Distribution and bioaccumulation of trace metals in urban semi-arid mangrove ecosystems
- 1Institut de Sciences Exactes et Appliquées (ISEA EA7484), Université de la Nouvelle-Calédonie, Nouméa, New Caledonia
- 2Institut des Sciences de la Terre de Paris, UMR 7193, Sorbonne Université, CNRS, Paris, France
- 3Aquaculture Biotechnology Research Group, School of Science, Faculty of Health and Environmental Sciences, Auckland University of Technology, Auckland, New Zealand
Mangrove ecosystems are known to act as filters for contaminants between land and sea. In New Caledonia, urbanization has increased along the coastline during the last decades. However, the impact of urbanization on contaminant cycling in mangrove forests has remained unexplored. In this study, we investigated trace metals (TM) dynamics in an urban mangrove soil and their transfer to mangrove tissues for the two dominant mangrove species in New Caledonia: Avicennia marina and Rhizophora stylosa. The results suggest that decades of urban rainwater runoff from an upper neighborhood induced large variations of mangrove soil physico-chemical properties compared to a control mangrove site sharing the same geological watershed. The urban mangrove site had a neutral pH and low salinity in the upper soil, while the control mangrove site presented acidic pH and a salinity ranging from 24 to 62 g L−1. Most TM were significantly less concentrated in the urban mangrove soil varying from 1.3 ± 0.3 μg g−1 at the urban site and 1.9 ± 0.5 μg g−1 at the control site for Cd, to 30 ± 8 mg g−1 and 49 ± 11 mg g−1 for Fe at the urban and control site, respectively. However, higher root bioconcentration factors were measured for As, Cd, Co, Cr, Fe, Mn, Ni, and Pb in the urban mangrove soil (1.7 ± 0.9, 0.14 ± 0.06, 0.23 ± 0.13, 0.042 ± 0.026, 0.088 ± 0.057, 0.47 ± 0.39, 0.21 ± 0.12, and 0.25 ± 0.09, respectively) compared to the control mangrove soil (0.11 ± 0.03, 0.041 ± 0.016, 0.045 ± 0.021, 0.010 ± 0.004, 0.013 ± 0.007, 0.094 ± 0.030, 0.022 ± 0.011, and 0.12 ± 0.03, respectively). The bioavailability of TM in the urban mangrove soil may be favored by suboxic conditions associated to less Cl-TM complexes and pyrite-TM complexes in the soil. Only Cu, Pb, Ti, and Zn, usually associated with urbanization, were more concentrated in the urban mangrove soil with mean concentrations of 27 ± 4, 17 ± 2, 4,571 ± 492, and 62 ± 12 μg g−1 at the urban site, respectively, and 21 ± 4, 10 ± 3, 2,834 ± 541, and 57 ± 12 μg g−1 at the control site, respectively. No significant difference in translocation factors was measured between the two sites, evidencing a regulation of TM translocation to the upper tissues by mangrove trees.
1 Introduction
Mangrove forests are tidal ecosystems developing in intertropical areas (Thomas et al., 2017). These ecosystems provide many ecosystem services, such as the function of buffers for sediments and contaminants between diverse watersheds and the adjacent aquatic ecosystems (e.g., lagoon, estuaries, sea) (Lee et al., 2014). Mangrove forests cover littoral areas of more than 100 countries. Out of the 20 countries with the most mangrove surface areas, 17 are referred to as developing countries, according to the International Monetary Fund (International Monetary Fund, 2022), and a third of their population lives on the coast (Center for International Earth Science Information Network, 2012). There is inevitably competition for space between mangrove ecosystems and economic and urban development.
Accordingly, mangrove forests are endangered ecosystems despite awareness of their benefits. Economic and urban development have resulted in high mangrove deforestation rates with about 35% of the mangrove surface area that was lost between the 80s and the 90s (Valiela et al., 2001; Alongi, 2002). In addition to direct mangrove forests destruction, urbanization can also impact mangrove forests through the input of anthropogenic effluents, and specifically rainwater runoff enriched with trace metals (TM). These effluents can contaminate and stress mangrove ecosystems, but also change hydrological and geochemical conditions, creating a disequilibrium in coastal areas (Cavalcante et al., 2009; Lewis et al., 2011; Kristensen et al., 2017; Alemu et al., 2021).
TM are one of the many types of pollutants in mangrove forests and mainly originate from anthropogenic activities. TM are naturally occurring elements in the Earth crust (<1,000 mg kg−1) and are therefore present in the environment (Turekian and Wedepohl, 1961; Thornton, 2012). Anthropogenic activities such as industrial, mine, agricultural, and housing development activities increase their concentrations in the environment (Bayen, 2012). TM are of great concern because even though most of them are micronutrients, which can be used by a range of organisms, they can have significant negative impacts beyond specific thresholds (e.g., toxicity). For instance, TM can inhibit developmental processes and decrease photosynthetic activity of plants (Bayen, 2012). In contrast to organic pollutants, TM cannot be biologically or chemically degraded and can therefore be transported over long distances or accumulate in the environment (Prasad et al., 2006), adsorbed on organic complexes and reactive mineral surfaces, or trapped within mineral structures (Brown and Parks, 2001).
Mangrove ecosystems act as reservoirs for TM due to their high sedimentation rate, high organic matter (OM) content, and high biogeochemical reactivity (Harbison, 1986). TM dynamics in mangrove soil are generally well documented and depend on many factors, with the main ones being bonding phases and physico-chemical parameters (Duan et al., 2020; Huang S et al., 2020; Jayachandran et al., 2018). For example, the modification of redox conditions can limit or favour the precipitation of pyrite (FeS2), which can trap TM, supporting TM immobility in the mangrove soil (Noël et al., 2015; Chakraborty et al., 2016). Also, the amount of OM in the soil and the quality of the OM can influence TM accumulation and bioavailability in mangrove soils. TM can form complexes with the OM, and the capacity of formation is dependent on the nature of the OM with a preference of TM for mature OM with high lignin content (Marchand et al., 2005; Kristensen et al., 2008; Thakur et al., 2016, 2014; Ge and Li, 2018; Duan et al., 2020). TM can also be exported to mangrove trees or outside the mangrove forest in the dissolved phase with litterfall or tidal flushing and tidal pumping (Silva et al., 1998; Ferreira et al., 2007; Holloway et al., 2018, 2016). In urban contexts, previous studies have measured moderate to highly TM contaminated mangrove soils due to urban inputs (Marx and McGowan, 2010; Singh et al., 2010; Bastakoti et al., 2019).
Mangrove plants are considered resistant to metallic stress, partly due to the production of antioxidants limiting reactive oxygen species (MacFarlane, 2002). However, some direct effects of TM on mangrove trees were reported, including growth inhibition, increased peroxidase activity, and death of vegetal species (MacFarlane and Burchett, 2002, 2001; Cheng et al., 2014; Naidoo et al., 2014). The transfer of TM from mangrove soils to mangrove trees depend on the bioavailability of the TM in the soil as plants can only assimilate TM in the soluble form (Tremel-Schaub and Feix, 2005). Soil factors such as redox potential, pH, and cationic exchange capacity drive TM bioavailability in mangrove soils, and therefore transfer to mangrove trees (Batty, 2000; Bourgeois et al., 2020; Fritioff et al., 2005; Huang X et al., 2020; Marchand et al., 2016; Robin et al., 2021). TM transfer to mangrove plants differs greatly between TM, tissues, and species since it depends on metabolic requirements (He et al., 2014; Marchand et al., 2016; Rezaei et al., 2021; Robin et al., 2021). Mangrove species have developed mechanisms to prevent metallic stress. Some species, such as Avicennia spp., have pneumatophores (aerial roots exhibiting negative geotropism), which allow for gas exchange between the atmosphere and the rhizosphere, notably O2 (Purnobasuki et al., 2017). The O2 can oxidize labile Fe(II) available in the rhizosphere to form an iron (III) plaque at the root surface (Taylor and Crowder, 1983). Studies have shown that this iron plaque can trap TM and therefore prevent their transfer to the plant tissues (Pi et al., 2011; Yamaguchi et al., 2014; Robin et al., 2021). Other species, such as Rhizophora spp., rely on the thickness of their roots and lignification processes to limit TM absorption within their tissues (Cheng et al., 2014, 2012).
In New Caledonia, mangrove ecosystems account for about 80% of the West coast of the main island and 15% of its East coast (Marchand et al., 2007). While the human population density is low (17 inhabitants per km2) (Insee, 2020), and the island is relatively pristine, anthropogenic activities still threaten these ecosystems to various degrees. Due to the lateritic nature of one-third of the soil on the island (e.g., rich in oxides and TM) (Tardy and Roquin, 1992), most studies were interested in the impact of mining activities, that increase erosional processes, and lateritic sediments inputs on mangrove forests (Marchand et al., 2016, 2012; Bourgeois et al., 2020; Robin et al., 2021). Few studies have also considered the impact of aquaculture and its discharge (Marchand et al., 2011). However, the impact of urbanization on TM inputs and distribution in mangrove forests remains unexplored and is of critical importance. The understanding of the impact of urbanization in a pristine island is necessary to manage future urban development and limit the threat of TM contamination. In addition, the Caledonian population rely on mangrove forests and their resources for personal and economic use. Studies worldwide have already shown the potential threat of urbanization as a source of TM contamination in mangrove forests (Ray et al., 2006; He et al., 2014; Celis-Hernandez et al., 2020).
