Impact of Land-Use Changes on Soil Properties and Carbon Pools in India: A Meta-analysis
- 1Department of Soil Science and Agricultural Chemistry, Bihar Agricultural University, Sabour, India
- 2ICAR-National Rice Research Institute, Cuttack, India
- 3International Rice Research Institute-India Office, New Delhi, India
- 4International Maize and Wheat Improvement Center-India Office, New Delhi, India
- 5ICAR-Indian Institute of Rice Research, Hyderabad, India
- 6Uttar Banga Krishi Viswavidyalaya, Coochbehar, India
- 7ICAR-Indian Agricultural Research Institute, New Delhi, India
- 8CSIRO-Agriculture and Food, Urrbrae, SA, Australia
Land-use changes (LUC), primarily due to deforestation and soil disturbance, are one of the major causes of soil quality degradation and greenhouse gas emissions. Effects of LUC on soil physicochemical properties and changes in soil quality and land use management strategies that can effectively restore soil carbon and microbial biomass levels have been reported from all over the world, but the impact analysis of such practices in the Indian context is limited. In this study, over 1,786 paired datasets (for meta-analysis) on land uses (LUs) were collected from Indian literature (1990–2019) to determine the magnitude of the influence of LUC on soil carbon, microbial biomass, and other physical and chemical properties at three soil depths. Meta-analysis results showed that grasslands (36.1%) lost the most soil organic carbon (SOC) compared to native forest lands, followed by plantation lands (35.5%), cultivated lands (31.1%), barren lands (27.3%), and horticulture lands (11.5%). Our findings also revealed that, when compared to forest land, the microbial quotient was lower in other LUs. Due to the depletion of SOC stock, carbon dioxide equivalent (CO2 eq) emissions were significantly higher in all LUs than in forest land. Results also showed that due to the conversion of forest land to cultivated land, total carbon, labile carbon, non-labile carbon, microbial biomass carbon, and SOC stocks were lost by 21%, 25%, 32%, 26%, and 41.2%, respectively. Changes in soil carbon pools and properties were more pronounced in surface (0–15 cm) soils than in subsurface soils (15–30 cm and 30–45 cm). Restoration of the SOC stocks from different LUs ranged from a minimum of 2% (grasslands) to a maximum of 48% (plantation lands). Overall, this study showed that soil carbon pools decreased as LUC transitioned from native forestland to other LUs, and it is suggested that adopting crop-production systems that can reduce CO2 emissions from the intensive LUs such as the ones evaluated here could contribute to improvements in soil quality and mitigation of climate change impacts, particularly under Indian agro-climatic conditions.
1 Introduction
Anthropogenic activities have changed the development of livelihood by altering the land-use changes (LUC) in the past century at a very rapid pace (Liu et al., 2005a; 2005b; Hurtt et al., 2006; Liu and Tian, 2010; Tian et al., 2014). The conversion of forest land (FL) into different land use (LU) systems such as barren land (BL), cultivated land (CL), grassland (GL), horticulture land (HL), and plantation land (PL) has been reported at the rate of 13 million hectares (mha) per year through deforestation (FAO, 2006), and sometimes caused a decline in soil quality, thereby reducing its potential for actual productivity (Wei et al., 2014; Nath et al., 2018). Reports from global studies indicated that LUC caused soil degradation resulting from intensive use and uneven terrain coupled with changing climatic conditions (Palni et al., 1998; Abera and Wolde-Meskel, 2013; Kumar et al., 2017; Kumar et al., 2021). This LUC altered the system’s capacity as a carbon source or sink (Abera and Wolde-Meskel, 2013; De Blécourt et al., 2013; Guillaume et al., 2015; Fan et al., 2016; Iqbal and Tiwari, 2016). A loss of soil organic carbon (SOC) and biodiversity due to the conversion of FL into different LUs has been well documented (De Blécourt et al., 2013; Ahrends et al., 2015; Guillaume et al., 2015; Nath et al., 2018). Therefore, quantifying the impacts of LUC is critical to better understand the interactions among human activities, climate systems, and ecosystems and to design government policies (Houghton and Hackler, 2003; Tian et al., 2003; Arora and Boer, 2010).
Detecting the impact of management and LUC in soil carbon pools is likely to be more sensitive than total SOC (Campbell et al., 1997; Padbhushan et al., 2015; Padbhushan et al., 2016a; Padbhushan et al., 2016b; Rakshit et al., 2018; Meetei et al., 2020; Padbhushan et al., 2020). Soil microbial activity is the central process in the terrestrial carbon cycle. The microbial quotient (MQ) refers to the ratio between microbial biomass carbon (MBC) to SOC, which is used as a measure of ecophysiological status of soil microorganisms (Anderson and Domsch, 1993). The MQ value can also reflect about the quality and nature of microbial activity in the soil. A large number of studies on MQ have shown its importance to evaluate or monitor the influence of short- or long-term changes in soil biological status due to management and other system-level manipulations (Brookes, 1995; Bastida et al., 2008; Anderson and Domsch, 2010; Padbhushan et al., 2021). Soil properties and SOC stocks may be altered due to soil disturbances (Guo and Gifford, 2002; Paul et al., 2002). A carbon dioxide equivalent (CO2 eq) emission is a soil indicator that provides information on the amount of carbon loss from SOC stocks into the atmosphere. Through meta-analysis studies, these parameters have been found to be altered in changing LU systems at a global scale (DeFries et al., 2002; Guo and Gifford, 2002; Achard et al., 2004; Houghton, 2008; Don et al., 2011). Therefore, knowledge of soil carbon pools, MQ and CO2 eq emission helps to understand their impacts in a changing LU system.
India is the world’s second largest populous country and is expected to overtake China by 2025 (United Nations Department of Economic and Social Affairs: Population Division, 2019). In India, the human population has increased from 200 million to 1,400 million during 1980–2020 and, coupled with economic growth, has brought significant change in LUs (Tian et al., 2014; World Population Prospects: The 2019 Revision United Nations Population Division, 2020). Total SOC stocks in India are about 20.67 Pg (soil depth 0–30 cm) and 63.19 Pg (soil depth 0–150 cm) covering a total geographical area of 329 m ha. Figure 1A depicts the SOC stocks in different physiographic regions of India (Bhattacharyya et al., 2000). The data are summarized into five categories, namely, Northern Mountains, The Great Plains, Peninsular India, Peninsular Plateau, and Coastal Plains and Islands representing the different physiographic regions of India. In the soil depth 0–30 cm, maximum and minimum SOC stocks have been recorded in Northern Mountains and Coastal Plains and Islands, respectively, whereas in the soil depth 0–150 cm, maximum and minimum were recorded in Northern Mountains and Peninsular Plateau. The area covered by the different regions is shown in Figure 1A.
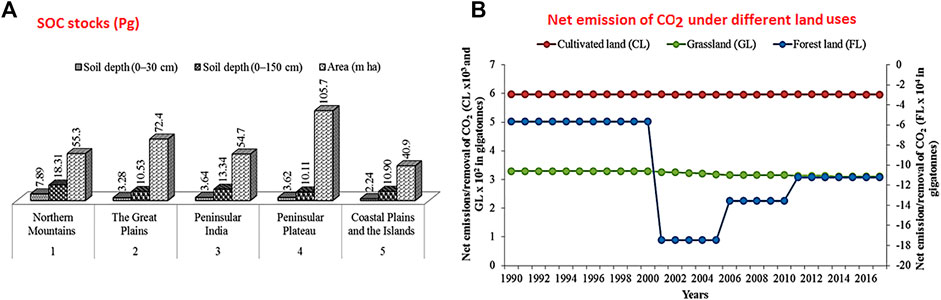
FIGURE 1. (A) Soil carbon stocks in different physiographic regions of India (source: Bhattacharyya et al., 2000). (B) Trend of net emissions/removals of CO2 from forest land (FL), cultivated land (CL), and grassland (GL) (1990–2017) of India (Y1-axis represent CL and GL; Y2-axis represent FL), FAO (http://faostat.fao.org/).
Currently, India ranks third with a share of 7% of total CO2 emissions in the world (IEA, 2019), but ranks 20th in the world for per-capita annual CO2 emissions, which is approximately 1.94 tons, less than half the global average of 4.8 tons CO2 (Ritchie and Roser, 2019). The per-capita emissions of CO2 are lower for India when compared to the other major CO2 emission contributors of the world (except China). During the period 1880–2020, India has experienced a decline in FL of 18 mha (from 89 mha in 1880 to 71 mha in 2019) and expansion of CL by 49 m ha (from 92 mha in 1880 to 141 mha in 2019) resulted from conversion from FL, GL, and BL into CL (Tian et al., 2014; Bodh, 2019). Due to these conversions, a significant reduction in SOC stocks and its impacts on soil quality and ecosystem health have been observed. Supplementary Figure S1 presents the trend for the share of FL and CL systems in the total land area during the period 1990 to 2017. Although the magnitude of change is small, the trends are encouraging, i.e., the FL area has increased from 21.5% to 23.7% while the CL area has decreased from 61.1% to 60.4%. Governmental initiatives in recent years have helped to reduce the rate of deforestation rate in India by reducing deforestation, rectifying deforestation by reforestation, and increasing afforestation by establishing forests in new areas (Don et al., 2011). This is evident from the governments’ effort to increase the area under forests for achieving the long-term target to fetch 33% of total area under FL cover. However, in the past few years, during 2000–2017, net emissions/removals of CO2 by the FL have increased (from −18 × 104 gigatons to −11 × 104 gigatons), whereas net emissions/removal of CO2 of CL and GL remained constant and consistently positive (Figure 1B).
A meta-analysis approach has generally been used to assess the magnitude and direction of treatment effects as well as pattern and sources of heterogeneity by combining the findings from several studies under various environmentally and ecologically variable regions (Hedges et al., 1999; Koricheva et al., 2013). For example, meta-analysis has been used to derive general conclusions in ecology, biogeographic patterns of biota, carbon, and nitrogen dynamics and climate change effects at global and regional scales (Treseder, 2008; Meiser et al., 2014; Liu et al., 2020;Porre et al., 2020). Similar research was also conducted to quantify the effects of various LUC on the global SOC, soil carbon pools and soil properties through meta-analysis (Guo and Gifford, 2002; Deng et al., 2016) but no such investigations to understand the impact of conversion of FL to other LUs were done in an Indian context.
The main aim of this study is to obtain a quantitative assessment of responses in soil properties under Indian climatic and edaphic conditions including soil carbon stocks, microbial biomass, MQ, and CO2 equivalent emissions, due to conversion of FL to other LUs. This was done through a meta-analysis approach using datasets obtained from published studies carried out in different regions of India. The general hypothesis was that conversion of FL into LUs with varying degrees of disturbance and plant diversity would cause a general decline in SOC stocks along with a decline in soil microbial capacity. Specific goals were to (1) determine the effect of LUC on general soil properties; (2) estimate the effect of LUC on SOC, soil carbon pools, and SOC stocks; (3) determine the relationship between SOC and bulk density (BD) under various LUs; and (4) analyze the variations in MQ and CO2 eq emission in various LUs in the Indian agroecological context.
