Responses of Phytoplanktonic Chlorophyll-a Composition to Inorganic Turbidity Caused by Mine Tailings
- 1Laboratory of Aquatic Ecology, Department of Biology, Institute of Biology, Federal University of Juiz de Fora, Juiz de Fora, Brazil
- 2Laboratory of Limnology, Department of Ecology, Institute of Biology, Federal University of Rio de Janeiro, Rio de Janeiro, Brazil
- 3Laboratory of Plankton, Department of Zoology, Institute of Biology, Federal University of Juiz de Fora, Juiz de Fora, Brazil
Inorganic turbidity can limit light penetration in water and reduce phytoplankton photosynthesis. Anthropogenic activities such as mining can produce or augment the amount of suspended inorganic particles in water. Recent mining disasters in Brazil have released tons of mine tailings into aquatic ecosystems, with known and unknown negative consequences for aquatic life, biodiversity, and ecosystem services beyond the human and material losses. Here, we investigated the effects of inorganic turbidity on phytoplankton chlorophyll content and composition caused by sediments from two areas in Lake Batata, one natural and the other impacted by bauxite tailings. We experimentally compared the effects of different levels of turbidity (12, 50, and 300 NTU) caused by the addition of sediments from the two lake areas on a chlorophyll-a gradient (5, 15, and 25 μg/L). Inorganic turbidity did not consistently reduce chlorophyll-a concentrations. In treatments with high chlorophyll-a, high turbidity was associated with lower chlorophyll-a concentrations at the end of the experiment. On the other hand, in low-chlorophyll treatments, high turbidity was associated with higher chlorophyll-a concentrations. In treatments with sediments from the natural area, overall chlorophyll-a levels were higher than in treatments with sediments from the impacted area. Phagotrophic algae dominated both in treatments with sediments from the impacted area (Chrysophyceae 34%, Chlorophyceae 26%, and Cyanobacteria 22% of total density) and in treatments with sediment from the natural area (Euglenophyceae 26%, Chrysophyceae 23%, and Chlorophyceae 20%). We conclude that high turbidity does not lead to a reduction in chlorophyll-a concentrations and sediment from the natural area allowed higher chlorophyll-a levels, indicating that impacted area sediment affected more phytoplankton.
1 Introduction
Turbidity is an important feature of aquatic ecosystems. It can be caused by dissolved organic matter or suspended particles in the water column and is usually measured by lateral dispersion (90°) of an incident light beam. Turbidity is often considered an aquatic environmental stressor because it directly modifies the scattering, absorption, and attenuation of light penetration in water, thereby affecting primary production and aquatic metabolism (Kirk, 1985). Suspended solids responsible for increasing water turbidity can derive from natural processes such as glacier melting (Dierssen et al., 2002), cyanobacterial blooms or high densities of planktonic microalgae (more often in shallow lakes) (Descy et al., 2013), and sediment resuspension by wind, benthivorous fish, or lateral flooding (Donohue and Garcia-Molinos, 2009). Recent studies suggest that climate warming can intensify turbidity by increasing evaporation rates and reducing lake depths (Jeppesen et al., 2014; Menezes et al., 2019). This tendency, together with seasonal variation in freshwater ecosystems, can exacerbate turbidity variation, creating larger range gradients, especially on floodplains (Sorribas et al., 2016).
Turbid waters (e.g., with suspended organic or inorganic solids) are often associated with low aesthetic value, limited recreational use, pollution, disease transmission, and human health impact, with increased treatment costs for consumption (Davies-Colley and Smith, 2001). Nowadays, inorganic turbidity is considered one of the most important aquatic pollutants (Parkhill and Gulliver, 2002), including turbidity generated by anthropogenic activities (Bentley et al., 2016). Anthropogenic interventions in the landscape increase soil erosion and sediment transport to freshwater environments, which are among the most threatened ecosystems in the world (Malmqvist and Rundle, 2002; Cantonati et al., 2020). Some examples of anthropogenic alterations are agricultural expansion, the development of large industries, and mining activities. These activities can alter sediment dynamics and transport to rivers and lakes, profoundly altering drainage basins (de Jong et al., 1995). One of the first large-scale mining environmental impacts known in Brazil is the discharge of 25,000 m³ of bauxite tailings daily for 10 years (1979–1989) in Lake Batata; a restoration project was initiated in 1989 (Esteves et al., 1990). Recently, large mining disasters in Brazil have released tons of waste into aquatic ecosystems. In 2015, a dam maintained by the company Samarco Mineração S. A. collapsed in the city of Mariana, Minas Gerais state, discharging approximately 60 million m³ of iron waste that spread along 660 km of the Doce River. A thick layer with low nutritional value was created along the river, increasing heavy-metal concentrations and causing silting, animal mortality, material damage, and loss of human lives (IBAMA, 2015; Grilo et al., 2018). In 2019, another dam owned by Vale S. A. collapsed in the city of Brumadinho, also in Minas Gerais. This accident released 12 million m³ of iron ore tailings into the Paraopeba River and resulted in severe environmental effects and even more loss of life (Thompson et al., 2020).
The effects of mine tailings on aquatic environments can differ from those of natural sediments. For example, tailings from the Mariana dam collapse have been linked to increased cytogenotoxicity (Quadra et al., 2018) and potential risk of DNA damage (Segura et al., 2016). In the plankton, the harmful effects of tailings may also be related to the fine particles (smaller than 50 µm) that according to laboratory tests tend to remain in suspension for long periods (Roland and Esteves, 1998). Since the inorganic particulate matter that composes tailings has low nutritional value and hinders zooplankton filtering (Levine et al., 2005), the tailings may reduce zooplankton density, reproduction, and feeding rates (Bilotta and Brazier, 2008). Although turbidity may be capable of structuring bacterioplankton communities (Sánchez et al., 2017), inorganic turbidity caused by tailings is not necessarily harmful as it can form aggregates with particulate material and become important sources of nitrogen and phosphorus for bacteria, considerably changing the trophic dynamics in turbid environments (Gerbersdorf and Wieprecht, 2015). However, because transparency decreases, turbidity caused by suspended tailings can affect visually oriented species, for example increasing predation effort by visually oriented planktivorous fish (Lin and Pellegrini Caramaschi, 2005), reducing predation on zooplankton and favoring an increase in their density, which in turn can affect primary producers through trophic-cascade effects (Sweka and Hartman, 2001; Liljendahl-Nurminen et al., 2008).
Another major effect of suspended material is light attenuation in the water (Izaguirre et al., 2012), with subsequent loss of phytoplankton photosynthesis (Lind et al., 1992; Phlips et al., 1995). In addition to reducing phytoplankton density and growth rate, light attenuation can also modify communities by promoting the prevalence of resistant species (Kruk and Segura, 2012; Bortolini et al., 2014). In environments that become artificially turbid, the phytoplankton composition may shift toward algae groups that are better adapted to low light conditions, such as Microcystis aeruginosa, which can outcompete green algae for light (Yang and Jin, 2008). In other examples, Cyanobacteria dominance shifted toward Cryptophytes in a mesocosm with turbidity from glacial flour (Cianci-Gaskill et al., 2020), and in turbid shallow lakes, diatoms such as Fragilaria or Cyclotella may dominate (Izaguirre et al., 2015). In general, large groups of diatoms can be resuspended together with sediments by wind action, and are likely to be selected in shallow, shaded environments (Crossetti et al., 2012). These are often classified in Kruk’s morpho-functional Group V, which is composed mainly of mixotrophic or phagotrophic unicellular flagellates. These organisms can compete better in turbid environments since they do not depend exclusively on light availability to acquire organic carbon (Kruk et al., 2010; Costa et al., 2019).
Turbid environments can vary widely in turbidity over time, due to seasonal environmental changes, which can also affect plankton distribution, generating gradient patterns. Considering that the effects of turbidity on primary production depend on the concentration and type of suspended particles, and also that both natural and anthropogenic turbidity are increasing in aquatic ecosystems, it is crucial to understand their role in fundamental processes such as phytoplankton primary production. By combining different levels of chlorophyll-a with different levels of inorganic turbidity, we experimentally tested the following hypotheses: 1) chlorophyll contents will be higher in treatments with lower inorganic turbidity; 2) high inorganic turbidity will promote a shift in phytoplankton composition by selecting species adapted to low light conditions; and 3) turbidity caused by inorganic sediments from an area impacted by mine tailings will have a stronger negative effect on chlorophyll concentration than will turbidity caused by natural sediments.
2 Materials and Methods
2.1 Experimental Design
The experiment was conducted in the Laboratory of Aquatic Ecology (UFJF, Minas Gerais, Brazil) with sediment originally collected in Lake Batata, an Amazonian floodplain lake in the state of Pará, Brazil. The sediments were collected from the natural area (non-impacted) and the impacted area (with remaining bauxite tailings). Lake Batata was chosen as a model ecosystem because of its historical impact from bauxite tailings, as described below. The water and phytoplankton community evaluated in the experiment were collected from a lake in the UFJF Botanical Garden, also described below.
Before the experiment, the water collected in the Botanical Garden lake was incubated for 24 h in a thermostated cabinet (WTW model TS 606/2-i) at a constant temperature of 18°C, the mean temperature of the region (Moreira, 2014). This procedure was required to keep the aquatic communities acclimated since changes in the original temperature could modify plankton metabolism and affect the experimental results. After the 24-h acclimation period, the water samples were divided to make stock solutions for treatment setup. The stock solutions received three different quantities of sediment according to the desired turbidity level. This procedure was conducted for both types of sediments in Lake Batata, i.e., from the impacted area (Impacted Batata, IB) and the non-impacted area (Natural Batata, NB). For individual treatments, we used 500-ml beakers filled with 400 ml of stock solution and covered with aluminum foil.
For each type of sediment (IB and NB), the experimental treatments were assembled to test the effects of three levels of inorganic turbidity: High (HT), Medium (MT), and Low (LT), and three levels of chlorophyll-a: High (HC), Medium (MC), and Low (LC). Three controls contained only High chlorophyll-a (HC-C), Medium chlorophyll-a (MC-C), or Low chlorophyll-a (LC-C) (Figure 1). Treatments were labeled by turbidity level, followed by chlorophyll-a level. For example, the treatment with high turbidity (HT) and high chlorophyll-a (HC) was labeled “HTHC”. This coding is used throughout the text. Each treatment was conducted in triplicate, including controls.
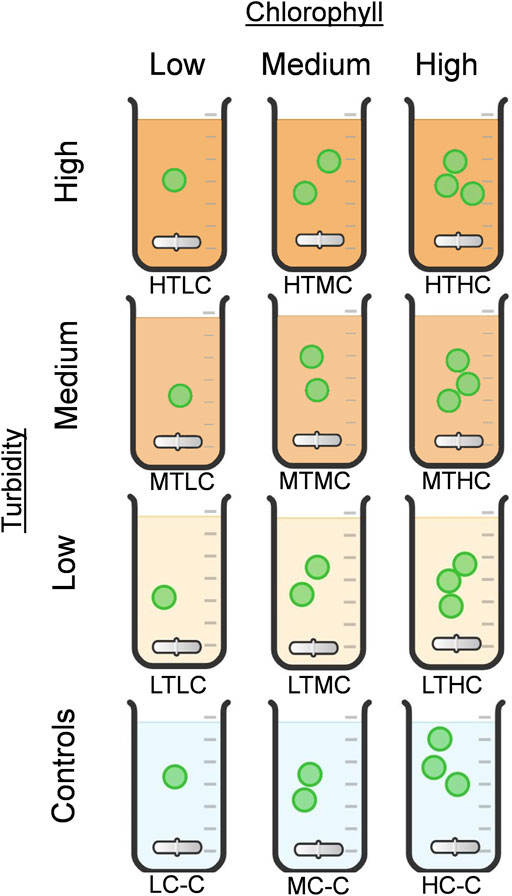
FIGURE 1. Experimental design: combinations of three chlorophyll levels (Low, Medium, High) and turbidity (Low, Medium, High), forming nine categories of triplicate treatments including controls. HT: High Turbidity; MT: Medium Turbidity; LT: Low Turbidity; HC: High Chlorophyll; MC: Medium Chlorophyll; LC: Low Chlorophyll.
