Submarine Groundwater Discharge and Stream Baseflow Sustain Pesticide and Nutrient Fluxes in Faga'alu Bay, American Samoa
- 1Department of Earth Sciences, School of Ocean Earth Science and Technology, University of Hawaií Mānoa, Honolulu, HI, United States
- 2Water Resources Research Center, University of Hawai‘i Mānoa, Honolulu, HI, United States
It is increasingly recognized that groundwater discharge in the form of stream baseflow and submarine groundwater discharge (SGD) plays an important role in contaminant transport. This study seeks to demonstrate the importance of groundwater flow for the distribution and transport of selected pesticides and nutrients in the Faga'alu aquifer on the island of Tutuila in American Samoa. Field measurements, including seepage runs and analysis of stream and groundwater for pesticides and nutrients, were combined with hydrological modeling. Selected analytes were glyphosate (GLY), dichlorodiphenyl-trichloroethane (DDT), imidacloprid, and azoxystrobin for pesticides and chemical species of nitrogen, phosphate, and silicate for nutrients. Hydrological flow and transport models of the aquifer were built to simulate groundwater flow and to provide estimates of GLY and dissolved inorganic nitrogen (DIN) fluxes. Stream baseflow was responsible for 59% and SGD for 41% of groundwater flow to the bay, which totaled 6,550 ± 980 m3/d in the dry season when surface runoff was negligible. DDT was found in 85% and GLY in 100% of tested samples. SGD and baseflow thus delivered 9 ± 2 g/d of DDT, 0.9 ± 0.2 g/d of GLY, 570 ± 100 g/d of DIN and 840 ± 110 g/d of dissolved inorganic phosphorus (DIP) into Faga'alu Bay. While all pesticide levels are below environmental limits, their presence in baseflow and SGD, which discharge continuously year-round, result in sustained fluxes of GLY and DDT to the reef. The presence of DDT in groundwater decades after its last application confirms its long-term environmental persistence.
Introduction
There is mounting evidence that populated Pacific islands struggle with water quality problems that affect human as well as ecosystem health (Mosley and Aalbersberg, 2003; Bolabola, 2007; Erler et al., 2018). Typically, population density is highest along coastlines, where centralized sanitary systems serve only a small fraction of the population and decentralized agriculture leaves room for unregulated practices, resulting in nutrient, pathogen, and pesticide pollution. While issues related to rising human population have been recognized for decades, such as water pollution and ecosystem health decline, the contributions from individual pollution sources and pathways of contaminants are diverse and still, at best, under investigation (Mosley and Aalbersberg, 2003; Craig et al., 2005). For example, in the U.S. territory of American Samoa, anthropogenic contaminants from point, and non-point sources such as onsite sewage disposal systems (OSDS), piggeries, and agriculture, have been a widespread problem (Vaouli et al., 2010). As 90% of the municipal water on the densely populated island is sourced from groundwater, the concern over polluted drinking water resources has become a serious issue (Shuler et al., 2017). Additional concern is that pollution from groundwater propagates to streams and coastline as well (Shuler et al., 2019). Groundwater discharge to streams and the coastal ocean is often hard to quantify and is the subject of this study, which focuses on the Faga'alu watershed on the island of Tutuila, American Samoa (Figure 1A). The benthic ecosystem in Faga'alu Bay is classified as one of the most impacted on the island (Houk et al., 2005; Tuitele et al., 2014) and it has been labeled a priority remediation watershed by the U.S. Coral Reef Task Force (Messina and Biggs, 2016).
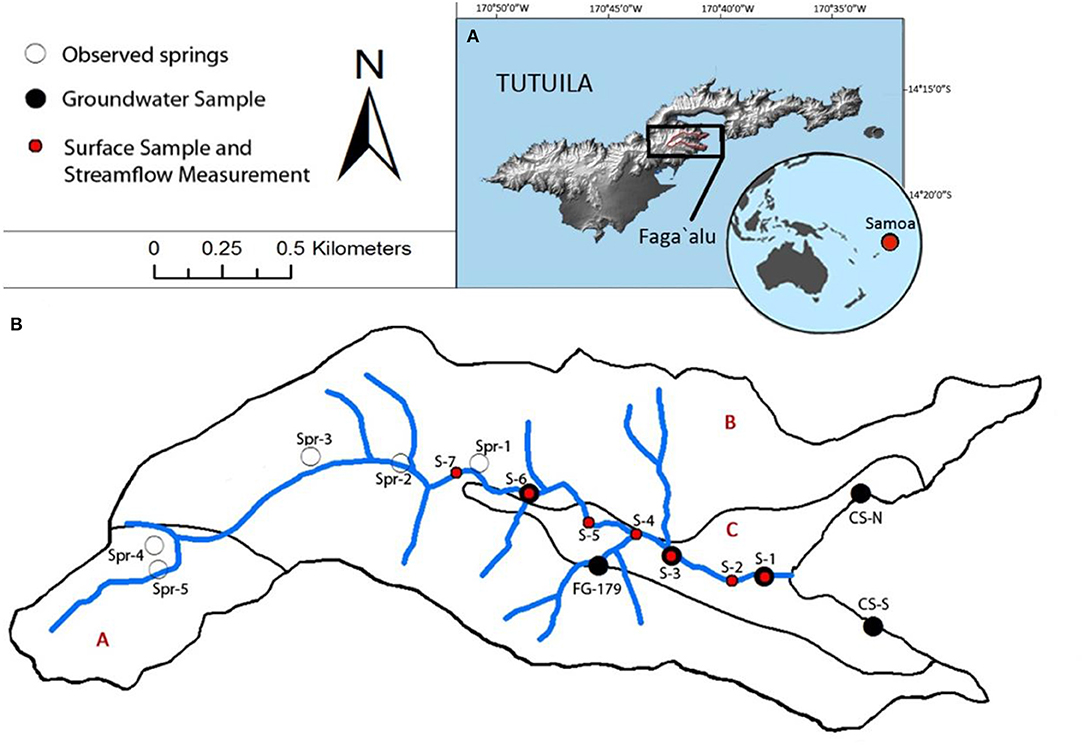
Figure 1. (A) The Faga'alu watershed is located in the central-south sector of Tutuila, American Samoa. (B) Sampling locations are depicted in the detailed map of the watershed, which is divided into primary geologic units: (A) Pago Volcanic Series, intra-caldera, trachyte plugs, and dikes, (B) Pago Volcanic Series, intra-caldera, (C) Beach sand and silty/clay alluvium (Stearns, 1944). Indicated are sampling sites of groundwater (black circles: well FG-179, shallow stream bank and coastal springs sampled using push point samplers 0.8 m below ground surface), stream water (red circles, also locations of seepage runs and 222Rn measurements), and mountain springs used as observation points for groundwater head level calibration (hollow circles).
Coral reefs support large biodiversity (Nyström et al., 2000), however, anthropogenic pollution from terrestrial sources makes reefs less resilient and more susceptible to diseases (Bruno et al., 2003). Although surface runoff collects and distributes large amounts of dissolved and suspended particulate contaminants to the coastal region (Polidoro et al., 2017), the contributions of submarine groundwater discharge (SGD) to the coastal contaminant budget is becoming more widely accepted as important, if not dominant (Johannes and Hearn, 1985; Dulai et al., 2016). Submarine groundwater discharge refers to the flow of groundwater across the land-ocean interface, which can carry dissolved compounds and often serves as an important pathway of contaminants from coastal aquifers into the ocean (Rodellas et al., 2015). SGD results from underflow, which collects solutes from the surrounding aquifer material and overlaying land-use as it moves downstream, eventually discharging at the coast when it crosses the land-ocean boundary. Studies in similar coastal volcanic geological settings (e.g., Korea, Japan, New Zealand) have found SGD to play a major role in overall water and contaminant budget (Kim et al., 2003; Hosono et al., 2012; Stewart et al., 2018). Stream baseflow, another process addressed in this study, results from underflow entering a stream where groundwater level and streambed conductivity are higher (Larkin, 1988). Stream baseflow is a connection between surface and groundwater systems and has been shown to affect stream water quality (Miller et al., 2016) and in case of small island watersheds, also coastal water quality.
Nutrient fluxes have been the focus of many SGD studies because they are widely known to leach into the water table and to be carried by groundwater flow to the coastline (Richardson et al., 2015; Amato et al., 2016; Shuler et al., 2019). Pesticides also commonly leach into and may be dispersed in aquifers (i.e., Schuette, 1998), although their mobility is species dependent and is usually lower than those of nutrients (Davidson, 1995). Chemical and physical properties such as solubility, adsorption, degradation, and volatilization determine the potential of pesticides to contaminate groundwater (Chin and Weber, 1988; Davidson, 1995). Many studies have described pesticide movement in groundwater (e.g., Zhang et al., 2009; Rendón-von Osten and Dzul-Caamal, 2017), including the presence of the ubiquitous herbicide glyphosate (Magga et al., 2008) but few have documented their transport across the land-ocean interface to the coastal ocean through SGD (Gallagher et al., 1996; Almasri, 2008).
Glyphosate has become the most heavily used herbicide in the world since its introduction as a key ingredient in the popular Roundup formula in 1974 (Benbrook, 2016). Its heavy use in both agricultural plots and roadside-to-backyard domestic settings and its mobility makes it an ideal tracer to demonstrate the chemical connection between land applications and receiving water bodies. This study thus used glyphosate as a tracer to demonstrate the role of groundwater in the transport of pesticides to streams and the coastal ocean. Other pesticides surveyed in this study include the insecticide dichlorodiphenyl-trichloroethane (DDT) along with its breakdown product dichlorodiphenyl-dichloroethylene (DDE) (from here on both collectively referred to as “DDT”), the insecticide imidacloprid, and the fungicide azoxystrobin. Application of DDT has not been legal in American Samoa since 1972, after the U.S. EPA officially banned the compound due to its adverse effects on wildlife and potential threat to human health (Lallanilla, 2019). The insecticide, however, was sprayed heavily across the island in the mid-twentieth century and is still persistent thanks to its long residence time in the environment (Travis et al., 1946; NPIC Oregon State University, 1999). Also quantified in this study were nutrient fluxes, in order to provide comparison to other island studies (e.g., Glenn et al., 2013; Shuler et al., 2019).
Field observations of contaminant concentrations and previously published land-use data were integrated with hydrological modeling to characterize groundwater fluxes and associated selected pesticide and nutrient fluxes in the Faga'alu aquifer on the island of Tutuila, in American Samoa. The modular 3-dimensional MODFLOW model (Harbaugh et al., 2000) was utilized to estimate fresh SGD and baseflow contribution while the multi-species transport model MT3DMS (Zheng and Wang, 1999) was used to model contaminant transport. The goal of the model simulation was to quantify relative contributions of the multiple glyphosate and dissolved inorganic nitrogen (DIN) sources to SGD and baseflow fluxes, including OSDS and piggeries as well as urban and agricultural non-point pollution sources. Groundwater contribution to the overall water and contaminant budget of the watershed via stream baseflow and SGD was assessed, and hypothetical scenarios focusing on changes in land-use and contaminant inputs were run to assess potential remediation actions to help develop better pollution management practices.
