Microplastics Reduce the Negative Effects of Litter-Derived Plant Secondary Metabolites on Nematodes in Soil
- 1Institute of Biology, Freie Universität Berlin, Berlin, Germany
- 2Berlin-Brandenburg Institute of Advanced Biodiversity Research, Berlin, Germany
Microplastics and plant litter are ubiquitous in the soil environment, and both materials can influence soil properties and biota. Plant litter releases secondary metabolites (e.g., phenolic compounds) during the decomposition process, including chemical compounds active in plant defense. Effects of microplastics and plant litter on soil biota have been studied independently but we have limited information about the combined effects of both sources of chemicals. Here, we specifically focused on the interaction between plant litter and microplastics, as well as their potential effects on soil biota (i.e., nematodes). We used soils from a previous experiment that included three different types of microplastic fibers (MFs) and four different types of plant litter, which were incubated in the soil in all combinations of materials. After soil incubation (42 days) in the previous experiment, we here tested for effects on nematodes (Caenorhabditis elegans). Plant litter treatments negatively affected the reproduction of nematodes, but these effects were reduced when the soils were incubated along with MFs. We measured the phenolic concentrations in plant litter extracts in a kinetic experiment and found that phenolic concentrations significantly decreased with some of the MF additions. Our results suggest that microplastics can affect the potential effects of natural chemicals such as plant phenolic compounds. We urge future studies to consider this possibility as a key explanatory process underpinning effects of microplastic in the soil environment.
Introduction
Microplastics (<5 mm) are ubiquitous in the soil environment, and their abundance has reached a level that cannot be ignored (Sridharan et al., 2021; Yang et al., 2021). The potential effects of microplastics in soil have been revealed in a growing body of research evidence (Rillig, 2012; Rillig and Lehmann, 2020). Notably, soil biota (e.g., nematodes and springtails) suffer from microplastics exposure, and their reproduction and behavior can be negatively changed (Kim and An, 2019; Kim et al., 2020). Microplastics alter soil physical properties such as soil aggregation and water holding capacity, and this can directly or indirectly influence plant growth (de Souza Machado et al., 2019; Lozano et al., 2021a; 2021b). Recently, chemical effects have been highlighted as an important mediator of microplastic toxicity. Chemical additives in the microplastics are released during the decomposition or fragmentation processes, and these chemicals can possess toxic properties (Rillig et al., 2021; Zhou et al., 2021). In addition, microplastics can act as an extra compartment for the partitioning and adsorption of chemical pollutants, and this is important since it potentially determines the fate and bioavailability of these other chemicals (Tourinho et al., 2019).
Recent studies regarding the chemical adsorption on microplastics have mainly targeted heavy metals or organic pollutants (e.g., polycyclic aromatic hydrocarbons) as they are toxic and abundant in environmental compartments (Tourinho et al., 2019; Agboola and Benson, 2021). However, there is an enormous number of chemicals in the environment, and some of them (e.g., semiochemicals) play important roles in processes such as inter- and intra-specific communication and defense (Thiel and Breithaupt, 2010; Keller-Costa et al., 2015). For instance, plants synthesize a variety of secondary metabolites to protect themselves from pathogens (Ahuja et al., 2012), fungi (Pusztahelyi et al., 2015), and insects (Chomel et al., 2020). There are more than 100,000 secondary metabolites in the plant kingdom (Wink, 2008), and they can be distinguished into three broad classes (alkaloids, phenolic, and terpenes) (Chomel et al., 2016). Phenolic compounds are the most abundant secondary metabolites in plants, and are comprised of an aromatic ring with one or more hydroxyl substituents (e.g., flavonoids, phenolic acids, tannins, stilbenes, and lignans) (Bärlocher and Graça, 2020). A large number of studies has evaluated the adsorption of phenolic groups on various adsorbents such as activated carbon and microplastics (Richard et al., 2009; Zhao et al., 2020), but mainly focused on simple phenols and specifically targeted phenolic pollutants (Richard et al., 2009; Tourinho et al., 2019).
Plant secondary metabolites are released into the environment by volatilization/diffusion, leaching from above-ground plant parts, exudation from the plant root, and litter decomposition (Chomel et al., 2016). A recent study found that the mortality of springtails can increase during the decomposition of plant litter containing high concentrations of phenolic metabolites, and the proportion of bioavailable fractions in the litter is strongly linked with the impacts of plant secondary metabolites (Chomel et al., 2020). Here, we specifically focused on testing for chemical correlations between plant litter and microplastics. The secondary metabolites, especially phenolic compounds, can be released during plant litter decomposition, and might be adsorbed on microplastics already present in the soil, as a function of varying properties of both phenolic compounds and microplastics (Richard et al., 2009; Galanakis et al., 2013; Tourinho et al., 2019). This is important since both plant litter and microplastics are very abundant materials in the soil, and such changes in the bioavailability of secondary metabolites would negatively or positively influence soil biodiversity (Chomel et al., 2020). In the present study, we used soils from a previous experiment that included three different microplastic fibers (MFs) and four different types of plant litter. We chose soil nematode Caenorhabditis elegans in order to evaluate the effects of each MF and plant litter since it is one of the most robust models for microplastic toxicity research (Bhagat et al., 2021). Following the previous experiment, we here carried out a nematode assay to test 1) if plant litter-derived secondary metabolites can affect soil nematodes, and 2) if the combined effects of microplastics and plant litter can induce changes compared to either microplastic or litter alone. Total phenolic contents were measured to determine the amount of a representative secondary metabolite group in each plant litter, and adsorption capacities on MFs were estimated by kinetic adsorption experiments.
Materials and Methods
Target MFs and Plant Litter Types From the Previous Experiment
In the previous experiment (Liang et al., 2021) in our lab, we used one polyacrylic (PA, 100% acrylic yarn, Bravo) and two different polyester products (PES1, Rope Paraloc 137 Mamutec polyester white, Hornbach.de; PES2, Knitting Wool, Himalaya). These polymer types are widely used in textiles (Almroth et al., 2018), and known as an important source of microplastics in the soil (Pirc et al., 2016). MFs were prepared by manually cutting each product into approximately 5 mm long pieces, and washed with tap water for 5 min, after which they were dried at 60°C for 24 h. Each MF was microwaved for 3 min to reduce any microbial contamination on the materials. Size ranges of each MF were as previously reported (Liang et al., 2021): 4.05 ± 0.1.14 (PA), 4.56 ± 0.94 (PES1), and 4.20 ± 1.37 mm (PES2).