Turbidity treatments were standardized to represent the three levels: HT = 300 NTU, MT = 50 NTU, and LT = 12 NTU. These values correspond to the highest, median, and lowest values historically recorded in Lake Batata (MRN, 2018). Turbidity was measured by an Instrutherm TD-300 turbidimeter. The chlorophyll-a levels were established as follows. The “high chlorophyll-a” threshold was based on the maximum chlorophyll-a level reached in the water at the Botanical Garden lake after concentration with a 25 μm-mesh plankton net, averaging 25 μg/L. “Medium chlorophyll-a” was a mixture containing 50% from HC and 50% LC, resulting in 15 μg/L on average. “Low chlorophyll-a” corresponded to the natural chlorophyll concentration in the water, 5 μg/L on average. Further details can be found in Supplementary Material, Supplementary Section S1.
The treatments were randomized to prevent stochastic effects on the results. They were further conditioned in a thermostated cabinet at 18°C, with constant stirring on a WTW Oxitop IS 12 stirring plate with magnetic bars (4 cm × 0.5 cm) over the 7 days of the experiment. In the experimental conditions, the particulate material was constantly resuspended, reproducing local environmental conditions for phytoplankton, for example in case of a natural event such as wind resuspension or other turbidity-increasing impacts. An LED light was kept inside the thermostated cabinet, with 12-h light and dark photoperiods. An International Light IL 14004 radiometer was used to measure the irradiance at each point of the stirring plate, which ranged from 434 μW/cm2 (min) to 496 μW/cm2 (max), average 445 ± 19.8 μW/cm2. Before each daily measurement, the pH of treatments was measured with a Hanna HI 8424 pH meter.
Water samples from all treatments and controls were taken at the beginning (t0) and the end of the experiment (t7) period. Water samples (except for total phosphorus analyses) were filtered through a 1.2-μm-pore glass-fiber filter and later analyzed for dissolved organic carbon (DOC), dissolved nitrogen (DN), and dissolved inorganic phosphorus or orthophosphate (DP) concentrations. DOC and DN were analyzed following sodium persulfate digestion in a Shimadzu 5000A TOC L Analyzer; TP and DP were estimated by the molybdenum-blue method (Wetzel and Likens, 1991).
2.2 Model Ecosystem
The sediments used in the experiments were collected from Lake Batata. Lake Batata is located on the floodplain of the Trombetas River and is ecologically important because of the bauxite tailings that were continuously dumped into the lake between 1979 and 1989. Nowadays, the deposited bauxite tailings cover approximately 30% of the lake area. Lake Batata is a unique environment with a suite of studies conducted since a long-term restoration project started in the late 1980s (Esteves et al., 1990; Esteves, 1998; Scarano et al., 2018; Josué et al., 2021). The restoration project consisted of replanting the impacted area with native species in order to keep the impacted sediments attached and immobilized on the lake margins and increase the organic matter content of the sediments (Dias et al., 2011). Even after the restoration actions, which are still in progress, the lake continues to have two distinct areas, one natural (Natural Batata, NB) and the other impacted (Impacted Batata, IB). Because of its importance, Lake Batata was used as a model system in this study.
Historically, sediments from NB have had higher total nitrogen (TN), dissolved organic carbon (DOC), and organic matter (OM) contents than IB (Roland and Esteves, 1993; Leal et al., 2004). However, due to restoration, the sediment organic-matter content was recently found to be very similar in NB and IB (Josué et al., 2021). NB also has higher interstitial water content, indicating a higher water-retention capacity (Callisto and Esteves, 1996a; Leal et al., 2003), and the sediments have a muddy-appearing organic layer composed of fine particles (Callisto and Esteves, 1996a), mainly fine sand and silt. In contrast, the IB sediments contain a large clay fraction, which remains suspended in the water column for a longer time (Callisto and Esteves, 1996b; Roland and Esteves, 1998) and in shallow areas is often resuspended by wind, increasing the turbidity. The bauxite tailings in IB are inert and nontoxic to phytoplankton and are composed mainly of silicates (47%), aluminum oxide (21%), and iron oxide (21%) (Lapa, 2000).
2.3 Sediment Samples
Sediment was collected from the uppermost 10 cm in NB. For IB we collected 10 cm of sediment just below the surface organic layer, which was formed following the lake’s restoration project. The organic layer was easily distinguishable in the field by its darker color, reaching a depth of nearly 6 cm in the restored areas, as also reported by Penha (2015).
Before the experimental treatments were assembled, the sediments were placed in sealed Erlenmeyer flasks, sterilized in an autoclave for 20 min, and dried at 60°C for 24 h (Quaggio and Raij, 1979). After cooling, the sediments were macerated to homogenize the particles, since the granulometry could affect the experimental results by creating noise through the turbidity effect (Merten et al., 2014; Yao et al., 2014). The grain size was analyzed to assess the mean size of particles. After maceration, sediment samples were sifted through sieves of different mesh sizes (1 mm, 500 μm, 250 μm, 150 μm, 75 μm, and <75 µm) coupled to a TecnoFund model AEP agitator to separate the material according to particle fractions, and classified according to the Wentworth scale (Buchanan, 1984). Organic-matter content in sediment aliquots was analyzed by loss-on-ignition for 4 h at 550°C, according to Carmouze (1994). All sediments were taken to the laboratory in the Federal University of Juiz de Fora (UFJF, Juiz de Fora, Minas Gerais) to set up the experiment.
2.4 Water Samples
Water samples were taken from an artificial lake in the UFJF Botanical Garden (21°44′18″ S, 43°22′07″ W). The water used in the experiments was sieved through a 68-μm plankton net to remove zooplankton that could affect the results (further details in Supplementary Material). To characterize the water samples, we analyzed the water temperature (°C), pH, oxidation/reduction potential (mV), electrical conductivity (μS/cm), turbidity (NTU), dissolved oxygen (mg/L and %), and total dissolved solids (mg/L), which were measured with a Horiba model U-50 multiparameter probe. Total chlorophyll-a of cyanobacteria and eukaryotic algae was measured in situ using a phytoplankton analyzer (PHYTO-PAM, Walz, Germany). Chlorophyll-a concentrations were measured for total chlorophyll-a (total Chl-a) and the main algal groups as given by the fluorometer: blue-green (Blue Chl-a), green (Green Chl-a), and brown (Brown Chl-a) as determined by the fluorescence readings at four wavelengths (470 nm, 520 nm, 645 nm, and 665 nm, respectively) (Schreiber et al., 1998). PHYTO-PAM separates algal groups by using the measurements of these four excitation wavelengths, according to the absorbance spectra of their light-harvesting complexes (LHC), which act as antennae pigments. For instance, Cyanobacteria show a strong signal at the 645 nm channel, most green algae show a signal at the 470 nm channel, and the majority of diatoms show a strong signal at 470 and 520 nm wavelengths. The equipment was calibrated using Anacystis sp. as the reference for “blue-green algae”, Ankistrodesmus sp. as the reference for “green algae”, and Phaeodactylum sp. as the reference for “brown algae” (Heinz Walz GmbH, 2003). Below we refer to algal groups by the fluorometric response, as chlorophyll groups as given by PHYTO-PAM, considering Cyanobacteria as blue-greens or “blue algae”; Chlorophyceae, Euglenophyceae, and Zygnematophyceae as “green algae”; and Chryptophyceae, Chrysophyceae, Bacillariophyceae, and Dinophyceae as “brown algae” (Schreiber, et al., 1998; Beecraft et al., 2017). PHYTO-PAM was also used to determine the daily algal yield, which corresponds to the effective quantum yield of photosystem II in an incident PAR radiation beam. These measurements can help to understand the photosynthetic performance of communities.
Phytoplankton samples from the initial (t0) and final (t7) experiment times were preserved with acid Lugol’s iodine solution and conditioned in the dark. Phytoplankton abundance was estimated using an Olympus IX71 inverted microscope, with the settling technique according to Utermohl (1958). Cells, filaments, and colonies were counted at ×40 magnification in random fields until the taxon accumulation curve stabilized. Taxa were identified to genus level and categorized as major taxonomic groups, using taxonomic keys (Komárek and Anagnostidis, 1998, 2005; van den Hoek et al., 1995).
2.5 Data Analysis
A pilot experiment indicated that the sediment suspension (only sediments and water) contributed to a small chlorophyll-a signal (here termed background chlorophyll-a) and could therefore affect the treatment readings by PHYTO-PAM. We corrected for this source of bias by subtracting the treatment readings at the initial time (containing the sum of real chlorophyll-a and background reading from sediment) from the control values (containing equivalent real chlorophyll-a value) for each treatment. This difference, the background chlorophyll-a calculated for each treatment, was then subtracted from each chlorophyll-a value generated on each day during the course of the experiment (details in Supplementary Material, Topic 2). The values resulting from this correction were termed “corrected chlorophyll”.
Nutrients and limnological parameters were submitted to paired t-tests in R software (R Core Team, 2019) to assess the variation between initial (t0) and final (t7) concentration values. The stoichiometric molar ratios between dissolved fractions of carbon (C), nitrogen (N), and phosphorus (P), i.e., C:N, N:P, and C:P, were calculated from the DOC, DN, and DP concentrations. We compared our results to the C:N:P ratio for tropical freshwater plankton calculated by (They et al., 2017; reference C:N:P ratios of 307:30:1), considering the particular features and dynamics of these environments that affect the nutrient dynamics.
Chlorophyll-a values were compared between treatments with a repeated-measures GLM (General Linear Model) analysis, also in R. In these models, we indicated the temporal non-independence of data, with “time” as a random factor. For comparison between sediment types, we used “sediment type” (IB or NB) as a comparison factor and “time” as the random factor. Bonferroni adjustment was used for post-hoc comparisons.
Chlorophyll-a values by treatment over the incubation time were submitted to linear regression in JMP-SAS 14 software to obtain general trend lines and linear-regression equations
3 Results
3.1 Sediment and Water Characterization
The sediments from Lake Batata were predominantly coarse and medium-grained sand for NB and medium and fine-grained sand for IB (Table 1). Organic-matter contents were 21.48% for NB and 20.43% for IB.
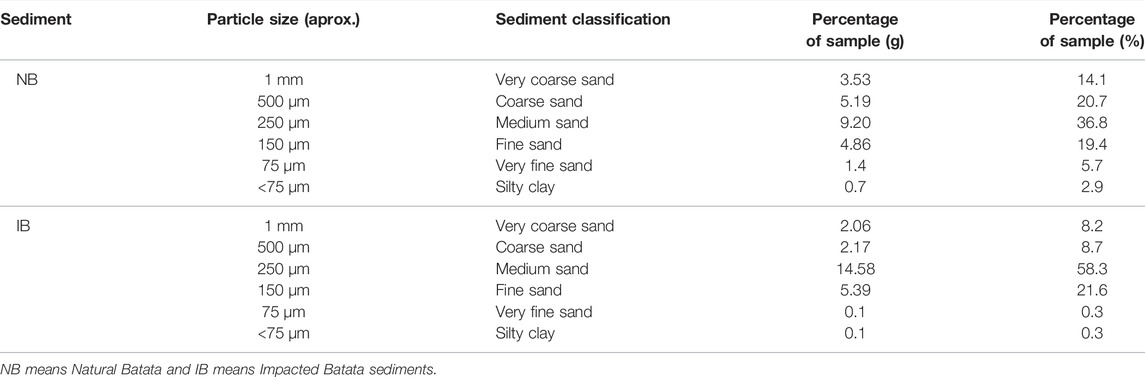
TABLE 1. Granulometric composition of sediments (NB) and (IB) by particle size, sediment classification by particle size, and amount of sediment retained in sieves (g) and in percentage (proportional to sample size 25 g).