2 Materials and Methods
2.1 Data Sources and Collections
Data on soil physical, chemical, and biological properties, including soil carbon pools, were obtained from studies that evaluated LUC effects in established experiments covering India’s five physiographic regions: Northern Mountains, Great Plains, Peninsular India, Peninsular Plateau, and Coastal Plains and Islands. Figure 2 shows the major soil taxonomic groups of India and the major study sites (31 locations) from which experimental results were obtained from published literature for the meta-analysis. Data were obtained from published research/review articles and theses using unique keywords related to the study’s objectives. The data were compiled following different categories of LUC, e.g., from FL to BL; from FL to CL; from FL to GL; from FL to HL; and from FL to PL. Data didn’t require any specific criteria other than have two minimum LUs including FL. To understand the effect of LUC on soil properties and soil carbon pools, a thorough analysis was conducted on different LU systems in the Indian context (details in Section 2.2) and only studies with a minimum of two LUs and with appropriate sets of soil physical, chemical, and biological parameters were considered as part of the selected studies irrespective of years of study as the magnitude of LUC change would depend on the duration that the LU was implemented (details in Section 2.2). In all studies with comparable LUs in different agroecological regions, FL use systems are native in nature and other LUs are converted from native forest due to human disturbances. Major soil types covered in this study included alluvial soil, black soil, red soil, laterite soil, and arid soil. Over the other LUs (BL, CL, GL, HL, and PL), FL was used as a control treatment. This study also covers the Himalayan zone, the Indo-Gangetic plains, the north-eastern region, and the peninsular region, which represent different subtropical climate regions of India. Specific soil types, taxonomy, management methods, crops and cropping systems, as well as specific trees and grasses were not included in this report.
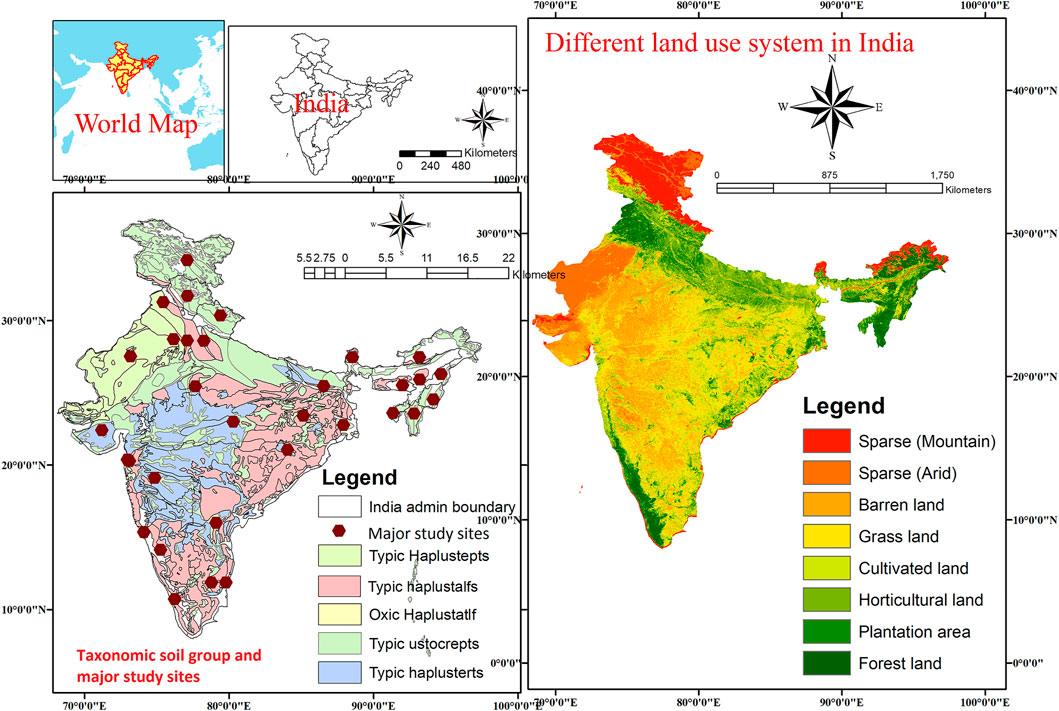
FIGURE 2. Different land use systems and taxonomic soil group in India showing location map of major study sites.
2.2 LU Selection and Soil Parameters Considered for the Study
Figure 2 shows the major LU systems present in the different agroecological regions of India. The description of different LUs selected for the assessment of changes in soil carbon in the Indian soils is given in Table 1. Details of the soil parameter data used in this study and the soil characteristics of various LUs are given in Table 2 and Table 3.
2.3 Data Compilation
Various published literatures (original articles, review papers, and theses) were collected from the period of 1990–2019 and reviewed critically in context to the impact of LUC on soil carbon pools and soil properties in different regions of India with an aim of finding the changes in these soil parameters due to conversion of FL to other LUs. Following a general analysis, data from a replicated studies on different LUs were used, with FL data serving as a control to better understand the impact of LUC on BL, CL, GL, HL, and PL in India. To understand the impact of LUC in various soil depths (0–0.15 m, 0.15–0.30 m, and 0.30–0.45 m), 1,786 paired datasets from 31 major study sites (reflected in Figure 2) with multiple LU comparisons including the FL system were analyzed for meta-analysis using MetaWin 2.1 software.
2.4 Meta-Analysis: Method of Analysis Using Diverse Datasets
Two stage-based random effect meta-analyses were used to analyze the database and understand the comparative changes (Rosenberg et al., 2000; Chakraborty et al., 2017; Sharma et al., 2019). Under this, the effect size (ES) was calculated for individual parameter as the natural log of the response ratio (InR) using the equation as proposed by Hedges et al. (1999):
Where XT is the average of response variables (SOC, SOC stocks, soil carbon pools, and other soil parameters) of the treatments (LUs), and XC is the average of these variables in FL with control.
In the second stage, combined effect estimate was determined as a weighted mean of the effects estimated in the individual studies. A weighted mean is calculated as
Where NT and NC represent the number of replications for each of the treatments (LUs), in an individual study. If more than one observation was included in a treatment, the weighted are divided by the number of observations from that study. Since the studies were from different soil and environmental conditions and with varying multiple replications, the standard deviation calculated was based on the number of observations with a simple statistical procedure in MS excel. ES from individual studies were then combined using a mixed-effect model to calculate the cumulative effect size and the 95% confidence intervals (CIs) through boot-strapping with 4,999 iterations (Adams et al., 1997). The mixed-effect model is a random-effect meta-analytic model for categorical data (Rosenberg et al., 2000), assuming random variation among studies within a group and fixed variation between groups. The cumulative effect was considered significant if the CIs did not pass over zero. Results were interpreted by back-transformation and presented as change in percentage caused by treatments in relation to control. The comparisons tested for their significance were between FL and different LUs and the meta-analyzed values presented in graphs with statistical significance (for p values < 0.05) marked with an asterisk.
2.5 Linear Model for Correlation Among SOC and BD in Different LUs
Data for SOC and BD within different LUs were log transformed for normalization and analyzed for potential relationships using a general linear regression model (Nelder and Wedderburn, 1972), and the regression equations plus R2 values are shown in the Figure 8.
3 Results
3.1 Impacts on Soil pH
LUC showed positive effects on soil pH for LUs like BL, CL, GL, HL, and PL when compared to FL (Figure 3A). For example, soil pH increased significantly for BL (5.0%), CL (6.0%), and HL (5.0%) but found non-significant changes for GL (1.1%) and PL (4.1%) over the FL, which is considered as control for this study. Soil pH showed positive effects for other LUs over the FL for depth-wise data (Figure 3A). In the 0–15 cm soil depth, pH increased significantly in CL (6.4%) and HL (5.6%) over the FL, but no significant changes were found for BL, GL, and PL. In the 15–30 cm soil depth, pH increased significantly in CL (6.3%) and HL (4.3%) over the FL but was non-significant for BL, GL, and PL, whereas in soil depth 30–45 cm, pH increased significantly in BL (8.1%), CL (3.7%), and HL (5.2%) over the FL and was non-significant for GL and PL (Figure 3A). The depth-wise results of pH were in concurrence to the findings of overall pH except for BL and similarly indicated that conversion of FL towards other LUs could result into increase in soil pH (Figure 3A).
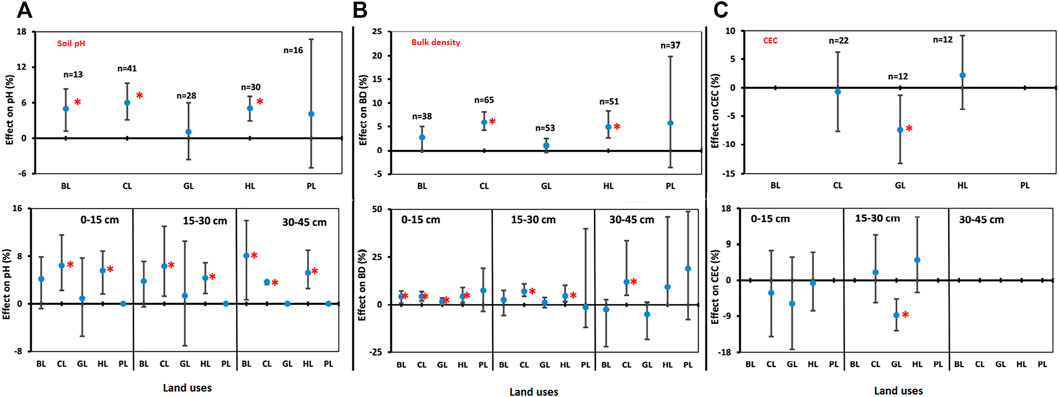
FIGURE 3. Comparisons of soil properties. (A) Soil reaction (pH). (B) Bulk density (BD) and (C) cation exchange capacity (CEC) under various land uses (BL, CL, GL, HL, and PL) with FL based on soil depths (0–15 cm, 15–30 cm, and 30–45 cm). The error bars show 95% confidence intervals (CI), and the difference is significant if it does not pass zero. *indicates significant difference at p-value is less than 0.05. Here, Forest land (FL) is used as control, BL—Barren land, CL—Cultivated land, GL—Grassland, HL—Horticulture land, and PL—Plantation land.