Study Area
American Samoa
The largest island in American Samoa, Tutuila (14.3258° S, 170.7325° W) lies within the South Pacific Convergence Zone (SPCZ). Rainfall varies geographically across the island, ranging from 3,000 to 6,000 mm/year (Craig, 2002). Wet season is between October and May, and the dry season is from June to September (Craig et al., 2005). Tutuila's mountainous terrain is a result of eroded shield volcanoes (Stearns, 1944). Alluvial valleys have been carved over time from erosion and are spaced along the island coastline (Stearns, 1944; Izuka et al., 2007). The 2 km2 Faga'alu watershed contains the Faga'alu Stream (3 km), with headwaters on the slopes of Matafao Mountain running down an alluvial valley, and emptying into the Faga'alu Bay of the outer Pago Harbor (Messina and Biggs, 2016). The undisturbed upper watershed is steeply sloped and forested, while the Faga'alu village lies on the lower alluvial plain. The valley slopes are comprised of dense basaltic rocks, which also underlie the alluvial deposits of silty and sandy clay loams of >50 m thickness on the lower plain where human development is highest (Messina and Biggs, 2016). One groundwater well, managed by the American Samoa Power Authority (ASPA) is used as a municipal water source, and is located halfway up the developed area, near the boundary where the alluvium intersects the basalt (Figure 1B). Alluvium thickness at the well is ~25 m (ASPA drilling logs, personal communication). No documented descriptions of the aquifer's hydrogeologic properties in Faga'alu exist. Although small perched bodies of groundwater have been documented in American Samoa (i.e., at Ototole and Olosega; Davis, 1963), none have yet been confirmed in Faga'alu.
Agriculture, both conventional and agroforestry, is widespread in American Samoa, and represents one of the major nutrient and pesticide sources (Misa and Vargo, 1993). Pigs are the most prevalent form of livestock raised on Tutuila, and are a source of organic matter, pathogens, and excess nitrogen (Zennaro, 2007). Cesspools are the prime method of human wastewater management, used in over 5,500 homes on the island, and contribute a significant amount of excess nitrogen into the water table (NOAA/EPA, 2003; Shuler et al., 2017). A fringing coral reef exists at the shoreline and extends 50–400 m offshore in Faga'alu Bay. Water quality of the bay is currently of major concern due to turbid, nutrient-enriched waters (Messina and Biggs, 2016; McCormick, 2017; Vargas-Angel and Schumacher, 2018; Shuler et al., 2019).
Methods
Sample Collection and Analysis
Groundwater samples were collected at six sites (three from stream bank sediments, two from coastal springs, and one from the only existing public well in the watershed, Figure 1B) in August 2016 during the dry season. Surface samples were taken at seven sites in the stream. Water from the well (screened from 20 to 32 m depth) was collected from a wellhead collection port while stream bank groundwater and spring sites were sampled using push-point samplers (MHE Products) and a peristaltic pump from 0.4–0.6 m depth below ground surface. All samples were filtered on-site with a 0.45 μm hydrophilic polyethersulfone capsule filter. Nutrients were collected in acid-washed high density polyethylene (HDPE) bottles. Pesticides were collected in combusted glass amber vials to prevent photodegradation. Once collected, all samples were chilled and then refrigerated for short-term storage. Water for radon analysis was collected in 250 mL glass bottles with no headspace. A YSI multiparameter sonde (6600 V2-2 model) was used to measure temperature, salinity, and dissolved oxygen in situ in each sample.
A seepage run, where streamflow was measured at seven stations along the main channel, was performed under baseflow conditions on the same day and locations as sample collection (Figure 1B). The SonTek FlowTracker Handheld Acoustic Doppler Velocimeter was used to measure streamflow. Samples were analyzed for radon (222Rn) at each surface and groundwater site to identify groundwater contribution and hyporheic exchange, thus identifying gaining and losing sections of the stream (Burnett and Dulaiova, 2003; Dulaiova et al., 2006). Radon samples were analyzed the day of collection (Supplementary Table 1) using a Rad-H2O instrument (Durridge).
Water samples were analyzed for their nutrient concentrations of total dissolved nitrogen (TDN), phosphate (), nitrate and nitrite (N+N), ammonium (NH3 from here on referred to as ), and silicate (Si(OH)4) at the University of Hawai‘i SOEST Laboratory for Analytical Biogeochemistry. Dissolved inorganic nitrogen (DIN) concentrations were calculated as the sum of the measured N+N and values. Pesticide analysis included GLY, DDT, imidacloprid, and azoxystrobin. An enzyme linked immunosorbent assay (ELISA) (Abraxis LLC) analysis was performed for the four chosen pesticides. The colored wells were analyzed in a spectrophotometric microplate reader (Abraxis, Model 4303) at a wavelength of 450 nm to obtain concentration values. The minimum detection limits for GLY, DDT, imidacloprid, and azoxystrobin were 50, 370, 6, and 9 ng/L, respectively (www.abraxiskits.com). Relative uncertainty of GLY concentrations based on field and method duplicates derived via the ELISA test was 10–22%. Relative uncertainty of DDT was 10–16%. Nutrient uncertainties, expressed as absolute errors were 17 μg/L for TDN, 0.3 μg/L for , 8 μg/L N+N, 2 μg/L , 56 μg/L Si(OH)4. Radon relative uncertainties derived from counting statistics were 10–30% at 1-sigma.
Modeling Approaches
Water flow and contaminant transport were modeled using the MODFLOW (Harbaugh et al., 2000) and MT3DMS (Zheng and Wang, 1999) models, respectively. The MODFLOW model was used to predict groundwater levels and water fluxes necessary for transport modeling via MT3DMS. The Groundwater Modeling System (GMS) software (https://www.aquaveo.com) served as a graphical interface in building the conceptual models. MODFLOW was calibrated using (1) stream baseflow from seepage runs taken during sample collection and (2) water table head levels obtained from the well and elevations of groundwater springs documented during this study. The elevations of the observed mountain springs were utilized as water levels where the groundwater spring represents an intersection of the top of the water table and the land's surface. This relies on the assumption that the observed spring is fed by a saturated zone that is hydraulically connected to the aquifer. Field-based groundwater levels and baseflow fluxes were utilized through both iterative and automatic approaches in calibrating MODFLOW. The iterative approach was used initially to constrain hydraulic conductivity, while the automatic approach via the PEST code (Doherty and Hunt, 2010) was used to refine the conductivity estimates. MT3DMS was used to model contaminant transport in the aquifer through convection, dispersion, adsorption and decay. MT3DMS was calibrated through an iterative approach to match observed against modeled GLY and DIN concentrations. A flow chart relating the models and their respective inputs and outputs can be found in Supplementary Figure 1.
Groundwater Flow Model
The MODFLOW-2000 (McDonald and Harbaugh, 1988) numerical model comprised of 4221 active cells (38 × 30 m) across two vertical layers. The top layer covered the zone between mean sea level (MSL) and the terrain elevation, while the lower layer covered MSL to 500 m below MSL. Recharge was based on estimates from the Soil and Water Assessment Tool (SWAT) model created by Leta et al. (2017), with daily recharge ranging from 1.8 to 3.5 mm/d across the watershed. Other data were sourced from available geographical information system data that included stream, well, geologic units, and domain boundary data. A pumping rate of 163.53 m3/d was assigned to the well based on data provided by American Samoa Power Authority (ASPA).
The six observation points for head level were supplemented by stream baseflow discharge rates, which were obtained from the seepage run measurements. The zonal parameter estimation method via PEST software (Kennedy, 2012; MODFLOW-PEST Pilot Points, 2016) was incorporated in the calibration process to obtain the best fit between measurements and model values. Conductivity-calibration zones were set based on known geological units (Figure 1B). The model was considered adequately calibrated when residuals for head and discharge were minimized to within 10% of maximum observed values.
Pesticide and Nutrient Fluxes in Baseflow and SGD
To separately estimate baseflow from high level and coastal aquifer portions of the watershed in the model, MODFLOW stream arcs were divided into upper and lower reaches (boundary set at S-5, Figure 1B). To obtain the respective mass flux rate, pesticide and nutrient concentrations from stream bank groundwater samples were multiplied by the modeled groundwater flow estimates for each segment.
SGD fluxes at the land-ocean interface were determined by summing up flow through coastal grid cells in three sections of the model: (1) the northern coastline, (2) the central coastline, and (3) the southern coastline. The central coastal segment representing the region from the stream mouth to S-1, was excluded from SGD and was included in stream baseflow instead. Pesticide and nutrient concentrations at the northern and southern coastal springs were used to represent their respective segment of the coast, while S-1 was used for the central coastal segment. To estimate the flux rates, the respective contaminant concentrations were multiplied by the estimated SGD flow for the corresponding coastal segment.
Contaminant Transport Model
An MT3DMS transport model was developed to simulate the advective and dispersive transport and transformation of contaminants through the aquifer. In addition to hydrologic data carried over from the hydrogeologic model, new parameters were incorporated in the transport model, including porosity, longitudinal dispersivity, dispersivity-anisotropy ratios, and DIN and GLY attenuation and decay coefficients. A sensitivity analysis test was run to determine which parameters most affected the calibration (Supplementary Figure 2) and adjustments were made to find the optimal match between the modeled and observed values (Supplementary Table 2). The simulation-time was about 55 years to obtain a steady-state condition for each contaminant.
Glyphosate served as the representative pesticide to trace movement through the aquifer. GLY concentrations were added in the model based on annual applied concentrations by agricultural activities derived from literature values (Supplementary Table 3), which were added as recharge concentrations. GLY, however, is not only used for agriculture, but also for smaller-scale applications along roadsides and in residential yards for weed control (Tang et al., 2015). As an approximation, and due to the lack of information, half the concentration applied to agricultural plots in the model was assumed to pertain to the domestic zones. A literature-based sorption coefficient (1.64 × 10−5 m3/mg) as well as decay rate constant (based on half-lives ranging from 91 to 197 days) were used in the model to represent natural degradation of GLY (Schuette, 1998; Henderson et al., 2010).
DIN loading rates were estimated for each endmember using attenuation rates specific to each source (Supplementary Table 3). Mass loading rates were applied to the point sources of cesspools and piggeries, while annual application concentrations were applied to non-point source agricultural N-inputs. A fixed level of N was additionally added into all non-agricultural domains to represent natural background levels of DIN (Shuler et al., 2017). Fluxes of DIN and GLY for ocean and stream segments of interest were then determined by the calibrated transport model.
Contaminant Source Contributions
The calibrated MT3DMS model was used to run several hypothetical land-use scenarios for DIN and GLY source contributions throughout the watershed. Each source of DIN was individually assessed for its relative contributions to the total N flux via baseflow and SGD. A scenario was then run when cesspools were improved (i.e., converted to septic systems) by doubling their DIN attenuation efficiency, which was simulated by halving the DIN concentration that entered the aquifer from each OSDS unit (Supplementary Table 3). To determine how the rates of agricultural or domestic GLY affect concentration at each observation point, scenarios were run to simulate each source individually. Two additional scenarios were run where the application amount of GLY was halved in the developed zones and then in all the application zones to simulate improved practices if half of the currently used herbicide was applied. These scenarios may give insight to what could be accomplished in the future to improve water quality of the stream and coastline.