Nutrient contents of water from the UFJF Botanical Garden lake were 30.7 ± 0.9 μg/L TP, 6.8 ± 0.4 μg/L DP 3.7 ± 0.1 mg/L DOC, and 0.2 ± 0.05 mg/L DN. At the time of the analysis, water turbidity was 25.2 NTU and pH was 7.4 (Table 2). The chlorophyll-a concentration in the lake was approximately 5.51 ± 0.22 μg/L. The green, brown, and blue pigment bands were, on average, 46.2, 46.9, and 5.2% respectively.
3.2 Experimental Results
3.2.1 Chlorophyll-a Trends
Chlorophyll-a in the NB sediment treatments ranged from 4.76 μg/L in MTLC to 34.32 μg/L in HTHC. Chlorophyll-a in IB sediment treatments ranged from 5.31 μg/L in LTLC to 30.89 μg/L in HTHC (Figure 2). Comparing treatments by sediment type showed that NB and IB were significantly different (F = 8.53, p < 0.01). For NB sediment, there were effects of turbidity (F = 51.36, p < 0.01), chlorophyll-a levels (F = 423.02, p < 0.01), and the interaction between them (F = 3.04, p = 0.01). For IB sediment, turbidity also had a significant effect in treatments (F = 32.57, p < 0.01), as well as chlorophyll levels (F = 513.29, p < 0.01) and the interaction (F = 14.02, p < 0.01). Post-hoc comparison tests for NB treatments showed that low-turbidity treatments were not significantly different from medium-turbidity treatments for both sediment types, but all chlorophyll-a treatments differed from each other (Table 3).
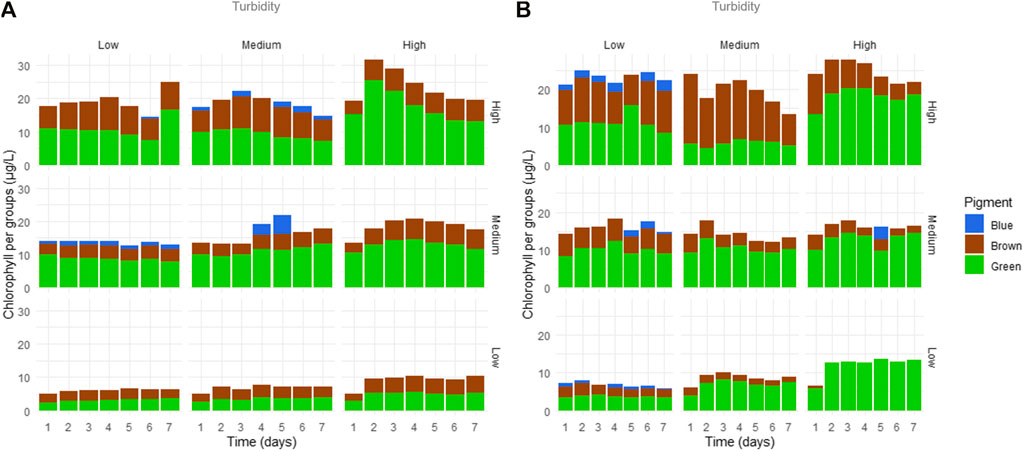
FIGURE 2. Chlorophyll-a variation (μg/L) by time (days) responding to turbidity levels. (A), NB results; (B), IB results. Horizontal labels above graphs show initial turbidity levels and vertical lateral labels show chlorophyll-a levels forming experimental design combinations. Colors refer to chlorophyll groups. Chlorophyll-a scales differ between (A) and (B). NB means Natural Batata and IB means Impacted Batata.
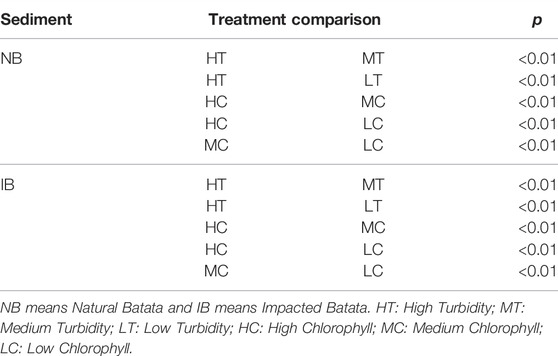
TABLE 3. Post-hoc tests by sediment (NB and IB) and treatment. Only statistically significant (p < 0.05) results are shown.
The slopes of chlorophyll-a overtime for NB treatments ranged from –1.06 to 0.82 (Figure 3), and chlorophyll-a in treatments LTLC and MTMC significantly increased over time (ANOVA, p < 0.05). The slopes of chlorophyll-a overtime for IB ranged from –1.28 (MTHC) to 0.82 (LTHC). LC treatment slopes were directly proportional to turbidity levels, and high-chlorophyll-a slopes were inversely proportional to turbidity, decreasing as turbidity increased (Figure 3).
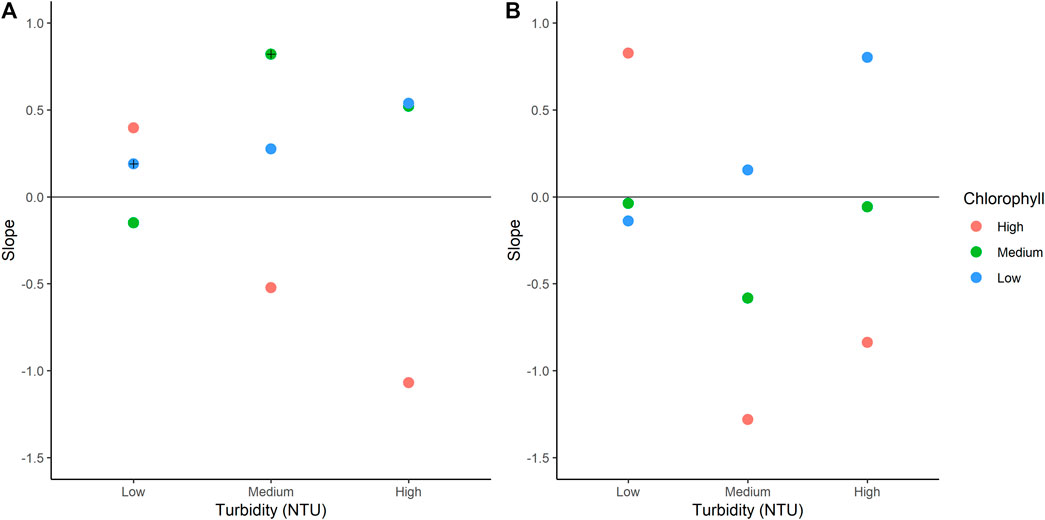
FIGURE 3. Treatment slopes by sediment concentration. (A), NB results; (B), IB results. Each dot shows the calculated slope of chlorophyll values over time. Turbidity levels are indicated on the x-axis and chlorophyll-a levels are separated by color. Treatments with significant changes in slope are highlighted (+). NB means Natural Batata and IB means Impacted Batata.
Algal yields ranged between 0.07 and 0.7 in NB sediment treatments. For IB sediments, algal yields ranged between 0.17 and 1.12 (Figure 4).
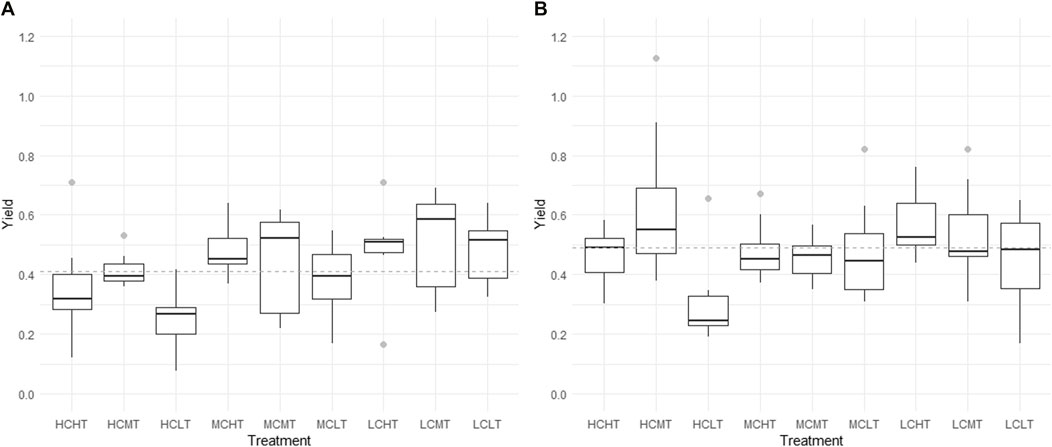
FIGURE 4. Differences in algal yield among treatments of the sediments. (A), NB results; (B), IB results. NB means Natural Batata and IB means Impacted Batata. HT: High Turbidity; MT: Medium Turbidity; LT: Low Turbidity; HC: High Chlorophyll; MC: Medium Chlorophyll; LC: Low Chlorophyll. The gray dotted line shows the mean yield value for NB (0.41) and for IB (0.49) treatments.
3.2.2 Pigments and Taxonomic Composition
In NB treatments, dominant species belonged mostly to the green group (51–53% average) and brown group (46–48%), especially in LC treatments. For other treatments such as MC, the green group was dominant (61–74%, Figure 2). The same was found for HC treatments, where the green group was also dominant (i.e., HTHC = 51–73%, Figure 2).
For IB sediment treatments, in LC and MC the green group dominated (55–98%) and (63–83%), respectively. In treatment MTHC the brown group (69%) prevailed, followed by the green group (30%). In HC treatments, the phytoplankton composition varied along the turbidity gradient. For instance, in LT the green group slightly dominated over (48%) over the brown group (43%), in MT the brown group dominated (69%) over the green group (30%), and in HT the green group predominated (73%).
In the IB treatments, Chrysophyceae, Cryptophyceae, Euglenophyceae, and Chlorophyceae were the dominant groups. The phytoplankton composition did not change drastically in most of the treatments except for IB HTHC and HTLC (Figures 5, 6). In the NB treatments, Euglenophyceae and Dinophyceae dominated, followed by Chlorophyceae and Cyanobacteria. In the HTLC treatment, Cyanobacteria increased markedly from the initial (t0) to the final (t7) time of the experiment. Other treatments also showed an increase in the Cyanobacteria contribution (e.g., HTMC, MTHC, MTLC, and LTHC).
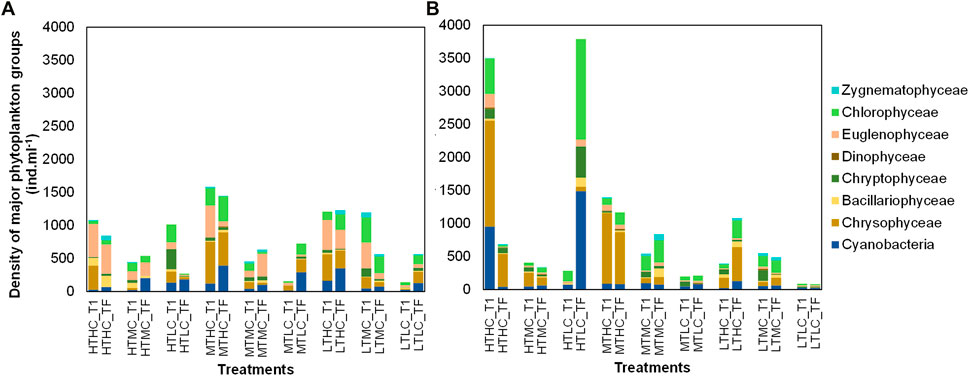
FIGURE 5. Density (ind/mL) of main phytoplankton groups by sediment and by treatment at the initial time (T1) and final time (TF). (A), NB results; (B), IB results. NB means Natural Batata and IB means Impacted Batata. HT: High Turbidity; MT: Medium Turbidity; LT: Low Turbidity; HC: High Chlorophyll; MC: Medium Chlorophyll; LC: Low Chlorophyll.