Four different types of plant litter were used in that study: hemp stems (Cannabis spp., MultiFit, Item no. 1,008,159, Krefeld, Germany), Medicago lupulina leaves, Plantago lanceolata leaves, and wheat straw (Triticum spp. REAL NATURE, Item no. 1,259,176, Krefeld, Germany). Medicago and Plantago were collected from plants previously grown in our greenhouse. Hemp and wheat served as examples of woody plant litter and agricultural amendments, respectively, and Medicago and Plantago were used to represent typical species from local grassland plant communities. The C:N ratios of the different plant litter type were 153.04 ± 0.70 (hemp), 12.85 ± 0.11 (Medicago), and 14.76 ± 0.29 (Plantago), 133.03 ± 2.18% (wheat) (Liang et al., 2021). Each plant litter was cut using a blender, and sieved to keep the size ranges between 0.5 and 2 mm.
Soil Samples Containing MFs and Plant Litter
We used soils from the previous soil incubation experiment conducted in our lab; this experiment used all combinations of each MF and plant litter (Liang et al., 2021). Soil samples were collected from this experiment in order to address our research questions. Briefly, in this previous experiment, fresh soil (sandy loam; Albic Luvisol) was collected from a local grassland (Berlin, Germany) (Rillig et al., 2010), and sieved by < 0.5 mm in order to reduce the amount of large soil aggregates. Each MF (3 types) and plant litter (4 types) was mixed into the soil with dry-weight based concentrations (0.3% for MFs; 0.8% for plant litter). Similarly, MF-plant litter mixtures (3 MFs x four plant litter types = 12 combinations) were prepared by using the same concentrations. Each concentration of MF (0.3%) and plant litter (0.8%) was determined according to the contamination range in a plastic industrial area (0.03–6.7%) (Fuller and Gautam, 2016) and the saturation level of plant litter addition (Liang et al., 2021), respectively. A control without MFs and plant litter was also included. Thus, a total of 20 treatments (3 MFs +4 plant litters +12 combinations +1 control) were prepared (n = 8). Deionized water was added to the soil until it reached a 60% of water holding capacity (18.83%, g water g−1 dry soil). Soil was incubated at 25°C in the dark for 42 days (see additional details in Liang et al., 2021). After 42 days of incubation, each soil was air-dried, and sieved through a 2 mm-sieve, after which used it for the nematode assay.
Nematode Assay
We obtained the nematode C. elegans (wild type, Bristol strain N2) from the Berlin Institute for Medical Systems Biology at the Max Delbrück Center for Molecular Medicine (Berlin, Germany). They were maintained on Nematode Growth Medium (NGM; NaCl 3 g L−1, peptone 2.5 g L−1, agar 17 g L−1, 1 M potassium phosphate 25 ml L−1, 1 M CaCl2?2H2O 1 ml L−1, 1 M MgSO4?7H2O 1 ml L−1, cholesterol 1 ml L−1) at 20 ± 2 °C in the dark with Escherichia coli (strain OP50) as a food source (Brenner, 1974). We added a Clorox solution (1 N NaOH:5% NaOCl, 1:1) into 3 days-cultured plates to synchronize the developmental stage of nematodes. After 20 min, the suspension was centrifuged at 5,000 rpm for 2 min to collect embryos, and the pellets were washed three times using K-medium (0.032 M KCl, 0.051 M NaCl) (Williams and Dusenbery, 1990). The embryos were transferred onto a new NGM plate, and incubated for 65 h for the nematode assay.
The nematode assay was conducted following Kim et al. (2020). We focused on number of offspring as a target parameter since previous studies have pointed out that the extracts from plant litters can inhibit the hatching of embryos (Meyer et al., 2013; Li et al., 2018). Briefly, we added 0.3 g of each test soil into each well of a 24-well plate (n = 6), moisturized with 82 μL of K-medium (80% of WHC). Then ten age-synchronized worms were added to each well, and maintained at 20 ± 2°C in the dark for 24 h. After this time, each test soil containing nematodes was placed onto soil-agar isolation plates. This soil-agar isolation plates were prepared according to Kim et al. (2020). Shortly, E. coli (strain OP50) was inoculated into Luria-Bertani medium (25 g L−1), and cultured at 37 °C overnight. Then, 75 μL of cell suspension was added on each side of a NGM agar plate, and dried in a sterile hood for 2 h. Each test soil was linearly arranged in the central area of the soil-agar isolation plate, and each plate was incubated at 20 ± 2°C in the dark for 3 h. The newly born offspring in test soils moved to each side of the soil-agar isolation plate searching for food, and we counted the number of offspring on each side (n = 6). The data were expressed as a percentage (%) of offspring compared to the average value of the control group.
Extractable Phenolic Content in Plant Litter
In order to measure extractable phenolic content in each plant litter, the Folin-Ciocalteu method was used to determine total phenolic concentration. This method is commonly used to measure phenolics in plant extracts (Folin and Ciocalteu, 1927; Bärlocher and Graça, 2020), and we chose various extraction solutions since the phenolic extraction highly depends on them (Galanakis et al., 2013). One hundred milligram of each plant litter was added into 15 ml-test tube containing 5 ml of four different extraction solutions (deionized water, 80% acetone, 80% ethanol, and 80% methanol), and each tube was shaken (200 rpm) for 60 min at room temperature. Then, each solution was centrifuged at 13,000 rpm for 5 min, and 0.1 ml of each supernatant was mixed with 0.5 ml of 2% Na2CO3 solution (in 0.1 M NaOH). After 5 min, 0.05 ml of Folin-Ciocalteu reagent (F-9252, Sigma) was added, and the reaction was completed after 20 min. Blank sets (no plant litter or MPs addition) of each extraction solution were prepared, and the absorbances of each solution were measured at 765 nm in a UV/Vis spectrophotometer (Benchmark Plus Microplate Spectrophotometer System, BioRad Laboratories, Hercules, CA, United States). Three replicates were analyzed for each extract, and data were corrected for the mean absorbance value of the blank. A calibration curve of tannic acid (50–250 mg L−1) was prepared (Bärlocher and Graça, 2020), and the regression equation of the calibration curve was determined (y = 0.0028x+0.0144, R2 = 0.9935) (Supplementary Figure S1). Absorbance data were expressed as milligram tannic acid equivalents per Gram of plant litter.
