2001). Physiological significance of the regulation of photosystem stoichiometry upon high light acclimation of Synechocystis sp. PCC 6803. Plant Cell Physiol. 42, 379–384. doi: 10.1093/pcp/pce046
Stachowski-Haberkorn, S., Becker, B., Marie, D., Haberkorn, H., Coroller, L., and de la Broise, D. (2008). Impact of Roundup on the marine microbial community, as shown by an in situ microcosm experiment. Aquat. Toxicol. 89, 232–241. doi: 10.1016/j.aquatox.2008.07.004
Staehr, P. A., and Birkeland, M. (2006). Temperature acclimation of growth photosynthesis and respiration in two mesophilic phytoplankton species. Phycologia 45, 648–656. doi: 10.2216/06-04.1
Staehr, P. A., and Sand-Jensen, K. A. J. (2006). Seasonal changes in temperature and nutrient control of photosynthesis, respiration and growth of natural phytoplankton communities. Freshw. Biol. 51, 249–262. doi: 10.1111/j.1365-2427.2005.01490.x
Steinheimer, T. R. (1993). HPLC determination of atrazine and principal degradates in agricultural soils and associated surface and ground water. J. Agric. Food Chem. 41, 588–595. doi: 10.1021/jf00028a016
Sullivan, D. J., Vecchia, A. V., Lorenz, D. L., Gillom, R. J., and Martim, J. D. (2009). Trends in Pesticide Concentrations in Corn-Belt Streams, 1996–2006. Reston, VA: National Water-Quality Assessment Program. Scientific Investigations Report 2009-5132. U.S. Department of the Interior and U.S. Geological Survey.
Sunda, W. G., Kieber, D. J., and Kiene, R. P. (2002). An antioxidant function for DMSP and DMS in marine algae. Nature 418, 317–320. doi: 10.1038/nature00851
Suzuki, N., and Mittler, R. (2006). Reactive oxygen species and temperature stresses: a delicate balance between signaling and destruction. Physiol. Plant. 126, 45–51. doi: 10.1111/j.0031-9317.2005.00582.x
Tang, J., Hoagland, K. D., and Siegfried, B. (1998). Uptake and bioconcentration of atrazine by selected freshwater algae. Environ. Toxicol. Chem. 17, 1085–1090. doi: 10.1002/etc.5620170614
Tasmin, R., Shimasaki, Y., Qiu, X., Honda, M., Tsuyama, M., Yamada, N., et al. (2014a). Elevated temperatures and low nutrients decrease the toxicity of diuron for growth of the green alga Pseudokirchneriella subcapitata subcapitata. Japan. J. Environ. Toxicol. 1, 1–10. doi: 10.11403/jset.17.1
Tasmin, R., Shimasaki, Y., Tsuyama, M., Qiu, X., Khalil, F., Okino, N., et al. (2014b). Elevated water temperature reduces the acute toxicity of the widely used herbicide diuron to a green alga, Pseudokirchneriella subcapitata. Environ. Sci. Pollut. Res. 21, 1064–1070. doi: 10.1007/s11356-013-1989-y
Tilman, D., Fargione, J., Wolff, B., D'Antonio, C., Dobson, A., Howarth, R., et al. (2001). Forecasting agriculturally driven global environmental change. Science 292, 281–284. doi: 10.1126/science.1057544
Torres, P. B., Chow, F., Ferreira, M. J. P., and dos Santos, D. Y. A. C. (2016). Mycosporine-like amino acids from Gracilariopsis tenuifrons (Gracilariales, Rhodophyta) and its variation under high light. J. Appl. Phycol. 28, 2035–2040. doi: 10.1007/s10811-015-0708-0
Tsui, M. T. K., and Chu, L. M. (2003). Aquatic toxicity of glyphosate-based formulations: comparison between different organisms and the effects of environmental factors. Chemosphere 52, 1189–1197. doi: 10.1016/S0045-6535(03)00306-0
Tuckey, D. M., Orcutt, D. M., and Hipkins, P. L. L. (2002). Inherent and growth stage-related differences in growth and lipid and sterol composition of algal species sensitive and tolerant to sterol-inhibiting fungicides. Environ. Toxicol. Chem. 21, 1715–1723. doi: 10.1002/etc.5620210825
USEPA (2004). Overview of the Ecological Risk Assessment Process in the Office of Pesticide Programs. US Environmental Protection Agency. Washington, DC: Office of Prevention, Pesticides and Toxic Substances Office of pesticides Programs.