3.2 Impacts on Bulk Density and Cation Exchange Capacity
BD was found to be significantly and positively affected in LUs CL and HL over the FL. The percent increase of BD in BL, CL, GL, HL, and PL was 2.7%, 5.9%, 1.0%, 4.9%, and 5.8%, respectively, when compared with FL (Figure 3B); however, the increase was lower in the GL system. BD improved with soil depths, particularly at 0–15 cm; a significant increase of 4.2% (BL), 4.1% (CL), 1.6% (GL), and 4.2% (HL) was observed over the FL. CL and HL showed a consistent increase in BD with increased depth, 6.8% and 4.5% (15–30 cm) and 11.8% and 9.1% (30–45 cm), respectively, which was significant over FL (Figure 3B). Others showed a non-significant change. Conversion of FL to GL reduced CEC significantly in particular in the 15–30 cm depth. For example, the percent decrease for GL in CEC was 8.8% over the FL (Figure 3C).
3.3 Impacts on Soil Organic Carbon
Negative effects of LUC on SOC were found for LUs like BL, CL, GL, HL, and PL when compared to FL (Figure 4A). The SOC decreased significantly for BL (−27.3%), CL (−31.1%), GL (−36.1%), and PL (−35.5%) over the FL considered as control for this study, but changes were non-significant for HL (−11.5%). SOC decreased with soil depth in LUs when compared to FL (Figure 4A). In the soil depth 0–15 cm, SOC decreased significantly in BL (−25.5%) and GL (−27.5%) over the FL but changed non-significantly for CL (−21.0%), HL (−17.5%), and PL (−31.1%) in comparison to FL. In soil depth 15–30 cm, SOC decreased significantly in BL (−29.6%), CL (−46.5%), GL (−41.3%), and PL (−40.5%) over the FL. The reduction was higher in soil depth 30–45 cm where SOC decreased significantly in BL (−27.7%), CL (−54.9%), GL (−63.7%), and PL (−36.4%) over the FL. In both soil depths, the observed changes for HL were −12.4% (15–30 cm) and 1.8% (30–45 cm), respectively, and were non-significant. The depth-wise results of SOC were in concurrence to the findings of overall SOC and similarly indicated that conversion of FL towards other LUs would cause a decline in SOC content.
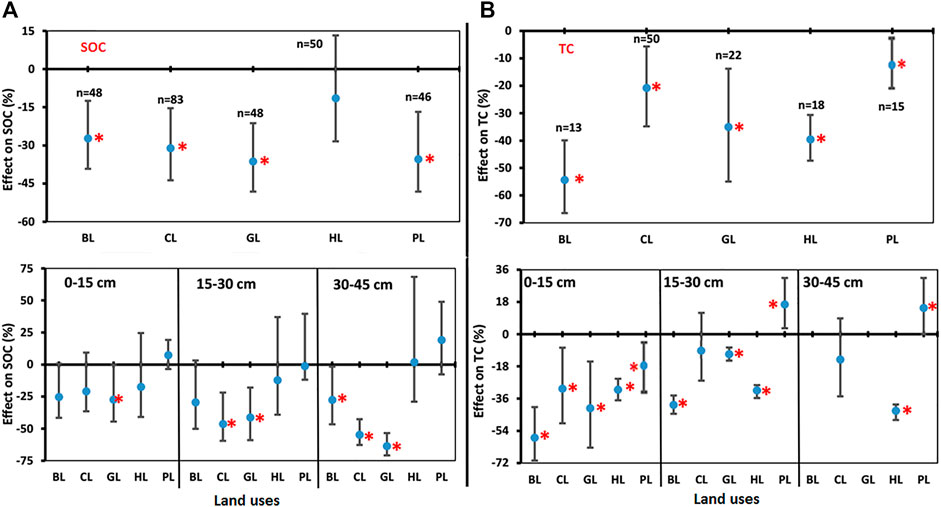
FIGURE 4. Comparisons of soil properties. (A) Soil organic carbon (SOC), and (B) total carbon (TC) under various land uses (BL, CL, GL, HL, and PL) with FL based on soil depths (0–15 cm, 15–30 cm, and 30–45 cm). The error bars show 95% confidence intervals (CI) and the difference is significant if it does not pass zero. *indicates significant difference at p-value is less than 0.05. Here, Forest land (FL) is used as control, BL—Barren land, CL—Cultivated land, GL—Grassland, HL—Horticulture land, and PL—Plantation land.
3.4 Impacts on Total Carbon
Negative effects of LUC on total carbon (TC) were found for LUs like BL, CL, GL, HL, and PL when compared to FL (Figure 4B). TC decreased significantly for BL (−54.3%), CL (−20.8%), GL (−35.0%), HL (−39.6%), and PL (−8.7%) over the FL. Total C decreased with soil depth for other LUs over the FL (Figure 4B). In soil depth 0–15 cm, TC decreased significantly in BL (−57.8%), CL (−30.5%), GL (−41.4%), HL (−31.2%), and PL (−20.2%) over the FL. In soil depth 15–30 cm, TC decreased significantly in BL (−39.5%) and GL (−31.6%) over the FL and increased significantly in PL (16.6%). However, TC for CL was not significantly different as compared to FL. In soil depth 30–45 cm, TC decreased significantly in HL (−40.0%) over the FL and increased significantly in PL (14.7%) over the FL (Figure 4B).
3.5 Impacts on Labile Carbon and Non-Labile Carbon
Labile carbon (LC) decreased significantly for BL (−34.7%), CL (−24.9%), GL (−35.5%), HL (−33.5%), and PL (−48.9%) over the FL (Figure 5A). These results indicated that conversion of FL towards other LUs could readily result into decline in LC content under most conditions. Labile C decreased significantly in the soil depth 0–15 cm in BL (−29.6%), CL (−9.7%), GL (−28.4%), HL (−31.0%), and PL (−46.8%) over the FL. Also, in soil depth 15–30 cm, LC decreased significantly in BL (−37.6%), CL (−45.6%), GL (−55.6%), HL (−31.4%), and PL (−50.0%) over the FL. In soil depth 30–45 cm, LC decreased significantly in BL (−45.9%), CL (−53.8%), GL (−64.6%), HL (−42.8%), and PL (−56.0%) over the FL (Figure 5A).
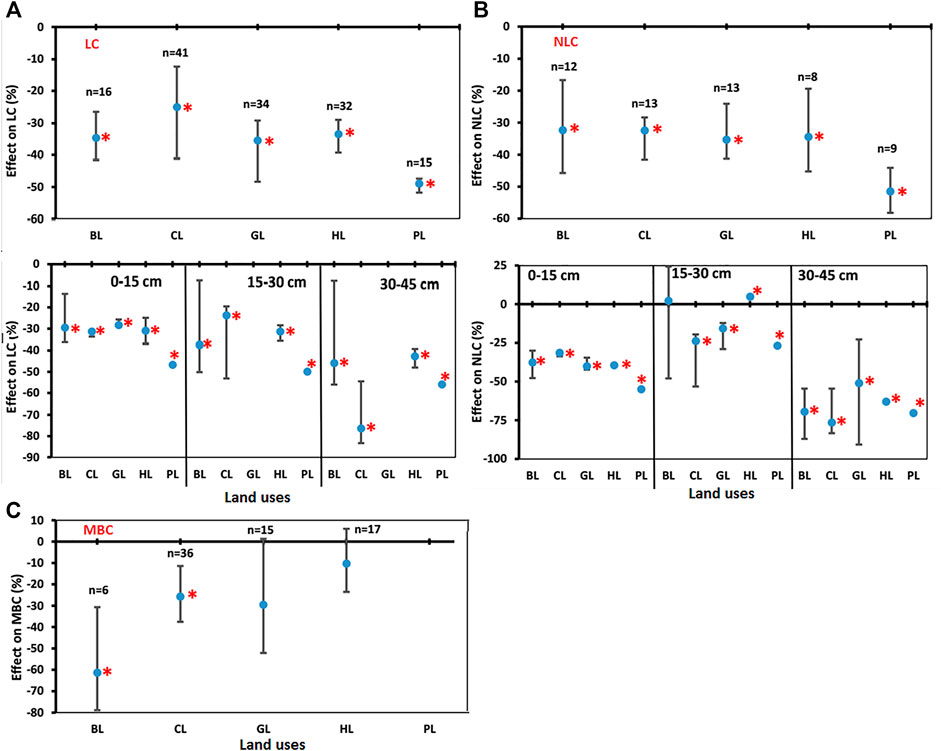
FIGURE 5. Comparisons of soil carbon pools (SCP). (A) Labile carbon (LC), (B) Non-Labile carbon (NLC), and (C) Microbial Biomass Carbon (MBC) under various land uses (BL, CL, GL, HL, and PL) with FL based on soil depths (0–15 cm, 15–30 cm, and 30–45 cm). The error bars show 95% confidence intervals (CI) and the difference is significant if it does not pass zero. *indicates significant difference at p-value is less than 0.05. Here, Forest land (FL) is used as control, BL—Barren land, CL—Cultivated land, GL—Grassland, HL—Horticulture land, and PL—Plantation land.
Non-labile carbon (NLC) decreased significantly for BL (−32.3%), CL (−32.4%), GL (−35.3%), HL (−34.5%), and PL (−51.5%) over the FL (Figure 5B). These results indicated that conversion of FL into other LUs could result into decline in NLC content under most conditions in all soil depths. In soil depth 0–15 cm, NLC decreased significantly in BL (−37.6%), CL (−31.4%), GL (−40.0%), HL (−39.44%), and PL (−54.9%) over the FL. In soil depth 15–30 cm, NLC decreased significantly in CL (−23.8%), GL (−15.6%), and PL (−26.8%) over the FL and increased significantly in HL (4.9%) as compared to FL. The BL was non-significantly changed in this depth. In soil depth 30–45 cm, NLC decreased significantly in BL (−69.5%), CL (−76.4%), GL (−51.0%), HL (−63.0%), and PL (−70.4%) over the FL (Figure 5B). In general, the percent decrease in 30–45 cm soil depth was greater than that in 15–30 cm followed by 0–15 cm (Figure 5B).
3.6 Impacts on Microbial Biomass Carbon (MBC)
Negative effects of LUC on soil MBC were found for LUs like BL, CL, GL, and HL (Figure 5C). For example, MBC levels decreased significantly for BL (−61.3%) and CL (−25.7%) over the FL but changes in GL (−29.5%) and HL (−10.3%) were non-significant. These results indicate that the conversion of FL to other LUs (BL/CL/GL/HL) could result in the decline of MBC content in soils (Supplementary Figure S2).