Results
Water Quality Results
Overall, 100% of Faga'alu samples had detectable levels of GLY and 85% had DDT. Groundwater GLY concentrations ranged between 80 and 300 ng/L and averaged 160 ± 80 ng/L. Stream bank groundwater samples had relatively higher value furthest upstream at S-6 (180 ng/L), and lower values at S-3 (80 ng/L) and S-1 (90 ng/L). The well (FG-179) had a concentration of 160 ng/L and the northern and southern coastal springs were 160 and 300 ng/L, respectively (Table 1, Figure 2A). Glyphosate in surface samples at the seven stream sites ranged between 60 and 230 ng/L with an average of 140 ± 70 ng/L (Table 2). Higher concentrations were found in the upper four sites (S-4 to S-7), averaging 180 ± 40 ng/L, the lower three stream samples (S-1 to S-3) averaged 80 ± 20 ng/L. When comparing the concentrations at sites which had both surface and groundwater samples (S-1, S-3, S-6), GLY was comparable in surface water to groundwater (S-1 80 vs. 90 ng/L, S-3 90 vs. 80 ng/L, S-6 200 vs. 180 ng/L).
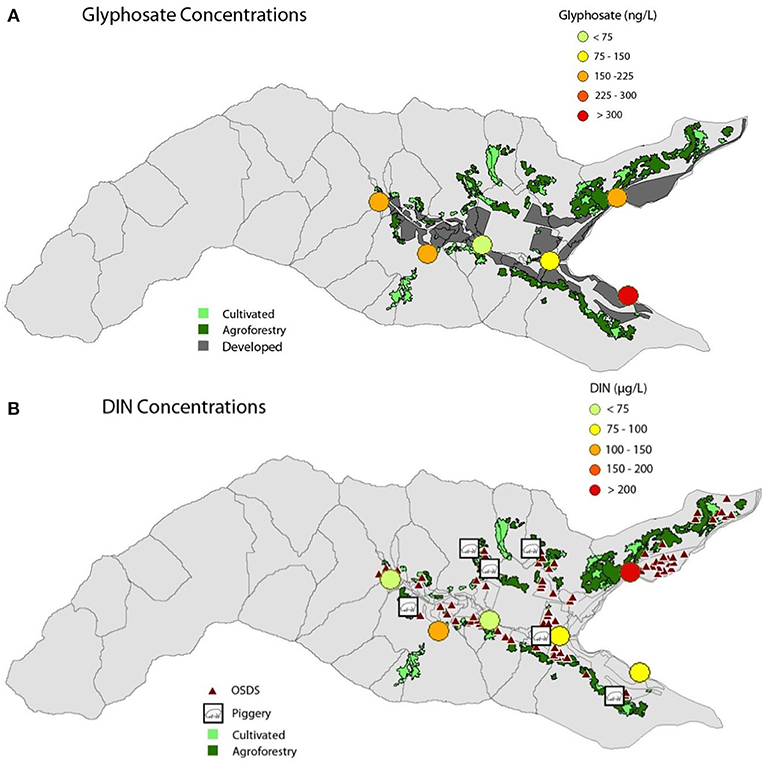
Figure 2. (A) GLY and (B) DIN concentrations at the six groundwater sampling sites in Faga'alu, shown with related point source and land-use contributors—cultivated (light green polygons), agroforestry (dark green polygons), OSDS units (triangles), piggeries (square icons), and unpaved developed/residential land (dark gray).
DDT was ubiquitously present in groundwater samples, ranging from below detection limit to 2,070 ng/L with an average of 1,400 ± 690 ng/L. In surface waters, DDT ranged from below detection limit at S-5 to 2,030 ng/L at S-7 with an average of 1,240 ± 720 ng/L (Tables 1, 2). The other two tested pesticides, imidacloprid and azoxystrobin, were not detected in any of the Faga'alu samples, and will not be discussed further.
DIN in Faga'alu groundwater ranged between 60 and 250 μg/L and averaged 110 ± 70 μg/L, with generally higher concentrations toward the coastal areas (Figure 2B). Stream bank groundwater samples had lower DIN than the coastal springs and the well (Table 1). DIN in stream surface waters were comparable to groundwater concentrations across the watershed, with a range from 60 to 140 μg/L and an average of 80 ± 30 μg/L. Ammonium did show notable differences, as it averaged 4 ± 2 μg/L in surface water compared to 19 ± 23 μg/L in groundwater samples with a maximum of 130 μg/L at S-1. This is expected because of limited oxygen present in streambank groundwater as opposed to the oxygenated stream.
Phosphate concentrations ranged from 50 to 260 μg/L in groundwater samples with an average of 140 ± 70 μg/L, and ranged from 80 to 120 μg/L with an average of 110 ± 10 μg/L in the surface water samples. Silica showed a relatively even distribution across stream bank groundwater and stream surface samples, averaging 16,210 ± 6,970 μg/L and 16,480 ± 300 μg/L, respectively, but showed much more diluted values at the northern and southern coastal springs (6,910 and 1,940 μg/L, respectively). The well had Si(OH)4 concentrations of 20,200 μg/L.
Groundwater Flow Model Results
The final calibrated MODFLOW model had a root mean squared residual (RMSR) of 12 m for head and a RMSR of 256 m3/d for discharge. The model was considered satisfactorily calibrated when the RMSR (head+flow) was 2.31 and the coefficients of determination (r2) were 0.99 for head and 0.82 for flow (Tables 3, 4).
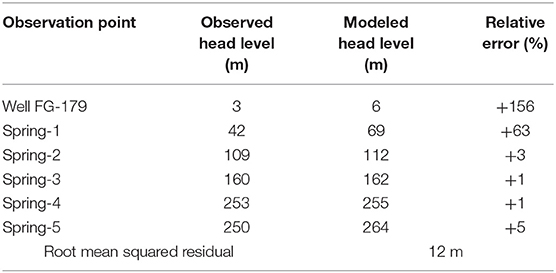
Table 3. Observed and modeled groundwater head levels of the calibrated MODFLOW model with relative error.
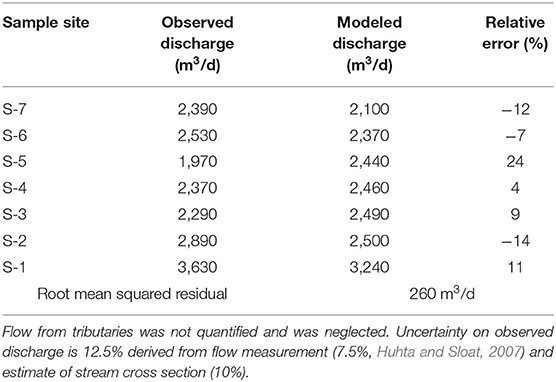
Table 4. Observed streamflow under baseflow conditions assuming no additional surface runoff contribution, in comparison to modeled baseflow results.
The modeled freshwater SGD from the coastline of the entire bay was 2,680 ± 290 m3/d, while the modeled stream baseflow was 3,870 ± 430 m3/d. Both estimates have 11% uncertainty assigned based on the relative error derived for baseflow (Table 4). The high-level aquifer baseflow in the upper reach of the stream contributed 63% of the stream's flow, while the lower reach contributed 37%, which reflects gaining stretches both upstream and near the coast. The field-based seepage run and radon concentration trends verified this pattern of gaining stretches of the stream (Supplementary Figure 3). Radon concentrations in stream bank groundwater were higher where baseflow was present, suggesting radon rich groundwater upwelling as opposed to intrusion of low-radon hyporheic flow. Accordingly, stream radon levels were also highest at sections predicted to be gaining reaches. While these observations are only qualitative, they confirm the model predicted patterns of surface water groundwater exchange. According to the model, most of the watershed's SGD came from the central coastline of the bay (69%), while the northern and southern coastlines contributed 18 and 13%, respectively (Figure 3). The modeled combined flow of groundwater to Faga'alu Bay under baseflow conditions, delivered by the combination of stream baseflow and freshwater SGD was thus 6,550 ± 980 m3/d (Table 5).
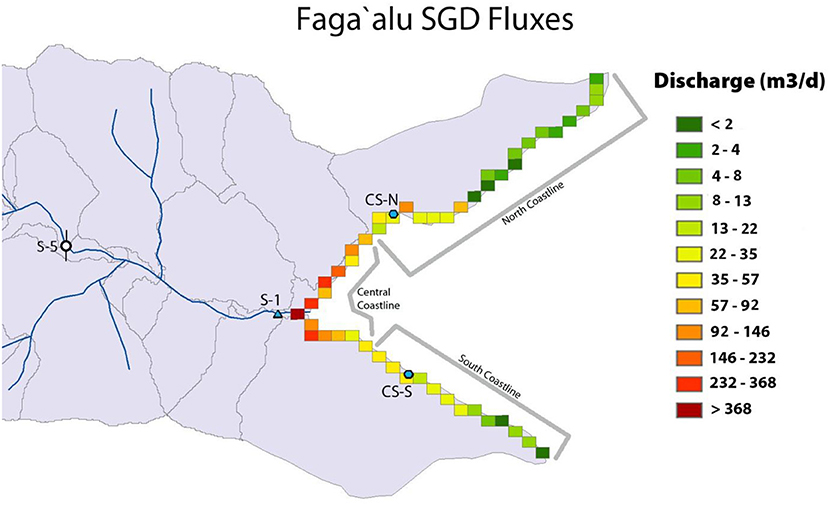
Figure 3. MODFLOW model derived SGD fluxes show larger groundwater discharge to the central portion of the bay, with diminishing fluxes outward toward the northern and southern ends of the bay. Grid cells belonging to the northern, central, and southern sectors of the coastline as well as coastal springs (CS-N, S-1, and CS-S) are shown.
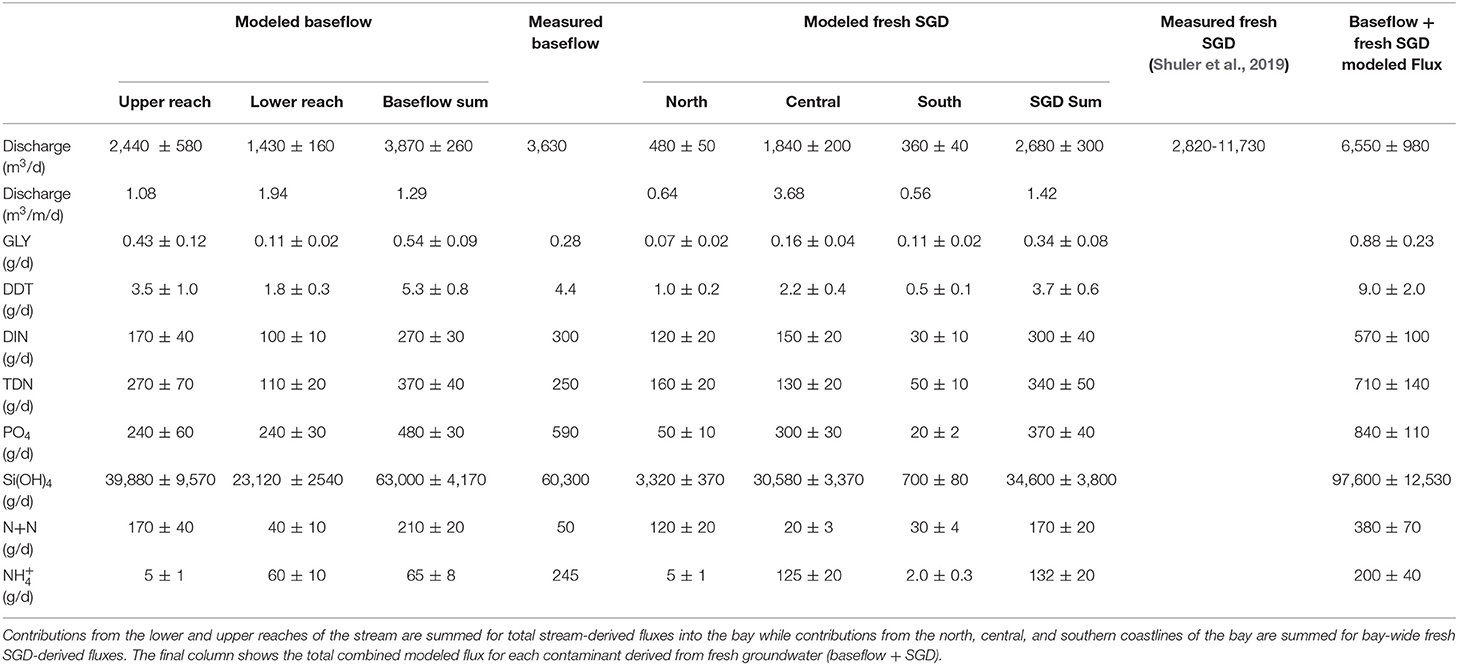
Table 5. Modeled groundwater discharge via stream baseflow and fresh SGD, and their associated pesticide and nutrient fluxes based on observed concentrations in each representative section of the watershed.