Testing Adsorption of Phenolic Compounds on Microplastics
For adsorption tests, each MF type (PA, PES1, and PES2) was added into each plant litter extract solution (hemp, Medicago, Plantago, and wheat), and total phenolic concentrations were recorded during four time intervals (0, 24, 48 and 96 h). In order to prepare water extracts, 400 mg of each plant litter was added into 20 ml of deionized water, and shaken (200 rpm) for 60 min at room temperature, after which it was filtered using 0.025 μm-membrane filters (Whateman, Maidstone, Kent, UK). A volume of 10 ml of each water extract was added into a 15 ml-test tube containing 50 mg of each MF, and agitated (200 rpm) at room temperature. After predetermined time intervals (0, 24, 48 and 96 h), the Folin-Ciocalteu method was used to measure total phenolic concentrations in each water extract. Three replicates were used for each time interval, and data were expressed as percentage (%) of initial total phenolic concentrations (0 h) in each extract.
In order to prepare solvent extracts for solvents other than water (80% acetone, 80% ethanol, and 80% methanol), we used a different extraction process, since the content of extraction solvents for adsorption tests should usually not exceed 0.1% (OECD, 2000). We prepared each solvent extract stock (1 g plant litter 10 ml−1 solvent), and each solution was shaken (200 rpm) for 60 min at room temperature, after which we centrifuged at 13,000 rpm for 5 min. We diluted each stock solution 1,000-fold with deionized water (approximately solvent content 0.08%), but could not detect their phenolic concentrations by the Folin-Ciocalteu method. Using an alternative method, we mixed 2.5 ml of each stock solution with 22.5 ml of deionized water (10-fold dilution, approximately solvent content 8%), and evaporated each solution in a hood in the dark. Since the evaporation rates of each solvent in our hood was 0.240 ± 0.010 (acetone), 0.087 ± 0.027 (ethanol), and 0.115 ± 0.014 (methanol) mL h−1, we maintained each solution for 24 h to approximately evaporate each solvent (2.5 ml). Total phenolic concentrations of each solvent extract slightly increased after the evaporation process (Supplementary Figure S2). A volume of 10 ml of each solvent extract was added into a 15 ml-test tube containing 50 mg of each MF, and agitated (200 rpm) at room temperature. After each time interval (0, 24, 48 and 96 h), the Folin-Ciocalteu method was used to measure total phenolic concentration.
Statistical Analysis
Data were analyzed using the OriginPro software (OriginPro 8 SR2, Ver. 8.0891, OriginLab Corporation, MA, United States). One-way analysis of variance (ANOVA) and Tukey’s tests were conducted to determine the significance (p < 0.05) of multiple comparisons.
Results
Effects of MFs and Plant Litter on Soil Nematodes
MF (PA, PES1, and PES2) showed no effect on nematode reproduction, whereas each type of plant litter significantly decreased the number of offspring to 66 ± 14 (hemp), 73 ± 10 (Medicago), 65 ± 11 (Plantago), and 61 ± 11 (wheat) % compared to the control group (Figure 1). The adverse effects of each type of plant litter were reduced by each MF addition, and these consequences depended on the combination of type of MF and plant litter (Figure 2). The number of offspring in MF-hemp soil mixtures increased to 76 ± 9 (PA), 91 ± 12 (PES1), and 96 ± 7 (PES2) %, and showed a clear increase in PES1 and PES2 treatments compared to only hemp treatment. The number of nematode offspring increased with Medicago when PA was added (94 ± 5%), and with Plantago litter when PA (83 ± 11%) and PES2 (86 ± 10%) were added. However, MF-wheat soil mixtures showed no change in nematodes reproduction compared to the only-wheat treatment.
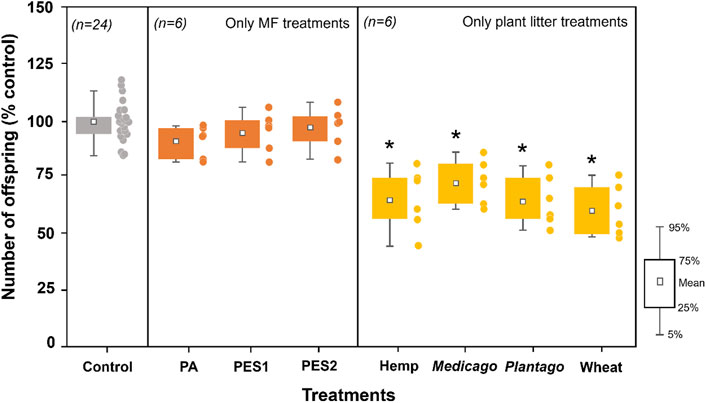
FIGURE 1. Effects of MFs (PA, PES1, and PES2) and different types of plant litter (hemp, Medicago, Plantago, and wheat) on nematode reproduction (number of offspring) in the incubated soils. All data are normalized to control group (% control), and the asterisks (*) indicate significant (p < 0.05) differences compared to the control group. Tukey’s multiple comparison tests were used after one-way analyses of variance.
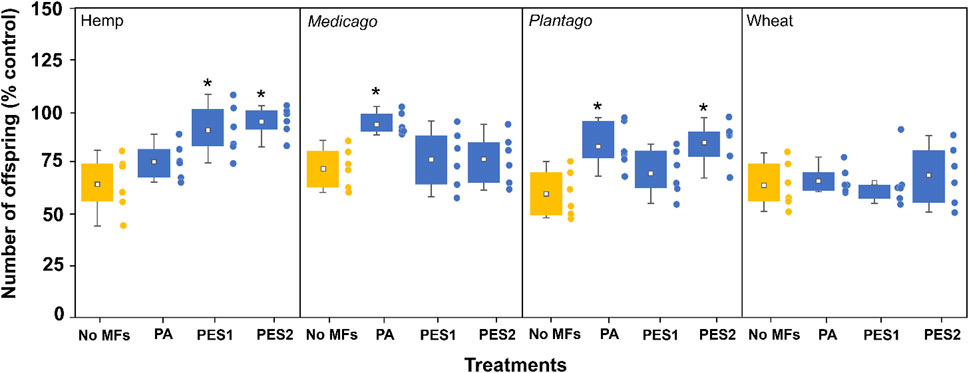
FIGURE 2. Effects of the combinations of each MF (PA, PES1, and PES2) and different types of plant litter (hemp, Medicago, Plantago, and wheat) on nematode reproduction (number of offspring) in the incubated soils. All data are normalized to control group (% control), and the data for single treatments of each type of plant litter (yellow bars) are adopted from Figure 1. The asterisks (*) indicate significant (p < 0.05) differences compared to single treatments of each plant litter. Tukey’s multiple comparison tests were used after one-way analyses of variance.