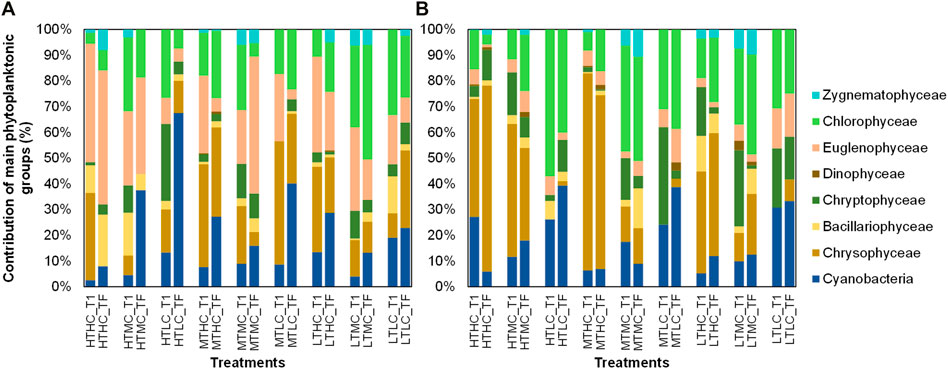
FIGURE 6. Percent contribution (%) of main phytoplankton groups by sediment and by treatment at the initial time (T1) and final time (TF). (A), NB results; (B), IB results. NB means Natural Batata and IB means Impacted Batata. HT: High Turbidity; MT: Medium Turbidity; LT: Low Turbidity; HC: High Chlorophyll; MC: Medium Chlorophyll; LC: Low Chlorophyll.
3.2.3 pH, Nutrient Concentrations, and Stoichiometric Ratios
During the experiment the pH varied between 7.45 and 8.97 in NB and between 7.49 and 8.98 in IB. Both showed significant changes (p < 0.05) over time for LT treatments of NB sediment and most IB treatments (Table 4). In the NB treatments, DOC concentrations varied from 4.0 to 8.3 mg/L, DN from 0.18 to 0.87 mg/L, TP from 24.92 to 179.22 μg/L, and DP from 3.74 to 15.74 μg/L. In the IB treatments, DOC concentrations varied from 4.2 to 5.7 mg/L, DN from 0.14 to 0.27 mg/L, TP from 35.47 to 134.87 μg/L (Table 5), and DP from 6.2 to 10.8 μg/L. There was significant variation (p < 0.05) over the incubation time for DOC in LT treatments of NB sediment and MT treatments of IB sediment. For DN, only the MTLC treatment of NB showed significant variation over the incubation time (Table 6).
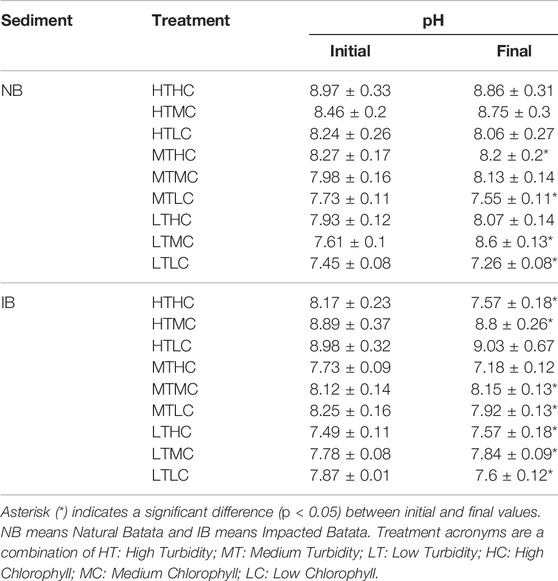
TABLE 4. pH values by sediment type (NB and IB) and treatment type at the beginning and end of the experiments.
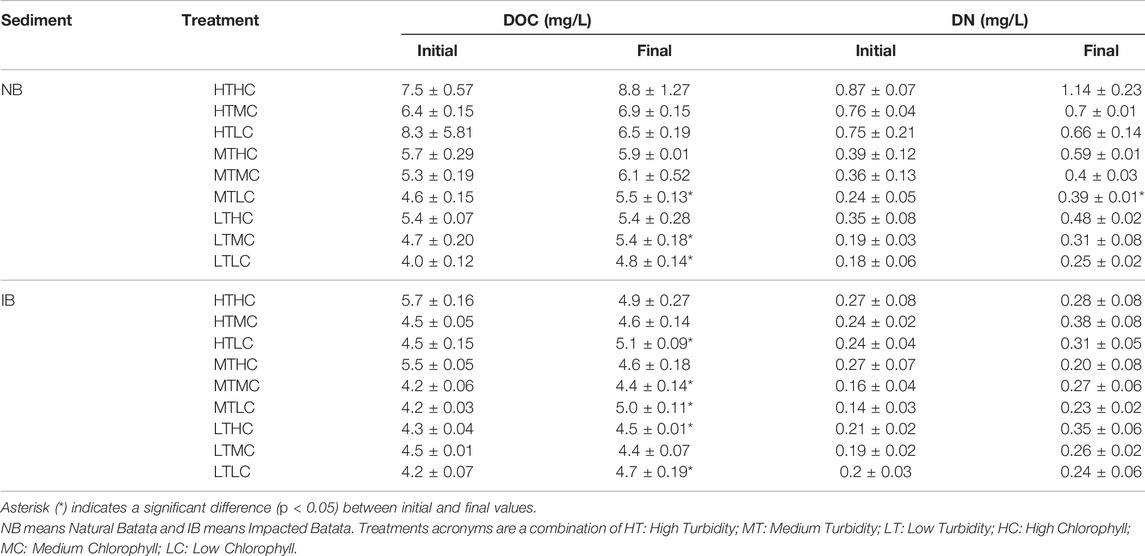
TABLE 5. Dissolved organic carbon (DOC) and dissolved nitrogen (DN) by sediment type (NB and IB) and treatment at the beginning and end of the experiments.
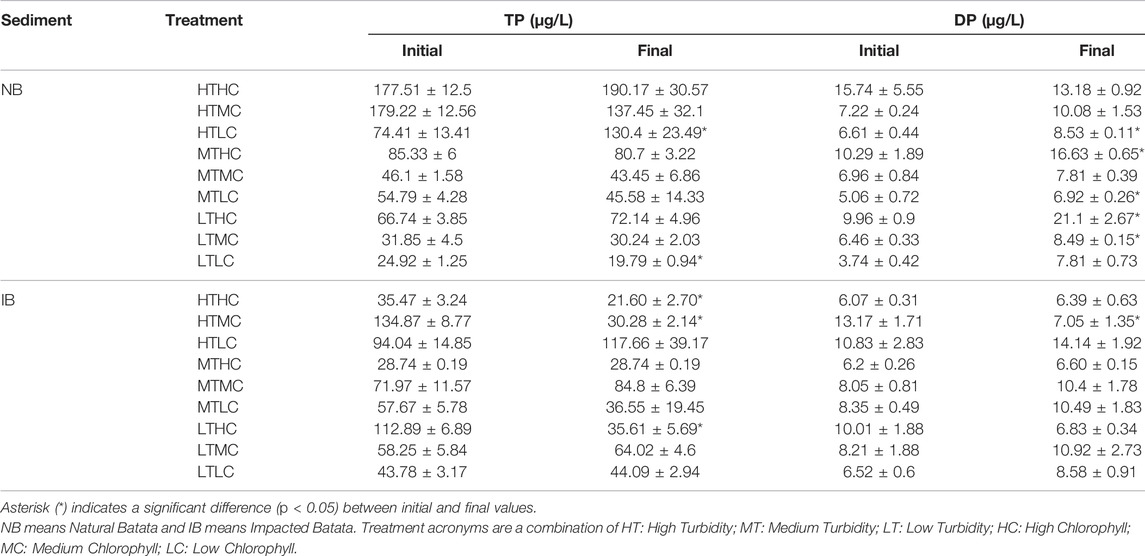
TABLE 6. Total phosphorus (TP) and dissolved phosphorus (DP), by sediment type (NB and IB) at the beginning and end of the experiments.
N:P ratios ranged from 3:1 to 11:1 in NB and from 1:1 to 5:1 in IB. The C: N ratios ranged from 18:1 to 56:1 for NB and from 44:1 to 72:1 for IB, with a tendency to decrease toward the end of the experiment. The C:P ratios ranged from 181:1 to 307:1 in NB and from 86:1 to 253:1 in IB (Table 7).
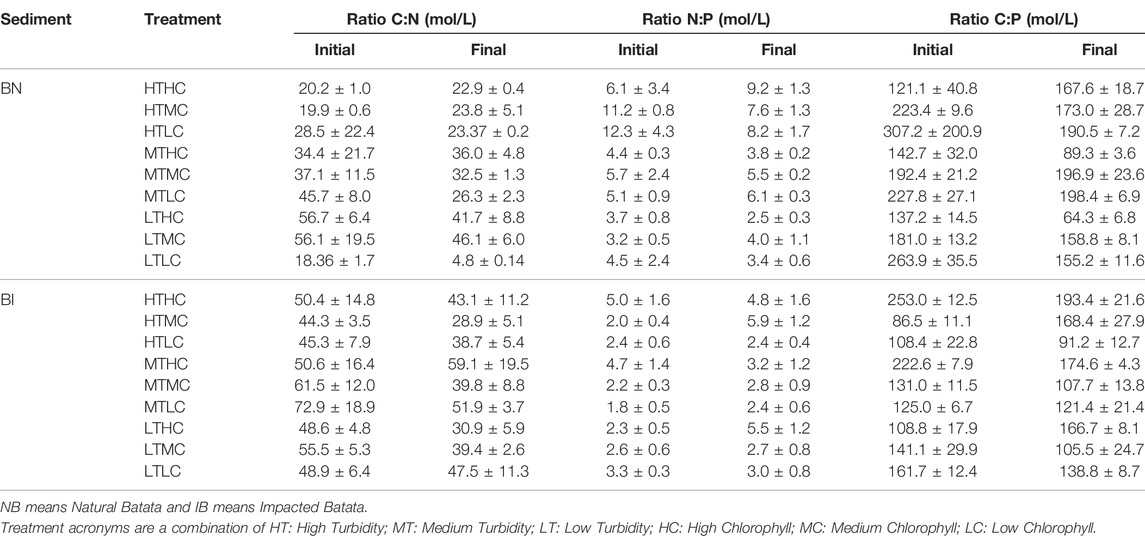
TABLE 7. C:N, N:P and C:P ratios (in mol/L) by sediment type and treatment at the beginning and end of the experiments.
4 Discussion
One of the main factors that influence aquatic photosynthesis is light availability in the water column, which is closely linked to turbidity (Kirk, 1985). Turbidity levels are increasing in many aquatic ecosystems as a result of anthropogenic factors such as urbanization, agricultural and industrial expansion, and mining activities (Rodrigues et al., 2018). In this study, we experimentally assessed the effect of turbidity on phytoplanktonic chlorophyll-a by combining different levels of chlorophyll-a and turbidity. Our results showed that despite the expected turbidity-shading effects, photosynthesis continued even in the high-turbidity treatments, with a prevalence of green and brown algae in water with both sediments tested (NB and IB) at the end of the experiment. The turbidity effect was stronger with IB sediments and in treatments with higher initial chlorophyll-a levels.