Contaminant Transport Model Results
The results of MT3DMS model are shown in Supplementary Table 4, where calibration was performed using GLY and DIN as tracers for agricultural, domestic and mass loading pathways within the watershed. For DIN, most sites were underestimated in the model in comparison to the measured values (1–91% relative error). The MT3DMS model was considered acceptable when the mean absolute error (MAE) of the total modeled vs. observed DIN values fell within 15% of the maximum observed DIN concentration (250 μg/L) and obtained an r2 of 0.66. Modeled glyphosate levels had an MAE of 125 ng/L, producing a low r2 value (0.024). The model produced estimates that were within an order of magnitude of the observed concentrations but did not capture GLY in the upper watershed (S-6) and therefore was only used as a screening tool to make qualitative assessments of GLY distribution and to compare various land-use scenarios. Fluxes of GLY and DIN determined using the MT3DMS model are listed in Supplementary Table 5 along with those estimated using the MODFLOW derived water fluxes multiplied by field observations.
Land-Use Contaminant Source Scenarios
From all the considered N-sources piggeries contributed 6% of the total DIN, agriculture contributed a very small fraction (<1%), and OSDS units added the largest fraction, 90% of DIN concentrations recorded at the observation points. Natural N which was added into each polygon resulted in a 4% contribution to the total concentration at our observation sites (Supplementary Table 6). The scenario where OSDS N-attenuation efficiency was doubled, resulted in 46% less DIN at the observation points, significantly reducing the amount of this anthropogenic pollutant discharging into the stream and the coastal ocean.
Agricultural inputs represented 28.5% and domestic GLY application contributed 71.5% of the total GLY observed at the sampling points, despite half as much GLY assumed to be applied for domestic uses. This is partially a result of the proximity of domestic applications to the stream but also an artifact of the model not capturing GLY groundwater concentrations at the gaining section of the stream at station S-6. In absence of available information, this study did not include any major agricultural or domestic GLY sources in the upper watershed despite elevated GLY being recorded consistently in groundwater and the stream. As domestic GLY applications delivered high concentrations at the stream and coastal observation points, a scenario was run where it was assumed that people used half of the glyphosate in the domestic zones. The concentrations at the observation points dropped by 37% in this scenario. When GLY application rates were cut by half in agricultural zones, the total concentrations entering our observation sites dropped an additional 13%, to total a 50% decrease.
Discussion
Contaminant Concentrations and Fluxes
Pesticides
Gaining sections of the stream were identified via multiple methods (seepage run, radon, hydrological model), where all methods were in good agreement that there was baseflow contribution from the high level as well as basal aquifers. GLY was observed in all groundwater samples and an important factor that seemed to drive stream GLY concentrations was the presence of baseflow in gaining reaches. Station S-6 marks the upper boundary of the village and there is a small plot of agriculture adjacent to it, with a quarry just upstream. Seepage runs and elevated stream and groundwater Rn showed the stream to be gaining water from the underlying aquifer in this region. Groundwater at S-6 was higher in GLY than any other stream bank station. Surface stations S-5, 6, 7 were also all higher in GLY than any other sections of the stream. In contrast, S-3, located downstream of the upper watershed in the losing reach of the stream had the lowest concentration of all sites (80 and 90 ng/L GLY in ground- and surface water, respectively).
The well and northern coastal spring (both 160 ng/L) are each downslope of agricultural plots, possibly contributing to their glyphosate load. The southern coastal spring probably integrates inputs from multiple sources, including agriculture as well as domestic uses and had the highest recorded concentrations (300 ng/L). Observed concentrations and transport model results suggest that because of their proximity to the stream, applications on domestic properties have the potential to deliver GLY to the stream sites in addition to the larger but more distant agricultural inputs. This can be attributed to GLY breakdown within the aquifer and its sorption to soil and so nearby applications to be more influential. For example, S-1 (90 and 80 ng/L GLY in ground- and surface water, respectively) in the lower village lies farthest from any significant agricultural plots, but lies within the central part of the village on a gaining section of the stream. Here, possible GLY application sites include roadsides and yards in upstream homes where herbicides containing glyphosate may be applied to control unwanted weeds, although contributions from upstream agricultural plots via deeper groundwater flow lines cannot be ruled out.
Glyphosate levels in Faga'alu stream and groundwater were orders of magnitude lower than the Maximum Contaminant Level (MCL) of 700,000 ng/L in drinking water (EPA, 2009) or environmental limits (mg/L; EPA, 1993). GLY concentrations found across the watershed were comparable to findings elsewhere, such as a study in Catalonia by Sanchis et al. (2012) which found average GLY to be 200 ng/L in groundwater. GLY concentrations in groundwater in Canada were reported at 660 ng/L (Van Stempvoort et al., 2014, 2016), even higher levels were recorded in a study in Mexico (440 to 1410 ng/L, Rendón-von Osten and Dzul-Caamal, 2017). In Faga'alu, groundwater flow was responsible for 0.54 ± 0.09 and 0.34± 0.08 g/d of GLY discharge via baseflow and fresh SGD, respectively.
The relatively uniform distribution of DDT reflects its method of island-wide application several decades ago (NPIC Oregon State University, 1999). Its presence in stream bank groundwater and coastal springs so long after its last application is notable, which reflects its persistent nature as it is adsorbed onto soils and slowly leaches into groundwater. Whitall and Holst (2015) reported sediment DDT concentrations of up to 2.3 ng/g in Faga'alu watershed, suggesting that sediments are likely to be a reservoir of DDT from which groundwater may mobilize it. In this study, Faga'alu had similar concentration ranges of DDT in both surface and groundwater. DDT fluxes to the reef were determined to be 5.3 ± 0.8 and 3.7 ± 0.6 g/d via baseflow and SGD, respectively (Table 5). DDT concentrations of up to 2,000 ng/L were observed in both stream and groundwater samples, nearly half of the water quality limit of 5,000 ng/L (EPA, 2016).
Nitrogen
Nitrite, nitrate, and ammonium concentrations (Tables 1, 2) were all orders of magnitude below recommended US limits [drinking water MCLs of nitrite 1 mg/L, nitrate 10 mg/L (EPA, 2009), ammonium environmental limit 0.25–32.3 mg/L Oregon Department of Human Services, 2000]. Measured nutrient concentrations followed land-use and groundwater flow patterns across the watershed with increasing concentrations downstream of the agricultural and populated areas in the watershed. In the samples, between 65 and 100% of TDN consisted of DIN, and thus both followed a similar distribution pattern. N+N represented between 20 and 70% of DIN, with representing the rest. The northern coastal spring (CS-N) had the highest DIN (250 μg/L) and TDN (340 μg/L) concentrations, likely a result of the high density of OSDS units along the coast, along with large agricultural plots up the slope of the adjacent ridge. As the transport model predicts, OSDS is likely the primary contributor of DIN in Faga'alu groundwater with as much as 90% of groundwater DIN originating from them.
Although N+N generally contributed more to DIN than did in Faga'alu samples, the stream bank sample at S-1 had lower N+N than , which can likely be explained by anoxic organic rich estuarine sediments. Additionally at site S-1, DIN was 72% higher in the surface samples than groundwater samples. At other sites, surface stream DIN levels were all comparable to the stream bank groundwater.
Of the sites sampled, the highest concentrations of N were observed at the two coastal springs and the well. This is not surprising, as coastal springs integrate all upstream inputs and are also a site of converging shallow and deeper groundwater flow lines. This also highlights the point this study is making, that SGD should be considered in coastal nutrient budgets, as it is a point of source and flow convergence and an important nutrient pathway to the coastal ocean.
Phosphate and Silicate
As phosphate acts as an essential constituent of organisms, the EPA has no set MCL for drinking water (Oram, 2014a). In freshwater bodies, phosphate is usually a limiting nutrient, thus in excess can lead to accelerated eutrophication, leading to algal blooms and lower dissolved oxygen. The EPA has set the maximum acceptable stream concentration to 100 μg/L (Oram, 2014b). Four of the six stream bank groundwater and five of the seven stream surface sites sampled exceeded this amount (Tables 1, 2) suggesting that groundwater contribution likely plays an important role in driving these elevated concentrations. Specifically, the well (FG-179) had the highest concentration of phosphate (260 μg/L), possibly due to the accumulation of upslope agricultural inputs in the deep groundwater, but more likely the longer groundwater pathways obtaining enriched P from natural rock weathering. It has been shown that DIP in volcanic aquifers can naturally be elevated (Porder and Ramachandran, 2013), so it is possible that natural sources (i.e., basalt weathering) are responsible for the elevated groundwater PO4 concentrations.
Silicate in groundwater reflects water-rock interactions, as Si dissolves from silica-rich minerals within the underlying rocks. Silica content is also directly proportional to groundwater residence times within the rocks in the aquifer (Pradeep et al., 2016). This is often reflected in deeper-sourced groundwater over shallow-sourced water (Khan et al., 2015). This seems to be the case in Faga'alu, as the highest concentration of Si(OH)4 was found in the well (FG-179), which is sourced from the deepest groundwater in the study. The coastal springs showed the lowest Si(OH)4 values, as they were diluted by the ocean. The range of Si(OH)4 concentrations in groundwater was 1,940–20,200 μg/L, with a mean concentration of 12,950 ± 6970 μg/L. The stream bank sites average, however, was <2% different from the surface stream sample average concentration. This confirms active surface water-groundwater interactions in the stream.