3.7 Changes in Soil Carbon Stocks (SOC Stocks) by Land-Use Change
LUC impacts on the SOC stocks were seen in all the regions of the country. For example, conversion of FL into LUs such as BL, CL, HL, and PL significantly reduced SOC stocks, whereas no significant change was observed under GL (Figure 6). The percent reduction of SOC stocks in BL, CL, GL, HL, and PL was 34.0%, 41.2%, 1.5%, 33.5%, and 47.9%, respectively, as compared with FL (Figure 6). There was a general trend of reduction in SOC stocks in all LUs. In soil depth 0–15 cm, SOC stocks decreased significantly in BL (−31.9%), CL (−38.3%), HL (−38.0%), and PL (−30.2%) over the FL but no significant change was observed for GL (5.7%) (Figure 6). Similarly, in soil depth 15–30 cm, SOC stocks decreased significantly in BL (−41.4%), CL (−44.6%), HL (−31.2%), and PL (−67.1%) over the FL, but the small change observed for GL (−17.3%) was not significant. Unlike the two top soil depths, in the soil depth 30–45 cm, SOC in GL decreased significantly (−14.2%), whereas the change in HL (−10.3%) was non-significant. There was a significant change in SOC in BL (−35.9%), CL (−47.6%), and PL (−67.2%) over the FL (Figure 6).
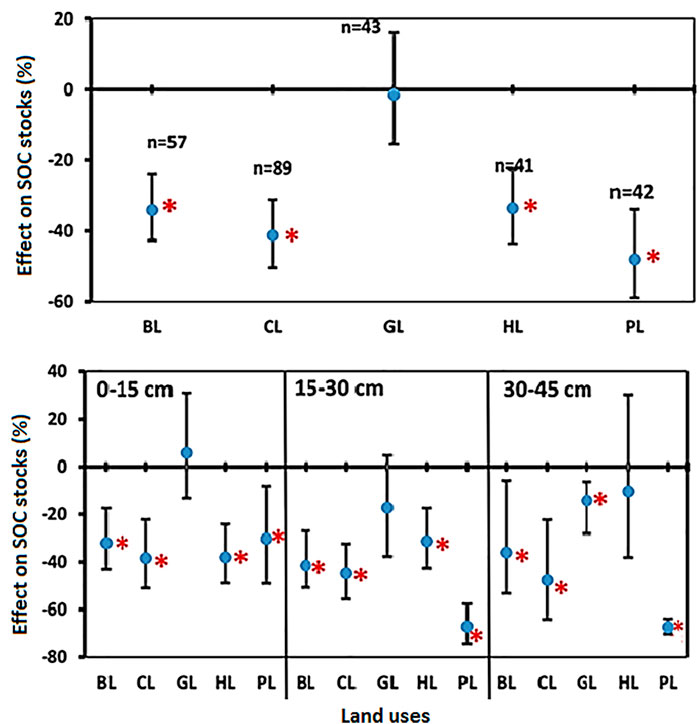
FIGURE 6. Comparisons of soil carbon stocks (SOC stocks) under various land uses (BL, CL, GL, HL, and PL) with FL based on soil depths (0–15 cm, 15–30 cm, and 30–45 cm). The error bars show 95% confidence intervals (CI) and the difference is significant if it does not pass zero. *indicates significant difference at p-value is less than 0.05. Here, Forest land (FL) is used as control, BL—Barren land, CL—Cultivated land, GL—Grassland, HL—Horticulture land, and PL—Plantation land.
3.8 Effect of LUC on Microbial Quotient (MQ) and CO2 Equivalent Emission
Results for MQ and CO2 equivalent emission showed significant differences in all LUs compared to FL systems (Figure 7; Supplementary Figure S2). MQ values in BL were lowest (0.91 ± 0.28) compared with those observed in other LUs (ranging from 3.31 ± 0.45 to 4.19 ± 0.49), whereas CO2 equivalent emissions were lower in GL and HL (23 ± 11 and 19 ± 8 Mg ha−1, respectively) and highest in CL and PL systems (66 ± 12 Mg ha−1) compared to FL (Figure 7).
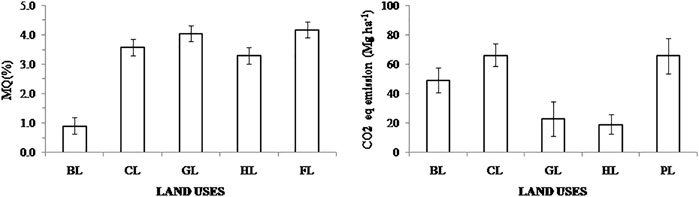
FIGURE 7. Comparison of microbial quotient (MQ) and CO2 eq. emissions in various land uses from the collected studies (mean ± standard error). Here, Forest land (FL) is used as control, BL—Barren land, CL—Cultivated land, GL—Grassland, HL—Horticulture land, and PL—Plantation land. Note: PL has no sufficient data for MQ analysis.
3.9 Correlation of SOC With BD in Land Uses
Bulk density (BD) was found to be significant and negatively correlated with SOC in all the LUs at p < 0.05 (Figure 8). The maximum correlation was observed in FL (R2 = 0.48**).
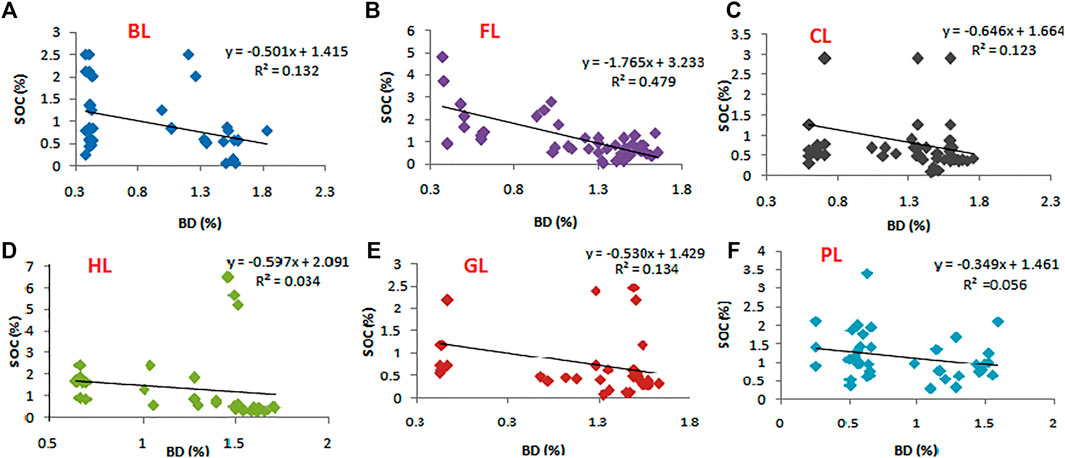
FIGURE 8. Linear regression with the variables of soil organic carbon (SOC) and bulk density (BD) in contrast with six different land uses, with significant difference in R2 value at p < 0.05. Here, FL, Forest land; BL, Barren land; CL, Cultivated land; GL, Grassland; HL, Horticulture land; PL, Plantation land.
4 Discussion
4.1 Changes in Soil Properties Following Land-Use Change
Deforestation and LUC from FL to different LU production systems with varying anthropogenic activities has been suggested to increase CO2 and other GHG emissions contributing to climate change (Lal, 2004; Wang et al., 2021). Through meta-analysis, this study attempted to determine the impact of LUC on soil properties, particularly SOC and microbial biomass, in the Indian context using datasets representing different agroecological regions. Appropriate number of datasets was used for conducting meta-analysis of soil parameters: soil pH (n = 155), SOC (n = 333), BD (n = 303), and TC (n = 163). A positive change in soil pH due to changes of LU as observed in our study resonates the earlier work done (Rabbi et al., 2016; Malik et al., 2018). The higher positive impact on soil pH in CL followed by HL, BL, PL, and GL could be due to the management practices including application of fertilizers and other soil amendments, irrigation practices, etc. It has been reported that LU intensification in lower pH soils positively affects the pH and leads to SOC loss through increased rate of decomposition from improved microbial growth and activity (Malik et al., 2018). Sharma et al. (2014) recorded higher soil pH in CL than the FL in Indian Himalaya’s foot hill. LUC have an impact on soil properties such as BD. A positive change in BD due to changes of LU was recorded in our study similar to previous reports (Vidya et al., 2002; Meena et al., 2018). Lower BD in FL than other LUs could be partly attributed to the higher organic matter (OM) content in the soil, better aggregation resulting in an increase in the volume of micropores, and overall better soil structure (Materechera and Mkhabela, 1995). The highest BD was found in soil depths of 30–45 cm, while the lowest was found in surface soil (0–15 cm), indicating that BD increased with soil depth due to the effects of the overlying soil’s weight (Vidya et al., 2002; Meena et al., 2018).
A better understanding of the dynamics and responses in SOC content is vital for detecting and forecasting changes in response to global climate change (Negi and Gupta, 2010). The evidence from the meta-analysis of experimental findings from different agroecological regions indicates a general trend of decline in SOC from the conversion of FL into different LUs. Similar findings for the reduction in SOC when native forest systems were converted into managed agroecosystems have been reported from other regions of the world (Mayer et al., 2020). The heterogeneity associated with various studies considered in this meta-analysis, in terms of environmental, edaphic, and specific management practices, contributed to the differences in the magnitude of effect seen for different LUs. Similar results were reported in the central Himalayan region of Uttarakhand, India (Kalambukattu et al., 2013).
Large annual additions of OM in the form of leaf litter, which are potentially highest in the FL, coupled with lack of tillage/disturbance activities and a slow rate of decomposition would have contributed to higher soil carbon values (Haynes, 2005; Baker et al., 2007; Kalambukattu et al., 2013). Whereas the lower SOC in other LUs compared to FL could be attributed to the tillage and other disturbance activities, removal of crop residues through burning, grazing, irrigation, etc. affects higher OM decomposition and nutrient mineralization (Batjes, 1999; Ogle et al., 2014). Decomposition of OM releases CO2 into the environment, resulting in a decrease in SOC (Ramzan et al., 2019). In the case of CL systems, crop residue removal, intensive cultivation practices, and increased microbial carbon turnover can increase carbon losses from the soil system (Lal and Kimble, 1997; Yang et al., 2004; Baker et al., 2007; Smith et al., 2008; Sharma et al., 2014). The loss of SOC through increased CO2 levels into the environment is suggested to contribute significantly to the global warming and climate change (Lal 2004; Sanderman et al., 2017). Therefore, there is a widespread belief that it is important to store SOC in the terrestrial environment through increased SOC sequestration and reduced losses in order to manage climate change and associated effects on overall ecosystem health. Any attempts to reduce SOC losses through changes in the management in different LUs would help sequester more carbon in terrestrial ecosystems. The study shows that there is scope for improvement of SOC in different LUs (BL/CL/GL/PL/HL) to become carbon equivalent to that in FL systems, i.e., a potential to increase SOC by 27.3% in BL, 31.1% in CL, 36.1% in GL, 35.5% in PL, and 11.5% in HL. This change could be possible through proper management strategies that promote C sequestration in soil. As with our findings, several studies have found a strong and negative association between SOC and BD over the LUC (Hati et al., 2007; Wang et al., 2011; Padbhushan et al., 2016a; Padbhushan et al., 2016b; Meena et al., 2018).