4.1 Turbidity Effects on Chlorophyll-a Concentrations
In general, it is expectable that high turbidity will reduce light incidence in the water and negatively affect primary production, reducing chlorophyll-a content in more-turbid systems, as we hypothesized here. In our experiments, the high-chlorophyll-a treatments showed lower and negative slopes as turbidity increased, but the low-chlorophyll-a treatments had increasing slopes along with turbidity, confirming our first hypothesis.
A tendency toward growth of primary producers in turbid environments has been reported in systems where wind resuspends the sediment periodically, such as estuaries (Pinckney et al., 2011) and shallow lakes. In these systems, internal nutrient remobilization can cause eutrophication (Søndergaard et al., 2003). In Lake Batata, resuspension of sediments in the impacted area increases chlorophyll-a concentration by increasing the nutrient supply (Panosso, 1993). In these cases, nutrients stored in the sediments are often resuspended in the water column, favoring phytoplankton primary production (Nõges and Nõges, 1999). Nutrient availability may be an associated explanation for our findings in the low and medium-chlorophyll treatments, as we recorded high phosphorus concentrations. Furthermore, in the low-chlorophyll-a treatments, the light spread and was reflected inside the systems as diffuse radiation, which can be better used by fewer phytoplankton cells. Productivity in the low-chlorophyll-a treatments may have been favored by light scattering, a factor determined by the properties of suspended particles, which can benefit phytoplankton in turbid systems because of the light wavelengths used for photosynthesis, generating different chlorophyll responses (Falkowski and Raven, 2007; Kirk, 2011). In our treatments, the light might not have been a limiting factor in the low-turbidity treatments because of the small size of the beakers used as microcosms, since there was not enough volume to create an aphotic zone. We, therefore, compare this situation to natural environments at the surface of the water column, where, besides not being light-limited, algae exposed to photoinhibition can benefit from microhabitats formed by suspended particles in glacier meltwater-fed lakes (Sommaruga, 2015), estuaries (MacIntyre and Cullen, 1996), or tropical ponds (Mayer, 2020). Further, particulate matter is reported to facilitate algae aggregation (Guenther and Bozelli, 2004), and in our experiment, it may have prevented the cells from sinking and helped in light-capturing in treatments with less competition. Sediment could have acted as a substrate for bacterial aggregation, facilitating nutrient cycling through bacterial-phytoplankton metabolism coupling in low-chlorophyll-a treatments (Zak and Grigal, 1991; Naeem et al., 2000; Barlett and Leff, 2010).
Along with competition for light, another plausible explanation for the decreasing slope patterns in treatments with high initial chlorophyll-a (HTHC, MTHC, and LTHC) is that sediment particles, together with shading from phytoplankton cells, could reduce light availability in the systems, resulting in low light availability for phytoplankton absorption; alternatively, the systems may have reached support capacity, limiting resources for phytoplankton growth. The values for algal yield were mostly below 0.83, a threshold where the phytoplankton community is under light stress in performing photosynthesis (Dau, 1994; Schreiber, 2004; Falkowski and Raven, 2007). Therefore, our findings support the hypothesis that inorganic turbidity may be an important bottom-up control factor by causing light limitation to primary production in aquatic ecosystems and reducing chlorophyll-a concentrations in these systems, especially in treatments with high initial chlorophyll-a. One consequence of this process could be the reduction of carbon transfer to herbivorous zooplankton and higher trophic levels (Kirk, 1991). For instance, cladocerans in turbid water can be strongly affected because most are filter-feeders (Hart, 1988; Kirk, and Gilbert, 1990).
Studies on natural systems impacted by bauxite tailings have reported lower primary and secondary production (Cole et al., 1992; Grobbelaar, 1992; Cuker, 1993), along with reductions in diversity, density, or biomass of phytoplankton in impacted areas (Huszar, 2000). However, communities can change in composition to groups that are better adapted to the new conditions. In our experiment, it is also likely that the plankton organisms that persisted might have adapted to low light conditions by developing compensatory mechanisms. Some of the mechanisms may involve reducing the respiration rate, increasing the concentration of photosynthetic pigments, and even changing the proportions of accessory pigments to increase photon capture, in a process called ontogenetic chromatic adaption (Müller et al., 2003; Reynolds, 2006; Kirk, 2011).
4.2 Turbidity Effects on Chlorophyll-a Pigments and Phytoplankton Composition
Different kinds of particles in suspension can cause different intensities and compositions of the light spectrum that reaches microorganisms (Kirk, 2011). As a consequence, the distribution and dominance of chlorophyll-a and other pigments may be related to light availability and quality. We hypothesized that high turbidity would cause a change in phytoplankton composition toward low-light-adapted algae; however, our results disagreed with this statement.
Mixotrophy is an algal nutrition mode that includes photosynthetic and heterotrophic sources. It allows protists to supplement their needs by absorbing organic substrates, phagocytizing particulate organic carbon and/or bacteria. However, energy acquisition from the environment does not ensure independence from photosynthesis, and light availability remains important for mixotrophic phytoplankton survival (Burkholder et al., 2008; Naselli-Flores and Barone, 2019). The use of organic nutrients when light is limited allows mixotrophic phytoplankton to maintain growth, lending an important competitive advantage over strict phototrophs and heterotrophs (Jones et al., 2009). It is important, therefore, to distinguish between forms of organic carbon acquisition performed by mixotrophs, since to some degree, most phytoplankton can absorb dissolved carbon through osmotrophy (Naselli-Flores and Barone, 2019). Individuals capable of ingesting particles and bacterial aggregates are considered phagotrophic and are normally flagellated. These terms are used here to distinguish among the most abundant genera in treatments (Supplementary Figure S2) and the predominant form of nutrition (Supplementary Table S4).
Phagotrophic algae such as Chrysophyceae can maintain heterotrophic metabolism under light-limiting conditions, thus compensating for a loss of photosynthesis (Katechakis et al., 2005). Chrysophyceae species often increase or even dominate in turbid systems (Costa et al., 2019). However, in our experiment, no consistent dominance shift in algal composition occurred between the initial and final times in treatments, disagreeing with our second hypothesis. In treatments where the brown group was dominant, phagotrophic groups such as Euglenophyceae, Crysophyceae, and Cryptophyceae were present. These organisms are capable of consuming organic carbon available in the water, facilitating survival and dominance even under low light (Kruk and Segura, 2012; Bortolini et al., 2014; Costa et al., 2019). Shifts to the dominance of brown algae in turbid environments were reported in semiarid regions (Costa et al., 2016), with phytoplankton shifting initially to cyanobacteria dominance and then to brown algae. In our systems, phytoplankton composition responded to turbidity, with the maintenance of initially present phagotrophic algae that further prevailed due to good adaptation to low light conditions.
Most freshwater species of green algae show wide plasticity in their responses to low light conditions (Karsten et al., 2017). In turbid systems, chlorophytes can be expected to decrease because they are sensitive to high turbidity (Reynolds et al., 2002). In our system, however, photosynthetic plasticity is a likely reason for the dominance of Chlorophyceae species in the high-chlorophyll-a treatments. This held even under high-turbidity conditions (HTLC in IB), where phagotrophs such as Chrysophyceae were also present, thus maintaining most of the photosynthetic activity. Cyanobacteria species occurred in almost all treatments, perhaps because of the presence of a favorable light spectrum for this group (Luimstra et al., 2020), i.e., the blue band (430 nm). Cyanobacteria can be especially dominant under nitrogen-limiting conditions because they can perform N2 fixation (Reynolds, 2006). In general, Cyanobacteria species are S strategists and perform well in nitrogen-limited environments (Wang et al., 2020). The Nutrient-Load hypothesis proposed by Brauer et al. (2012) states that Cyanobacteria species are better competitors in light and/or nitrogen-limited environments, so light availability and the nutrient ratio in our systems could have been favorable for Cyanobacteria to establish, since this group can grow both in low C:P (Penha, 2015) and low N:P (Schindler, 1975; Smith, 1983) ratios, as in our systems. Also, picocyanobacteria tend to increase under high inorganic turbidity (Brasil et al., 2017; Somogyi et al., 2017). This agrees with the increase in Cyanobacteria in our experiment since filamentous cyanobacteria were found at the end of the experiment, indicating an adaption to low light (Kirk, 2011). In our treatments, Leptolyngbya and Chroococcus were the most numerous species; they are considered good competitors and able to dominate in shallow and turbid environments (Scheffer et al., 1997).
4.3 Effects of Sediment Type
Even though the sediments used in our experiments came from the same lake (Lake Batata), they had different chemical compositions and physical properties, causing different turbidity effects, as shown elsewhere (Esteves et al., 1990; Roland et al., 1997). Roland and Esteves (1998) reported that particles from natural-area sediments in Lake Batata usually settle faster and more easily than the light bauxite-tailing particles (Roland and Esteves, 1998). Thus, the effect of bauxite tailings on chlorophyll-a and to some extent on photosynthesis can last longer. We hypothesized that inorganic turbidity containing bauxite tailings would generate stronger negative effects on chlorophyll-a levels, and our hypothesis was confirmed. The effect of NB sediment on chlorophyll-a was milder since NB sediment led to higher chlorophyll-a values (6.39% higher than IB) but low yield, indicating more difficult conditions for these communities to photosynthesize. NB sediment favored Crysophyceae and Euglenophyceae, which have a lower requirement for light irradiance because they can compensate through mixotrophy (Flynn et al., 2013; Mitra et al., 2016). The most likely explanation is that the NB sediment had a shading effect, allowing algae to persist but at a higher metabolic cost.
The mine-tailing sediments led to lower slopes in IB, indicating the same negative effect seen in in-situ measurements, where lower primary production in the impacted area has been reported (Roland, 1995; Roland et al., 2002; Guenther and Bozelli, 2004). Similar results were found in another system impacted by gold-mine tailings (Moreira et al., 2016). Nowadays, the negative effects of bauxite tailings in Lake Batata are not the same as at the beginning of the monitoring program 30 years ago. In the dry period, when the water is more turbid, phytoplankton reaches high densities because nutrient availability has increased with the recovery of the lake (Bozelli et al., 2015). In turbid environments with low phytoplankton primary production related to tailings, the principal effect on the trophic web is loss of energy transfer through trophic levels, indirectly affecting zooplankton (Bozelli and Esteves, 1995) and fish (Shoup and Wahl, 2009). In our tailings turbidity treatments, chlorophyll-a, and to some extent primary production, were maintained, mainly due to the success of phagotrophic Chlorophyceae and Chrysophyceae, groups adapted to low light conditions (Reynolds, 2006). Despite the lower chlorophyll-a values, IB sediments allowed higher yields, even higher than 0.83 in some treatments, a level at which algae are not considered to be under light stress. This may be due to the physical properties of bauxite tailings, which despite reducing light input, may have allowed favorable wavelength dispersion. This requires further investigation beyond the scope of our study.
5 Conclusion
Our results showed that the turbidity effect was stronger in treatments with higher sediment concentrations. However, high turbidity did not necessarily lead to a linear reduction in chlorophyll-a concentrations, and photosynthesis was maintained even with the turbidity shading effect in treatments with both sediment types (NB and IB). In NB the conditions for photosynthesis were better since treatments with this sediment type had higher chlorophyll-a values than IB, and also higher contributions of brown algae (Euglenophyceae, Dinophyceae, Bacillariophyceae). In IB, the phytoplankton was composed mainly of Chrysophiceae, Cryptophyceae, Chlorophyceae, and Cyanobacteria. Accordingly, the IB sediments affected the phytoplankton composition and chlorophyll-a more than the NB sediments.