Extractable Phenolic Contents in Plant Litter
The water extractable phenolic contents in each plant litter were 1.34 ± 0.04 (hemp), 13.05 ± 0.47 (Medicago), 6.53 ± 0.12 (Plantago), and 5.68 ± 0.24 (wheat) mg g−1 (Figure 3). The solvent extractable phenolic contents of hemp were determined as 4.95 ± 0.46 mg g−1 (acetone) 0.82 ± 0.50 mg g−1 (ethanol), and 3.88 ± 0.90 mg g−1 (methanol), and each solvent extractable contents of Medicago was relatively lower (7.24–8.12 mg g−1) than the water extractable content. The solvent extractable contents of Plantago were 6.48 ± 0.69 (acetone), 7.54 ± 1.22 (ethanol), and 5.29 ± 0.33 (methanol), and those of wheat were 7.20 ± 0.37 (acetone), 4.28 ± 1.24 (ethanol), and 6.20 ± 0.61 (methanol) mg g−1. Overall, Medicago and Plantago (leave litters) showed a low variation between each solvent extractable contents, and Medicago seemed to have high level of the water extractable content. The phenolic contents in hemp and wheat (stem and straw litters) was more easily extracted by acetone and methanol, but seemed to contain a low level of the ethanol extractable contents.
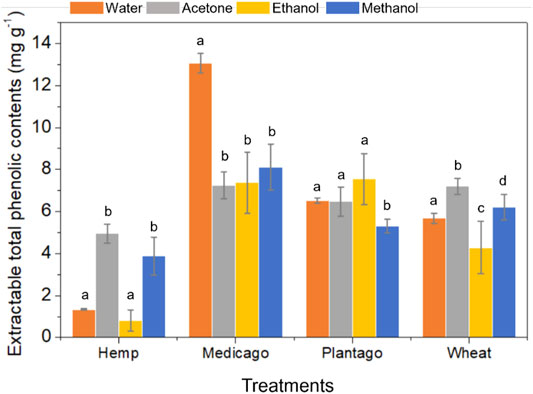
FIGURE 3. Extractable total phenolic contents in each plant litter type (hemp, Medicago, Plantago, and wheat). Each plant litter extract was prepared using four different extraction solutions (deionized water, 80% acetone, 80% ethanol, and 80% methanol), and the Folin-Ciocalteu method was used to determine total phenolic concentrations. Significant differences (p < 0.05; denoted with a-d) were determined using Tukey’s multiple comparison tests after one-way analyses of variance.
Adsorption of Plant Phenolic Compounds on MFs
In the results of water extracts, total phenolic concentrations at the initial time (0 h) were determined as 2.28 ± 0.45 (hemp), 14.80 ± 0.83 (Medicago), 8.47 ± 0.92 (Plantago), and 7.28 ± 0.80 mg g−1 (wheat). The phenolic concentration in hemp extract significantly decreased with PA and PES2 additions compared to each initial concentration (Figure 4A), but other plant litter extracts (Medicago, Plantago, and wheat) showed no change. In the results of solvent extracts, we observed each total phenolic coentration at initial time (0 h, after evaporation): 6.72 ± 0.45 (hemp), 9.07 ± 0.23 (Medicago), 10.33 ± 1.52 (Plantago), and 8.28 ± 0.60 mg g−1 (wheat) for the acetone extracts, 4.15 ± 0.74 (hemp), 8.14 ± 0.20 (Medicago), 9.49 ± 2.36 (Plantago), and 6.51 ± 0.95 mg g−1 (wheat) for the ethanol extracts, 4.55 ± 0.88 (hemp), 8.82 ± 0.72 (Medicago), 8.68 ± 0.30 (Plantago), and 8.90 ± 0.66 mg g−1 (wheat) for the methanol extracts. The acetone extract of Plantago was the only treatment group that showed a reduction of phenolic concentration with PES1 addition, and there was no significant difference in other acetone extracts (Figure 4B). In the results of ethanol extracts, the phenolic concentrations in hemp-PES2, Medicago-PES1, Plantago-PES1, and Plantago-PES2 treatments were gradually reduced (Figure 4C). The methanol extracts of hemp and Medicago were influenced by all MFs (PA, PES1, and PES2), but Plantago and wheat showed no significant differences (Figure 4D).