Our main objectives were to assess TM dynamics in mangrove soils receiving urban rainwater runoff for more than 50 years, as well as their bioaccumulation in tissues of two main mangrove species, Avicennia marina and Rhizophora stylosa. To this end, we collected soil, porewater, roots, and leaves in an urban and a control mangrove forest. The control site had the same geological watershed as the urban site, but was not exposed to urban runoff. Our hypotheses are 1) that trace metals are in higher concentrations at the urban site due to a greater input of these contaminants within the mangrove forest with the urban rainwater runoff, 2) that their physico-chemical conditions also differ because of a more waterlogged soil at the urban site, and 3) that their bioaccumulation in mangrove tissues at the urban mangrove site will differ from natural mangrove forests such as the control mangrove site due to those differences in physico-chemical conditions. To evaluate these hypotheses, sequential extractions were performed on soil samples and bioconcentration factors (BCF) were determined for the roots and the leaves of the two mangrove species.
2 Material and methods
2.1 Study sites
New Caledonia is a French archipelago located between 20°S and 23°S in the South Pacific. Mangrove forests cover more than 35,000 ha of the archipelago (Marchand et al., 2007). The climate of the western coast of the main island is semi-arid with semi-diurnal tidal cycles (Douillet et al., 2001). In New Caledonia, mangrove species develop in monospecific stands along gradients depending on soil geochemical parameters, such as salinity and topography (Marchand et al., 2011; Deborde et al., 2015). More than 20 mangrove species are found in New Caledonia, but two are dominant. Rhizophora spp. represent 50% of mangrove area and grow along the sealine in soils with porewater salinity ranging from 5 to 40 g L−1. Avicennia marina represents more than 15% of the mangrove area and grows in areas of higher elevation with porewater salinity between 35 and 70 g L−1 (Marchand et al., 2016, 2007).
The archipelago is composed of 33 towns and is characterized by a very low human population density. 57% of the population lives in the four main cities, all located in the southwest coast of the main island (Insee, 2020). This population aggregation generates substantial urban activities. Dumbéa, the second most populated city, has increased its population by 33% in the past 10 years, leading to an outbreak of anthropogenic activities and urban development (Insee, 2020), while the littoral area of this city is almost completely covered by mangrove forests.
Two mangrove sites containing both dominant mangrove species were chosen as study sites on the littoral of Dumbéa to investigate the impact of urbanization (Figure 1). Both sites have the same geological watershed, characterized by a Cretaceous sedimentary formation (sandstone and limestone) that often host volcano-clastic fragments (Service de la Géologie de Nouvelle-Calédonie, 2016), and are only 2 km apart. The first mangrove site is the undisturbed “control” mangrove site located in the Apogoti Bay (22°12′08″S, 166°26′20″E). The site is adjacent to a recent housing lot, still under construction, without any direct anthropogenic inputs. The wastewater discharges and rainwater effluents are not released in this control mangrove site. The second mangrove site is the “urban” mangrove site (22°12′39″S, 166°27′19″E) and is characterized by an input of rainwater runoff streaming from the upper allotments for more than 50 years. The upper allotments have few petrol stations and are mainly residential with a population of about 10,000 inhabitants. This urban freshwater is therefore potentially charged in contaminants. At the urban site, the R. stylosa trees are about 8–10 m tall, which is much higher than those at the control site and other typical mangrove stands in New Caledonia, which are usually about 2 m tall. Also, in contrast to the control site, the species zonation at the urban site is unusual, with R. stylosa trees developing further away from the sealine where A. marina trees usually develops and where the runoff enters the mangrove ecosystem.
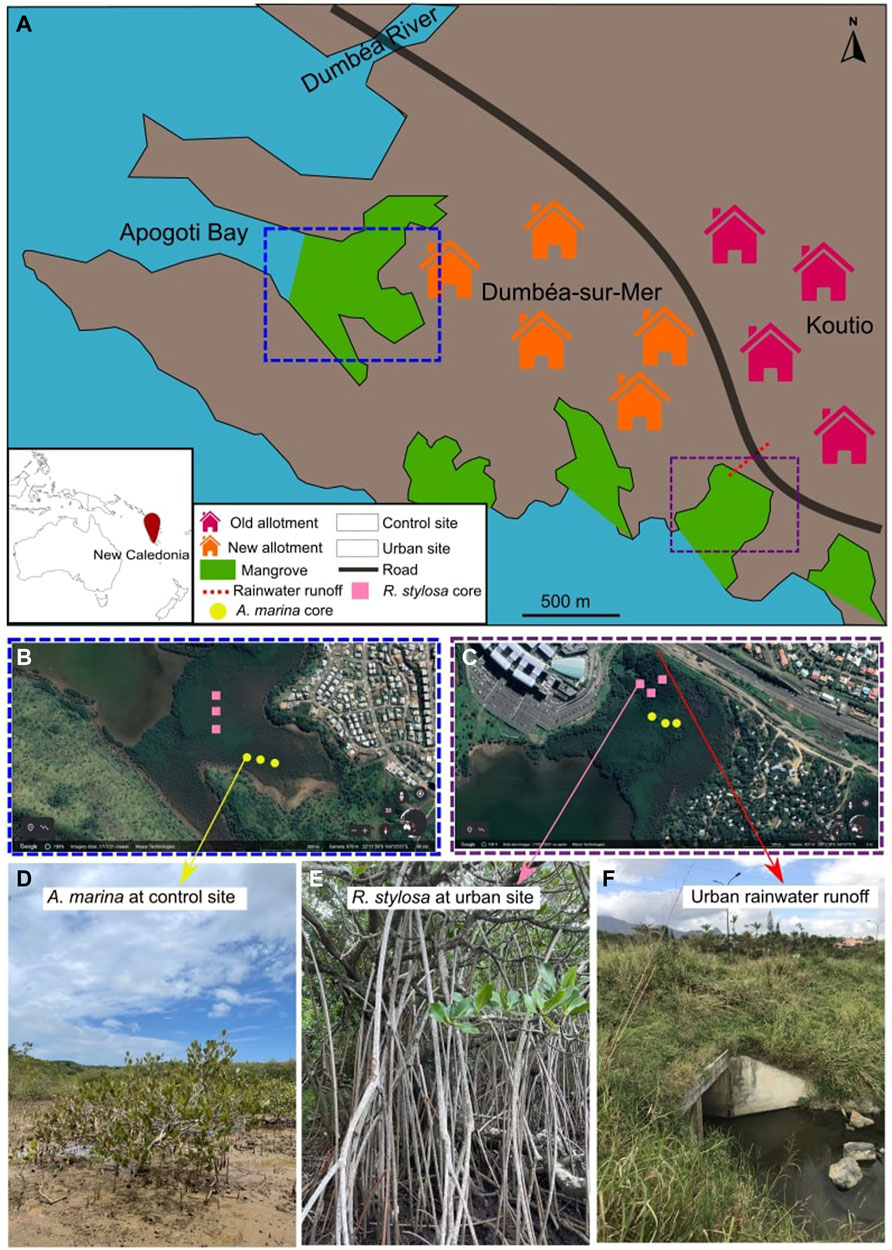
FIGURE 1. Map of the two studied mangrove sites (A) with triplicate soil cores taken under A. marina and R. stylosa at the control site (B) and urban site (C), and pictures of A. marina at the control site (D), R. stylosa at the urban site (E), and the entrance of the urban rainwater in the urban site (F).
2.2 Sampling and processing
2.2.1 Sampling
Samples were collected in May 2021, at the end of the rainy season, characterized by a La Niña phenomena. In both sites and at each mangrove stand (A. marina and R. stylosa), triplicate soil cores (40 cm long) were collected with an Eijkelkamp gouge auger about 30 m apart from one another. The 40 cm depth allowed for differential analysis with an above and below portion of the root system. Soil cores were cut along the depth with a wooden knife into six sections (0–5 cm, 5–10 cm, 10–15 cm, 15–20 cm, 20–30 cm, and 30–40 cm). The pH and redox potential were immediately measured using a pH meter (pH3110—WTW). A glass electrode (SENTIX–Xylem Analytics) was inserted in the middle of the soil section to measure the pH, calibrated with standards prior to measurements, while a combined Pt and Ag/AgCl electrode (SENTIX–Xylem Analytics) was inserted in the soil to measure the redox, calibrated with standards prior to measurements. Eh values correspond to the redox values obtained on site +202 mV to adjust to a H electrode. The soil sections were placed into tightly closed plastic bags. To extract porewater from soil samples, rhizon samplers (Rhizon SMS—10 cm, diameter 2.5 mm–Rhizosphere) were inserted into the soil sections. Each rhizon was connected to a 20 ml syringe. The plastic bags with the soil and the attached syringes were immediately placed into a cooler (∼4°C) until processed at the laboratory, less than 6 h after collection. Within the same area where the soil cores were collected, coarse roots of R. stylosa were extracted with a stainless-steel saw. For A. marina, pneumatophores were gently teared from the main roots. Leaf samples were also collected from both tree species. One leaf sample corresponds to 20 leaves collected from five different trees. All biotic samples were immediately placed into plastic bags and kept in a cooler (∼4°C) until processed at the laboratory, less than 6 h after sampling.
2.2.2 Processing
Upon arrival at the laboratory, salinity was measured in the porewater samples using a refractometer (ATC). Porewater samples were filtered at 0.45 µm and two drops of H2SO4 were added before storage at 4°C. The soil samples were tightly closed and kept in the freezer at −20°C. The biotic samples (roots and leaves) were thoroughly washed with MilliQ water and dried in a heat chamber at 40°C until reaching constant mass.
Frozen soil samples were lyophilized 72 h (FreeZone—LABCONCO) before sieving at 2 mm. Half of each sample was grounded with a ball mill (FRITSCH) and the other half kept ungrounded for scanning electron microscopy (SEM). Biotic samples for TM analysis were grounded using a cutting mill (POLYMIX–px-mfc90d) after drying. Dried samples were kept at room temperature, in the dark, and away from humidity.