Although the effect of turbidity on the metabolism of freshwater phytoplankton is widely known, our study advances the comprehension of how turbidity caused by mine tailings affects photosynthesis and phytoplankton composition, which may support recovery protocols and environmental policies for aquatic ecosystems.
Data Availability Statement
The original contributions presented in the study are included in the article/Supplementary Material, further inquiries can be directed to the corresponding author.
Author Contributions
Conceptualization: PN, FR, AA, SC; Methods: PN, FR, AA, SC, NR; Resources: FR; Writing original draft: PN; Algae taxonomic composition analysis: NR; Discussion Writing, Review, and Editing: FR, AA, SC, NR.
Funding
This study was partly funded by Brazil’s National Council for Scientific and Technological Development (CNPq), which provided Productivity grants to FR (PQ 311.892/2017-5) and AA (PQ 310.033/2017-9 and PQ 312.772/2020-2).
Conflict of Interest
The authors declare that the research was conducted in the absence of any commercial or financial relationships that could be construed as a potential conflict of interest.
Publisher’s Note
All claims expressed in this article are solely those of the authors and do not necessarily represent those of their affiliated organizations, or those of the publisher, the editors and the reviewers. Any product that may be evaluated in this article, or claim that may be made by its manufacturer, is not guaranteed or endorsed by the publisher.
Acknowledgments
The present study is based on the dissertation of PN, which is available online from the UFJF institutional repository at https://repositorio.ufjf.br/jspui/handle/ufjf/10294. The authors would like to thank Rafael M. Almeida for revising the manuscript and Janet Reid for language revision.
Supplementary Material
The Supplementary Material for this article can be found online at: https://www.frontiersin.org/articles/10.3389/fenvs.2021.605838/full#supplementary-material
References
Barlett, M. A., and Leff, L. G. (2010). The Effects of N:P Ratio and Nitrogen Form on Four Major Freshwater Bacterial Taxa in Biofilms. Can. J. Microbiol. 56, 32–43. pmid:20130692. doi:10.1139/w09-099
Beecraft, L., Watson, S. B., and Smith, R. E. H. (2017). Multi-Wavelength Pulse Amplitude Modulated Fluorometry (Phyto-PAM) Reveals Differential Effects of Ultraviolet Radiation on the Photosynthetic Physiology of Phytoplankton Pigment Groups. Freshw. Biol. 62 (1), 72–86. doi:10.1111/fwb.12850
Bentley, S. J., Blum, M. D., Maloney, J., Pond, L., and Paulsell, R. (2016). The Mississippi River Source-To-Sink System: Perspectives on Tectonic, Climatic, and Anthropogenic Influences, Miocene to Anthropocene. Earth-Sci. Rev. 153, 139–174. doi:10.1016/j.earscirev.2015.11.001
Bilotta, G. S., and Brazier, R. E. (2008). Understanding the Influence of Suspended Solids on Water Quality and Aquatic Biota. Water Res. 42 (12), 2849–2861. doi:10.1016/j.watres.2008.03.018
Bortolini, J. C., Rodrigues, L. C., Jati, S., and Train, S. (2014). Phytoplankton Functional and Morphological Groups as Indicators of Environmental Variability in a Lateral Channel of the Upper Paraná River Floodplain. Acta Limnol. Bras. 26 (1), 98–108. doi:10.1590/S2179-975X2014000100011
Bozelli, R. L., and Esteves, F. A. (1995). Species Diversity, Evenness and Richness of the Zooplankton Community of Batata and Mussurá Lakes of the Trombetas River, Amazônia, Brazil. Int. Conf. Trop. Limnol. Trop. Lakes Reserv. 2, 87–93.
Bozelli, R. L., Thomaz, S. M., Padial, A. A., Lopes, P. M., and Bini, L. M. (2015). Floods Decrease Zooplankton Beta Diversity and Environmental Heterogeneity in an Amazonian Floodplain System. Hydrobiologia 753, 233–241. doi:10.1007/s10750-015-2209-1
Brasil, J., Huszar, V. L. M., Attayde, J. L., Marinho, M. M., Van Oosterhout, F., and Lürling, M. (2018). Effect of Suspended clay on Growth Rates of the Cyanobacterium Cylindrospermopsis Raciborskii. fal 191 (1), 13–23. doi:10.1127/fal/2017/1096
Brauer, V. S., Stomp, M., and Huisman, J. (2012). The Nutrient-Load Hypothesis: Patterns of Resource Limitation and Community Structure Driven by Competition for Nutrients and Light. Am. Natur. 179, 721–740. doi:10.1086/665650
Buchanan, J. B. (1984). “Sediment Analysis,” in Methods for the Study of marine Benthos. 2nd ed. Editors N. A. Holme, and A. D. McIntyre (Oxford: Blackwell Scientific Publications), pp. 41–65. 0-632-00894-6.
Burkholder, J. M., Glibert, P. M., and Skelton, H. M. (2008). Mixotrophy, a Major Mode of Nutrition for Harmful Algal Species in Eutrophic Waters. Harmful Algae 8, 77–93. doi:10.1016/j.hal.2008.08.010
Callisto, M. F. P., and Esteves, F. A. (1996b). Composição granulométrica Do sedimento de um lago amazônico impactado por rejeito de bauxita e um lago natural. Acta Limnol. Bras. 8, 115–126.
Callisto, M. F. P., and Esteves, F. A. (1996a). Macroinvertebrados Bentônicos em dois Lagos Amazônicos: Lago Batata (um Ecossistema Impactado por Rejeito de Bauxita) e Lago Mussurá (Brasil). Acta Limnol. Bras. 8, 137–147.
Cantonati, M., Poikane, S., Pringle, C. M., Stevens, L. E., Turak, E., Heino, J., et al. (2020). Characteristics, Main Impacts, and Stewardship of Natural and Artificial Freshwater Environments: Consequences for Biodiversity Conservation. Water 12 (85), 260. doi:10.3390/w12010260
Carmouze, J. P. (1994). O metabolismo dos ecossistemas aquáticos: fundamentos teóricos, métodos de estudo e análise squímicas. São Paulo: Edgard Blucher/FAPESP.
Cole, J. J., Caraco, N. F., and Peierls, B. L. (1992). Can Phytoplankton Maintaina Positive Carbon Balance in a Turbid, Freshwater, Tidal Estuary? Limnol. Oceanogr. 37, 1608–1617. doi:10.4319/lo.1992.37.8.1608
Costa, M. R. A., Menezes, R. F., Sarmento, H., Attayde, J. L., Sternberg, L. d. S. L., and Becker, V. (2019). Extreme Drought Favors Potential Mixotrophic Organisms in Tropical Semi-arid Reservoirs. Hydrobiologia 831, 43–54. doi:10.1007/s10750-018-3583-2
Crossetti, L. O., Becker, V., Cardoso, L. d. S., Rodrigues, L. R., da Costa, L. S., and da Motta-Marques, D. (2013). Is Phytoplankton Functional Classification a Suitable Tool to Investigate Spatial Heterogeneity in a Subtropical Shallow lake? Limnologica 43 (3), 157–163. doi:10.1016/j.limno.2012.08.010
Cuker, B. E. (1993). Suspended Clays Alter Trophic Interactions in the Plankton. Ecology 74, 944–953. doi:10.2307/1940818
da Costa, M. R. A., Attayde, J. L., and Becker, V. (2016). Effects of Water Level Reduction on the Dynamics of Phytoplankton Functional Groups in Tropical Semi-arid Shallow Lakes. Hydrobiologia 778, 75–89. doi:10.1007/s10750-015-2593-6
Dau, H. (1994). Short-term Adaptation of Plants to Changing Light Intensities and its Relation to Phytosystem II Photochemistry and Fluorescence Emission. J. Photochem. Photobiol. 39, 873–885.
Davies-Colley, R. J., and Smith, D. G. (2001). Turbidity Suspeni)ed Sediment, and Water Clarity: a Review. J. Am. Water Resour. Assoc. 37, 1085–1101. doi:10.1111/j.1752-1688.2001.tb03624.x
de Jong, J., van Rooy, P. T. J. C., and Hosper, S. H. (1995). Living with Water: at the Cross-Roads of Change. Water Sci. Technol. 31 (8), 393–400. doi:10.2166/wst.1995.0337
Descy, J. P., Reynolds, C. S., and Padisák, J. (2013). Phytoplankton in Turbid Environments: Rivers and Shallow Lakes. Boston, London: Springer Science & Business Media.
Dias, A. T. C., Bozelli, R. L., Darigo, R. M., Esteves, F. d. A., dos Santos, H. F., Figueiredo-Barros, M. P., et al. (2011). Rehabilitation of a Bauxite Tailing Substrate in Central Amazonia: The Effect of Litter and Seed Addition on Flood-Prone Forest Restoration. Restor. Ecol. 20, 483–489. doi:10.1111/j.1526-100X.2011.00811.x
Dierssen, H. M., Smith, R. C., and Vernet, M. (2002). Glacial Meltwater Dynamics in Coastal Waters West of the Antarctic peninsula. Proc. Natl. Acad. Sci. 99 (4), 1790–1795. doi:10.1073/pnas.032206999
Donohue, I., and Garcia Molinos, J. (2009). Impacts of Increased Sediment Loads on the Ecology of Lakes. Biol. Rev. 84, 517–531. doi:10.1111/j.1469-185x.2009.00081.x
Esteves, F. A., Bozelli, R. L., and Roland, F. (1990). Lago Batata: um Laboratório de Limnologia Tropical. Ciência Hoje 11 (64), 26–33.
Falkowski, P. G., and Raven, J. A. (2007). Aquatic Photosynthesis. 2nd ed. Princeton: Princeton University Press, 484.
Flynn, K. J., Stoecker, D. K., Mitra, A., Raven, J. A., Glibert, P. M., Hansen, P. J., et al. (2013). Misuse of the Phytoplankton-Zooplankton Dichotomy: the Need to Assign Organisms as Mixotrophs within Plankton Functional Types. J. Plankton Res. 35 (1), 3–11. January/February 2013. doi:10.1093/plankt/fbs062
Gaskill, J. A., Harris, T. D., and North, R. L. (2020). Phytoplankton Community Response to Changes in Light: Can Glacial Rock Flour Be Used to Control Cyanobacterial Blooms? Front. Environ. Sci. 8, 180. doi:10.3389/fenvs.2020.540607
Gerbersdorf, S. U., and Wieprecht, S. (2015). Biostabilization of Cohesive Sediments: Revisiting the Role of Abiotic Conditions, Physiology and Diversity of Microbes, Polymeric Secretion, and Biofilm Architecture. Geobiology 13 (1), 68–97. doi:10.1111/gbi.12115
Grilo, C. F., Quaresma, V. d. S., Amorim, G. F. L., and Bastos, A. C. (2018). Changes in Flocculation Patterns of Cohesive Sediments after an Iron Ore Mining Dam Failure. Mar. Geology. 400, 1–11. doi:10.1016/j.margeo.2018.03.004
Grobbelaar, J. U. (1992). Nutrients versus Physical Factors in Determining the Primary Productivity of Waters with High Inorganic Turbidity. Hydrobiologia 238, 177–182. doi:10.1007/bf00048786
Guenther, M., and Bozelli, R. (2004). Effects of Inorganic Turbidity on the Phytoplankton of an Amazonian lake Impacted by bauxite Tailings. Hydrobiologia 511, 151–159. doi:10.1023/B:HYDR.0000014095.47409.39
Hart, R. C. (1988). Zooplankton Feeding Rates in Relation to Suspended Sediment Content: Potential Influences on Community Structure in a Turbid Reservoir. Freshw. Biol. 19, 123–139. doi:10.1111/j.1365-2427.1988.tb00334.x
Heinz Walz GmbH (2003). Phytoplankton Analyzer PHYTO-PAM and Phyto-Win Software V 1.45 System Components and Principles of Operation. Germany, 135p.