TC content is one of the key indicators of soil quality that has been linked to the long-term addition of organic residues to the soil (Lemke et al., 2010; Yang et al., 2012; Padbhushan et al., 2016b), and it plays an important role in improving other physico-chemical properties of soils. Total C includes all carbon pools, i.e., inorganic carbon, charcoal carbon, and organic carbon (which includes labile carbon pools). While the changes in TC reflect the overall changes in SOC stocks, it may not give true reflection of changes in SOC components that may differentially respond or change due to management in different LUs. Measuring changes in TC content due to LUC has been shown to take longer periods, i.e., decades, compared to the changes that can be seen in the more labile components of SOC (Lal, 2004; Sanderman et al., 2017). Over the FL, the TC trend changed from BL < HL < GL < CL < PL (higher negative value to lower negative value). In terms of soil depths, the 0–15 cm soil depth showed the greatest difference in negative value, indicating that changes in TC were greater in the surface soil than the other soil depths due to more litter addition on the undisturbed soil.
It is now well accepted that changes in TC may not be a sensitive indicator for short-term responses on SOC stocks, and carbon sequestration and measurement of SOM composition or soil carbon pools have been suggested as better indicators of changes in soil quality due to LUC (Gregorich et al., 1994; Leifeld and Kögel-Knabner, 2005).
4.2 Changes in Soil Carbon Pools and Microbial Quotient (MQ) Following Land-Use Change
Soil carbon pools such as LC, NLC, and MBC were shown to give more useful information about carbon cycling and loss through CO2 emissions and more sensitive soil-quality parameters for carbon dynamics under different management practices (Yang et al., 2012). PL showed greater loss both for LC and NLC than other LUs used in our study over the FL. This could be due to post burn cultivation in the PL system. Similar results were corroborated by Sahoo et al. (2019) in Mizoram, Northeast India who reported lower LC and NLC in PL than other LUs compared to FL. The negative effects of LUC from FL extended to all the SOC pools including LC pool. Thus, FL systems showed the highest values for SOC pools compared to all the LUs considered in this study. High soil carbon pools (for all the three soil quality parameters) in FL could be attributed to undisturbed litter additions for long periods. In contrast, disturbances caused by cultivation and low additions of OM are reasons for low LC in CL. Consequences are nutrient loss and impacting macroaggregate formation, which result in lowering in soil fertility and quality, including higher BD and soil compaction. In general, LC was more in surface soil than the subsurface soil potentially due to higher amounts of added root biomass and exudation coupled with surface crop residues (Padre et al., 2007; Brar et al., 2013; Padbhushan et al., 2015).
MBC represents the living component of SOC and is considered to reflect LC levels in soil systems (Gonzalez-Quiñones et al., 2011; Mendoza et al., 2020). Soils from FL systems showed the highest amount of MBC levels mostly from the larger amounts of aboveground residues and root biomass (Holden and Treseder, 2013; Kumar et al., 2021). MBC can be a sensitive indicator of SOC changes as it has a much faster rate of turnover; hence, trends in MBC have been suggested to predict longer-term trends in SOC (Gupta et al., 1994; Lal, 2004; Haynes 2005; Padbhushan et al., 2020). The lack of carbon inputs from plants would be the primary reason for the lowest MBC in the BL systems. It is well known that the practice of fallowing in cropping lands can cause a significant reduction in MBC and microbial activities (Sarkar et al., 2020). Similarly, the lower value of MBC in CL is attributed to the intensive tillage practice promoting microbial turnover of biologically available soil OM and crop residues and the loss of carbon as CO2 emissions (Gougoulias et al., 2014; Tiefenbacher et al., 2021). There are several individual and meta-analysis studies that reported significant reductions in MBC from conventional tillage practices, in particular when compared to no-till practices (Roper et al., 2010; Zuber and Villamil, 2016).
MQ is one of the important derived measures to indicate changes in MBC, potential for microbial carbon turnover, and the general soil quality in different LU systems (Anderson and Domsch, 1993). Unlike the absolute values of microbial biomass, MQ being a ratio avoids the problems from comparing values across soils and systems with different total SOM levels. Results in this study indicate that MQ showed greater effect in BL followed by PL, CL, and GL. The lowest values for MQ coupled with lower SOC in BL suggest that changing FL systems to BL not only reduced the overall SOC stocks but also affected microbial carbon turnover, with an overall decline in soil quality. The general trend of higher MQ values in LUs such as FL and GL suggests the presence of perennial vegetation and/or lower disturbance supporting higher biological activity and associated ecosystem functions. It is suggested that a greater decrease in MQ values, in particular LUs, indicates that soil is being used in an exploitative manner and microbial pools are declining faster than changes in the total SOC (Sparling, 1997).
4.3 Changes in SOC Stocks Following Land-Use Change
LUC-associated fluctuations in SOC stocks have been reported in many agroecological regions from different parts of the world (Chatterjee et al., 2018; Pellikka et al., 2018). Also, the conversion of LUs from FL to other LUs leading in lower SOC stocks can result in a decrease in soil quality (De Blécourt et al., 2013; Guillaume et al., 2015; Fan et al., 2016; Iqbal and Tiwari, 2016). Changes in LU from FL to other LUs can serve as a carbon source while also affecting soil characteristics (Abera and Wolde-Meskel, 2013). According to a report, about 350 mha of FL has been transferred to other LUs (ITTO, 2002), resulting in biodiversity loss (Ahrends et al., 2015) and 20–40% SOC storage losses (De Blécourt et al., 2013; Guillaume et al., 2015). According to a meta-analysis, when native FL was transformed to PL and CL, SOC stocks decreased by 13%–42% (Guo and Gifford, 2002). Similarly, in this study, LUs had a negative effect on SOC stocks. The absence of deep-rooted trees and fewer canopy covers in this study resulted in lower SOC stocks for PL and HL as compared to FL. Also, SOC content is an indicator of the influence of nature of crops and its management practice. These PL use types undergo regular intercultural operations, which thereby reduces the input of carbon to the soil (Sahoo et al., 2019). The equilibrium between the rate of deposition of photosynthetic materials and the rate of respiration by decomposer microorganisms influences the ability for soils to sequester carbon from the atmosphere (Mathieu et al., 2015). In addition, since root tissue is more resistant to decomposition and mineralization than top soil litters, root-derived carbon has a longer residence period (Rasse et al., 2005).
This meta-analysis study shows that the scope of improvement of SOC stocks in other LUs (BL/CL/GL/PL/HL) to become carbon equivalent to FL can be possible by increasing SOC stocks by 33.5%–41.2% in BL, CL, and HL systems. The level of increase in SOC stocks required was lowest in GL (1.47%) systems and highest in PL. The general trend for the required increase of SOC stocks with depth was similar to the total SOC. However, this change being more in lower depths as compared to surface soil is due to the differences in SOC stocks brought out by LUCs in the subsurface soil over the surface soil. As restoring the lost SOC stocks under different LUs is a difficult job, it is worthwhile to make it possible through management practices. A large amount of atmospheric CO2 can be restored into the soil, which may help mitigate the problems of climate change. Integration of organic inputs with chemical fertilizer in cultivated soil can be one of the better LU management strategies for restoring carbon in the soil and improving the crop productivity and thus managing soil health and ensuring food security (Padbhushan et al., 2020).
4.4 Effects of SOC on Soil Health and Food Security
Greenhouse gases (GHGs) are the main players to maintain the Earth’s habitable temperature. A small change in their amount in the atmosphere can affect the climatic conditions on Earth. Anthropogenic emissions of CO2 are likely to increase with increase in human population (Lelieveld et al., 2019). Human led LUC can result in significant exchanges of CO2 between the soil and air (Lal, 2004). Carbon dioxide equivalent (CO2 eq) emissions in our study were found to be affected in all the LUs over the FL, suggesting loss in SOC stocks. CL and PL had more SOC stocks lost and added more CO2 in the atmosphere (Sahoo et al., 2019). Therefore, restoration of carbon in the soil can be one of the options to counteract the effect of climate change and the problems created due to climate change. Since OM plays a multifaceted role in several soil processes (Gregorich et al., 1994), SOC is one of the essential components for sustaining soil health and food security by the maintenance of the production system (Anantha et al., 2018).
The findings in this study show that changes in LU have an effect on not only SOC but also other soil resources. Some studies have found links between SOC and total nitrogen and other parameters, implying that OM turnover has an effect on these variables (Xu et al., 2019). The importance of soil management and carbon storage is becoming more widely recognized. However, due to continued LUC to meet the ever-growing food production needs, maintaining or improving low levels of SOC stocks is a major challenge. This issue can be mitigated by employing proper crop production management strategies that include systems with lower disturbance/tillage practices, retention of crop residues, application of organic manures, and inclusion of perennial crops as part of an integrated system approach.
5 Conclusion
Our study found that LUC had a positive effect on soil pH and BD, while SOC, TC, and soil carbon pools were negatively affected, in comparison with FL systems. The conversion of FL to other LUs resulted in losses of overall SOC stocks and the trends were similar in all the soil carbon pools such as LC, NLC, and MBC. LUC, in general, affected soil carbon pools and soil properties in surface as well as subsurface layers. SOC stocks declined by a minimum of 2% in GL, 42% in CL, and 48% in PL. There was a negative association between SOC and BD in several LUs. Similarly, when compared to FL, MQ and CO2 eq emissions were negatively impacted in all LUs (BL/CL/GL/HL/PL). Overall, in view of the evidence for the potential impact of LUC on SOC stocks, C turnover, and soil quality, there is an urgent need for sustainable management of current production systems and natural resources that reduce CO2 emissions and increase soil carbon in LU systems in India.
Data Availability Statement
The original contributions presented in the study are included in the article/Supplementary Material, further inquiries can be directed to the corresponding authors.
Author Contributions
SS framed the notion and was overall in charge of this manuscript preparation. RP, UK, MK, and PK collected literature, analyzed data, and drafted the manuscript. DR helped in meta-analysis. RK did preparation of map. KA, VG, AK, BP, and AS edited the manuscript. All contributors discussed the outcomes and added to the final document. All authors have studied and approved the in print version of the paper.
Conflict of Interest
The authors declare that the research was conducted in the absence of any commercial or financial relationships that could be construed as a potential conflict of interest.
Publisher’s Note
All claims expressed in this article are solely those of the authors and do not necessarily represent those of their affiliated organizations, or those of the publisher, the editors, and the reviewers. Any product that may be evaluated in this article, or claim that may be made by its manufacturer, is not guaranteed or endorsed by the publisher.