2.2.3 Iron plaque extraction
In order to extract the iron plaque from the root surface, a treatment with a solution of dithionite-citrate-bicarbonate (DCB) established by Taylor and Crowder (1983) and modified by Lin et al. (2018) was used. Briefly, pneumatophores were immersed in 40 ml of the DCB solution (13% w/v Na3C6H5O7 •2H2O, 16% w/v NaHCO3, and 37% w/v Na2S2O4 in MilliQ water) for 3 h at room temperature. Samples were then rinsed with MilliQ water to obtain 50 ml of final volume. The extract was filtered at 0.45 µm and kept at 4°C. Treated samples were dried in a heat chamber at 40°C until reaching constant mass.
2.3 Analyses
2.3.1 Total elements extraction in soil samples
Total elements were extracted from soil samples by multiwave digestion. Samples between 5 and 20 cm, where the root system is mainly located, were used for BCF calculations. Briefly, 100 mg of sample was weighed in the vessel. 5 ml of 70% HNO3, 1 ml of 32% HCl, and 1 ml of 70% HF were added. Digestion was performed on an Ethos Easy–Milestone apparatus starting with a 25 min heating step from room temperature to 220°C followed by a 15 min plateau and a 20 min cooldown step. The extract was transferred to a Teflon tube and heated at 180°C on a heating plate to get rid of HF. After complete evaporation, 1 ml of HCl was added for dissolution of resistant particles. The extract was then transferred to a polypropylene tube, the volume was adjusted to 10 ml with MilliQ water and placed at 4°C until analysis. For quality control, certified material (MESS-3 Marine sediments) was also extracted and analyzed (Supplementary Table S1).
2.3.2 Trace metals extraction in biota
Trace metals were extracted from biotic samples by multiwave digestion. Briefly, 500 mg of sample was weighed in the vessel. 5 ml of 70% HNO3 and 1 ml of 30% H2O2 were added. The digestion program started with a 10 min heating step from room temperature to 160°C, then 15 min step to 210°C, followed by a 10 min plateau and a 20 min cooldown step. The extract was transferred to a polypropylene tube, the volume was adjusted to 15 ml with MilliQ water and placed at 4°C until analysis. For quality control, certified material (IPE sample ID 949) was also extracted and analyzed (Supplementary Table S2).
2.3.3 Soil sequential extractions
The Community Bureau of Reference sequential extractions were used for elemental analysis in soil samples (Rauret et al., 1999). Briefly, 500 mg of sample were weighed in a centrifuge tube. For the exchangeable fraction, 20 ml of 0.11 M acetic acid (pH 5) was added, and the sample was placed on a horizontal shaker for 16 h. The sample was centrifuged at 3,000 rpm for 20 min, and the supernatant was filtered at 0.45 µm and transferred to another tube. Water was added to the sample for washing, and after 20 min centrifugation at 3,000 rpm the water was discarded. For the reducible fraction, 20 ml of 0.5 M hydroxylamine hydrochloride (pH 2) was added to the sample. After 16 h of shaking, the extract was filtered and transferred to a tube. After the washing step, 5 ml of 8.8 M H2O2 was added to the sample to extract the oxidizable fraction. The sample sat for 1 h partially opened, and then placed in a diethylene glycol bath fully opened at 85°C. After 1 h, 5 ml of 8.8 M H2O2 were added and heated until completely dried. A total of 25 ml of 1 M ammonium acetate was then added to the sample, which was shaken for 16 h. After transfer of the supernatant to a tube and washing, the sample was dried at 80°C in a heat chamber. A dried residue of 400 mg was weighed in a vessel for total microwave digestion of the residual fraction (see 2.3.1).
2.3.4 Elemental analyses
Elemental concentrations in soil extracts, biotic extracts (leaves, roots of R. stylosa, and pneumatophores of A. marina), DCB solutions, and porewater were obtained via ICP-OES (Varian 730-ES) at the chemistry laboratory LAMA of the French Research Institute for Sustainable Development in New Caledonia. Concentrations were obtained using a calibration curve previously prepared with the right matrices from a stock solution of elements at 100 mg L−1. Certified reference materials were used to calculate a z-score for each element when available (Supplementary Table S1 and Supplementary Table S2). The |z-scores| range from 0.30 to 3.00 for the soil samples and 0.17 and 2.33 for the biotic samples.
2.3.5 Mineralogical analyses
The mineralogical composition of each soil sample was determined by X-Ray Diffraction (XRD), using a PANalytical - AERIS XRD Diffractometer equipped with a Co source at the ISEA laboratory. XRD patterns were recorded between 5 and 80 °2θ, with steps of 0.01, an acquisition time of 480 ms step−1, and using a generator power of 600 W. Diffractograms were treated and analyzed with the High Score software. XRD peaks were identified based on the Crystallography Open Database (COD_may22_2019).
2.3.6 Scanning electron microscopy
The petrography of bulk soil samples was performed by SEM using a JEOL JSM-IT 300 LV apparatus coupled with an energy dispersive spectroscopy Oxford CAM 80 device. The measurements were performed at a working distance of 10 mm and an energy of 15 kV.
2.4 Data analyses
2.4.1 Bioconcentration factors and translocation factors
The root and leaf BCF were calculated by dividing the concentration of TM in the tissue by the total mean concentration of TM in the soil between 5 and 20 cm, which corresponds to the depth sections where roots and pneumatophores were collected. The translocation factor (TF) was calculated by dividing the concentration of TM in leaves by the TM concentration in the roots of the same sample.
2.4.2 Iron plaque concentration calculation
The iron plaque was calculated following this equation:
where 0.1591 is the correction due to the fact that the iron plaque has the formula FeOOH but only Fe is measured in the DCB solution.
2.4.3 Statistical analyses
Statistical analyses were performed using R studio software (version 1.2.5001). For comparison between species or study sites the Mann-Whitney test was performed. All other statistical analyses with more than two variables were tested with a Kruskal–Wallis test followed by a Wilcoxon test. All tests were performed with a 95% confidence interval and n ≥ 3. kendall correlation analyses were performed to obtain correlation matrices.
3 Results
3.1 Physico-chemical parameters
Soil porewater salinity was significantly (p < 0.001) lower at the urban site compared to the control site. Soil porewater salinity at the urban site ranged between 0 g L−1 and 39 g L−1. At the control site, salinity ranged between 25 and 62 g L−1. Along the soil core of the control site, salinity under A. marina (mean = 48 g L−1) was significantly (p < 0.05) higher than under R. stylosa (mean = 39 g L−1). For both sites and both species, salinity was significantly (p < 0.05) lower close to the surface (Figure 2A).
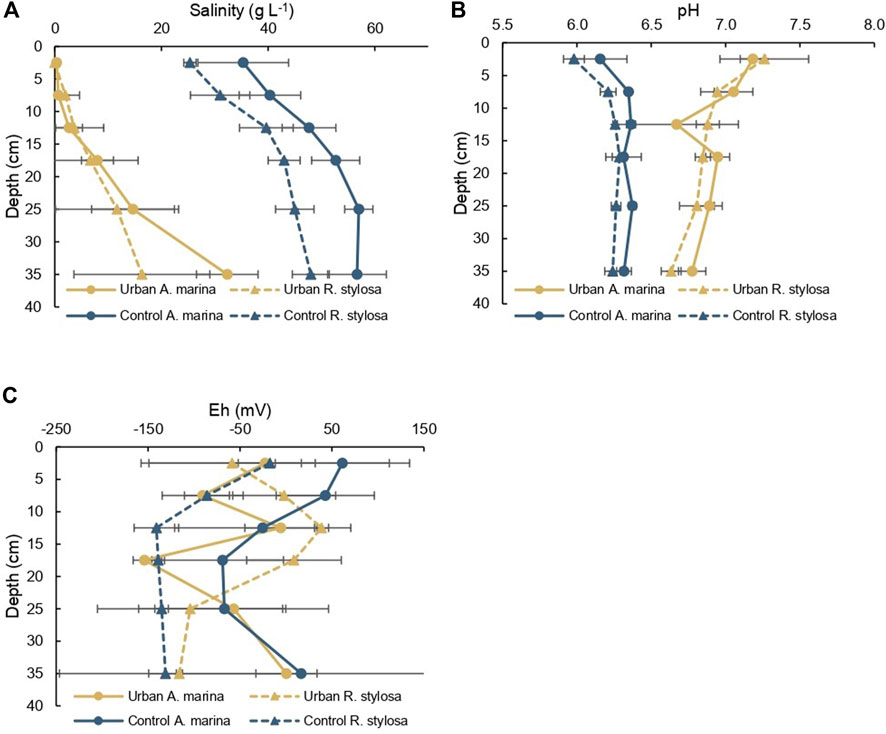
FIGURE 2. Mean values for (A) salinity in g L−1, (B) pH, and (C) Eh in mV along the soil cores of the urban and control sites under both mangrove species (A. marina and R. stylosa) with error bars corresponding to standard deviation of triplicates.
The urban site was significantly (p < 0.001) less acidic with a mean pH value of 6.9, compared with the control site, which had a mean pH value of 6.3. At the control site, the pH along the soil core was significantly (p < 0.01) more acidic beneath R. stylosa. At the urban site, the pH was significantly (p < 0.05) more basic at the surface than in depth (Figure 2B).
Eh values varied between −214 and 134 mV, and variability among triplicates was high. The Eh of the soil at the control site under R. stylosa (mean = −108 mV) was significantly more negative than the soil of the control site under A. marina (mean = −7 mV, p < 0.01), and the urban site under R. stylosa (mean = −39 mV, p < 0.05) (Figure 2C).
3.2 Mineralogy
Both sites develop downstream the same geological watershed characterized by a rock basement made of sandstones and limestones rich in quartz (SiO2). Quartz is the main species present in both mangrove soils. Several minerals from the mangrove soils seem to be also inherited from this substrate in both sites such as illite (KAl2Si4O12), laumontite (Ca[AlSi2O6]2), lizardite (Mg3Si2O5(OH)4) and plagioclase (Na/Ca[AlSi3O8]), likely albite (Figure 3). Pyrite (FeS2) was detected at both sites, from the surface to the bottom of the sample soil core at the control site, and only observed at 10 cm and below at the urban site. At the urban site, chlorite ((Fe,Mg,Al)6(Si,Al)4O10(OH)8) was observed above 20 cm (Figure 3A). At the control site only, hematite (Fe2O3) was also determined (Figure 3B).