Huszar, V. L. M. (2000). “Fitoplâncton,” in Lago Batata: Impacto e recuperação de um ecossistema amazônico. Editors R. L. Bozelli, F. A. Esteves, and F. Roland (Rio de Janeiro, RJ, Brazil: Universidade Federal do Rio de Janeiro/Sociedade Brasileira de Limnologia), 342. 89–104.
IBAMA (2015). Laudo técnico preliminar. Impactos sociais decorrentes do desastre envolvendo o rompimento da barragem de Fundão, em Mariana, Minas Gerais. Available at: http://www.ibama.gov.br/phocadownload/noticiasambientais/laudotecnicopreliminar.pdf (Accessed May 2020).
Izaguirre, I., Allende, L., Escaray, R., Bustingorry, J., Pérez, G., and Tell, G. (2012). Comparison of Morpho-Functional Phytoplankton Classifications in Human-Impacted Shallow Lakes with Different Stable States. Hydrobiologia 698, 203–216. doi:10.1007/s10750-012-1069-1
Izaguirre, I., Sánchez, M. L., Schiaffino, M. R., O’Farrell, I., Huber, P., Ferrer, N., et al. (2015). Which Environmental Factors Trigger the Dominance of Phytoplankton Species across a Moisture Gradient of Shallow Lakes? Hydrobiologia 752, 47–64. doi:10.1007/s10750-014-2007-1
Jeppesen, E., Meerhoff, M., Davidson, T. A., Trolle, D., Søndergaard, M., Lauridsen, T. L., et al. (2014). Climate Change Impacts on Lakes: An Integrated Ecological Perspective Based on a Multi-Faceted Approach, with Special Focus on Shallow Lakes. J. Limnol. 73, 88–111. doi:10.4081/jlimnol.2014.844
Jones, H., Cockell, C. S., Goodson, C., Price, N., Simpson, A., and Thomas, B. (2009). Experiments on Mixotrophic Protists and Catastrophic Darkness. Astrobiology 9, 563–571. doi:10.1089/ast.2008.0283
Josué, I. I. P., Sodré, E. O., Setubal, R. B., Cardoso, S. J., Roland, F., Figueiredo‐Barros, M. P., et al. (2021). Zooplankton Functional Diversity as an Indicator of a Long‐term Aquatic Restoration in an Amazonian lake. Restor. Ecol. 29, 1–10. doi:10.1111/rec.13365
Karsten, U., Herburger, K., and Holzinger, A. (2017). Photosynthetic Plasticity in the green Algal Species Klebsormidium Flaccidum (Streptophyta) from a Terrestrial and a Freshwater Habitat. Phycologia 56, 213–220. doi:10.2216/16-85.1
Katechakis, A., Haseneder, T., Kling, R., and Stibor, H. (2005). Mixotrophic versus Photoautotrophic Specialist Algae as Food for Zooplankton: The Light : Nutrient Hypothesis Might Not Hold for Mixotrophs. Limnol. Oceanogr. 50, 1290–1299. doi:10.4319/lo.2005.50.4.1290
Kirk, J. T. O. (1985). Effects of Suspensoids (Turbidity) on Penetration of Solar Radiation in Aquatic Ecosystems. Hydrobiologia 125, 195–208. doi:10.1007/bf00045935
Kirk, J. T. O. (2011). Light and Photosynthesis in Aquatic Ecosystems. 3rd ed. Cambridge UK: Cambridge University Press. Available from https://www.worldcat.org/title/light-and-photosynthesis-inaquatic-ecosystems/oclc/900461245?referer=br&ht=edition (Retrieved January 2019).
Kirk, K. L., and Gilbert, J. J. (1990). Suspended clay and the Population Dynamics of Planktonic Rotifers and Cladocerans. Ecology 71, 1741–1755. doi:10.2307/1937582
Kirk, K. L. (1991). Inorganic Particles Alter Competition in Grazing Plankton: The Role of Selective Feeding. Ecology 72 (3), 915–923. doi:10.2307/1940593
Komárek, J., and Anagnostidis, K. (1998). “Cyanoprokaryota. 1. Teil Chroococcales,” in Süßwasserflora von Mitteleuropa. Editors H. Ettl, G. Gärtner, H. Heynig, and D. Möllenhauer (Stuttgart: Gustav Fischer Verlag), 1–548.
Komárek, J., and Anagnostidis, K. (2005). “Cyanoprokaryota. 2. Teil Oscillatoriales,” in .Süßwasserflora von Mitteleuropa. Editors B. Büdel, L. Krienitz, G. Gärtner, and M. Schagerl (Munich: Elsevier:Spektrum Akademischer Verlag).
Kruk, C., Huszar, V. L. M., Peeters, E. T. H. M., Bonilla, S., Costa, L., Lürling, M., et al. (2010). A Morphological Classification Capturing Functional Variation in Phytoplankton. Freshwat. Biol. 55, 614–627. doi:10.1111/j.1365-2427.2009.02298.x
Kruk, C., and Segura, A. M. (2012). The Habitat Template of Phytoplankton Morphology-Based Functional Groups. Hydrobiologia 698, 191–202. doi:10.1007/s10750-012-1072-6
Lapa, R. P. (2000). “A bauxita e o Rejeito de Bauxita,” in Lago Batata: Impacto e recuperação de um Ecossistema Amazônico. Editors R. L. Bozelli, F. A. Esteves, and F. Roland (Rio de Janeiro, RJ, Brazil: IB-UFRJ/SBL), 27–35.
Leal, J. J. F., de Assis Esteves, F., Farjalla, V. F., and Enrich-Prast, A. (2003). Effect of Campsurus Notatus on NH4, DOC Fluxes, O2 Uptake and Bacterioplankton Production in Experimental Microcosms with Sediment-Water Interface of an Amazonian Lake Impacted by Bauxite Tailings. Internat. Rev. Hydrobiol. 88 (2), 167–178. doi:10.1002/iroh.200390012
Leal, J. J. F., Esteves, F. A., and Callisto, M. (2004). Distribution of Chironomidae Larvae in an Amazonian Flood-plain lake Impacted by bauxite Tailings (Brazil). Amazoniana 18 (1/2), 109–123.
Levine, S. N., Zehrer, R. F., and Burns, C. W. (2005). Impact of Resuspended Sediment on Zooplankton Feeding in Lake Waihola, New Zealand. Freshw. Biol. 50 (9), 1515–1536. doi:10.1111/j.1365-2427.2005.01420.x
Liljendahl-Nurminen, A., Horppila, J., and Lampert, W. (2008). Physiological and Visual Refuges in a Metalimnion: An Experimental Study of Effects of clay Turbidity and an Oxygen Minimum on Fish Predation. Freshw. Biol. 53 (5), 945–951. doi:10.1111/j.1365-2427.2008.01952.x
Lin, D. S. C., and Pellegrini Caramaschi, É. (2005). Responses of the Fish Community to the Flood Pulse and Siltation in a Floodplain lake of the Trombetas River, Brazil. Hydrobiologia 545, 75–91. doi:10.1007/s10750-005-2186-x
Lind, O. T., Doyle, R., Vodopich, D. S., Trotter, B. G., Limón, J. G., and Dávalos-Lind, L. (1992). Clay Turbidity: Regulation of Phytoplankton Production in a Large, Nutrient-Rich Tropical lake. Limnol. Oceanogr. 37 (3), 549–565. doi:10.2307/2837984
Luimstra, V. M., Verspagen, J. M. H., Xu, T., Schuurmans, J. M., and Huisman, J. (2020). Changes in Water Color Shift Competition between Phytoplankton Species with Contrasting Light‐Harvesting Strategies. Ecology 101 (3), e02951. doi:10.1002/ecy.2951
MacIntyre, H., and Cullen, J. (1996). Primary Production by Suspended and Benthic Microalgae in a Turbid Estuary:time-Scales of Variability in San Antonio Bay, Texas. Mar. Ecol. Prog. Ser. 145, 245–268. doi:10.3354/meps145245
Malmqvist, B., and Rundle, S. (2002). Threats to the Running Water Ecosystems of the World. Envir. Conserv. 29 (2), 134–153. doi:10.1017/S0376892902000097
Mayer, T. (2020). Interactions of Fish, Algae, and Abiotic Factors in a Shallow, Tropical Pond. Hydrobiologia 847, 4145–4160. doi:10.1007/s10750-020-04375-y
Menezes, R. F., Attayde, J. L., Kosten, S., Lacerot, G., e Souza, L. C., Costa, L. S., et al. (2019). Differences in Food Webs and Trophic States of Brazilian Tropical Humid and Semi-arid Shallow Lakes: Implications of Climate Change. Hydrobiologia 829, 95–111. doi:10.1007/s10750-018-3626-8
Merten, G. H., Capel, P. D., and Minella, J. P. G. (2014). Effects of Suspended Sediment Concentration and Grain Size on Three Optical Turbidity Sensors. J. Soils Sediments 14 (7), 1235–1241. doi:10.1007/s11368-013-0813-0
Mitra, A., Flynn, K. J., Tillmann, U., Raven, J. A., Caron, D., Stoecker, D. K., et al. (2016). Defining Planktonic Protist Functional Groups on Mechanisms for Energy and Nutrient Acquisition: Incorporation of Diverse Mixotrophic Strategies. Protist 167 (2), 106–120. Epub 2016 Feb 3. PMID: 26927496. doi:10.1016/j.protis.2016.01.003
Moreira, B. (2014). Estrutura, diversidade e regeneração arbórea de uma Floresta Atlântica secundária submetida à supressão do sub-bosque. Minas GeraisMG, Brazil: Universidade Federal de Juiz de Fora, 62. Instituto de Ciências Biológicas, Universidade Federal de Juiz de Fora). M.Sc., Programa de Pós-graduação em Ecologia Aplicada ao Manejo e Conservação de Recursos Naturais, 2014. Dissertação (Mestrado em Ecologia).
Moreira, F. W. A., Leite, M. G. P., Fujaco, M. A. G., Mendonça, F. P. C., Campos, L. P., and Eskinazi-Sant’Anna, E. M. (2016). Assessing the Impacts of Mining Activities on Zooplankton Functional Diversity. Acta Limnol. Bras. 28, e7. doi:10.1590/s2179-975x0816
MRN (2018). Relatório Monitoramento Ecológico Do Lago Batata (PA, Brasil). Porto Trombetas: Mineração Rio do Norte.
Müller, M. C., Rodriguez-Amaya, D. B., and Lourenço, S. O. (2003). Carotenóides da cianobactéria Synechocystis pevalekii produzida em condições normais e sob limitação de nutrientes. Rev. Bras. Cienc. Farm. 39 (4), 415–423. doi:10.1590/S1516-93322003000400009
Naeem, S., Hahn, D. R., and Schuurman, G. (2000). Producer-Decomposer Co-Dependency Influences Biodiversity Effects. Nature 403, 762–764. doi:10.1038/35001568
Naselli-Flores, L., and Barone, R. (2019). Mixotrophic Phytoplankton Dynamics in a Shallow Mediterranean Water Body: How to Make a Virtue Out of Necessity. Hydrobiologia 831, 33–41. doi:10.1007/s10750-018-3507-1
Nõges, T., and Nõges, P. (1999). The Effect of Extreme Water Level Decrease on Hydrochemistry and Phytoplankton in a Shallow Eutrophic lake. Hydrobiologia 408, 277–283. doi:10.1007/978-94-017-2986-4_30
Panosso, R. F. (1993). Influência do Regime Hidrológico e das Características Morfométricas Sobre Algumas Variáveis Limnológicas de um Lago Amazônico (Lago Batata, PA) Impactado por Rejeito de Bauxita. MSc thesis. Rio de Janeiro, RJ, Brazil: UFRJ, 140.