Acknowledgments
We are grateful to all of the researchers whose contributions are listed in this paper for their assistance in the preparation of this manuscript. We are also grateful to the International Rice Research Institute (IRRI) for providing the necessary funds and facilities. Participation by VG was supported through ACIAR (WAC/2018/164) and Australian Water Partnership (660118.66) project funded by the Australian Government.
Supplementary Material
The Supplementary Material for this article can be found online at: https://www.frontiersin.org/articles/10.3389/fenvs.2021.794866/full#supplementary-material
Supplementary Figure S1 | Trend of share of forest land (FL) in Y1-axis and cultivated land (CL) in Y2-axis area of total land (1990–2017) of India, FAO (http://faostat.fao.org/).
Supplementary Figure S2 | Relationship between microbial biomass carbon (MBC, mg carbon/kg soil) and soil organic carbon (SOC, g carbon/kg soil) from the studies used in the study. Dotted lines indicate microbial quotient (MQ) of 2.5% and 5%.
References
Abera, G., and Wolde-Meskel, E. (2013). Soil Properties, and Soil Organic Carbon Stocks of Tropical Andosol under Different Land Uses. Open J. Soil Sci. 03, 153–162. doi:10.4236/ojss.2013.33018
Achard, F., Eva, H. D., Mayaux, P., Stibig, H.-J., and Belward, A. (2004). Improved Estimates of Net Carbon Emissions from Land Cover Change in the Tropics for the 1990s. Glob. Biogeochem. Cycles 18, GB2008. doi:10.1029/2003gb002142
Adams, D. C., Gurevitch, J., and Rosenberg, M. S. (1997). Resampling Tests for Meta-Analysis of Ecological Data. Ecology 78 (4), 1277–1283. doi:10.1890/0012-9658(1997)078[1277:rtfmao]2.0.co;2
Ahrends, A., Hollingsworth, P. M., Ziegler, A. D., Fox, J. M., Chen, H., and Su, Y. (2015). Current Trends of Rubber Plantation Expansion May Threaten Biodiversity and Livelihoods. Glob. Environ. Change 34, 48–58. doi:10.1016/j.gloenvcha.2015.06.002
Anantha, K. C., Majumder, S. P., Padhan, D., BadoleDatta, S. A., Datta, A., Mandal, B., et al. (2018). Carbon Dynamics, Potential and Cost of Carbon Sequestration in Double rice Cropping System in Semi Arid Southern India. J. Soil Sci. Plant Nutr. 18, 1–15. doi:10.4067/s0718-95162018005001302
Anderson, T-H., and Domsch, K. H. (2010). Soil Microbial Biomass: The Eco-Physiological Approach. Soil Biol. Biochem. 42 (12), 2039–2043. doi:10.1016/j.soilbio.2010.06.026
Anderson, T., and Domsch, K. (1993). The Metabolic Quotient for CO2 (qCO2) as a Specific Activity Parameter to Assess the Effects of Environmental Conditions, Such as Ph, on the Microbial Biomass of forest Soils. Soil Biol. Biochem. 25, 393–395. doi:10.1016/0038-0717(93)90140-7
Arora, V. K., and Boer, G. J. (2010). Uncertainties in the 20th Century Carbon Budget Associated with Land Use Change. Glob. Chang. Biol. 16, 3327–3348. doi:10.1111/j.1365-2486.2010.02202.x
Baker, J. M., Ochsner, T. E., Venterea, R. T., and Griffis, T. J. (2007). Tillage and Soil Carbon Sequestration-What Do We Really Know? Agric. Ecosyst. Environ. 118 (1-4), 1–5. doi:10.1016/j.agee.2006.05.014
Bastida, F., Zsolnay, A., Hernández, T., and García, C. (2008). Past, Present and Future of Soil Quality Indices: a Biological Perspective. Geoderma 147, 159–171. doi:10.1016/j.geoderma.2008.08.007
Batjes, N. H. (2012). Management options for reducing CO2-concentrations in the atmosphere by increasing carbon sequestration in the soil. Report 410-200-031, Dutch National Research Programme on Global air pollution and Climate Change & Technical Paper 30. Wageningen: International Soil Reference and information Centre.
Bhattacharyya, T., Pal, D. K., Mandal, C., and Velayutham, M. (2000). Organic Carbon Stocks in Indian Soils and Their Geographical Distribution. Curr. Sci. 79 (5), 655–660. http://www.jstor.org/stable/24105084.
Bodh, P. C. (2019). Agricultural Situation in India. Available at: https://eands.dacnet.nic.in/PDF/February 2019.pdf (Accessed June 15, 2020).
Brar, B. S., Singh, K., Dheri, G. S., and Balwinder-Kumar, B. (2013). Carbon Sequestration and Soil Carbon Pools in a rice?wheat Cropping System: Effect of Long-Term Use of Inorganic Fertilizers and Organic Manure. Soil Tillage Res. 128, 30–36. doi:10.1016/j.still.2012.10.001
Brookes, P. C. (1995). The Use of Microbial Parameters in Monitoring Soil Pollution by Heavy Metals. Biol. Fertil. Soils 19, 269–279. doi:10.1007/bf00336094
Campbell, C. A., Janzen, H. H., and Juma, N. G. (1997). “Chapter 17 Case Studies of Soil Quality in the Canadian Prairies: Long-Term Field Experiments,” in Soil Quality for Crop Production. Editors E. G. Gregorich, and M. R. Carter (Amsterdam: Elsevier), 351–397. doi:10.1016/s0166-2481(97)80044-x
Chakraborty, D., Ladha, J. K., Rana, D. S., Jat, M. L., Gathala, M. K., Yadav, S., et al. (2017). A Global Analysis of Alternative Tillage and Crop Establishment Practices for Economically and Environmentally Efficient rice Production. Sci. Rep. 7, 9342. doi:10.1038/s41598-017-09742-9
Chatterjee, N., Nair, P. K. R., Chakraborty, S., and Nair, V. D. (2018). Changes in Soil Carbon Stocks across the Forest-Agroforest-Agriculture/Pasture Continuum in Various Agroecological Regions: A Meta-Analysis. Agric. Ecosyst. Environ. 266, 55–67. doi:10.1016/j.agee.2018.07.014
de Blécourt, M., Brumme, R., Xu, J., Corre, M. D., and Veldkamp, E. (2013). Soil Carbon Stocks Decrease Following Conversion of Secondary Forests to Rubber (Hevea Brasiliensis) Plantations. PLoS One 8, e69357. doi:10.1371/journal.pone.0069357
DeFries, R. S., Houghton, R. A., Hansen, M. C., Field, C. B., Skole, D., and Townshend, J. (2002). Carbon Emissions from Tropical Deforestation and Regrowth Based on Satellite Observations for the 1980s and 1990s. Proc. Natl. Acad. Sci. 99, 14256–14261. doi:10.1073/pnas.182560099
Deng, L., Zhu, G.-Y., Tang, Z.-S., and Shangguan, Z.-P. (2016). Global Patterns of the Effects of Land-Use Changes on Soil Carbon Stocks. Glob. Ecol. Conservation 5, 127–138. doi:10.1016/j.gecco.2015.12.004
Don, A., Schumacherw, J., and Freibauer, A. (2011). Impact of Tropical Land-Use Change on Soil Organic Carbon Stocks – a Meta-Analysis. Glob. Change Biol. 17, 1658–1670. doi:10.1111/j.1365-2486.2010.02336.x
Fan, S., Guan, F., Xu, X., Forrester, D., Ma, W., and Tang, X. (2016). Ecosystem Carbon Stock Loss after Land Use Change in Subtropical Forests in China. Forests 7, 142. doi:10.3390/f7070142
FAO (2006). Available at: http://faostat.fao.org/ (Accessed September 24, 2020).
Gonzalez-Quiñones, V., Stockdale, E. A., Banning, N. C., Hoyle, F. C., Sawada, Y., Wherrett, A. D., et al. (2011). Soil Microbial Biomass-Interpretation and Consideration for Soil Monitoring. Soil Res. 49 (4), 287. doi:10.1071/sr10203
Gougoulias, C., Clark, J. M., and Shaw, L. J. (2014). The Role of Soil Microbes in the Global Carbon Cycle: Tracking the Below-Ground Microbial Processing of Plant-Derived Carbon for Manipulating Carbon Dynamics in Agricultural Systems. J. Sci. Food Agric. 94 (12), 2362–2371. doi:10.1002/jsfa.6577
Gregorich, E. G., Carter, M. R., Angers, D. A., Monreal, C. M., and Ellert, B. H. (1994). Towards a Minimum Data Set to Assess Soil Organic Matter Quality in Agricultural Soils. Can. J. Soil Sci. 74, 367–385. doi:10.4141/cjss94-051
Guillaume, T., Damris, M., and Kuzyakov, Y. (2015). Losses of Soil Carbon by Converting Tropical forest to Plantations: Erosion and Decomposition Estimated by δ 13 C. Glob. Change Biol. 21, 3548–3560. doi:10.1111/gcb.12907
Guo, L. B., and Gifford, R. M. (2002). Soil Carbon Stocks and Land Use Change: A Meta Analysis. Glob. Chang Biol. 8, 345–360. doi:10.1046/j.1354-1013.2002.00486.x
Gupta, V., Roper, M., Kirkegaard, J., and Angus, J. (1994). Changes in Microbial Biomass and Organic Matter Levels during the First Year of Modified Tillage and Stubble Management Practices on a Red Earth. Soil Res. 32, 1339–1354. doi:10.1071/sr9941339
Hati, K. M., Swarup, A., Dwivedi, A. K., Misra, A. K., and Bandyopadhyay, K. K. (2007). Changes in Soil Physical Properties and Organic Carbon Status at the Topsoil Horizon of a Vertisol of central India after 28 Years of Continuous Cropping, Fertilization and Manuring. Agric. Ecosyst. Environ. 119, 127–134. doi:10.1016/j.agee.2006.06.017
Haynes, R. J. (2005). Labile Organic Matter Fractions as central Components of the Quality of Agricultural Soils: An Overview. Adv.Agron. 85, 221–268. doi:10.1016/s0065-2113(04)85005-3
Hedges, L. V., Gurevitch, J., and Curtis, P. S. (1999). The Meta-Analysis of Response Ratios in Experimental Ecology. Ecology 80, 1150–1156. doi:10.1890/0012-9658(1999)080[1150:tmaorr]2.0.co;2
Holden, S. R., and Treseder, K. K. (2013). A Meta-Analysis of Soil Microbial Biomass Responses to forest Disturbances. Front. Microbiol. 4, 1–17. doi:10.3389/fmicb.2013.00163
Houghton, R. A. (2008). “Carbon Flux to the Atmosphere from Land-Use Changes: 1850– 2005,” in TRENDS: A Compendium of Data on Global Change, Carbon Dioxide Information Analysis Center (Oak Ridge, TN, U.S.A: Oak Ridge National Laboratory, U.S. Department of Energy). Available at: https://cdiac.ess-dive.lbl.gov/trends/landuse/houghton/houghton.html (Accessed May 15, 2020).