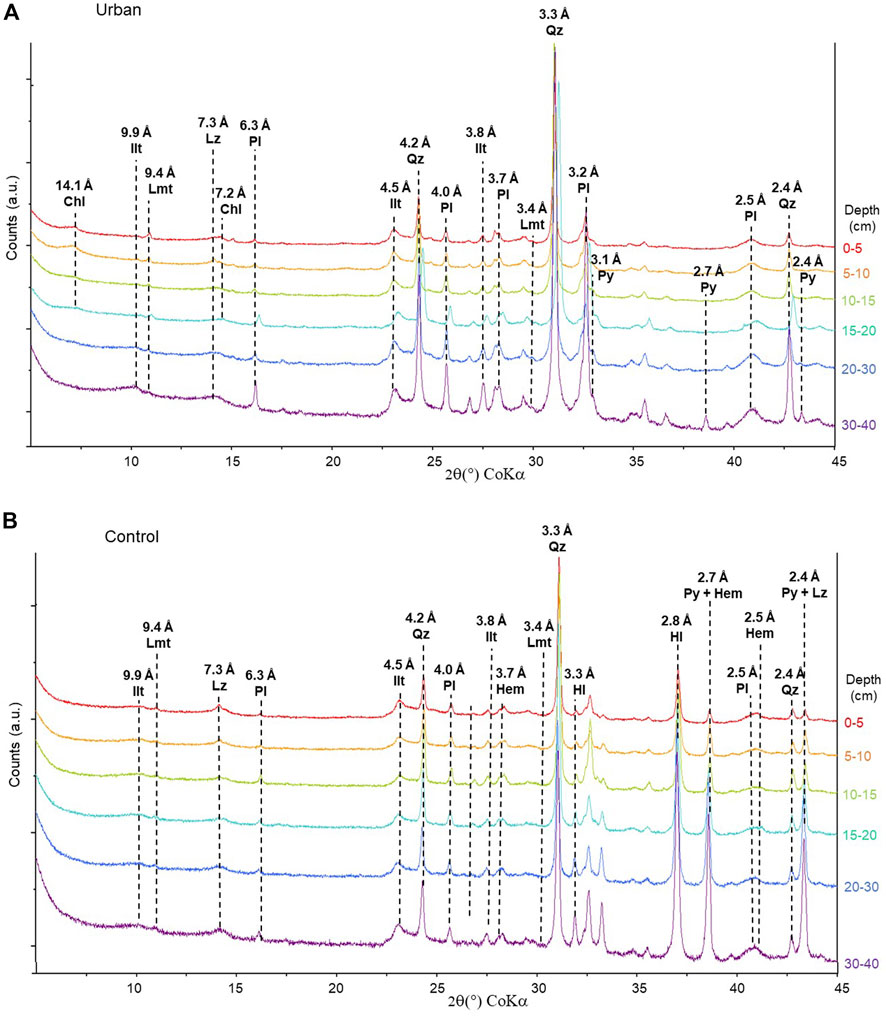
FIGURE 3. XRD diffractograms of the (A) urban site and (B) control site along soil core with depth and characteristic peaks labelled in d-spacing (Å). Chl–Chlorite, Hl–Halite, Hem–Hematite, Ilt–Illite, Lmt–Laumontite, Lz–Lizardite, Pl–Plagioclase, Py–Pyrite, Qz–Quartz.
3.3 Total elemental concentrations in soil
Most elements in the soil had concentrations significantly higher at the control site compared to the urban site (Table 1). Only, Cu, Pb, Ti beneath both mangrove species, and Mn and Zn beneath R. stylosa, were found in significantly higher concentrations in the urban mangrove soil compared with the control site. Under both mangrove species, Fe was the most concentrated measured element in the soil, with mean values of 30 g kg−1 at the urban site and 49 g kg−1 at the control site. The most concentrated TM after Fe at the urban site were Ti and Mn (4.6 and 0.1 g kg−1, respectively), and Ti and Cr at the control site (2.8 and 0.8 g kg−1, respectively). The least concentrated TM at both sites were As and Cd, with mean values of 1.5 and 1.3 mg kg−1 at the urban site, and 9.4 and 1.9 mg kg−1 at the control site, respectively.
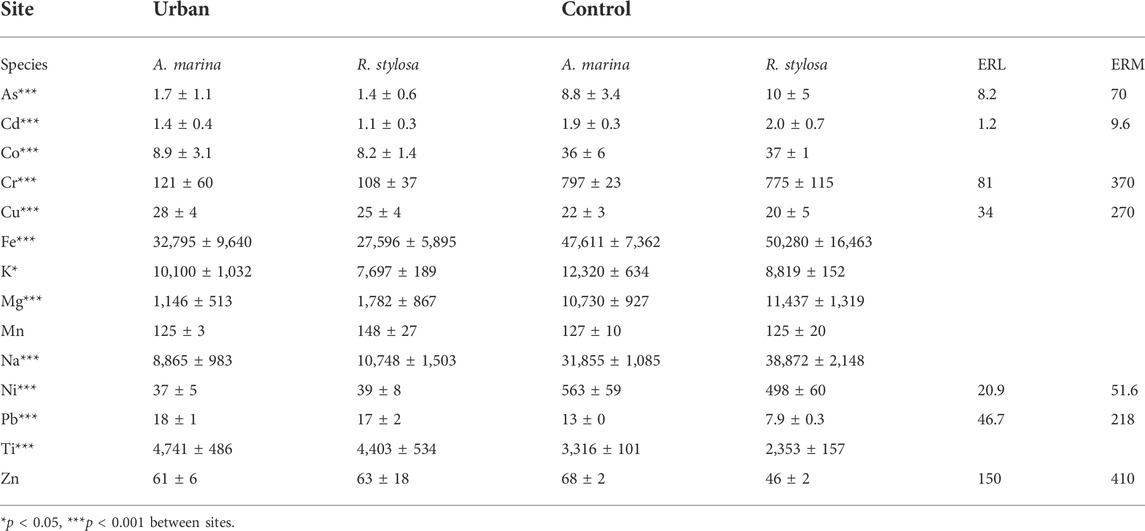
TABLE 1. Mean ± SD elemental total concentrations in soil (mg kg−1) from both sites and under both mangrove species with significant differences displayed and Effect Range Low (ERL) and Effect Range Median (ERM) of TM in marine sediments from NOAA.
3.4 Elemental soil fractions distribution
At the control site, all TM, except for As, were predominantly measured in the refractory fraction of the soil, representing 41% for Mg and 96% for Al (Figure 4A). At the urban site, Cd and Mg were primarily determined in the exchangeable fraction of the soil with 34% and 46%, respectively; As in the reducible fraction (86%), and Al and Cr in the oxidizable fraction of the soil with 58% and 50%, respectively (Figure 4B). In the exchangeable fraction of the soil, Al, Cu, Fe, and Mn were significantly (p < 0.05) more concentrated at the urban site. The same elements and Pb were significantly (p < 0.01) more concentrated in the reducible fraction at the urban site. Cu was significantly (p < 0.001) more concentrated in the oxidizable fraction at the urban site (Supplementary Table S3). All elements except for As and K were significantly (p < 0.05) less concentrated in the refractory fraction of the urban site compared to the control site (Supplementary Table S3).
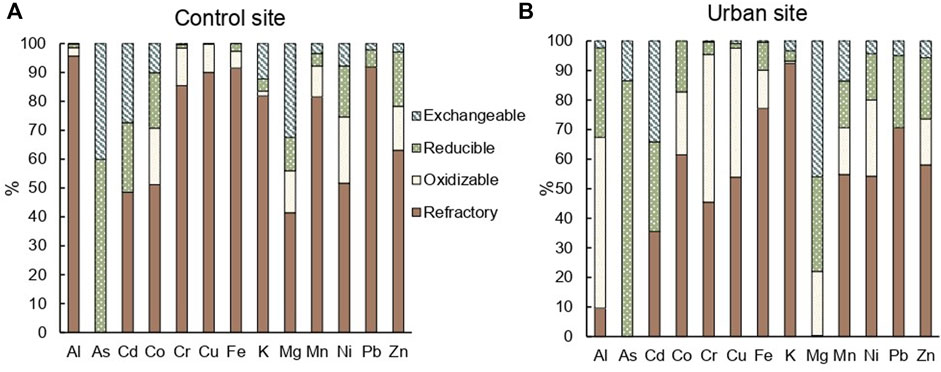
FIGURE 4. Mean percentage composition of elements in each fraction (exchangeable, reducible, oxidizable, refractory) obtained by sequential extractions of the entire soil cores in triplicates at the (A) control site and (B) urban site.
3.5 Trace metals in biota
Fe was the most concentrated TM in the roots of both species and at both sites with mean concentrations reaching up to 5.1 g kg−1 followed by Al with mean values up to 4.1 g kg−1. The least concentrated TM in the roots was Cd with mean values up to 0.2 mg kg−1. Al, Cd, Mn, and Ti were significantly more concentrated in the roots collected at the urban site compared to those collected at the control site (Table 2). At the urban site, Cd, Mn, Ni, and Pb concentrations in the roots were positively correlated to their concentrations in the exchangeable fraction of the soil with r = 0.73, 0.58, 0.47, and 0.47, respectively.