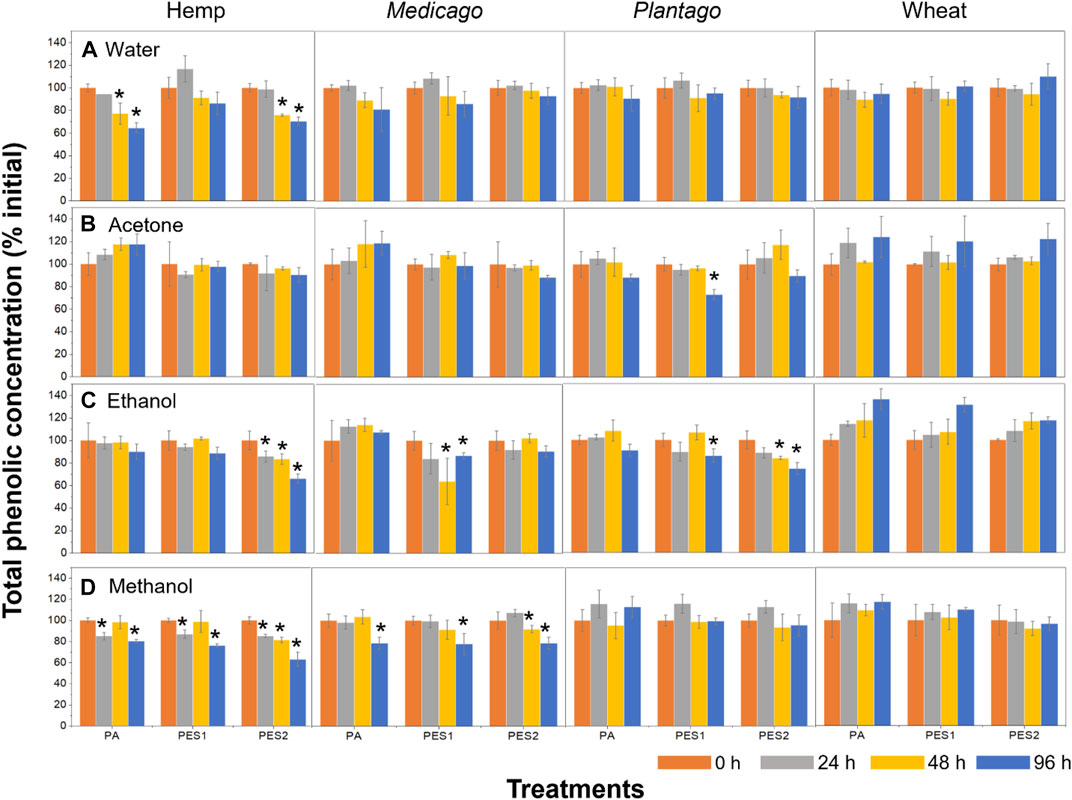
FIGURE 4. Total phenolic concentrations in each plant litter with each MF addition. Each plant litter extract was prepared using (A) deionized water (B) 80% acetone (C) 80% ethanol, and (D) 80% methanol, and each extract was shaken with each MF for 96 h. The total phenolic concentrations was measured at each time interval (0, 24, 48, and 96 h). The asterisks (*) indicate significant (p < 0.05) differences compared to the initial concentrations (0 h) of each treatment. Tukey’s multiple comparison tests were used after one-way analyses of variance.
Discussion
Negative Effects of Plant Litters Is Neutralized by Microplastics
C. elegans is one of the most robust models to evaluate microplastic toxicity (Bhagat et al., 2021). Although a large number of studies has been reported, there is just one study conducted with MFs in soil (Kim et al., 2020). Since the potential effects of MFs would depend on many factors (e.g., chemical composition), we need more data for various MF samples. A recent study found that polyacrylonitrile MFs can decrease nematode reproduction in the soil, which was found to be correlated with the extractable chemical additives in the MFs (Kim et al., 2020). Contrary to this, we could not find an effect of MFs here, which is likely because of toxicity reduction due to the loss of easily extractable additives in the microplastics (Kim et al., 2020; Pflugmacher et al., 2021) as a result of the pre-treatment (e.g., washing) of the material in our study. Microplastics contain “easily” extractable additives on their surface, and a large part of them could be removed by first washing (Kim et al., 2020). For the plant litter additions, we found negative effects of each plant litter on nematodes, an effect likely related to the release of secondary metabolites that are present in plant litter (Supplementary Table S1). Several studies have reported that the extracts from dried plant litter can inhibit the hatching of embryos (Meyer et al., 2013; Li et al., 2018), and Katiki et al. (2013) has reported that plant extracts containing condensed or hydrolysable tannins can induce high mortality on C. elegans.
Although plant litter is as an important nutrient source for decomposer communities (Marschner and Kalbitz, 2003; Joly et al., 2016), the proportion of easily-available secondary metabolites in plant litter has also been linked with negative effects on soil biota (Chomel et al., 2020). We found that the adverse effects of plant litter on nematode reproduction decreased with MF additions, an effect that we expected to be due to decreased bioavailability of secondary metabolites following their adsorption on the MFs. Although chemical pollutants adsorbed on microplastics can induce toxic effects on soil organisms (Yu et al., 2021), several antagonistic effects have been also observed (Sleight et al., 2017; Zhang et al., 2018). Here we avoided nematodes feeding on microplastics, because the size range of each MFs was larger than the edible size (≤3.4 μm) (Fueser et al., 2019); as a consequence, bioavailable secondary metabolites in the medium, i.e. the soil, may have decreased by the adsorption on the MFs. Alternatively, effects of MFs in the soil, which could have included changes in microbial activity and soil physicochemical characteristics, could have led to a differential decomposition of the litter material and phenolic compounds leaching from them. In the previous experiment (Liang et al., 2021) in our lab, plant litter addition increased enzyme activities in the soil, but these effects were reduced with MF addition. When MFs become incorporated into soil aggregates, this generally lead to decreased aggregate water stability, and this could result in a shift in microbial activity (de Souza Machado et al., 2019). This could be linked with decreased litter decomposition and release of secondary metabolites.
Adsorption of Plant Phenolic Compounds on MFs
The release of secondary metabolites is closely correlated with litter mass loss and different phases of the decomposition process. (Chomel et al., 2016). The plant litter would be chemically and biologically decomposed during the soil incubation, and various phenolic compounds would be released. We considered the potentially extractable compounds in the litter mass, using three different extraction solutions. The phenolic compounds are concentrated in specific plant tissues such as leaves (1–25% of dry mass) (Hättenschwiler and Vitousek, 2000), and this might be a reason why Medicago and Plantago (leaf litter) contain higher phenolic contents in the present study. We also observed solvent-dependent differences in the stem and straw litter (hemp and wheat), whereas the leaf litter types (Medicago and Plantago) had low variation (Figure 3), which can be linked to the different chemical composition between the plant litters that produce the solvent-dependent differences in the extraction yields (Galanakis et al., 2013). For instance, tannin is a major polyphenol with high molecular weight, and it can be divided into hydrolyzable and condensed tannins (Hättenschwiler and Vitousek, 2000). They are widely distributed in woody plants, and certain members of the condensed tannins (procyanidins and prodelphinidins) have been only observed in the stem but not in the leaves (Goufo et al., 2020).