Evaluation of the Groundwater Flow Model
It should be noted that MODFLOW and related simulation software assumes validity of the continuum approach implying that aquifers can be treated as porous media (Bear, 1979). Such an approach defines a representative elementary volume (REV) that is large enough to represent the medium properties, such as porosity or hydraulic conductivity, but still small enough to be considered as a point in the mathematical formulation of the water flow process. In practical terms, field measurements are considered as average values over the REV. In a case where significant preferential flow exists, for example due to the existence of large size fractures, applicability of porous media models will be questionable. Two interacting flow systems would exist in this case with Darcyan and non-Darcyan flows in the porous media and fractures, respectively (Bear, 1979). Modeling efforts will thus be complicated by requiring data about the location, size, and properties of the fractures. Volcanic aquifers may include conduits, fractures, dikes, and other features. However, many modeling studies completed in American Samoa, Hawaii, and other Pacific Islands have successfully utilized porous media models in managing groundwater resources (e.g., Oki, 2005; Izuka et al., 2007; Whittier et al., 2010; Gingerich and Engott, 2012). In this regard, hydraulic conductivity is typically calibrated for various geologic units integrating smaller-scale heterogeneities within the aquifer. In our case, although all calibrated head elevations of the modeled water table lie within 10% of total head values in the watershed, two sites (Spring-1 and the well) had a high relative error of 60 to 150%, respectively (Table 3). The poor match at these sites is likely the result of unknown small-scale hydrogeological properties of the subsurface.
For our purposes of estimating baseflow and SGD, the model adequately estimated discharge observations and gave us confidence in its applicability to study contaminant fluxes. The modeled stream baseflow measurements matched the observations to an average of 11%, with absolute error ranging from 90 to 464 m3/d across the sites. The RMSR of 256 m3/d falls well below 10% of the total modeled stream baseflow (3,870 m3/d). The modeled baseflow entering the bay at the lowest stream station, S-1 overestimated the total measured surface flow by only 11 %. Additionally, Faga'alu Stream gauge measurements from August 10th, 2016 recorded a total streamflow of 3,830 m3/d, with baseflow delineated at 3,670 m3/d on the day of the seepage run (https://github.com/cshuler/ASPA-UH_Stream_REPO), only 5% relative error compared to the modeled flow. Contrary to observations, however, the model did not calculate any of the sections to be losing water to the aquifer. Every reach of stream calculated in the model was gaining from the last (Table 4), while the seepage run identified the presence of both gaining and losing sections. Specifically, between site S-6 to S-5, discharge dropped by over 550 m3/d, while the model predicted a 67 m3/d increase in flow. Such a discrepancy is again most likely due to simplifications in the conceptual model that do not accurately capture the true geologic features of the site. Our study approach was therefore to combine field observations and modeling to derive contaminant transport pathways.
The MODFLOW-derived groundwater fluxes were compared to the measured values collected during a previous study in 2014-2015 (Shuler et al., 2019), which consisted of baseflow separation and indirect measurement of SGD entering the bay calculated by a 222Rn mass balance (Shuler et al., 2019). Groundwater flow as a sum of SGD and stream baseflow was 5,500–14,400 m3/d (Shuler et al., 2019) by comparison to 6,550 ± 980 m3/d in this 2016 study. Although both measurements occurred during American Samoa's dry season under baseflow conditions, some variability in the elevation of the water table due to seasonal conditions likely exists. This study's estimate is on the lower end but within the range of the previously published results. The shore length-normalized fresh SGD rates of 0.6 to 3.7 m3/m/d (Table 5) are comparable to rates found at other volcanic island settings such as Jeju, Hawaii, Japan, and New Zealand (Kim et al., 2003; Hosono et al., 2012; Dulai et al., 2016; Stewart et al., 2018). In such settings reported fresh SGD rates can be up to 100 m3/m/d and it is interesting that a relatively wet place such as Tutuila is on the lower end of the spectrum of observed fresh SGD rates. Otherwise, fresh SGD volume of ~2,000 m3/d discharging to the whole Faga'alu Bay is comparable to similarly-sized embayments, see for example synthesis of previous work in Hawaii Islands by Kelly et al. (2019).
Nearly as much water was delivered to the bay via SGD (41%) as from the stream (59%) during baseflow conditions, making SGD a considerable fraction of the daily water and solute load in the dry season. The central coastline of the bay contributed more SGD than both the north and south coasts combined. Other studies similarly found elevated groundwater discharge to occur in estuaries of streams and rivers (e.g., Buddemeier, 1996; Dulaiova et al., 2006). The northern coastline contributed slightly more SGD than the southern shore, which agrees with overall SGD distribution patterns in Faga'alu found by Shuler et al. (2019). This agreement supports that the water fluxes determined by the model can justifiably be used to estimate contaminant transport. It is, however, important to point out that the study only addresses a one-time observation during dry season, so it should not be extrapolated to annual average conditions. At the same time, SGD and baseflow are a continuous year-round input to the coastline so the results presented are minimum contaminant fluxes. Land-based pollution runoff probably increases when surface runoff is present in the wet season.
The approach only estimated freshwater SGD, which is considered the major pathway of terrestrial contaminants and probably captures GLY fluxes appropriately. DIN however may also be contributed by the saline component of SGD as demonstrated elsewhere. Mulligan and Charette (2006) showed that ammonium may be produced in the subterranean estuary (STE) by remineralization processes and released within the salinity transition zone. At the same time nitrate removal by denitrification in the STE may attenuate the terrestrial DIN flux. This study did not investigate nutrient transformation and attenuation within the salinity transition zone, instead it uses nutrient concentrations past the STE sampled at the groundwater discharge point. Likewise, due to lack of information on the salinity transition zone, this study did not develop a density-dependent groundwater model to account for salt-fresh water interaction at the land-ocean interface. Density effects were ignored due to the lack of salinity data but future studies could explore the role of the STE for contaminant attenuation and SGD via density dependent groundwater models such as SEAWAT.
Groundwater-Derived Contaminant Fluxes Based on Observed Concentrations and Model-Derived Groundwater Fluxes
SGD from the central coast delivered the most contaminants across the land-ocean interface, with the exception of TDN and N+N (Table 5), the majority of which, according to the model were delivered from the northern shoreline. This assumes that CS-N TDN levels are valid for the whole northern section of the coastline. The upper stream reach contributed much more discharge than the lower reach. Likewise, contaminant fluxes entering the stream from baseflow were higher in the upper reach, partly because of higher concentrations, also because of much higher water discharge. The only exception to this was DIP, which was distributed equally, and ammonium, which had over 10 times higher concentrations in the lower reach although it may be oxidized during discharge. Field observations and modeled groundwater discharge in this study estimated that SGD contributes a comparable amount of DIN, DDT and GLY as the stream. The combined total flux of each contaminant entering the bay each day via fresh SGD and baseflow thus has the potential to greatly influence water quality and reef health in this designated priority watershed.
Evaluation of the Contaminant Transport Model
MODFLOW derived groundwater flow was utilized in MT3DMS to determine contaminant flow. Observations at one well and five stream sites (6 GLY, 6 DIN) were evaluated in the transport model. Limitations of the study were the low number of observations and that concentrations at each site could fluctuate greatly over seasons and years, so we are reporting results as “per day” and concentrations and fluxes represent dry season estimates. Additionally, in contrast to groundwater flow models, porous-media transport models are less accurate under the assumption of continuous media where preferential flow can prevail due to fractures (e.g., Glenn et al., 2013). Another uncertainty in this study is the absence of actual site-specific chemical application rates and attenuation coefficients of nutrients and pesticides. Given such difficulties, we were able to obtain model estimates that matched observations to within an order-of-magnitude for both GLY and DIN concentrations at all sites. Such uncertainties support the use of this model primarily as a screening tool in demonstrating the importance of groundwater fluxes and predicting scenarios involving variables relative to one another, rather than for precise quantitative analysis (Zheng et al., 2012). Future studies should emphasize a wider spatial and temporal distribution of sampling sites as well as identify application rates of fertilizers and herbicides along with OSDS and piggery mass loading rates, rather than relying on literature data.
Toward using the model as a screening tool, a sensitivity analysis was performed. Dispersivity played the largest factor in affecting end concentrations of both solutes at all sites, whereas porosity specifically affected GLY concentrations more than DIN (Supplementary Figure 2). Sensitivity to anisotropy ratios was most noticeable at the northern coastal spring (CS-N). Extremely high fluxes of DIN appear in this northern coastal sector compared to other regions of the watershed with similar OSDS density, which might be the result of how PEST zonally estimated hydraulic conductivity in this location.
The solute fluxes derived from the transport model fell within the same order of magnitude as the values estimated by MODFLOW's derived groundwater discharges and observed concentrations (Supplementary Table 5). The MT3DMS model underestimated GLY fluxes and overestimated DIN fluxes, due to inconsistencies in the modeled upper reach of the stream. Fluxes based on MODFLOW estimates are thus more realistic than those based on MT3DMS estimates, considering the model limitations discussed above.
Scenarios of GLY and DIN Source Variability and Resulting Fluxes in the Watershed
Scenarios were run using the transport model to look for relative contributions of each point and non-point source to dissolved contaminant loads. The transport model predicted that 90% of groundwater DIN is being contributed to the observation points by OSDS input. As these are installed below ground, possibly interacting with the water table, OSDS have the most direct nutrient loading pathway. The other 10% of DIN transported in the aquifer according to the model is contributed mostly by piggeries and natural sources. The trends are not valid across the entire watershed though, for example, piggeries are estimated to contribute 20% DIN in the southern coastal spring (CS-S), indicating that the model predicts the nearby piggery to play a larger role at this site than piggeries on average at other sample sites. Fertilizer application was the source of only <1% of DIN contribution estimated by the model to arrive at our observation points. In Hawai‘i, it has been found that very small fractions of agricultural DIN leach into groundwater in the root zone of plants (El-Kadi and Yabusaki, 1996), which could explain why fertilizer N shows such small contribution to the DIN inventory in the model. Watering techniques and high precipitation could also inhibit fertilizer's capability to make it into the groundwater by creating a surface runoff to the nearest surface water body. However, other studies attribute heavy agriculture to play a larger role in groundwater nutrient budgets and coastal SGD N-fluxes (e.g., Amato et al., 2016).
In a study that looked at watershed nutrient sources on the same island but a different watershed, Shuler et al. (2017) showed that OSDS contributed 60 ± 7% of the DIN flux. The fraction of groundwater-derived DIN estimated by this study's MT3DMS model (90%) would thus fall a bit higher. The piggery contribution was 20 ± 6% in comparison to 6% in this study. The agricultural N inputs were 9 ± 4% in Shuler et al. study 2017 in comparison to <1% in Faga'alu. While the absolute fractions were different between the two studies and watersheds, the relative role of each source was similar, OSDS being the dominant DIN contributor.
Scenarios of improved OSDS installations and decreased pesticide-use were run to predict the impact of changes in land-use management practices on groundwater quality. When DIN mass loading rates were halved for each OSDS-unit, simulating a 2-fold improvement to the assumed cesspools that occupy the lower Faga'alu watershed, the average DIN concentrations of the observation points improved by 46%. Thus, upgrading many of the current cesspools in the watershed to septic systems could provide a considerable improvement in future groundwater quality, due to more effective nitrogen attenuation and resulting smaller amounts of nutrients leaching into the coastal waters.
Glyphosate-use scenarios were performed to see the relative contributions from domestic and agricultural inputs. The results show that degradation of GLY may influence which sources contribute the most to the observation sites. According to the model, the domestic inputs, which were modeled with half the initial concentrations as the agricultural zones, delivered a significant amount of the herbicide to the observation points. This indicates that proximity to the stream may be an important factor in glyphosate contamination. Agricultural GLY may be more diluted and degraded before reaching observation points within the village.