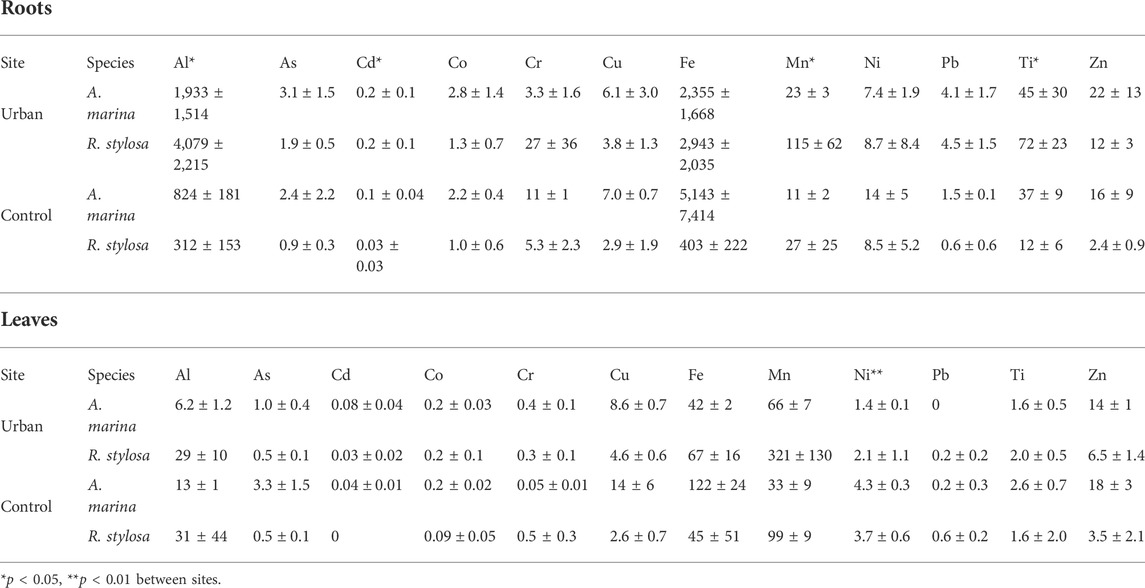
TABLE 2. Mean ± SD TM concentrations in roots and leaves in mg kg−1 at both sites for both mangrove species.
No significant difference was observed between sites for TM concentrations in the leaves, except for Ni, which had higher concentrations at the control site compared with the urban site. Mn and Fe were the most concentrated TM in the leaves with mean concentrations up to 321 and 122 mg kg−1, respectively. Cd, Co, and Pb were the least concentrated in the leaves. At both sites, Mn was more concentrated in the leaves than in the roots. At the control site, Zn was more concentrated in the leaves than in the roots of both species, and As and Cu only for A. marina (Table 2).
3.6 Bioconcentration factors and translocation factors
Mean root BCF ranged between 0.008 and 1.7. All TM, except Cu, Ti, and Zn, had significantly higher root BCF at the urban site. This site displayed the highest root BCF for As and Mn with 1.7 and 0.5, respectively. At the control site, Cu and Zn were the most proportionally transferred to the roots with root BCF of 0.23 and 0.14, respectively. Cr and Ti had the lowest root BCF with 0.042 and 0.013 at the urban site, and 0.010 and 0.008 at the control site, respectively (Figure 5A).
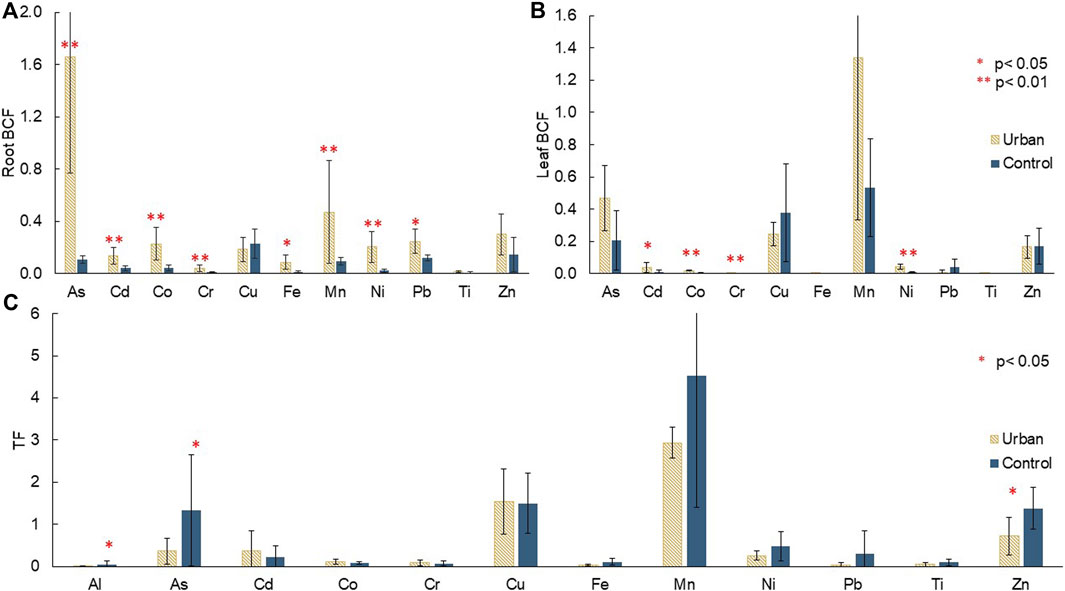
FIGURE 5. Mean (A) root bioconcentration factors (root BCF), (B) leaf bioconcentration factors (leaf BCF), and (C) translocation factors (TF) with error bars corresponding to standard deviation of triplicates for both species (A. marina and R. stylosa) at the urban and control sites, with significant differences displayed.
Some elements (Cd, Co, Cr, Ni) showed higher leaf BCF at the urban site. The most transferred TM from soil to leaves was Mn with leaf BCF of 1.34 at the urban site and 0.53 at the control site. The least transferred TM were Cr, Fe, and Ti with leaf BCF of 0.003, 0.002, and under detection limit at the urban site, and 0.0005, 0.002, and 0.001 at the control site, respectively (Figure 5B).
A greater proportion of As, Al, and Zn was significantly transferred from roots to leaves at the control site compared to the urban site (Figure 5C). Mn was once again the most transferred with TF values of 2.9 and 4.5 at the urban and control sites, respectively, and Al the least with 0.008 at the urban site and 0.51 at the control site.
3.7 Iron plaque
The iron plaque concentration at the surface of the pneumatophores of A. marina at the urban site is significantly higher than at the control site with means value of 81 mg kg−1 and 16 mg kg−1, respectively (Figure 6).
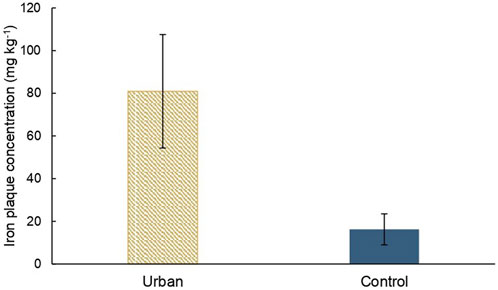
FIGURE 6. Mean iron plaque concentrations on A. marina pneumatophores’ surfaces (mg kg−1) at both sites with error bars.
4 Discussion
4.1 Soil characteristics of the mangrove forests
Mangrove species zonation at the control site is typical of semi-arid mangrove forests, with Rhizophora spp. trees developing on the seaside of the mangrove ecosystem, and A. marina developing on the landside at higher elevation. Being less frequently inundated, the soil in A. marina stand at the control site is more subjected to evaporation, explaining why porewater salinity was higher (Deborde et al., 2015). These differences in soil porewater salinity between mangrove species were not observed at the urban site. The inputs of urban rainwater runoff during decades, with a salinity of almost zero, disrupt the typical mangrove forests zonation. In fact, at the urban site, Rhizophora spp. trees develop also in the landside of the urban mangrove forest where the urban rainwater runoff enters the site. In addition, mangrove trees at the urban site, especially R. stylosa trees, are visually much taller than in most mangrove forests in New Caledonia, including the control site. The lower soil porewater salinity can explain this observation as mangrove trees spend less energy to adapt to highly saline conditions and have therefore more energy to spend to growth (Clough, 1984). At both sites, porewater salinity increased with depth, which may result from dilution with rain in the upper layer and salts percolation down the cores (Marchand et al., 2004; Bourgeois et al., 2020).
Beneath R. stylosa at the control site, Eh values were low (<100 mv), dissolved Fe concentrations were close to zero (Supplementary Figure S1), and pyrite was observed all along the core, even in the top soil, suggesting that sulphate-reduction was the dominant decay process as early as the first upper centimetres. Conversely at the urban mangrove site, Eh values were less negative, dissolved Fe concentrations reached up to 4,164 μg L−1 (Supplementary Figure S1), and pyrite was observed only at depth. Consequently, under R. stylosa, conditions were less anoxic at the urban mangrove site and other decay processes, like iron respiration, may dominate in the upper soil.
Despite suboxic conditions in the upper soil, pH values were less acidic than at the control site, and almost basic for some layers. Usually, in mangrove ecosystems, OM decomposition, along with pyrite oxidation, which can both be enhanced in suboxic conditions, induces a soil acidification (Marchand et al., 2004; Kristensen et al., 2017, 2008; Noël et al., 2014). However, in this specific urban mangrove ecosystem, the main pH driver is the amount of rainwater from the urban runoff, as the rainwater collected had a pH higher than 7.
Regarding A. marina, it is known that oxic to suboxic conditions usually prevail in the upper soil due to its root system, capable of diffusing O2 in its rhizosphere (Purnobasuki and Suzuki, 2005; Kristensen et al., 2017). At the control site, Eh values under A. marina varied between 61 and -69 mV, and large concentrations of dissolved Fe were measured (3,000–13,000 μg L−1) (Supplementary Figure S1), confirming suboxic conditions. Surprisingly, at the urban site, despite similar redox values, dissolved Fe concentrations were low (114–760 μg L−1) (Supplementary Figure S1). This result may either indicate different OM decay processes or export of dissolved elements from the mangrove soil. However, the absence of pyrite in the upper layer indicates that sulphate-reducing process is limited.