Vona, V., Di Martino Rigano, V., Lobosco, O., Carfagna, S., Esposito, S., and Rigano, C. (2004). Temperature responses of growth, photosynthesis, respiration and NADH: nitrate reductase in cryophilic and mesophilic algae. New Phytol. 163, 325–331. doi: 10.1111/j.1469-8137.2004.01098.x
Wang, C., Lin, X., Li, L., and Lin, S. (2016). Differential growth responses of marine phytoplankton to herbicide glyphosate. PLoS ONE 11:e0151633. doi: 10.1371/journal.pone.0151633
Waring, J., Klenell, M., Bechtold, U., Underwood, G. J. C., and Baker, N. R. (2010). Light-induced responses of oxygen photoreduction, reactive oxygen species production and scavenging in two diatom species. J. Phycol. 46, 1206–1217. doi: 10.1111/j.1529-8817.2010.00919.x
Widenfalk, A., Bertilsson, S., Sundh, I., and Goedkoop, W. (2008). Effects of pesticides on community composition and activity of sediment microbes—responses at various levels of microbial community organization. Environ. Pollut. 152, 576–584. doi: 10.1016/j.envpol.2007.07.003
Wilkinson, A. D., Collier, C. J., Flores, F., and Negri, A. P. (2015). Acute and additive toxicity of ten photosystem-II herbicides to seagrass. Sci. Rep. 5:17443. doi: 10.1038/srep17443
Wilson, K. E., and Huner, N. O. A. (2000). The role of growth rate, redox-state of the plastoquinone pool and the trans-thylakoid ΔpH in photoacclimation of Chlorella vulgaris to growth irradiance and temperature. Planta 212, 93–102. doi: 10.1007/s004250000368
Wong, P. K. K. (2000). Effects of 2,4-D, glyphosate and paraquat on growth, photosynthesis and chlorophyll-a synthesis of Scenedesmus quadricauda Berb 614. Chemosphere 41, 177–182. doi: 10.1016/S0045-6535(99)00408-7
Wood, R. J., Mitrovic, S. M., Lim, R. P., and Kefford, B. J. (2016). The influence of reduced light intensity on the response of benthic diatoms to herbicide exposure. Environ. Toxicol. Chem. 35, 2252–2260. doi: 10.1002/etc.3379
Keywords: environment, toxicity, hazardous, pollutants, algae, cyanobacteria
Citation: Gomes MP and Juneau P (2017) Temperature and Light Modulation of Herbicide Toxicity on Algal and Cyanobacterial Physiology. Front. Environ. Sci. 5:50. doi: 10.3389/fenvs.2017.00050
Received: 04 April 2017; Accepted: 28 July 2017;
Published: 14 August 2017.
Edited by:
Johann G. Zaller, University of Natural Resources and Life Sciences, AustriaReviewed by:
Elisabeth Bondar-Kunze, Wasser Cluster Lunz, AustriaYohei Shimasaki, Kyushu University, Japan
Copyright © 2017 Gomes and Juneau. This is an open-access article distributed under the terms of the Creative Commons Attribution License (CC BY). The use, distribution or reproduction in other forums is permitted, provided the original author(s) or licensor are credited and that the original publication in this journal is cited, in accordance with accepted academic practice. No use, distribution or reproduction is permitted which does not comply with these terms.
*Correspondence: Marcelo Pedrosa Gomes, marcelopgom@icb.ufmg.br
Philippe Juneau, juneau.philippe@uqam.ca