Houghton, R. A., and Hackler, J. L. (2003). Sources and Sinks of Carbon from Land-Use Change in China. Glob. Biogeochem. Cycles 17 (2), 1034. doi:10.1029/2002gb001970
Hurtt, G. C., Frolking, S., Fearon, M. G., Moore, B., Shevliakova, E., Malyshev, S., et al. (2006). The Underpinnings of Land-Use History: Three Centuries of Global Gridded Land-Use Transitions, wood-harvest Activity, and Resulting Secondary Lands. Glob. Chang. Biol. 12 (7), 1208–1229. doi:10.1111/j.1365-2486.2006.01150.x
International Energy Agency (2019). Available at: https://www.iea.org/reports/global-energy-co2-status-report-2019 (Accessed May 23, 2020).
Iqbal, S., and Tiwari, S. C. (2016). Soil Organic Carbon Pool under Different Land Uses in Achanakmar Amarkantak Biosphere Reserve of Chhattisgarh, India. Curr. Sci. 110, 771–773. http://www.jstor.org/stable/24907957
ITTO (2002). ITTO Guidelines for the Restoration, Management and Rehabilitation of Degraded and Secondary Tropical Forests. Policy Development Series 13. ITTO, Yokohama. Available at: https://www.cifor.org/library/1175/itto-guidelines-for-the restoration management-and rehabilitation-of-degraded-and-secondary-tropical-forests/ISBN: 4-902045-01-X (Accessed August 28, 2020).
Kalambukattu, J. G., Singh, R., Patra, A. K., and Arunkumar, K. (2013). Soil Carbon Pools and Carbon Management index under Different Land Use Systems in the Central Himalayan Region. Acta Agriculturae Scand. Section B - Soil Plant Sci. 63 (3), 200–205. doi:10.1080/09064710.2012.749940
Koricheva, J., Gurevitch, J., and Mengersen, K. (2013). Handbook of Meta-Analysis in Ecology and Evolution. Princeton: Princeton University Press.
Kumar, A., Padbhushan, R., Singh, Y. K., Kohli, A., and Ghosh, M. (2021). Soil Organic Carbon under Various Land Uses in Alfisols of Eastern India. Indian J. Agril. Sci. 91 (7), 975–979.
Kumar, U., Shahid, M., Tripathi, R., Mohanty, S., Kumar, A., Bhattacharyya, P., et al. (2017). Variation of Functional Diversity of Soil Microbial Community in Sub-Humid Tropical Rice-Rice Cropping System under Long-Term Organic and Inorganic Fertilization. Ecol. Indicators 73, 536–543. doi:10.1016/j.ecolind.2016.10.014
Lal, R., and Kimble, J. M. (1997). Conservation Tillage for Carbon Sequestration. Nutr. Cycling Agroecosyst. 49, 243–253. doi:10.1023/a:1009794514742
Lal, R. (2004). Soil Carbon Sequestration in India. Climatic Change 65, 277–296. doi:10.1023/b:clim.0000038202.46720.37
Leifeld, J., and Kögel-Knabner, I. (2005). Soil Organic Matter Fractions as Early Indicators for Carbon Stock Changes under Different Land-Use? Geoderma 124 (1), 143–155. doi:10.1016/j.geoderma.2004.04.009
Lelieveld, J., Klingmüller, K., Pozzer, A., Burnett, R. T., Haines, A., and Ramanathan, V. (2019). Effects of Fossil Fuel and Total Anthropogenic Emission Removal on Public Health and Climate. Proc. Natl. Acad. Sci. USA 116 (15), 7192–7197. doi:10.1073/pnas.1819989116
Lemke, R. L., VandenBygaart, A. J., Campbell, C. A., Lafond, G. P., and Grant, B. (2010). Crop Residue Removal and Fertilizer N: Effects on Soil Organic Carbon in a Long-Term Crop Rotation experiment on a Udic Boroll. Agric. Ecosyst. Environ. 135, 42–51. doi:10.1016/j.agee.2009.08.010
Liu, J., Tian, H. Q., Liu, M., Zhuang, D., Melillo, J. M., and Zhang, Z. (2005a). China's Changing Landscape during the 1990s: Large-Scale Land Transformations Estimated with Satellite Data. Geophys. Res. Lett. 32, L02405. doi:10.1029/2004gl021649
Liu, J., Liu, M., Tian, H., Zhuang, D., Zhang, Z., Zhang, W., et al. (2005b). Spatial and Temporal Patterns of China's Cropland during 1990-2000: An Analysis Based on Landsat TM Data. Remote Sensing Environ. 98, 442–456. doi:10.1016/j.rse.2005.08.012
Liu, M., and Tian, H. (2010). China's Land Cover and Land Use Change from 1700 to 2005: Estimations from High-Resolution Satellite Data and Historical Archives. Glob. Biogeochem. Cycles 24, GB3003. doi:10.1029/2009gb003687
Liu, W., Zhou, P., Chen, K., Ye, Z., Liu, F., Li, X., et al. (2020). Efficacy and Safety of Antiviral Treatment for COVID-19 from Evidence in Studies of SARSCoV-2 and Other Acute Viral Infections: A Systematic Review and Meta-Analysis. CMAJ. 192 (27), E734–E744. doi:10.1503/cmaj.200647
Malik, A. A., Puissant, J., Buckeridge, K. M., Goodall, T., Jehmlich, N., Chowdhury, S., et al. (2018). Land Use Driven Change in Soil pH Affects Microbial Carbon Cycling Processes. Nat. Commun. 9 (1), 3591. doi:10.1038/s41467-018-05980-1
Materechera, S. A., and Mkhabela, T. S. (1995). Microbial Biomass in Agricultural Top Soils after 6 Years of Bare Fallow. Biol. Fertil. Soils 19, 129–134.
Mathieu, J. A., Hatté, C., Balesdent, J., and Parent, É. (2015). Deep Soil Carbon Dynamics Are Driven More by Soil Type Than by Climate: A Worldwide Meta-Analysis of Radiocarbon Profiles. Glob. Change Biol. 21, 4278–4292. doi:10.1111/gcb.13012
Mayer, M., Prescott, C. E., Abaker, W. E. A., Augusto, L., Cécillon, L., Ferreira, G. W. D., et al. (2020). Tamm Review: Influence of forest Management Activities on Soil Organic Carbon Stocks: A Knowledge Synthesis. For. Ecol. Manage. 466, 118127. doi:10.1016/j.foreco.2020.118127
Meena, V. S., Mondal, T., Pandey, B. M., Mukherjee, A., Yadav, R. P., Choudhary, M., et al. (2018). Land Use Changes: Strategies to Improve Soil Carbon and Nitrogen Storage Pattern in the Mid-Himalaya Ecosystem, India. Geoderma 321, 69–78. doi:10.1016/j.geoderma.2018.02.002
Meetei, T. T., Kundu, M. C., and Devi, Y. B. (2020). Long-Term Effect of Rice-Based Cropping Systems on Pools of Soil Organic Carbon in Farmer's Field in Hilly Agroecosystem of Manipur, India. Environ. Monit. Assess. 192, 209. doi:10.1007/s10661-020-8165-x
Meiser, A., Bálint, M., and Schmitt, I. (2014). Meta-Analysis of Deep-Sequenced Fungal Communities Indicates Limited Taxon Sharing Between Studies and the Presence of Biogeographic Patterns. New Phytologist. 201 (2), 623–635. doi:10.1111/nph.12532
Mendoza, B., Béjar, J., Luna, D., Osorio, M., Jimenez, M., and Melendez, J. R. (2020). Differences in the Ratio of Soil Microbial Biomass Carbon (MBC) and Soil Organic Carbon (SOC) at Various Altitudes of Hyperalic Alisol in the Amazon Region of Ecuador. F1000Res 9, 443. doi:10.12688/f1000research.22922.1
Nath, A. J., Brahma, B., Sileshi, G. W., and Das, A. K. (2018). Impact of Land Use Changes on the Storage of Soil Organic Carbon in Active and Recalcitrant Pools in a Humid Tropical Region of India. Sci. Total Environ. 624, 908–917. doi:10.1016/j.scitotenv.2017.12.199
Negi, S. S., and Gupta, M. K. (2010). Soil Organic Carbon Store under Different Land Use Systems in Giri Catchment of Himachal Pradesh. Indian Forester 136 (9), 1147.
Nelder, J. A., and Wedderburn, R. W. M. (1972). Generalized Linear Models. J. R. Stat. Soc. Ser. A (General) 135 (3), 370–384. doi:10.2307/2344614
Ogle, S. M., Adler, P. R., Breidt, F. J., Del Grosso, S., Derner, J., Franzluebbers, A., et al. (2014). “Chapter 3: Quantifying Greenhouse Gas Sources and Sinks in Cropland and Grazing Land Systems,” in Quantifying Greenhouse Gas Fluxes in Agriculture and Forestry: Methods for Entity‐Scale Inventory. Editors M. Eve, D. Pape, M. Flugge, R. Steele, D. Man, M. Riley‐Gilbertet al. (Washington, DC: Technical Bulletin Number 1939. Office of the Chief Economist, U.S. Department of Agriculture), 606.
Padbhushan, R., Das, A., Rakshit, R., Sharma, R. P., Kohli, A., and Kumar, R. (2016b). Long-Term Organic Amendment Application Improves Influence on Soil Aggregation, Aggregate Associated Carbon and Carbon Pools under Scented Rice-Potato-Onion Cropping System after the 9th Crop Cycle. Commun. Soil Sci. Plant Anal. 47 (21), 2445–2457. doi:10.1080/00103624.2016.1254785
Padbhushan, R., Rakshit, R., Das, A., and Sharma, R. P. (2015). Assessment of Long-Term Organic Amendments Effect on Some Sensitive Indicators of Carbon under Subtropical Climatic Condition. The Bioscan 10 (3), 1237–1240.