The disturbed mangrove forest zonation, the very low porewater salinity, and the high pH values measured at the urban site are clear indicators of the impact of the urban rainwater runoff on the mangrove forest. TM dynamics in the environment being dependant on these parameters, the partitioning and bioaccumulation of TM in soil and mangrove tissues must be evaluated in the urban and control mangrove sites.
4.2 Trace metals concentrations in mangrove soils
The two mangrove sites studied have a Cretaceous sedimentary watershed, dominated by sandstone and limestone (Service de la Géologie de Nouvelle-Calédonie, 2016), and are therefore less subjected to high concentrations of TM compared to mangrove forests located downstream lateritic soils (Noël et al., 2014). In both the urban and the control mangrove sites, lower soil concentrations of Co, Cr, Fe, Mn, and Ni (characteristic of New Caledonia’s lateritic soils) were measured compared to mangrove forests developing downstream mining activities in New Caledonia (Bourgeois et al., 2020; Robin et al., 2021). For instance, Cr and Ni concentrations measured in a mangrove site downstream a lateritic watershed were 17 times and 89 times greater than the mean concentrations at the urban site (Supplementary Table S3) (Robin et al., 2021). However, the studied mangrove sites were still characterized by higher concentrations of Cd, Cr, Fe, and Ni than many mangrove soils in the world such as in Australia, in New Zealand, in Mexico, in China, or in the Sundarbans (Supplementary Table S3) (Chaudhuri et al., 2014; Chowdhury et al., 2015; Bastakoti et al., 2019; Deng et al., 2019; Celis-Hernandez et al., 2020). Cr and Ni soil concentrations at the control site were much higher than the effect range median (ERM), which corresponds to the level of contamination of marine sediments, where biological toxicity effects are generally observed according to the National Oceanic and Atmospheric Administration (NOAA) (Table 1) (Buchman, 1999).
Regarding some TM (As, Cu, Pb, Zn) often associated to urbanization and of major public health concern according to the World Health Organization (Vracko et al., 2007), their concentrations were lower than the effect range low (ERL) (Table 1), a value below which biological toxicity effects are scarcely observed (Buchman, 1999). For instance, As, Cu, Pb, and Zn soil concentrations were up to 10 times lower than in the other mangrove forests of the world such as in China (He et al., 2014; Deng et al., 2019), in Tanzania (Kruitwagen et al., 2008), in Mexico (Celis-Hernandez et al., 2020), or in India (Ray et al., 2006), where extensive urbanization and industrial activities occur (Supplementary Table S3).
The high TM background of New Caledonia must therefore affect both sites, even though they have a sedimentary rocks-dominated watershed (Fernandez et al., 2006; Beliaeff et al., 2011; Maurizot et al., 2020), but the concentrations are well under those measured in mangrove forests directly affected by upstream lateritic soils. In addition, TM associated to urbanization and industrial activities do not reach hazardous concentrations, and are lower than in other urbanized world mangrove forests (Ray et al., 2006; Kruitwagen et al., 2008; He et al., 2014; Cao et al., 2018), implying that a large part of New Caledonia has not reached an alarming state for urban mangrove forest TM contamination.
The two studied sites share the same geological watershed, but the urban site has been influenced by an urban rainwater runoff for decades. It has been observed and mathematically modelled that urbanization leads to an increase in watershed erosion during the construction process, but can then inhibit sediment transport from the upper watershed to the coastal area (Randhir, 2003; O’Driscoll et al., 2010). Therefore, soil erosion and TM transport into the urban mangrove stands from the volcano-sedimentary watershed may be limited, explaining lower concentrations of the majority of TM in the urban mangrove soils. Conversely, at the control site, the presence of hematite, which is generally absent of the New Caledonia volcano-sedimentary watershed (Maurizot et al., 2020), indicates possible inputs from an adjacent watershed composed of lateritic soils (Service de la Géologie de Nouvelle-Calédonie, 2016; Robin et al., 2021). Even though the currents do not directly flow from the lateritic watershed to the control mangrove site (Douillet, 2001), the intense rainfall events that occurred during the rainy season impacted by a La Niña phenomena, or punctual cataclysmic events such as cyclones, may have induced larger inputs of minerals from the adjacent watershed in this control mangrove soil. For the elements significantly more concentrated at the urban site (Cu, Pb, Ti, Zn), they are typical of urban anthropogenic activities. In the environmental monitoring of the construction of the Dumbéa-sur-Mer allotment, the urban mangrove site showed higher Cu and Zn concentrations than all other monitored mangrove sites in Dumbéa (Ruiz et al., 2020). Cu, Pb, Ti, and Zn are used in many urban items in buildings and transportation. For instance, particles from car brakes increase the amount of Cu in environments close to highways, which is the case for our urban mangrove site (Davis et al., 2001). Cu, Pb, Ti, and Zn are also used in building sidings (e.g., bricks, painted walls), roofs, and tire wear (Davis et al., 2001; Neal et al., 2011; Panagos et al., 2018). These TM can therefore be in higher concentrations in the urban mangrove soils due to greater inputs from the rainwater runoff.
As detailed in the previous section, mangrove soil at the urban site is characterized by suboxic conditions in the upper layer, and anoxic ones at depth. These suboxic conditions in the upper layer result in high TM concentrations in the dissolved phase. In fact, Cr and Fe were significantly more concentrated in the porewater of the urban site beneath R. stylosa stand (Supplementary Figure S1). Noël et al. (2014) suggested that tidal fluctuations may be a major cause for continuous Fe reduction-oxidation cycles in mangrove soils, which could significantly affect the Fe mass balance with the exports of dissolved Fe in stands closest to the sea. Deborde et al. (2015) suggested that the loss of some elements towards the seaside may be related to sulphur oxidation and to more intense tidal flushing of dissolved elements. In the present study, redox conditions induced the presence of high concentrations of dissolved TM, which can be easily exported towards adjacent ecosystems or being subject to plant uptake. These observations could explain in part why concentrations of most TM are lower in the urban mangrove soils compared to the control site.
4.3 Trace metals transfer to mangrove roots
In the mangrove sites studied, root concentrations of Co, Cr, Cu, Fe, Ni, Pb, and Zn were lower than in roots collected downstream highly eroded lateritic soil in New Caledonia (Marchand et al., 2016; Bourgeois et al., 2020; Robin et al., 2021). In fact, Fe and Ni mean root concentrations were 8 and 150 times greater in mangrove plants collected downstream a Ni mine (Bourgeois et al., 2020). However, root concentrations of As, Cr, Fe, and Ni were between 8 and 30 times greater in the present study than in the poorly urbanized mangrove forest of the Sundarbans (Chowdhury et al., 2015), which can be attributed to the high TM background of New Caledonia. Nonetheless, Cu, Pb, and Ti, which are usually associated to urbanization, were 20–40 times more concentrated in the roots of mangrove trees collected in the Sydney harbour, where there is an extensive boat traffic that can cause greater discharge of those TM in the environment (Chaudhuri et al., 2014).
In the urban mangrove site, despite lower TM concentrations in the soil, concentrations of some TM in the roots were higher than at the control site, suggesting different TM uptake mechanisms by the mangrove trees between sites, and/or distinct TM bioavailability in the soil. Al, Cd, Mn, and Ti were between 2 and 5 times more concentrated in the roots collected at the urban site. In addition, root BCF were significantly higher at the urban site, up to 20 times for some TM, with values similar or higher to what is observed in mangrove stands developing downstream highly eroded lateritic soils in New Caledonia (Marchand et al., 2016; Bourgeois et al., 2020).
TM in the exchangeable fraction of the soil are supposedly more bioavailable for plant uptake (Marchand et al., 2016; Bourgeois et al., 2020). Here, in the exchangeable fraction, Al, Cu, Fe, and Mn were significantly more concentrated at the urban site compared to the control site (Supplementary Table S4) explaining higher root BCF at the urban site. In addition, there was a positive correlation between concentrations in the roots and concentrations in the exchangeable fractions of the soil for Cd, Mn, Ni, and Pb in the urban site. This relationship was not observed at the control site possibly because of sulphate-reducing processes and the presence of salt. As soon as TM enters the dissolved phase, they can be trapped immediately either by pyrite (Noël et al., 2017) or salt (Cheng et al., 2014). XRD analysis showed that pyrite was present all along the core at the control site, but only at depth in the urban mangrove site, which has suboxic conditions in top soils. In addition, Ni associated to framboidal pyrite has been observed on SEM at the surface of the control site (Supplementary Figure S2), but not at the urban site. Consequently, mangrove roots at the urban site have more access to TM such as Ni and Cd that are not trapped within pyrite crystals. In addition, soil porewater salinity was significantly lower at the urban site due to the input of urban rainwater. It has been reported that soil salinity affects TM uptake by mangrove roots as Cl-TM complexes can form, which makes it more difficult to absorb by the plant (Cheng et al., 2014). Li et al. (2018) observed high negative correlations between the concentrations of Cd in the roots of a mangrove species and its content of Na and Cl. The urban mangrove soil may form less Cl-TM complexes, favouring TM uptake by mangrove roots. TM bioavailability in the soil seems therefore to be the main factor influencing the transfer to mangrove roots, as previously suggested (Ray et al., 2006; Chaudhuri et al., 2014; Thanh-Nho et al., 2019). A combined analysis with leaf BCF and TF helps identify the role and the biological mechanisms of mangrove trees facing TM bioavailability.