Scenarios were thus run with (1) half the domestic input of GLY and (2) with half the total GLY (domestic and agricultural) applied. The first scenario lowered average concentrations by 37% across the watershed, and the second scenario lowered GLY arrival another 13%, to a total of 50%. This shows that improving application techniques or limiting the use of GLY-based herbicides near the stream and coastline could significantly lower the amount of the herbicide in discharging groundwater (Supplementary Table 6). It should be noted that assumptions about application rates, geographical distribution of application, and degradation rates were made. Finally, as mentioned previously, the underlying geology and soil properties may play a role in the attenuation of glyphosate and their heterogeneity may have not been captured in the model.
Potential Threat to the Reef Ecosystem
Glyphosate is the most common ingredient in top-selling herbicides around the world today (e.g., Roundup), and its harmful effects have been discussed, specifically in regards to its carcinogenic nature to humans (Tarazona et al., 2017). There are a few studies that reported on the effects of GLY on aquatic ecosystems (e.g., Diu, 2016; Pérez et al., 2017). According to our study, 880 ± 230 mg/d of GLY is entering Faga'alu Bay, with near-ocean groundwater concentrations reaching values likely similar to those measured in coastal springs (160 to 300 ng/L). In a study by Diu (2016) focusing on coral exposed to different concentrations of GLY, the herbicide was generally found not to have harmful effects on reef fertilization or settlement. Only in contained studies, at concentrations above 690,000 ng/L does GLY affect coral fertilization (Diu, 2016), which is three orders of magnitude higher than what was found in Faga'alu coastal springs. The GLY that enters Faga'alu Bay each day via baseflow and coastal SGD is likely diluted relatively quickly as it enters a large body of water and mixes offshore. Gallagher et al. (1996) found low concentrations of pesticides across coastal sites in Virginia, but detected none in the offshore surface water or sediment samples. The chronic effects of the GLY itself are suggested not to be a factor on reef health, as its dilution and degradation are relatively rapid in open water (Schuette, 1998). With a half-life ranging from 47 to 267 days, depending on the type of microbial communities present (Mercurio et al., 2014), GLY is not known to bioaccumulate in organisms as it has low lipid solubility (Schuette, 1998). Diu (2016), however, found that lower fertilization rates in coral can be attributed to the runoff of Roundup (Monsanto®) into marine environments. Despite GLY itself not being detrimental to reef health, in combination with other chemicals (e.g., Roundup solution), the formulation is likely to present increased toxicity to the reef (Diu, 2016). Thus, monitoring GLY fluxes in stream and across the land-ocean interface from Roundup application may still be of importance in Faga'alu.
The insecticide DDT has a much longer residence time than glyphosate and is able to bioaccumulate in the tissues of organisms. Studies have shown that DDT likely has an effect on the calcification of adult corals and other calcifying organisms in the reef community (Kwok, 2015; Porter et al., 2018), similar to the effects it had on the calcium-related eggshell thickness of bird eggs in early studies (Porter and Wiemeyer, 1969; Peakall, 1970). The fact that 9,000 ± 2,000 mg of DDT is contributed to Faga'alu Bay via stream baseflow and SGD each day, highlights its persistence and ubiquity in the environment decades after its ban (EPA, 2016). Despite that it is no longer sprayed, DDT's long half-life allows it to accumulate in the ecosystem. DDT has the potential to chronically affect growth and reproduction of marine organisms within the reef as its daily load into the bay has spanned several decades, and may continue for several more. This study sheds light on groundwater fluxes of DDT into the bay but it should be noted that it has multiple other pathways. Thanks to its high sorption coefficient DDT sorbs to soil and sediment particles (Magga et al., 2008) and can readily get transported by stream suspended loads and also released by sediment resuspension in the bay. A study in coastal California demonstrated that sediment resuspension resulted in DDT release and contaminant levels in the water column closely correlated to sediment DDT levels (Zeng and Venkatesan, 1999). Studying DDT in tissues of organisms in the future would thus be beneficial to understanding the long-term effects the insecticide is having in Faga'alu Stream and Bay.
Total N entering Faga'alu Bay via baseflow and SGD together is 710 ± 140 g/d and total P is 840 ± 110 g/d. Elevated nutrient concentrations have already been documented in Faga'alu Bay in other studies (Whitall and Holst, 2015; Shuler et al., 2019). In American Samoa, the National Coastal Condition Report (Colianni et al., 2012) determined cut-points for coastal DIN at <500 μg/L, and all groundwater and stream DIN measured in our study were lower than that. For DIP, the cut-point of fair condition is <70 μg/L of P, which is exceeded by all but three stream and groundwater samples. In addition, corals are sensitive to excess nutrients, and levels above 14 μg/L-N (DIN) and 3 μg/L-P can be considered unhealthy for a reef ecosystem, as it may promote more algal growth (Goreau and Thacker, 1994; Mosley et al., 2005). These high levels of nutrients can lead to eutrophication in the bay, upsetting the delicate balance of the reef ecosystem. Excess algae can smother the corals and block sunlight from reaching important photosynthetic organisms, and can promote the spread of harmful bacteria (Coral Reef Alliance, 2019). Thus, in the case of Faga'alu, a reduction in nutrient fluxes could be beneficial to the bay. As this study demonstrates, groundwater quality directly affects coastal nutrient levels, and the nutrient fluxes from baseflow and SGD should be considered when nutrient pathways into the reef are evaluated.
Conclusions
This study further implicates the importance of the role of fresh SGD and baseflow to overall water and contaminant fluxes in coastal aquifers with volcanic geologic properties. By integrating observed groundwater contaminant concentrations with a hydrogeological model of Faga'alu Watershed in American Samoa, relative SGD and baseflow fluxes of pesticides and nutrients were determined for each sector of coastline and reach of stream. Although flow rates modeled in this study are only calibrated against a snapshot of observations, the model-estimated contributions reasonably captured groundwater flow in comparison to a study from a previous dry season (Shuler et al., 2019). The model predicted the central coastline to be contributing the most SGD to Faga'alu Bay and the upper reach of the stream was contributing the most baseflow. Under baseflow conditions SGD flux was 41% of the total fresh groundwater flow to the bay and stream baseflow was responsible for 59%. It is evident that groundwater plays a significant role in water and solute transport to the bay, especially during the dry season of July through September.
Clearly, a more robust modeling approach would be based on year-round observations capturing seasons and extreme weather conditions as well as based on geographically extended hydrogeological observations. In addition, as a next step, a hydrological model capturing the salinity transition zone and total SGD would help further in interpreting nutrient cycling and fluxes across the land-ocean interface.
Field investigation confirmed pesticides to be present in the groundwater, and by combining their measured concentrations with model-derived groundwater discharge, fluxes across the land-ocean interface were slightly <1 g of GLY to the bay each day and nearly 9 g/d of DDT. The continued flux of these chemicals could have chronic effects on reef health and should be investigated in more detail. Nutrient fluxes were 710 ± 140 g of TDN and 840 ± 110 g of DIP per day where 90% of TDN was estimated to be derived from OSDS. Although concentrations of N at any individual sampling site did not exceed EPA recommended values, concentrations of DIP were above the acceptable limits (Oram, 2014b) in many locations.
Better land-use practices, specifically reducing the amount of applied pesticides and improving the wastewater infrastructure can help reduce the leaching of pesticides and nutrients into groundwater. Models like the one developed here but perfected with more observations could be applied for optimization of elimination of pollution sources that have the largest impact. As Faga'alu has been designated a priority watershed in monitoring reef health, it is important to consider not only contaminants entering the bay via surface pathways, but also as demonstrated by this study, to consider groundwater transport via SGD and stream baseflow as pathways for pesticides and nutrients into the bay.
Data Availability Statement
All datasets generated for this study are included in the manuscript/Supplementary Files.
Author Contributions
HD and AE-K contributed conception and design of the study. EW and CS designed and performed the field-based aspects of the study. HD and EW performed the laboratory analysis. AE-K and EW developed the hydrological models. EW wrote the first draft of the manuscript. HD, AE-K, and CS wrote sections of the manuscript. All authors contributed to manuscript revision, read and approved the submitted version.
Funding
Funding for this project was provided to HD by the USGS Water Resources Research Institute Program (WRRIP) project number 2016AS455B and the Hawai'i EPSCoR Program funded by the National Science Foundation's Research Infrastructure Improvement (RII) OIA-1557349, to AE-K from the Pacific Regional Integrated Sciences and Assessments (Pacific RISA), NOAA Climate Program Office project number NA15OAR4310146, and to EW from the University of Hawaii Undergraduate Research Opportunities Program (UROP).
Conflict of Interest
The authors declare that the research was conducted in the absence of any commercial or financial relationships that could be construed as a potential conflict of interest.
Acknowledgments
The authors acknowledge continued support from the American Samoa Environmental Protection Agency, the American Samoa Power Authority, the NOAA AS office, American Samoa Community College, and the Coral Reef Advisory Group for providing essential logistical assistance on Tutuila and enabling the field component of this work. The authors would also like to thank the village of Faga'alu, the Pulenuu of Faga'alu, and others who generously provided access to study sites. We would like to thank Dr. Mark Schmaedick and Dr. Ian Gurr at the American Samoa Community College Land Grant for their time and expertise when developing research methods used in this study. We thank Drs. Olkeba Tolessa Leta, Trent Biggs, and Alex Messina for collaboration support, advice, and feedback at various stages of the study. This is contributed paper WRRC-CP-2020-04 of the Water Resources Research Center, and SOEST publication # 10802 at the University of Hawaii at Manoa, Honolulu, Hawaii.
Supplementary Material
The Supplementary Material for this article can be found online at: https://www.frontiersin.org/articles/10.3389/fenvs.2019.00162/full#supplementary-material
References
Almasri, M. N. (2008). Assessment of intrinsic vulnerability to contamination for Gaza coastal aquifer, Palestine. J. Environ. Manage. 88, 577–593. doi: 10.1016/j.jenvman.2007.01.022
Amato, D. W., Bishop, J. M., Glenn, C. R., Dulai, H., and Smith, C. M. (2016). Impact of submarine groundwater discharge on marine water quality and reef biota of Maui. PLoS ONE 11:e0165825. doi: 10.1371/journal.pone.0165825
Bear, J. (1979). Hydraulics of Groundwater, McGraw-Hill Series in Water Resources and Environmental Engineering. New York, NY: McGraw-Hill.
Benbrook, C. M. (2016). Trends in glyphosate herbicide use in the United States and globally. Environ. Sci. Eur. 28:3. doi: 10.1186/s12302-016-0070-0
Bolabola, A. (2007). “Pollution a growing concern in Fiji Waters,” in Pacific Islands Report, Pacific Islands Development Program. Available online at: www.pireport.org/articles/2007/07/05/pollution-growing-concern-fiji-waters.
Bruno, J. F., Petes, L. E., Harvell, C. D., and Hettinger, A. (2003). Nutrient enrichment can increase the severity of coral diseases. Ecol. Lett. 6, 1056–1061. doi: 10.1046/j.1461-0248.2003.00544.x
Buddemeier, R. W. (1996). “Groundwater flux to the ocean: definitions, data, applications, uncertainties,” in LOICZ Reports Studies 8, 16–21.