Padbhushan, R., Rakshit, R., Das, A., and Sharma, R. P. (2016a). Effects of Various Organic Amendments on Organic Carbon Pools and Water Stable Aggregates under a Scented rice-potato-onion Cropping System. Paddy Water Environ. 14 (4), 481–489. doi:10.1007/s10333-015-0517-8
Padbhushan, R., Sharma, S., Kumar, U., Rana, D. S., Kohli, A., Parmar, B., et al. (2021). Meta-analysis Approach to Measure Effect of Integrated Nutrient Management on Crop Performance, Microbial Activity and Carbon Stocks in Indian Soils. Front. Environ. Sci. 9, 724702. doi:10.3389/fenvs.2021.724702
Padbhushan, R., Sharma, S., Rana, D. S., Kumar, U., Kohli, A., and Kumar, R. (2020). Delineate Soil Characteristics and Carbon Pools in Grassland Compared to Native Forestland of India: A Meta-Analysis. Agronomy 10, 1969. doi:10.3390/agronomy10121969
Palni, L. M. S., Maikuri, R. K., and Rao, K. S. (1998). “Conservation of the Himalayan Agroecosystem: Issues and Priorities,” in Technical Paper-V in Eco Regional Cooperation for Biodiversity Conservation in the Himalaya (New York: United Nation Development Programme), 253–290.
Paul, K. I., Polglase, P. J., Nyakuengama, J. G., and Khanna, P. K. (2002). Change in Soil Carbon Following Afforestation. For. Ecol. Manage. 168, 241–257. doi:10.1016/s0378-1127(01)00740-x
Pellikka, P. K. E., Heikinheimo, V., Hietanen, J., Schäfer, E., Siljander, M., and Heiskanen, J. (2018). Impact of Land Cover Change on Aboveground Carbon Stocks in Afromontane Landscape in Kenya. Appl. Geogr. 94, 178–189. doi:10.1016/j.apgeog.2018.03.017
Porre, R. J., van der Werf, W., De, D., Gerlinde, B., Stomph, T. J., and Hoffland, E. (2020). Is Litter Decomposition Enhanced in Species Mixtures? A Meta-Analysis. Soil Biol. Biochem. 145, 107791. doi:10.1016/j.soilbio.2020.107791
Rabbi, S. M. F., Tighe, M., Delgado-Baquerizo, M., Cowie, A., Robertson, F., Dalal, R., et al. (2015). Climate and Soil Properties Limit the Positive Effects of Land Use Reversion on Carbon Storage in Eastern Australia. Sci. Rep. 5 (1), 17866. doi:10.1038/srep17866
Rakshit, R., Das, A., Padbhushan, R., Sharma, R. P., Sushant, S., and Kumar, S. (2018). Assessment of Soil Quality and Identification of Parameters Influencing System Yield under Long-Term Fertilizer Trial. Jour. Indian Socie. Soil Scie. 66, 166–171. doi:10.5958/0974-0228.2018.00021.x
Ramzan, S., ZahoorAhmad, I. P., Ashraf, I., Wani, M. A., and Rasool, R. (2019). Effect of Agricultural Land-Use on Carbon Sequestration in Soils. Biol. Forum Inter. J. 11 (1), 117.
Rasse, D. P., Rumpel, C., and Dignac, M. F. (2005). Is Soil Carbon Mostly Root Carbon? Mechanisms for a Specific Stabilization. Plant Soil 269, 341–356. doi:10.1007/s11104-004-0907-y
Ritchie, H., and Roser, M. (2019). CO2 and Greenhouse Gases Emissions. Published online at Our World In Data.org. Available at: [Online Resource] https://ourworldindata.org/co2-and-other-greenhouse-gas-emissions.
Roper, M. M., Gupta, V. V. S. R., and Murphy, D. V. (2010). Tillage Practices Altered Labile Soil Organic Carbon and Microbial Function without Affecting Crop Yields. Soil Res. 48, 274–285. doi:10.1071/sr09143
Rosenberg, M. S., Adams, D. C., and Gurevitch, J. (2000). MetaWin Statistical Software for Meta-Analysis Version 2.0. Sunderland, MA: Sinauer Associates.
Sahoo, U. K., Singh, S. L., Gogoi, A., Kenye, A., and Sahoo, S. S. (2019). Active and Passive Soil Organic Carbon Pools as Affected by Different Land Use Types in Mizoram, Northeast India. PLoS One 14 (7), e0219969. doi:10.1371/journal.pone.0219969
Sanderman, J., Hengl, T., and Fiske, G. J. (2017). Soil Carbon Debt of 12,000 Years of Human Land Use. Proc. Natl. Acad. Sci. USA 114, 9575–9580. doi:10.1073/pnas.1706103114
Sarkar, S., Skalicky, M., Hossain, A., Brestic, M., Saha, S., Garai, S., et al. (2020). Management of Crop Residues for Improving Input Use Efficiency and Agricultural Sustainability. Sustainability 12 (23), 9808. doi:10.3390/su12239808
Sharma, S., Padbhushan, R., and Kumar, U. (2019). Integrated Nutrient Management in Rice-Wheat Cropping System: An Evidence on Sustainability in the Indian Subcontinent through Meta-Analysis. Agronomy 9, 71. doi:10.3390/agronomy9020071
Sharma, V., Hussain, S., Sharma, K. R., and Arya, V. M. (2014). Labile Carbon Pools and Soil Organic Carbon Stocks in the Foothill Himalayas under Different Land Use Systems. Geoderma 232-234, 81–87. doi:10.1016/j.geoderma.2014.04.039
Smith, P., Martino, D., Cai, Z., Gwary, D., Janzen, H., Kumar, P., et al. (2008). Greenhouse Gas Mitigation in Agriculture. Phil. Trans. R. Soc. B 363, 789–813. doi:10.1098/rstb.2007.2184
Sparling, G. P. (1997). “Soil Microbial Biomass, Activity and Nutrient Cycling as Indicators of Soil Health,” in Biological Indicators of Soil Health. Editors C. E. Pankhurst, B. M. Doube, and V. V. S. R. Gupta (Wallingford, UK: CAB International), 97–119.
Tian, H., Banger, K., Bo, T., and DadhwalDadhwal, V. K. B. (2014). History of Land Use in India during 1880-2010: Large-Scale Land Transformations Reconstructed from Satellite Data and Historical Archives. Glob. Planet. Change 121, 78–88. doi:10.1016/j.gloplacha.2014.07.005
Tian, H. Q. (2003). Regional Carbon Dynamics in Monsoon Asia and its Implications for the Global Carbon Cycle. Glob. Planet. Chang. 37 (3–4), 201–217. doi:10.1016/s0921-8181(02)00205-9
Tiefenbacher, A., Sandén, T., Haslmayr, H.-P., Miloczki, J., Wenzel, W., and Spiegel, H. (2021). Optimizing Carbon Sequestration in Croplands: A Synthesis. Agronomy 11 (5), 882. doi:10.3390/agronomy11050882
Tirol-Padre, A., Ladha, J. K., Regmi, A. P., BhandariandNubushi, A. L. I. K., and Inubushi, K. (2007). Organic Amendments Affect Soil Parameters in Two Long-Term Rice-Wheat Experiments. Soil Sci. Soc. Am. J. 71, 442–452. doi:10.2136/sssaj2006.0141
Treseder, K. K. (2008). Nitrogen Additions and Microbial Biomass: A Meta-Analysis of Ecosystem Studies. Ecol. Lett. 11 (10), 1111–1120. doi:10.1111/j.1461-0248.2008.01230.x
United Nations Department of Economic and Social Affairs: Population Division (UNDESA) (2019). Available at: https://www.un.org/en/development/desa/population/index.asp (Accessed June 27, 2020).
Vidya, K. R., Hareesh, G. R., Rajanna, M. D., Sringeswara, A. N., Balakrishna, G., Balakrishna, A. N., et al. (2002). Changes in Microbial Biomass C, N and P as Influenced by Different Land-Uses. Myforest 38, 323–328.
Wang, F., Harindintwali, J. D., Yuan, Z., Wang, M., Wang, F., Li, S., et al. (2021). Technologies and Perspectives for Achieving Carbon Neutrality. The Innovation 2, 100180. doi:10.1016/j.xinn.2021.100180
Wang, S., Liu, J., Zhang, C., Yi, C., and Wu, W. (2011). Effects of Afforestation on Soil Carbon Turnover in China's Subtropical Region. J. Geogr. Sci. 21, 118–134. doi:10.1007/s11442-011-0833-x
Wei, X., Shao, M., Gale, W., and Li, L. (2014). Global Pattern of Soil Carbon Losses Due to the Conversion of Forests to Agricultural Land. Sci. Rep. 4, 4062. doi:10.1038/srep04062
World Population Prospects: The 2019 Revision United Nations Population Division (2020). Last update in UN data: 2019/06/17. Available at: http://data.un.org/Data.aspx?q=india&d=PopDiv&f=variableID%3a12%3bcrID%3a356.
Xu, S., Liu, X., Li, X., and Tian, C. (2019). Soil Organic Carbon Changes Following Wetland Cultivation: a Global Meta-Analysis. Geoderma 347, 49–58. doi:10.1016/j.geoderma.2019.03.036
Yang, X., Ren, W., Sun, B., and Zhang, S. (2012). Effects of Contrasting Soil Management Regimes on Total and Labile Soil Organic Carbon Fractions in a Loess Soil in China. Geoderma 177-178, 49–56. doi:10.1016/j.geoderma.2012.01.033
Yang, Z., Singh, B. R., and Sitaula, B. K. (2004). Fractions of Organic Carbon in Soils under Different Crop Rotations, Cover Crops and Fertilization Practices. Nutrient Cycling in Agroecosystems 70, 161–166. doi:10.1023/b:fres.0000048479.30593.ea
Keywords: land-use change, soil carbon pools, microbial quotient, meta-analysis, India
Citation: Padbhushan R, Kumar U, Sharma S, Rana DS, Kumar R, Kohli A, Kumari P, Parmar B, Kaviraj M, Sinha AK, Annapurna K and Gupta VVSR (2022) Impact of Land-Use Changes on Soil Properties and Carbon Pools in India: A Meta-analysis. Front. Environ. Sci. 9:794866. doi: 10.3389/fenvs.2021.794866
Received: 14 October 2021; Accepted: 20 December 2021;
Published: 07 March 2022.
Edited by:
Dima Chen, China Three Gorges University, ChinaReviewed by:
Zhang Juan, Northeast Agricultural University, ChinaBing Wang, China Three Gorges University, China
Copyright © 2022 Padbhushan, Kumar, Sharma, Rana, Kumar, Kohli, Kumari, Parmar, Kaviraj, Sinha, Annapurna and Gupta. This is an open-access article distributed under the terms of the Creative Commons Attribution License (CC BY). The use, distribution or reproduction in other forums is permitted, provided the original author(s) and the copyright owner(s) are credited and that the original publication in this journal is cited, in accordance with accepted academic practice. No use, distribution or reproduction is permitted which does not comply with these terms.
*Correspondence: Sheetal Sharma, sheetal.sharma@irri.org; Rajeev Padbhushan, rajpd01@gmail.com; Upendra Kumar, ukumarmb@gmail.com