4.4 Trace metals transfer to mangrove leaves
There was no difference in TM concentrations in leaves between the urban and the control mangrove sites, except for Ni, which was more concentrated in the leaves at the control site. Concentrations in leaves of Cr, Pb, and Zn, TM with potentially an urban origin, were at least 5 times lower than what is reported in the literature for a large urban area in Futian, China (He et al., 2014). Regarding TM that mainly originate from lateritic soils in New Caledonia, Co, Fe, and Ni, their concentrations in the leaves of the urban mangrove site were 10 to 50 times lower than in leaves collected downstream an open-cast Ni mine in New Caledonia (Marchand et al., 2016). Therefore, the studied mangrove sites do not seem affected in terms of TM accumulation in the leaves compared to other stressed mangrove forests.
With lower TM total concentrations in the soil, and similar concentrations in the leaves, the urban mangrove trees have higher leaf BCF for Cd, Co, Cr, and Ni. The leaf BCF of Co and Ni were actually greater at the urban site compared to the values obtained in a mangrove forest developing nearby a large urban area in China (He et al., 2014) and compared to a mangrove forest downstream a big city in Vietnam (Thanh-Nho et al., 2019). Ni leaf BCF were also higher than downstream a Ni mine in New Caledonia (Bourgeois et al., 2020). The results suggest that transfer of elements in the leaves, other than macronutrients, are rather negatively correlated to soil concentrations, either because TM are not bioavailable in the soil for plant uptake, or because of biological mechanisms, immobilizing TM in the root system. For example, chelation of TM cations with the roots cell walls can inhibit TM transfer to the vascular bundle, and thus to other organs (MacFarlane et al., 2007). Arbuscular mycorrhizal fungi in symbiosis with mangrove trees in the root system (D’Souza, 2016) can also help prevent TM transfer to the higher organs (Garg and Chandel, 2011; Hassan et al., 2013). For A. marina, the formation of an iron plaque at the surface of the roots and pneumatophore can help retain extensive amounts of some TM (Pi et al., 2011; Robin et al., 2021). The results show that there is significantly more iron plaque formation at the surface of the pneumatophores at the urban site. This mechanism limits the uptake of TM in the roots of the urban mangrove trees, and therefore protects the higher tissues from metallic stress.
The measured TF for most TM were similar to those measured in other mangrove forests around the globe, such as in China (Huang X et al., 2020; Zhou et al., 2011), in Hong Kong (Eric, 2003), and in Iran (Rezaei et al., 2021). However, TF were lower in our studied mangrove sites by up to two order of magnitudes, compared to the TF values obtained in the Sundarbans (Ray et al., 2021). The concentrations of Al, Cd, Co, Fe, Pb, and Zn measured in the leaves by Ray et al. (2021) were similar or higher than the concentrations measured in this study, while TM concentrations in the roots were much lower than those measured herein. This result indicates transfer regulation of TM from roots to leaves. Ray et al. (2021) suggested that exceeding nutrients or toxic elements can be excluded from the plant organism via litterfall, but the lower TF measured in our study sites suggests that this is not the primary strategy adopted by the mangrove trees in the urban context studied. With similar concentrations in the roots at the urban site, and comparable concentrations in the leaves, TF of most TM were similar at the urban and control sites implying that, even in an urban context, mangrove trees limit the transfer of potentially toxic TM to the higher organs where vital processes occur such as photosynthesis.
Our results show that the urban rainwater runoff increases the bioavailability of TM in the urban mangrove soil compared to the control site by modifying TM bearing phases and soil physico-chemical parameters. Consequently, urbanization, via the inputs of urban effluents in the mangrove forest, is a factor able to affect roots uptake. However, its influence on TM transfer from roots to shoots seems to be limited. TM soil concentrations and bearing phases influence primarily the root BCF, while mangrove species biology and physiology regulate TF and accumulation in the leaves.
5 Conclusion
This study provides new insights on the influence of urban rainwater runoff on mangrove forests. The comparison between an urban mangrove forest and a control mangrove forest sharing the same geological watershed demonstrates that the almost continuous runoff of urban rainwater modified the natural physico-chemical properties of the mangrove soil and disrupt TM dynamics. The basic freshwater urban runoff controlled mangrove soil porewater salinity and pH, and induced suboxic conditions influencing TM distribution. Most total TM soil concentrations were lower in the mangrove soil influenced by urbanization due potentially to, on the one hand, watershed urbanization that reduced TM inputs, and on the other hand, to more TM bioavailability resulting from the suboxic conditions. Only Cu, Pb, Ti, and Zn presented higher concentrations in the urban mangrove soils; these TM are known to derive from urban activities and inputs can be attributed to the urban rainwater runoff. The urban rainwater runoff limits salt-TM complexes or pyrite-TM trapping in soil, which can lead to the lower TM bioavailability. The higher bioavailability resulted in higher root bioconcentration factors for both mangrove species, A. marina and R. stylosa. However, TM concentrations in leaves are similar between the two studied mangrove forests and translocation factors do not differ between sites suggesting a regulation of the transfer of TM to the leaves by the mangrove trees. In order to improve the understanding of the effects of the urban runoff on the mangrove ecosystem, further analysis should be performed on the nature of the OM in the soil and on TM exports to adjacent coastal waters. A monitoring of various parameters of the urban rainwater runoff should also be done periodically over a year to assess the nature of the inputs in the urban mangrove site.
Data availability statement
The original contributions presented in the study are included in the article/Supplementary material, further inquiries can be directed to the corresponding author.
Author contributions
SR: Conceptualization, Methodology, Investigation, Resources, Writing—Original draft, Writing—Review and editing, Visualization CM: Conceptualization, Methodology, Resources, Writing—Review and editing, Supervision, Funding acquisition MM: Term, Investigation, Writing—Review and editing FB: Investigation, Writing—Review and editing AA: Writing—Review and editing.
Funding
This work was supported by Banque de la Nouvelle-Calédonie, Cegelec, SECAL, and Fibrelec via the University of New Caledonia Foundation.
Acknowledgments
The authors acknowledge Kapeliele Gututauava for technical help on site. Aurélie Monin and Olivia Barthélémy are acknowledged for the technical assistance during SEM observations. The authors thank Leocadie Jamet and Monika Lemestre for ICP-OES measurements.
Conflict of interest
The authors declare that the research was conducted in the absence of any commercial or financial relationships that could be construed as a potential conflict of interest.
Publisher’s note
All claims expressed in this article are solely those of the authors and do not necessarily represent those of their affiliated organizations, or those of the publisher, the editors and the reviewers. Any product that may be evaluated in this article, or claim that may be made by its manufacturer, is not guaranteed or endorsed by the publisher.
Supplementary material
The Supplementary Material for this article can be found online at: https://www.frontiersin.org/articles/10.3389/fenvs.2022.1054554/full#supplementary-material
Abbreviations
BCF, Bioconcentration factor; DCB, Dithionite citrate bicarbonate; ERL, Effect range low; ERM, Effect range median; NOAA, National oceanic and atmospheric administration; OM, Organic matter; TF, Translocation factor; TM, Trace metals; SEM, Scanning electron microscopy; XRD, X-ray diffraction.
References
Alemu, J. B., Richards, D. R., Gaw, L. Y.-F., Masoudi, M., Nathan, Y., and Friess, D. A. (2021). Identifying spatial patterns and interactions among multiple ecosystem services in an urban mangrove landscape. Ecol. Indic. 121, 107042. doi:10.1016/j.ecolind.2020.107042
Alongi, D. M. (2002). Present state and future of the world’s mangrove forests. Envir. Conserv. 29, 331–349. doi:10.1017/S0376892902000231
Bastakoti, U., Bourgeois, C., Marchand, C., and Alfaro, A. C. (2019). Urban-rural gradients in the distribution of trace metals in sediments within temperate mangroves (New Zealand). Mar. Pollut. Bull. 149, 110614. doi:10.1016/j.marpolbul.2019.110614
Batty, L. (2000). The effect of pH and plaque on the uptake of Cu and Mn in phragmites australis(cav.) trin ex. Steudel. Ann. Bot. 86, 647–653. doi:10.1006/anbo.2000.1191
Bayen, S. (2012). Occurrence, bioavailability and toxic effects of trace metals and organic contaminants in mangrove ecosystems: A review. Environ. Int. 48, 84–101. doi:10.1016/j.envint.2012.07.008
Beliaeff, B., Bouvet, G., Fernandez, J.-M., David, C., and Laugier, T. (2011). Guide pour le suivi de la qualité du milieu marin en Nouvelle-Calédonie, programme ZONECO et programme CNRT Le Nickel. Nouméa: CNRT Nickel et son environnement.
Bourgeois, C., Alfaro, A. C., Bisson, E., Alcius, S., and Marchand, C. (2020). Trace metal dynamics in soils and plants along intertidal gradients in semi-arid mangroves (New Caledonia). Mar. Pollut. Bull. 156, 111274. doi:10.1016/j.marpolbul.2020.111274
Brown, G. E., and Parks, G. A. (2001). Sorption of trace elements on mineral surfaces: Modern perspectives from spectroscopic studies, and comments on sorption in the marine environment. Int. Geol. Rev. 43, 963–1073. doi:10.1080/00206810109465060
Buchman, M. F. (1999). NOAA screening quick reference tables (No. 99–1), HAZMAT report. Seattle, WA: National Oceanic and Atmospheric Administration.
Cao, C., Wang, L., Li, H., Wei, B., and Yang, L. (2018). Temporal variation and ecological risk assessment of metals in soil nearby a Pb–Zn mine in Southern China. Int. J. Environ. Res. Public Health 15, 940. doi:10.3390/ijerph15050940
Cavalcante, R. M., Sousa, F. W., Nascimento, R. F., Silveira, E. R., and Freire, G. S. S. (2009). The impact of urbanization on tropical mangroves (Fortaleza, Brazil): Evidence from PAH distribution in sediments. J. Environ. Manag. 91, 328–335. doi:10.1016/j.jenvman.2009.08.020
Celis-Hernandez, O., Giron-Garcia, M. P., Ontiveros-Cuadras, J. F., Canales-Delgadillo, J. C., Pérez-Ceballos, R. Y., Ward, R. D., et al. (2020). Environmental risk of trace elements in mangrove ecosystems: An assessment of natural vs oil and urban inputs. Sci. Total Environ. 730, 138643. doi:10.1016/j.scitotenv.2020.138643
Center for International Earth Science Information Network (2012). Population, landscape, and climate estimates. (PLACE), v3 (1990, 2000, 2010) [WWW Document]. NASA Socioeconomic Data and Applications Center. Available at: https://sedac.ciesin.columbia.edu/data/set/nagdc-population-landscape-climate-estimates-v3 (accessed 18 4, 22).