Adsorption and desorption of plant phenolic compounds are considered an efficient method for their recovery and purification (Soto et al., 2011). Hydrophobic carbon materials (e.g., activated carbons), siliceous materials (e.g., clay and zeolites), and synthetic polymeric materials (e.g., resin and polystyrene–divinylbenzene copolymers) efficiently adsorb phenolic compounds and their derivatives (Soto et al., 2011), and the maximum adsorption capacity of salicylic acid on polystyrene–divinylbenzene copolymers has been calculated as 43.01–85.06 mg g−1 (Otero et al., 2005). This implies that plant phenolic compounds can be adsorbed onto polymeric materials, but we have very limited information about microplastics. Our results showed that MFs can decrease total phenolic concentrations of each plant extract (Figure 4), which is indirect evidence of the adsorption of the plant phenolic compounds on MFs. We found some correlations between the adsorption and nematode tests: the effects of wheat litters were not changed by MF additions (Figure 2), and the phenolic compounds in wheat extracts seemed to not be adsorbed on MFs (Figure 4). However, we could not find a clear trend in the solvent-dependent differences, and the adsorption results did not match the reduction of the adverse effects on nematodes. For instance, the adverse effects of hemp litter was reduced by PES1 and PES2 additions (Figure 2), but the phenolic adsorption of hemp extracts occurred in all MF treatments (Figure 4). These inconsistencies may be linked to the fact that specific chemical compounds (e.g., the condensed tannins) can directly affect soil organisms (Poinsot-Balaguer et al., 1993; Das and Joy, 2009), an effect that would not be captured using our detection method, assaying for total amounts. Since plant secondary metabolites vary depending on many factors (plant species, organs, and detection methods), identifying key chemicals that adsorbed onto microplastics requires an elaborate test design that is beyond the scope of our current study. In addition, the adsorptive interactions between the phenolic compounds and microplastics in the actual soil environment might be governed by many factors such as soil chemical properties (pH and ionic strength) (Gao et al., 2017) and soil minerals (Kalbitz et al., 2005). The adsorption capacity of plant phenolic compounds (1,2-Dichlorobenzene phenol) on soil particles have been estimated as 34.27–51.83 mg g−1 (Subramanyam and Das, 2009), which implies that there would be an adsorptive competition between the microplastics and soil particles.
Conclusion
Our results suggest that microplastics can potentially adsorb plant secondary metabolites, affecting their bioavailability in the soil with consequences for soil nematodes. This is the first study calling attention to a potential interaction between plant secondary metabolites and microplastics. We provide indirect evidence that microplastics can alter the potential effects and bioavailability of naturally occurring compounds, such as plant defense chemicals, with potential consequences for soil nutrient cycles and biodiversity. We suggest that future research should consider the interaction between plant-derived chemicals and microplastics since this could be a key explanatory factor of microplastic effects on the soil environment and its biodiversity.
Data Availability Statement
The original contributions presented in the study are included in the article/Supplementary Material, further inquiries can be directed to the corresponding author.
Author Contributions
SK, YL and YL: conceptualization, design of the study, experiment set up, analysis of data, and writing. YL and MR: review and editing. All authors contributed to the article and approved the submitted version.
Funding
We acknowledge support by the Open Access Publication Initiative of Freie Universität Berlin. MR acknowledges support from an ERC Advanced Grant (grant no. 694368) and from the EU H2020 projects MINAGRIS (“Micro- and nano-plastics in agricultural soils”; Grant Agreement number: 101000407) and PAPILLONS (“Plastic in Agricultural Production: Impacts, Lifecycles and Long-term Sustainability”; Grant Agreement number: 101000210). YL acknowledges a scholarship from the China Scholarship Council.
Conflict of Interest
The authors declare that the research was conducted in the absence of any commercial or financial relationships that could be construed as a potential conflict of interest.
Publisher’s Note
All claims expressed in this article are solely those of the authors and do not necessarily represent those of their affiliated organizations, or those of the publisher, the editors and the reviewers. Any product that may be evaluated in this article, or claim that may be made by its manufacturer, is not guaranteed or endorsed by the publisher.
Supplementary Material
The Supplementary Material for this article can be found online at: https://www.frontiersin.org/articles/10.3389/fenvs.2021.790560/full#supplementary-material
References
Agboola, O. D., and Benson, N. U. (2021). Physisorption and Chemisorption Mechanisms Influencing Micro (Nano) Plastics-Organic Chemical Contaminants Interactions: A Review. Front. Environ. Sci. 9. doi:10.3389/fenvs.2021.678574
Ahuja, I., Kissen, R., and Bones, A. M. (2012). Phytoalexins in Defense against Pathogens. Trends Plant Sci. 17, 73–90. doi:10.1016/j.tplants.2011.11.002
Bärlocher, F., and Graça, M. A. S. (2020). “Total Phenolics,” in Methods to Study Litter Decomposition (Cham: Springer), 157–161. doi:10.1007/978-3-030-30515-4_18
Bhagat, J., Nishimura, N., and Shimada, Y. (2021). Worming into a Robust Model to Unravel the Micro/nanoplastic Toxicity in Soil: A Review on Caenorhabditis elegans. Trac Trends Anal. Chem. 138, 116235. doi:10.1016/j.trac.2021.116235
Brenner, S. (1974). The Genetics of Caenorhabditis elegans. Genetics 77, 71–94. doi:10.1093/genetics/77.1.71
Carney Almroth, B. M., Åström, L., Roslund, S., Petersson, H., Johansson, M., and Persson, N.-K. (2018). Quantifying Shedding of Synthetic Fibers from Textiles; a Source of Microplastics Released into the Environment. Environ. Sci. Pollut. Res. 25, 1191–1199. doi:10.1007/s11356-017-0528-7
Chomel, M., Baldy, V., Guittonny, M., Greff, S., and DesRochers, A. (2020). Litter Leachates Have Stronger Impact Than Leaf Litter on Folsomia candida Fitness. Soil Biol. Biochem. 147, 107850. doi:10.1016/j.soilbio.2020.107850
Chomel, M., Guittonny‐Larchevêque, M., Fernandez, C., Gallet, C., DesRochers, A., Paré, D., et al. (2016). Plant Secondary Metabolites: a Key Driver of Litter Decomposition and Soil Nutrient Cycling. J. Ecol. 104, 1527–1541. doi:10.1111/1365-2745.12644
Das, S., and Joy, V. C. (2009). Chemical Quality Impacts of Tropical forest Tree Leaf Litters on the Growth and Fecundity of Soil Collembola. Eur. J. Soil Biol. 45, 448–454. doi:10.1016/j.ejsobi.2009.09.001
de Souza Machado, A. A., Lau, C. W., Kloas, W., Bergmann, J., Bachelier, J. B., Faltin, E., et al. (2019). Microplastics Can Change Soil Properties and Affect Plant Performance. Environ. Sci. Technol. 53, 6044–6052. doi:10.1021/acs.est.9b01339
Folin, O., and Ciocalteu, V. (1927). On Tyrosine and Tryptophane Determination in Proteins. J. Biol. Chem. 27, 239–343.