Parkhill, K. L., and Gulliver, J. S. (2002). Effect of Inorganic Sediment on Whole‐stream Productivity. Hydrobiologia 472, 5–17. doi:10.1023/A:1016363228389
Penha, L. N. (2015). Dinâmica da matéria orgânica no sedimento de um lago amazônico impactado por rejeito de bauxita (lago Batata - Porto Trombetas, PA) e sua importância para o processo de restauração. Rio de Janeiro, Brazil: UFRJ/NUPEM. 70. Dissertação (Mestrado) - Programa de Pós-graduação em Ciências Ambientais e Conservação
Phlips, E. J., Aldridge, F. J., Schelske, C. L., and Crisman, T. L. (1995). Relationships between Light Availability, Chlorophyll a, and Tripton in a Large, Shallow Subtropical lake. Limnol. Oceanogr. 40 (2), 416–421. doi:10.4319/LO.1995.40.2.0416
Pinckney, J. L., Paerl, H. W., Tester, P., and Richardson, T. L. (2001). The Role of Nutrient Loading and Eutrophication in Estuarine Ecology. Environ. Health Perspect. 109, 699–706. doi:10.1289/ehp.01109s5699
Quadra, G. R., Roland, F., Barros, N., Malm, O., Lino, A. S., Azevedo, G. M., et al. (2019). Far-reaching Cytogenotoxic Effects of Mine Waste from the Fundão Dam Disaster in Brazil. Chemosphere 215, 753–757. doi:10.1016/j.chemosphere.2018.10.104
Quaggio, J. A., and Raij, B. V. A. N. (1979). Comparação de métodos rápidos para a determinação da matéria orgânica em solos. Rev. Bras. Ciênc. Solo 3, 184–187.
R Core Team (2019). R: A Language and Environment for Statistical Computing. Vienna, Austria: R Foundation for Statistical Computing. Available at: http://www.R-project.org/.
Reynolds, C. S., Huszar, V., Kruk, C., Naselli-Flores, L., and Melo, S. (2002). Towards a Functional Classification of the Freshwater Phytoplankton. J. Plankton Res. 24, 417–428. doi:10.1093/plankt/24.5.417
Rodrigues, V., Estrany, J., Ranzini, M., de Cicco, V., Martín-Benito, J. M. T., Hedo, J., et al. (2018). Effects of Land Use and Seasonality on Stream Water Quality in a Small Tropical Catchment: The Headwater of Córrego Água Limpa, São Paulo (Brazil). Sci. Total Environ. 622-623, 1553–1561. doi:10.1016/j.scitotenv.2017.10.028
Roland, F., and de Assis Esteves, F. (1993). Dynamics of Phosphorus, Carbon and Nitrogen in an Amazonian lake Impacted by bauxite Tailings (Batata Lake, Pará, Brazil). SIL Proc. 1922-2010 25 (2), 925–930. doi:10.1080/03680770.1992.11900283
Roland, F., and de Assis Esteves, F. (1998). Effects of bauxite Tailing on PAR Attenuation in an Amazonian Crystalline Water lake. Hydrobiologia 377, 1–7. doi:10.1023/a:1003252805671
Roland, F., Esteves, F. A., and Barbosa, F. A. R. (2002). Relationship between Antropogenically Caused Turbidity and Phytoplankton Production in a clear Amazonian Floodplain lake. Amazoniana (Kiel), Germany 1/2, 65–77. XVII.
Roland, F., Esteves, F. A., and Barbosa, F. A. R. (1997). The Influence of bauxite Tailings on the Light Regime and its Consequence on Phytoplankton Primary Production in an Amazonian Floodplain lake. SIL Proc. 1922-2010 26, 765–767. doi:10.1080/03680770.1995.11900819
Roland, F. (1995). Produção primária fitoplanctônica em um lago amazônico impactado por rejeito de bauxita – Lago Batata, Pará. Tese, 117. São Carlos, SP, Brazil: Universidade Federal de São Carlos UFSCar. Programa de Pós-graduação em Ecologia e Recursos Naturais.
Sánchez, L. M., Schiaffino, M. R., Pizarro, H., and Izaguirre, I. (2017). Periphytic and Planktonic Bacterial Community Structure in Turbid and clear Shallow Lakes of the Pampean Plain (Argentina): a CARD-FISH Approach. Lat. Am. J. Aquat. Res. 43 (4), 662–674. doi:10.3856/vol43-issue4-fulltext-5
Scarano, F. R., Bozelli, R. L., Dias, A. T. C., Assireu, A., Capossoli, D. J., de Assis Esteves, F., et al. (2018). “Twenty-Five Years of Restoration of an Igapó Forest in Central Amazonia, Brazil,” in Igapó (Black-Water Flooded Forests) of the Amazon Basin (Cham: Springer), 279–294. doi:10.1007/978-3-319-90122-0_15
Scheffer, M., Rinaldi, S., Gragnani, A., Mur, L. R., and van Nes, E. H. (1997). On the Dominance of Filamentous Cyanobacteria in Shallow, Turbid Lakes. Ecology 78, 277–282. doi:10.1890/0012-9658(1997)078[0272:otdofc]2.0.co;2
Schindler, D. W. (1975). Whole-lake Eutrophication Experiments with Phosphorus, Nitrogen and Carbon. SIL Proc. 1922-2010 19, 3221–3231. doi:10.1080/03680770.1974.11896436
Schreiber, U. (2004). “Pulse-Amplitude-Modulation (PAM) Fluorometry and Saturation Pulse Method: an Overview,” in Chorophyll a Fluorescence: A Signature of Photosynthesis. Editors G. Papageorgiou, and Govindjee (Dordrecht: Springer), 279–319. doi:10.1007/978-1-4020-3218-9_11
Schreiber, U. S., Bilger, W., Hormann, H., and Neubauer, C. (1998). “Chlorophyll Fluorescence as a Diagnostic Tool: Basics and Some Aspects of Practical Relevance,” in Photosynthesis: A Comprehensive Treatise. Editor A. S. Raghavendra (Cambridge: Cambridge University Press), P320–P336.
Segura, F. R., Nunes, E. A., Paniz, F. P., Paulelli, A. C. C., Rodrigues, G. B., Braga, G. Ú. L., et al. (2016). Potential Risks of the Residue from Samarco's Mine Dam Burst (Bento Rodrigues, Brazil). Environ. Pollut. 218, 813–825. doi:10.1016/j.envpol.2016.08.005
Shoup, D. E., and Wahl, D. H. (2009). The Effects of Turbidity on Prey Selection by Piscivorous Largemouth Bass. Trans. Am. Fish. Soc. 138 (5), 1018–1027. doi:10.1577/T09-015.1
Smith, V. H. (1983). Low Nitrogen to Phosphorus Ratios Favor Dominance by Blue-green Algae in lake Phytoplankton. Science 221, 669–671. doi:10.1126/science.221.4611.669
Sommaruga, R. (2015). When Glaciers and Ice Sheets Melt: Consequences for Planktonic Organisms. J. Plankton Res. 37 (3), 509–518. doi:10.1093/plankt/fbv027
Somogyi, B., Pálffy, K., V. -Balogh, K., Botta-Dukát, Z., and Vörös, L. (2017). Unusual Behaviour of Phototrophic Picoplankton in Turbid Waters. PLoS One 12 (3), e0174316. doi:10.1371/journal.pone.0174316
Søndergaard, M., Jensen, J. P., and Jeppesen, E. (2003). Role of Sediment and Internal Loading of Phosphorus in Shallow Lakes. Hydrobiologia 506-509, 135–145. doi:10.1023/B:HYDR.0000008611.12704.dd
Sorribas, M. V., Paiva, R. C. D., Melack, J. M., Bravo, J. M., Jones, C., Carvalho, L., et al. (2016). Projections of Climate Change Effects on Discharge and Inundation in the Amazon basin. Climatic Change 136, 555–570. doi:10.1007/s10584-016-1640-2
Sweka, J. A., and Hartman, K. J. (2001). Effects of Turbidity on Prey Consumption and Growth in brook trout and Implications for Bioenergetics Modeling. Can. J. Fish. Aquat. Sci. 58, 386–393. doi:10.1139/f00-260
They, N. H., Amado, A. M., and Cotner, J. B. (2017). Redfield Ratios in Inland Waters: Higher Biological Control of C:N:P Ratios in Tropical Semi-arid High Water Residence Time Lakes. Front. Microbiol. 8, 1505. doi:10.3389/fmicb.2017.01505
Thompson, F., de Oliveira, B. C., Cordeiro, M. C., Masi, B. P., Rangel, T. P., Paz, P., et al. (2020). Severe Impacts of the Brumadinho Dam Failure (Minas Gerais, Brazil) on the Water Quality of the Paraopeba River. Sci. Total Environ. 705, 135914. 1–6. doi:10.1016/j.scitotenv.2019.135914
Utermohl, H. (1958). Zur Vervollkommnung der quantitativen Phytoplankton-Methodik. Mitt. Int. Ver. Theoret. Angew. Limnol. 9 (1–38), 39p. doi:10.1080/05384680.1958.11904091
van den Hoek, C., Mann, D., and Jahns, H. M. (1995). Algae: An Introduction to Phycology. New York: Cambridge University Press.
Wang, H., Li, H., Sun, K., Huang, H., Zhu, P., and Lu, Z. (2020). Impact of Exogenous Nitrogen on the Cyanobacterial Abundance and Community in Oil-Contaminated Sediment: A Microcosm Study. Sci. Total Environ. 710, 136296. doi:10.1016/j.scitotenv.2019.136296
Yang, S., and Jin, X. (2008). Critical Light Intensities forMicrocystis Aeruginosa, Scenedesmus quadricaudaandCyclotellasp. And Competitive Growth Patterns under Different Light:N:P Ratios. J. Freshw. Ecol. 23, 387–396. doi:10.1080/02705060.2008.9664215
Yao, M., Nan, J., and Chen, T. (2014). Effect of Particle Size Distribution on Turbidity under Various Water Quality Levels during Flocculation Processes. Desalination 354, 116–124. doi:10.1016/j.desal.2014.09.029
Keywords: inorganic turbidity, phytoplankton, chlorophyll-a, freshwater ecosystems, bauxite tailing
Citation: Nunes P, Roland F, Amado AM, Resende NS and Cardoso SJ (2022) Responses of Phytoplanktonic Chlorophyll-a Composition to Inorganic Turbidity Caused by Mine Tailings. Front. Environ. Sci. 9:605838. doi: 10.3389/fenvs.2021.605838
Received: 13 September 2020; Accepted: 23 December 2021;
Published: 01 April 2022.
Edited by:
Oladele Ogunseitan, University of California, Irvine, United StatesReviewed by:
Irina Izaguirre, University of Buenos Aires, ArgentinaKristiina Marita Vuorio, Finnish Environment Institute (SYKE), Finland
Copyright © 2022 Nunes, Roland, Amado, Resende and Cardoso. This is an open-access article distributed under the terms of the Creative Commons Attribution License (CC BY). The use, distribution or reproduction in other forums is permitted, provided the original author(s) and the copyright owner(s) are credited and that the original publication in this journal is cited, in accordance with accepted academic practice. No use, distribution or reproduction is permitted which does not comply with these terms.
*Correspondence: Patrícia Nunes, patricia.nunes@ecologia.ufjf.br