Burnett, W. C., and Dulaiova, H. (2003). Estimating the dynamics of groundwater input into the coastal zone via continuous radon-222 measurements. J. Environ. Radioact. 69, 21–35. doi: 10.1016/S0265-931X(03)00084-5
Chin, Y.-P., and Weber, W. J. (1988). The Interaction of Hydrophobic Organic Compounds with Natural Solids and Dispersed Organic Polymers (ProQuest Dissertations and Theses). Web.
Colianni, G., Aquarone, M., Balthis, L., Bourgeois, P., Casey, J., Cooksey, C., et al. (2012). National Coastal Condition Report IV. Environmental Protection Agency, Office of Water and Office of Research and Development. Available online at: https://www.epa.gov/sites/~production/files/2014-10/documents/0_nccr_4_report_508_bookmarks.pdf
Coral Reef Alliance (2019). Clean Water for Reefs. Available online at: https://coral.org/what-we-do/clean-water-for-reefs/.
Craig, P. (2002). Natural History Guide to American Samoa: A Collection of Articles. Pago Pago, American Samoa: National Park Service and the Dept. of Marine and Wildlife Resources.
Craig, P., DiDonato, G., Fenner, D., and Hawkins, C. (2005). “The state of coral reef ecosystems of American Samoa,” in The State of Coral Reef Ecosystems of the United States and Pacific Freely Associated States, ed J. Waddell (Silver Spring, MD: NOAA Technical Memorandum NOS NCCOS 11. NOAA/NCCOS Center for Coastal Monitoring and Assessment's Biogeography Team, 312–337.
Davidson, J. H. (1995). South Dakota Groundwater Protection Law. University of Arkansas System Division of Agriculture.
Davis, D. A. (1963). Ground-Water Reconnaissance of American Samoa. Washington, DC: US Government Printing Office.
Diu, S. (2016). Trouble in Paradise: The Effect of Glyphosate and Roundup Toxicity on Coral Fertilization and Settlement (Master's thesis). Princeton University. Available online at: https://dataspace.princeton.edu/jspui/handle/88435/dsp01sj139440v
Doherty, J. E., and Hunt, R. J. (2010). Approaches to Highly Parameterized Inversion: A Guide to Using PEST for Groundwater-Model Calibration. Reston, VA: US Department of the Interior; US Geological Survey. doi: 10.3133/sir20105169
Dulai, H., Kleven, A., Ruttenberg, K., Briggs, R., and Thomas, F. (2016). “Evaluation of submarine groundwater discharge as coastal nutrient source and its role in coastal groundwater quality and quantity,” in Emerging Issues in Groundwater Resources, Advances in Water Security, ed A. Fares (Springer International Publishing), 187–221. doi: 10.1007/978-3-319-32008-3_8
Dulaiova, H., Burnett, W. C., Wattayakorn, G., and Sojisuporn, P. (2006). Are groundwater inputs into river-dominated areas important? The Chao Phraya River—Gulf of Thailand. Limnol. Oceanogr. 51, 2232–2247. doi: 10.4319/lo.2006.51.5.2232
El-Kadi, A., and Yabusaki, K. (1996). Assessment of Nutrient Use and Nitrate Contamination in Central Oahu, Hawai‘i. Water Resources Research Center, University of Hawai‘i at Mānoa. Honolulu, HI: WRRC unedited report.
EPA (1993). Reregistration Eligibility Decision (RED): Glyphosate; EPA-738-R-93-014. Washington, DC: U.S. Environmental Protection Agency, Office of Prevention, Pesticides, and Toxic Substances, Office of Pesticide Programs, U.S. Government Printing Office.
EPA (2009). National Primary Drinking Water Regulations. Environmental Protection Agency, Office of Groundwater and Drinking Water, 816-F-09-004. Available online at: https://www.epa.gov/sites/production/files/2016-06/documents/npwdr_complete_table.pdf.
EPA (2016). DDT- A Brief History and Status. Environmental Protection Agency. Available online at: https://19january2017snapshot.epa.gov/ingredients-used-pesticide-products/ddt-brief-history-and-status_html.
Erler, D. V., Shepherd, B., Linsley, B., and Lough, J. M. (2018). Coral skeletons record increasing agriculture-related groundwater nitrogen inputs to a South Pacific reef over the past century. Geophys. Res. Lett. 45, 8370–8378. doi: 10.1029/2018GL078656
Gallagher, D. L., Dietrich, A. M., Reay, W. G., Hayes, M. C., and Simmons, G. M. Jr. (1996). Ground water discharge of agricultural pesticides and nutrients to estuarine surface water. Groundwater Monit. Remediat. 16, 118–129. doi: 10.1111/j.1745-6592.1996.tb00579.x
Gingerich, S. B., and Engott, J. A. (2012). Groundwater Availability in the Lahaina District, West Maui, Hawai'i. U.S. Geological Survey Report. doi: 10.3133/sir20125010
Glenn, C. R., Whittier, R. B., Dailer, M. L., Dulaiova, H., El-Kadi, A. I., Fackrell, J., et al. (2013). Lahaina Groundwater Tracer Study–Lahaina, Maui, Hawaii. Final Report, Honolulu, HI: Hawaii DOH.
Goreau, T. J., and Thacker, K. (1994). “Coral Reefs, Sewage, and Water Quality Standards,” in Caribbean Water and Wastewater Association Conference (Kingston).
Harbaugh, A. W., Banta, E. R., Hill, M. C., and McDonald, M. G. (2000). MODFLOW-2000, The U. S. Geological Survey Modular Ground-Water Model-User Guide to Modularization Concepts and the Ground-Water Flow Process. Open-file Report. U. S. Geological Survey 92, 134. doi: 10.3133/ofr200092
Henderson, A. M., Gervais, J. A., Luukinen, B., Buhl, K., Stone, D., Strid, A., et al. (2010). Glyphosate Technical Fact Sheet. Corvallis, OR: National Pesticide Information Center; Oregon State University Extension Services.
Hosono, T., Ono, M., Burnett, W. C., Tokunaga, T., Taniguchi, M., and Akimichi, T. (2012). Spatial distribution of submarine groundwater discharge and associated nutrients within a local coastal area. Environ. Sci. Technol. 46, 5319–5326. doi: 10.1021/es2043867
Houk, P., Didonato, G., Iguel, J., and Van Woesik, R. (2005). Assessing the effects of non-point source pollution on American Samoa's coral reef communities. Environ. Monit. Assess. 107, 11–27. doi: 10.1007/s10661-005-2019-4
Huhta, C., and Sloat, J. (2007). Discharge Uncertainty Calculations Using a SonTek FlowTracker. SonTek/YSI Inc. Available online at: www.sontek.com.
Izuka, S. K., Perreaultm, J. A., and Presley, T. K. (2007). Areas Contributing Recharge to Wells in the Tafuna-Leone Plain, Tutuila, American Samoa. No. 2007-5167. Geological Survey (US).
Johannes, R. E., and Hearn, C. J. (1985). The effect of submarine groundwater discharge on nutrient and salinity regimes in a coastal lagoon off Perth, Western Australia. Estuarine Coast. Shelf Sci. 21, 789–800. doi: 10.1016/0272-7714(85)90073-3
Kelly, J. L., Dulai, H., Glenn, C. R., and Lucey, P. G. (2019). Integration of aerial infrared thermography and in situ radon-222 to investigate submarine groundwater discharge to Pearl Harbor, Hawaii, USA. Limnol. Oceanogr. 64, 238–257 doi: 10.1002/lno.11033
Kennedy, J. (2012). A Closer Look at Parameter Estimation (PEST) for Visual MODFLOW Flex 2012.2. Waterloo Hydrogeologic, Nova Metrix, LLC. Available online at: www.waterloohydrogeologic.com/2012/12/05/a-closer-look-at-parameter-estimation-pest-for-visual-modflow-flex-20122/.
Khan, A., Umar, R., and Khan, H. H. (2015). Significance of silica in identifying the processes affecting groundwater chemistry in parts of Kali watershed, Central Ganga Plain, India. Appl. Water Sci. 5, 65–72. doi: 10.1007/s13201-014-0164-z
Kim, G., Lee, K. K., Park, K. S., Hwang, D. W., and Yang, H. S. (2003). Large submarine groundwater discharge (SGD) from a volcanic island. Geophys. Res. Lett. 30:2098. doi: 10.1029/2003GL018378
Kwok, C. K. (2015). Spatial and temporal signatures of heavy metals in Hong Kong corals and the responses of their life history stages to heavy metal and organic pollutions (Diss). The Chinese University of Hong Kong, Hong Kong.
Lallanilla, M. (2019). What is the Story About the Banned Pesticide DDT? The Spruce, Dotdash. Availabale online at: www.thespruce.com/what-is-ddt-history-impacts-1708897.
Larkin, R. G. (1988). Hydrogeologic Controls on Underflow in Alluvial Valleys: Implications for Texas Water Law.
Leta, O. T., Dulai, H., El-Kadi, A. I., Messina, A. M., and Biggs, T. W. (2017). “Assessing sediment yield and the effect of best management practices on sediment yield reduction for Tutuila island, American Samoa,” in Final Paper # H31H-1600, Presented at the 2017 AGU Fall Meeting (New Orleans, LA).
Magga, Z., Tzovolou, D. N., Theodoropoulou, M. A., Dalkarani, T., Pikios, K., and Tsakiroglou, C. D. (2008). Soil column experiments used as a means to assess transport, sorption, and biodegradation of pesticides in groundwater. J. Environ. Sci. Health Part B 43, 732–741. doi: 10.1080/03601230802388868
McCormick, G. R. (2017). Water quality and sources of nutrient loads in watersheds of American Samoa (Dissertation). San Diego State University, San Diego, CA, United States.
McDonald, M. G., and Harbaugh, A. W. (1988). A Modular Three-Dimensional Finite-Difference Ground-Water Flow Model. Vol. 6. Reston, VA: US Geological Survey.
Mercurio, P., Flores, F., Mueller, J. F., Carter, S., and Negri, A. P. (2014). Glyphosate persistence in seawater. Marine Pollut. Bull. 85, 385–390. doi: 10.1016/j.marpolbul.2014.01.021
Messina, A. M., and Biggs, T. W. (2016). Contributions of human activities to suspended sediment yield during storm events from a small, steep, tropical watershed. J. Hydrol. 538, 726–742. doi: 10.1016/j.jhydrol.2016.03.053
Miller, M. P., Buto, S. G., Susong, D. D., and Rumsey, C. (2016). The importance of base flow in sustaining surface water flow in the Upper Colorado River Basin. Water Resour. Res. 52, 3547–3562. doi: 10.1002/2015WR017963
Misa, M. M., and Vargo, A. M. (1993). “Indigenous agroforestry in American Samoa,” in Proceedings of the Workshop on Research Methodologies and Applications for Pacific Island Agroforestry; July 16-20, 1990; Kolonia, Pohnpei, Federated States of Micronesia. Gen. Tech. Rep. PSW-GTR-140, Vol. 140, eds B. Raynor, and R. R. Bay (Pacific Southwest Research Station, Forest Service, US Department of Agriculture), 83.
Mosley, L., Singh, S., and Aalbersberg, B. (2005). Water Quality Monitoring in Pacific Island Countries. SOPAC Technical Reports 381, 42.