Fueser, H., Mueller, M. T., Weiss, L., Höss, S., and Traunspurger, W. (2019). Ingestion of Microplastics by Nematodes Depends on Feeding Strategy and Buccal Cavity Size. Environ. Pollut. 255, 113227.
Galanakis, C. M., Goulas, V., Tsakona, S., Manganaris, G. A., and Gekas, V. (2013). A Knowledge Base for the Recovery of Natural Phenols with Different Solvents. Int. J. Food Properties 16, 382–396. doi:10.1080/10942912.2010.522750
Gao, J., Jansen, B., Cerli, C., Helmus, R., Mikutta, R., Dultz, S., et al. (2017). Competition and Surface Conditioning Alter the Adsorption of Phenolic and Amino Acids on Soil Minerals. Eur. J. Soil Sci. 68, 667–677. doi:10.1111/ejss.12459
Goufo, P., Singh, R. K., and Cortez, I. (2020). A Reference List of Phenolic Compounds (Including Stilbenes) in grapevine (Vitis vinifera L.) Roots, Woods, Canes, Stems, and Leaves. Antioxidants 9, 398. doi:10.3390/antiox9050398
Hättenschwiler, S., and Vitousek, P. M. (2000). The Role of Polyphenols in Terrestrial Ecosystem Nutrient Cycling. Trends Ecol. Evol. 15, 238–243. doi:10.1016/s0169-5347(00)01861-9
Joly, F.-X., Fromin, N., Kiikkilä, O., and Hättenschwiler, S. (2016). Diversity of Leaf Litter Leachates from Temperate forest Trees and its Consequences for Soil Microbial Activity. Biogeochemistry 129, 373–388. doi:10.1007/s10533-016-0239-z
Kalbitz, K., Schwesig, D., Rethemeyer, J., and Matzner, E. (2005). Stabilization of Dissolved Organic Matter by Sorption to the mineral Soil. Soil Biol. Biochem. 37, 1319–1331. doi:10.1016/j.soilbio.2004.11.028
Katiki, L. M., Ferreira, J. F. S., Gonzalez, J. M., Zajac, A. M., Lindsay, D. S., Chagas, A. C. S., et al. (2013). Anthelmintic Effect of Plant Extracts Containing Condensed and Hydrolyzable Tannins on Caenorhabditis elegans, and Their Antioxidant Capacity. Vet. Parasitol. 192, 218–227. doi:10.1016/j.vetpar.2012.09.030
Keller-Costa, T., Canário, A. V. M., and Hubbard, P. C. (2015). Chemical Communication in Cichlids: a Mini-Review. Gen. Comp. Endocrinol. 221, 64–74. doi:10.1016/j.ygcen.2015.01.001
Kim, S. W., and An, Y.-J. (2019). Soil Microplastics Inhibit the Movement of Springtail Species. Environ. Int. 126, 699–706. doi:10.1016/j.envint.2019.02.067
Kim, S. W., Waldman, W. R., Kim, T.-Y., and Rillig, M. C. (2020). Effects of Different Microplastics on Nematodes in the Soil Environment: Tracking the Extractable Additives Using an Ecotoxicological Approach. Environ. Sci. Technol. 54, 13868–13878. doi:10.1021/acs.est.0c04641
Li, T., Wang, H., Xia, X., Cao, S., Yao, J., and Zhang, L. (2018). Inhibitory Effects of Components from Root Exudates of Welsh Onion against Root Knot Nematodes. PLoS One 13, e0201471. doi:10.1371/journal.pone.0201471
Liang, Y., Lehmann, A., Yang, G., Leifheit, E. F., and Rillig, M. C. (2021). Effects of Microplastic Fibers on Soil Aggregation and Enzyme Activities Are Organic Matter Dependent. Front. Environ. Sci. 9, 97. doi:10.3389/fenvs.2021.650155
Lozano, Y. M., Aguilar‐Trigueros, C. A., Onandia, G., Maaß, S., Zhao, T., and Rillig, M. C. (2021b). Effects of Microplastics and Drought on Soil Ecosystem Functions and Multifunctionality. J. Appl. Ecol. 58, 988–996. doi:10.1111/1365-2664.13839
Lozano, Y. M., Lehnert, T., Linck, L. T., Lehmann, A., and Rillig, M. C. (2021a). Microplastic Shape, Polymer Type, and Concentration Affect Soil Properties and Plant Biomass. Front. Plant Sci. 12, 169. doi:10.3389/fpls.2021.616645
Marschner, B., and Kalbitz, K. (2003). Controls of Bioavailability and Biodegradability of Dissolved Organic Matter in Soils. Geoderma 113, 211–235. doi:10.1016/s0016-7061(02)00362-2
Meyer, S. L. F., Nyczepir, A. P., Rupprecht, S. M., Mitchell, A. D., Martin, P. A. W., Brush, C. W., et al. (2013). Tall Fescue 'Jesup (Max‐Q)': Meloidogyne incognita Development in Roots and Nematotoxicity. Agron.j. 105, 755–763. doi:10.2134/agronj2012.0374
OECD (2000). Adsorption - Desorption Using a Batch Equilibrium Method. Paris, France: OECD Publishing. OECD Guidelines for the Testing of Chemicals. Section 1 , Test No. 106.
Otero, M., Zabkova, M., Grande, C. A., and Rodrigues, A. E. (2005). Fixed-bed Adsorption of Salicylic Acid onto Polymeric Adsorbents and Activated Charcoal. Ind. Eng. Chem. Res. 44, 927–936. doi:10.1021/ie049227j
Pflugmacher, S., Tallinen, S., Kim, Y. J., Kim, S., and Esterhuizen, M. (2021). Ageing Affects Microplastic Toxicity over Time: Effects of Aged Polycarbonate on Germination, Growth, and Oxidative Stress of Lepidium Sativum. Sci.Total Environ. 790, 148166. doi:10.1016/j.scitotenv.2021.148166
Pirc, U., Vidmar, M., Mozer, A., and Kržan, A. (2016). Emissions of Microplastic Fibers from Microfiber Fleece during Domestic Washing. Environ. Sci. Pollut. Res. 23, 22206–22211. doi:10.1007/s11356-016-7703-0
Poinsot-Balaguer, N., Racon, L., Sadaka, N., and Le Petit, J. (1993). Effects of Tannin Compounds on Two Species of Collembola. Eur. J. Soil Biol. 29, 13–16.