Mosley, L. M., and Aalbersberg, W. G. L. (2003). Nutrient levels in sea and river water along the ‘Coral Coast'of Viti Levu, Fiji. S. Pac. J. Nat. Appl. Sci. 21, 35–40. doi: 10.1071/SP03007
Mulligan, A. E., and Charette, M. A. (2006). Intercomparison of submarine groundwater discharge estimates from a sandy unconfined aquifer. J. Hydrol. 327, 411–425. doi: 10.1016/j.jhydrol.2005.11.056
NOAA/EPA (2003). American Samoa Coastal Nonpoint Program NOAA/EPA Decisions on Conditions of Approval. American Samoa Decision Document.
NPIC Oregon State University (1999). DDT [General Fact Sheet]. Retrieved from: http://npic.orst.edu/factsheets/ddtgen.pdf.
Nyström, M., Folke, C., and Moberg, F. (2000). Coral reef disturbance and resilience in a human-dominated environment. Trends Ecol. Evol. 15, 413–417. doi: 10.1016/S0169-5347(00)01948-0
Oki, D. S. (2005). Numerical Simulation of the Effects of Low-Permeability Valley-Fill Barriers and the Redistribution of Ground-Water Withdrawals in the Pearl Harbor Area, Oahu, Hawaii. U.S. Geological Survey Scientific Investigations Report 2005-5253, 111. doi: 10.3133/sir20055253
Oram, B. (2014a). Phosphates in the Environment. Water Research Center, Water Research Watershed Center. Available online at: www.water-research.net/index.php/glossary.
Oram, B. (2014b). Water Quality Terms Glossary. Water Research Center, Water Research Watershed Center. Available online at: www.water-research.net/index.php/glossary.
Oregon Department of Human Services (2000). Ammonia- Health Effects Information. Environmental Toxicology Section, Office of Environmental Public Health, Technical Bulletin. Available online at: https://www.oregon.gov/oha/PH/HealthyEnvironments/DrinkingWater/Monitoring/Documents/health/ammonia.pdf.
Peakall, D. B. (1970). p, p'-DDT: Effect on calcium metabolism and concentration of estradiol in the blood. Science 168, 592–594. doi: 10.1126/science.168.3931.592
Pérez, D. J., Okada, E., Menone, M. L., and Costa, J. L. (2017). Can an aquatic macrophyte bioaccumulate glyphosate? Development of a new method of glyphosate extraction in Ludwigia peploides and watershed scale validation. Chemosphere 185, 975–982. doi: 10.1016/j.chemosphere.2017.07.093
Polidoro, B. A., Comeros-Raynal, M. T., Cahill, T., and Clement, C. (2017). Land-based sources of marine pollution: Pesticides, PAHs and phthalates in coastal stream water, and heavy metals in coastal stream sediments in American Samoa. Marine Pollut. Bull. 116, 501–507. doi: 10.1016/j.marpolbul.2016.12.058
Porder, S., and Ramachandran, S. (2013). The phosphorus concentration of common rocks—a potential driver of ecosystem P status. Plant Soil 367, 41–55. doi: 10.1007/s11104-012-1490-2
Porter, R. D., and Wiemeyer, S. N. (1969). Dieldrin and DDT: effects on sparrow hawk eggshells and reproduction. Science 165, 199–200. doi: 10.1126/science.165.3889.199
Porter, S. N., Humphries, M. S., Buah-Kwofie, A., and Schleyer, M. H. (2018). Accumulation of organochlorine pesticides in reef organisms from marginal coral reefs in South Africa and links with coastal groundwater. Marine Pollut. Bull. 137, 295–305. doi: 10.1016/j.marpolbul.2018.10.028
Pradeep, K., Nepolian, M., Anandhan, P., Chandran Kaviyarasan, R., Prasanna, M. V., et al. (2016). “A study on variation in dissolved silica concentration in groundwater of hard rock aquifers in Southeast coast of India,” in IOP Conference Series: Materials Science and Engineering, Vol. 121. No. 1. (IOP Publishing). doi: 10.1088/1757-899X/121/1/012008
Rendón-von Osten, J., and Dzul-Caamal, R. (2017). Glyphosate residues in groundwater, drinking water and urine of subsistence farmers from intensive agriculture localities: a survey in Hopelchén, Campeche, Mexico. Int. J. Environ. Res. Public Health 14:595. doi: 10.3390/ijerph14060595
Richardson, C. M., Dulai, H., and Whittier, R. B. (2015). Sources and spatial variability of groundwater-delivered nutrients in Maunalua Bay, O'ahu, Hawai ‘i. J. Hydrol. 11, 178–193. doi: 10.1016/j.ejrh.2015.11.006
Rodellas, V., Garcia-Orellana, J., Masqué, P., Feldman, M., and Weinstein, Y. (2015). Submarine groundwater discharge as a major source of nutrients to the Mediterranean Sea. Proc. Nat. Acad. Sci. 112, 3926–3930. doi: 10.1073/pnas.1419049112
Sanchis, J., Kantiani, L., Llorca, M., Rubio, F., Ginebreda, A., Fraile, J., et al. (2012). Determination of glyphosate in groundwater samples using an ultrasensitive immunoassay and confirmation by on-line solid-phase extraction followed by liquid chromatography coupled to tandem mass spectrometry. (Report). Anal. Bioanal. Chem. 402, 2335–2345. doi: 10.1007/s00216-011-5541-y
Shuler, C. K., Amato, D. W., Gibson, V., Baker, L., Olguin, A. N., Dulai, H., et al. (2019). Assessment of terrigenous nutrient loading to coastal ecosystems along a human land-use gradient, Tutuila, American Samoa. Hydrogeology 6:18. doi: 10.3390/hydrology6010018
Shuler, C. K., El-Kadi, A., Dulaiova, H., Glenn, C. R., and Fackrell, J. K. (2017). Source partitioning of anthropogenic groundwater nitrogen in a mixed-use landscape, Tutuila, American Samoa. Hydrogeol. J. 25, 2419–2434. doi: 10.1007/s10040-017-1617-x
Stearns, H. T. (1944). Geology of the Samoan Islands. GSA Bull. 55, 1279–1332. doi: 10.1130/GSAB-55-1279
Stewart, B. T., Bryan, K. R., Pilditch, C. A., and Santos, I. R. (2018). Submarine groundwater discharge estimates using radium isotopes and related nutrient inputs into Tauranga Harbour (New Zealand). Estuaries Coasts 41, 384–403. doi: 10.1007/s12237-017-0290-6
Tang, B., Boënne, W., Desmet, N., Seuntjens, P., Bronders, J., and van Griensven, A. (2015). Quantification and characterization of glyphosate use and loss in a residential area. Sci. Total Environ. 517, 207–214. doi: 10.1016/j.scitotenv.2015.02.040
Tarazona, J. V., Court-Marques, D., Tiramani, M., Reich, H., Pfeil, R., Istace, F., et al. (2017). Glyphosate toxicity and carcinogenicity: a review of the scientific basis of the European Union assessment and its differences with IARC. Arch. Toxicol.91, 2723–2743. doi: 10.1007/s00204-017-1962-5
Travis, B. V., Maple, J. D., Hurlbut, H. S., and Husman, C. N. (1946). Cub airplanes in the South Pacific for application of DDT. J. Econ. Entomol. 39, 726–728. doi: 10.1093/jee/39.6.726
Tuitele, C., Buchan, E. L., Regis, J., Wiles, P., and Ilaoa, N (2014). Territory of American Samoa Integrated Water Quality Monitoring and Assessment Report. Hawaii: American Samoa Environmental Protection Agency, Pago Pago, American Samoa, Nimbus Environmental Services.
Van Stempvoort, D. R., Roy, J. W., Brown, S. J., and Bickerton, G. (2014). Residues of the herbicide glyphosate in riparian groundwater in urban catchments. Chemosphere 95, 455–463. doi: 10.1016/j.chemosphere.2013.09.095
Van Stempvoort, D. R., Spoelstra, J., Senger, N. D., Brown, S. J., Post, R., and Struger, J. (2016). Glyphosate residues in rural groundwater, Nottawasaga River Watershed, Ontario, Canada. Pest Manage. Sci. 72, 1862–1872. doi: 10.1002/ps.4218
Vaouli, E., Tuiteli, C., Buchan, E. L., Regis, J., Wiles, P., and Ilaoa, N. (2010). Territory of American Samoa Integrated Water Quality Monitoring and Assessment Report 2010. ASEPA.
Vargas-Angel, B., and Schumacher, B. (2018). Baseline Surveys for Coral Reef Community Structure and Demographics in Vatia and Faga'alu Bay, American Samoa. NOAA.
Whitall, D. R., and Holst, S. (2015). Pollution in Surface Sediments in Faga'alu Bay, Tutuila, American Samoa. NOAA Technical Memorandum NOS NCCOS 201. Silver Spring, MD, 54. doi: 10.7289/V5/TM-NOS-NCCOS-201
Whittier, R., Rotzoll, K., Dhal, S., El-Kadi, A. I., Ray, C., and Chang, D. (2010). Groundwater source assessment program for the state of Hawaii, USA: Methodology and example application. Hydrogeology 18, 711–723. doi: 10.1007/s10040-009-0548-6
Zeng, E. Y., and Venkatesan, M. I. (1999). Dispersion of sediment DDTs in the coastal ocean off southern California. Sci. Total Environ. 229, 195–208. doi: 10.1016/S0048-9697(99)00064-9
Zennaro, B. (2007). Regulating illegal piggery waste runoff. ArcNews Online. ESRI. Available online at: https://www.esri.com/news/arcnews/fall07articles/regulating-illegal.html.
Zhang, P., Aagaard, P., Nadim, F., Gottschalk, L., and Haarstad, K. (2009). Sensitivity analysis of pesticides contaminating groundwater by applying probability and transport methods. Integr. Environ. Assess. Manage. 5, 414–425. doi: 10.1897/IEAM_2008-087.1
Zheng, C., Hill, M. C., Cao, G., and Ma, R. (2012). MT3DMS: model use, calibration, and validation. Trans. ASABE 55, 1549–1559. doi: 10.13031/2013.42263
Keywords: submarine groundwater discharge, stream baseflow, pesticides, nutrients, water quality, hydrological model, groundwater hydrology
Citation: Welch EM, Dulai H, El-Kadi A and Shuler CK (2019) Submarine Groundwater Discharge and Stream Baseflow Sustain Pesticide and Nutrient Fluxes in Faga'alu Bay, American Samoa. Front. Environ. Sci. 7:162. doi: 10.3389/fenvs.2019.00162
Received: 29 June 2019; Accepted: 30 September 2019;
Published: 17 October 2019.
Edited by:
Alberto Tiraferri, Politecnico di Torino, ItalyReviewed by:
Jordi Garcia-Orellana, Autonomous University of Barcelona, SpainHenry Bokuniewicz, The State University of New York (SUNY), United States
Copyright © 2019 Welch, Dulai, El-Kadi and Shuler. This is an open-access article distributed under the terms of the Creative Commons Attribution License (CC BY). The use, distribution or reproduction in other forums is permitted, provided the original author(s) and the copyright owner(s) are credited and that the original publication in this journal is cited, in accordance with accepted academic practice. No use, distribution or reproduction is permitted which does not comply with these terms.
*Correspondence: Henrietta Dulai, hdulaiov@hawaii.edu