Pusztahelyi, T., Holb, I. J., and Pã³csi, I. n. (2015). Secondary Metabolites in Fungus-Plant Interactions. Front. Plant Sci. 6, 573. doi:10.3389/fpls.2015.00573
Richard, Dominique., Núñez, Maria. de. Lourdes. Delgado., and Schweich, Daniel. (2009). Adsorption of Complex Phenolic Compounds on Active Charcoal: Adsorption Capacity and Isotherms. Chem. Eng. J. 148, 1–7. doi:10.1016/j.cej.2008.07.023
Rillig, M. C., Kim, S. W., Kim, T.-Y., and Waldman, W. R. (2021). The Global Plastic Toxicity Debt. Environ. Sci. Technol. 55, 2717–2719. doi:10.1021/acs.est.0c07781
Rillig, M. C., and Lehmann, A. (2020). Microplastic in Terrestrial Ecosystems. Science 368, 1430–1431. doi:10.1126/science.abb5979
Rillig, M. C., Mardatin, N. F., Leifheit, E. F., and Antunes, P. M. (2010). Mycelium of Arbuscular Mycorrhizal Fungi Increases Soil Water Repellency and Is Sufficient to Maintain Water-Stable Soil Aggregates. Soil Biol. Biochem. 42, 1189–1191. doi:10.1016/j.soilbio.2010.03.027
Rillig, M. C. (2012). Microplastic in Terrestrial Ecosystems and the Soil. Environ. Sci. Technol. 46, 6453–6454. doi:10.1021/es302011r
Sleight, V. A., Bakir, A., Thompson, R. C., and Henry, T. B. (2017). Assessment of Microplastic-Sorbed Contaminant Bioavailability through Analysis of Biomarker Gene Expression in Larval Zebrafish. Mar. Pollut. Bull. 116, 291–297. doi:10.1016/j.marpolbul.2016.12.055
Soto, M. L., Moure, A., Domínguez, H., and Parajó, J. C. (2011). Recovery, Concentration and Purification of Phenolic Compounds by Adsorption: A Review. J. Food Eng. 105, 1–27. doi:10.1016/j.jfoodeng.2011.02.010
Sridharan, S., Kumar, M., Bolan, N. S., Singh, L., Kumar, S., Kumar, R., et al. (2021). Are Microplastics Destabilizing the Global Network of Terrestrial and Aquatic Ecosystem Services. Environ. Res. 198, 111243. doi:10.1016/j.envres.2021.111243
Subramanyam, B., and Das, A. (2009). Study of the Adsorption of Phenol by Two Soils Based on Kinetic and Isotherm Modeling Analyses. Desalination 249, 914–921. doi:10.1016/j.desal.2009.05.020
Thiel, M., and Breithaupt, T. (2010). “Chemical Communication in Crustaceans: Research Challenges for the Twenty-First century,” in Chemical Communication in Crustaceans (New York, NY: Springer), 3–22. doi:10.1007/978-0-387-77101-4_1
Tourinho, P. S., Kočí, V., Loureiro, S., and van Gestel, C. A. M. (2019). Partitioning of Chemical Contaminants to Microplastics: Sorption Mechanisms, Environmental Distribution and Effects on Toxicity and Bioaccumulation. Environ. Pollut. 252, 1246–1256. doi:10.1016/j.envpol.2019.06.030
Williams, P. L., and Dusenbery, D. B. (1990). Aquatic Toxicity Testing Using the Nematode,Caenorhabditis elegans. Environ. Toxicol. Chem. 9, 1285–1290. doi:10.1002/etc.5620091007
Wink, M. (2008). Plant Secondary Metabolism: Diversity, Function and its Evolution. Nat. Prod. Commun. 3, 1934578X0800300801. doi:10.1177/1934578x0800300801
Yang, L., Zhang, Y., Kang, S., Wang, Z., and Wu, C. (2021). Microplastics in Soil: A Review on Methods, Occurrence, Sources, and Potential Risk. Sci. Total Environ. 146546. doi:10.1016/j.scitotenv.2021.146546
Yu, Y., Mo, W. Y., and Luukkonen, T. (2021). Adsorption Behaviour and Interaction of Organic Micropollutants with Nano and Microplastics–A Review. Sci.Total Environ. 797, 149140. doi:10.1016/j.scitotenv.2021.149140
Zhang, H., Wang, J., Zhou, B., Zhou, Y., Dai, Z., Zhou, Q., et al. (2018). Enhanced Adsorption of Oxytetracycline to Weathered Microplastic Polystyrene: Kinetics, Isotherms and Influencing Factors. Environ. Pollut. 243, 1550–1557. doi:10.1016/j.envpol.2018.09.122
Zhao, H.-J., Xu, J.-K., Yan, Z.-H., Ren, H.-Q., and Zhang, Y. (2020). Microplastics Enhance the Developmental Toxicity of Synthetic Phenolic Antioxidants by Disturbing the Thyroid Function and Metabolism in Developing Zebrafish. Environ. Int. 140, 105750. doi:10.1016/j.envint.2020.105750
Keywords: adsorption, Caenorhabditis elegans, fiber, phenolic compounds, solvent
Citation: Kim SW, Liang Y, Lozano YM and Rillig MC (2021) Microplastics Reduce the Negative Effects of Litter-Derived Plant Secondary Metabolites on Nematodes in Soil. Front. Environ. Sci. 9:790560. doi: 10.3389/fenvs.2021.790560
Received: 06 October 2021; Accepted: 05 November 2021;
Published: 25 November 2021.
Edited by:
Ilika Ghosh, Max Planck Florida Institute for Neuroscience (MPFI), United StatesReviewed by:
Defu He, East China Normal University, ChinaLal Singh, National Environmental Engineering Research Institute (CSIR), India
Copyright © 2021 Kim, Liang, Lozano and Rillig. This is an open-access article distributed under the terms of the Creative Commons Attribution License (CC BY). The use, distribution or reproduction in other forums is permitted, provided the original author(s) and the copyright owner(s) are credited and that the original publication in this journal is cited, in accordance with accepted academic practice. No use, distribution or reproduction is permitted which does not comply with these terms.
*Correspondence: Shin Woong Kim, swkim@zedat.fu-berlin.de