Chakraborty, P., Chakraborty, S., Vudamala, K., Sarkar, A., and Nath, B. N. (2016). Partitioning of metals in different binding phases of tropical estuarine sediments: Importance of metal chemistry. Environ. Sci. Pollut. Res. 23, 3450–3462. doi:10.1007/s11356-015-5475-6
Chaudhuri, P., Nath, B., and Birch, G. (2014). Accumulation of trace metals in grey mangrove Avicennia marina fine nutritive roots: The role of rhizosphere processes. Mar. Pollut. Bull. 79, 284–292. doi:10.1016/j.marpolbul.2013.11.024
Cheng, H., Chen, D.-T., Tam, N. F.-Y., Chen, G.-Z., Li, S.-Y., and Ye, Z.-H. (2012). Interactions among Fe2+, S2–, and Zn2+ tolerance, root anatomy, and radial oxygen loss in mangrove plants. J. Exp. Bot. 63, 2619–2630. doi:10.1093/jxb/err440
Cheng, H., Jiang, Z.-Y., Liu, Y., Ye, Z.-H., Wu, M.-L., Sun, C.-C., et al. (2014). Metal (Pb, Zn and Cu) uptake and tolerance by mangroves in relation to root anatomy and lignification/suberization. Tree Physiol. 34, 646–656. doi:10.1093/treephys/tpu042
Chowdhury, R., Favas, P. J. C., Pratas, J., Jonathan, M. P., Ganesh, P. S., and Sarkar, S. K. (2015). Accumulation of trace metals by mangrove plants in Indian sundarban wetland: Prospects for phytoremediation. Int. J. Phytoremediation 17, 885–894. doi:10.1080/15226514.2014.981244
Clough, B. (1984). Growth and salt balance of the mangroves Avicennia marina (Forsk.) Vierh. and Rhizophora stylosa Griff. in relation to salinity. Funct. Plant Biol. 11, 419. doi:10.1071/PP9840419
Davis, A. P., Shokouhian, M., and Ni, S. (2001). Loading estimates of lead, copper, cadmium, and zinc in urban runoff from specific sources. Chemosphere 44, 997–1009. doi:10.1016/S0045-6535(00)00561-0
Deborde, J., Marchand, C., Molnar, N., Patrona, L., and Meziane, T. (2015). Concentrations and fractionation of carbon, iron, sulfur, nitrogen and phosphorus in mangrove sediments along an intertidal gradient (semi-arid climate, New Caledonia). J. Mar. Sci. Eng. 3, 52–72. doi:10.3390/jmse3010052
Deng, J., Guo, P., Zhang, X., Shen, X., Su, H., Zhang, Y., et al. (2019). An evaluation on the bioavailability of heavy metals in the sediments from a restored mangrove forest in the Jinjiang Estuary, Fujian, China. Ecotoxicol. Environ. Saf. 180, 501–508. doi:10.1016/j.ecoenv.2019.05.044
Douillet, P. (2001). Atlas hydrodynamique du lagon sud-ouest de Nouvelle-Calédonie. Nouméa: Institut national de la recherche et du développement (IRD).
Douillet, P., Ouillon, S., and Cordier, E. (2001). A numerical model for fine suspended sediment transport in the southwest lagoon of New Caledonia. Coral Reefs 20, 361–372. doi:10.1007/s00338-001-0193-6
D’Souza, J. (2016). “Arbuscular mycorrhizal diversity from mangroves: A review,” in Recent advances on mycorrhizal fungi, fungal biology. Editor M. C. Pagano (Cham: Springer International Publishing), 109–116. doi:10.1007/978-3-319-24355-9_10
Duan, D., Lan, W., Chen, F., Lei, P., Zhang, H., Ma, J., et al. (2020). Neutral monosaccharides and their relationship to metal contamination in mangrove sediments. Chemosphere 251, 126368. doi:10.1016/j.chemosphere.2020.126368
Eric, T. P. K. (2003). Heavy metals contents in sediments, mangroves and bivalves from Ting Kok, Hong Kong. China Environ. Sci. 23, 480–484.
Fernandez, J.-M., Ouillon, S., Chevillon, C., Douillet, P., Fichez, R., and Gendre, R. L. (2006). A combined modelling and geochemical study of the fate of terrigenous inputs from mixed natural and mining sources in a coral reef lagoon (New Caledonia). Mar. Pollut. Bull. 52, 320–331. doi:10.1016/j.marpolbul.2005.09.010
Ferreira, T. O., Otero, X. L., Vidal-Torrado, P., and Macías, F. (2007). Effects of bioturbation by root and crab activity on iron and sulfur biogeochemistry in mangrove substrate. Geoderma 142, 36–46. doi:10.1016/j.geoderma.2007.07.010
Fritioff, Å., Kautsky, L., and Greger, M. (2005). Influence of temperature and salinity on heavy metal uptake by submersed plants. Environ. Pollut. 133, 265–274. doi:10.1016/j.envpol.2004.05.036
Garg, N., and Chandel, S. (2011). “Arbuscular mycorrhizal networks: Process and functions,” in Sustainable agriculture volume 2. Editors E. Lichtfouse, M. Hamelin, M. Navarrete, and P. Debaeke (Dordrecht: Springer Netherlands), 907–930. doi:10.1007/978-94-007-0394-0_40
Ge, Y., and Li, Z. (2018). Application of lignin and its derivatives in adsorption of heavy metal ions in water: A review. ACS Sustain. Chem. Eng. 6, 7181–7192. doi:10.1021/acssuschemeng.8b01345
Harbison, P. (1986). Mangrove muds—a sink and a source for trace metals. Mar. Pollut. Bull. 17, 246–250. doi:10.1016/0025-326X(86)90057-3
Hassan, S. E., Hijri, M., and St-Arnaud, M. (2013). Effect of arbuscular mycorrhizal fungi on trace metal uptake by sunflower plants grown on cadmium contaminated soil. New Biotechnol. 30, 780–787. doi:10.1016/j.nbt.2013.07.002
He, B., Li, R., Chai, M., and Qiu, G. (2014). Threat of heavy metal contamination in eight mangrove plants from the Futian mangrove forest, China. Environ. Geochem. Health 36, 467–476. doi:10.1007/s10653-013-9574-3
Holloway, C. J., Santos, I. R., and Rose, A. L. (2018). Porewater inputs drive Fe redox cycling in the water column of a temperate mangrove wetland. Estuar. Coast. Shelf Sci. 207, 259–268. doi:10.1016/j.ecss.2018.04.016
Holloway, C. J., Santos, I. R., Tait, D. R., Sanders, C. J., Rose, A. L., Schnetger, B., et al. (2016). Manganese and iron release from mangrove porewaters: A significant component of oceanic budgets? Mar. Chem. 184, 43–52. doi:10.1016/j.marchem.2016.05.013
Huang, S., Jiang, R., Song, Q., Zhang, Y., Huang, Q., Su, B., et al. (2020). Study of mercury transport and transformation in mangrove forests using stable mercury isotopes. Sci. Total Environ. 704, 135928. doi:10.1016/j.scitotenv.2019.135928
Huang, X., Wang, X., Li, X., Yan, Z., and Sun, Y. (2020). Occurrence and transfer of heavy metals in sediments and plants of Aegiceras corniculatum community in the Qinzhou Bay, southwestern China. Acta Oceanol. Sin. 39, 79–88. doi:10.1007/s13131-020-1555-7
Insee (2020). Population légale de la Nouvelle-Calédonie en 2019. Institut de la statistique et des études économiques.
Jayachandran, S., Chakraborty, P., Ramteke, D., Chennuri, K., and Chakraborty, S. (2018). Effect of pH on transport and transformation of Cu-sediment complexes in mangrove systems. Mar. Pollut. Bull. 133, 920–929. doi:10.1016/j.marpolbul.2018.03.054
Kristensen, E., Bouillon, S., Dittmar, T., and Marchand, C. (2008). Organic carbon dynamics in mangrove ecosystems: A review. Aquat. Bot. 89, 201–219. doi:10.1016/j.aquabot.2007.12.005
Kristensen, E., Connolly, R. M., Otero, X. L., Marchand, C., Ferreira, T. O., and Rivera-Monroy, V. H. (2017). “Biogeochemical cycles: Global approaches and perspectives,” in Mangrove ecosystems: A global biogeographic perspective. Editors V. H. Rivera-Monroy, S. Y. Lee, E. Kristensen, and R. R. Twilley (Cham: Springer International Publishing), 163–209. doi:10.1007/978-3-319-62206-4_6
Kruitwagen, G., Pratap, H. B., Covaci, A., and Wendelaar Bonga, S. E. (2008). Status of pollution in mangrove ecosystems along the coast of Tanzania. Mar. Pollut. Bull. 56, 1022–1031. doi:10.1016/j.marpolbul.2008.02.018
Lee, S. Y., Primavera, J. H., Dahdouh-Guebas, F., McKee, K., Bosire, J. O., Cannicci, S., et al. (2014). Ecological role and services of tropical mangrove ecosystems: A reassessment: Reassessment of mangrove ecosystem services. Glob. Ecol. Biogeogr. 23, 726–743. doi:10.1111/geb.12155
Lewis, M., Pryor, R., and Wilking, L. (2011). Fate and effects of anthropogenic chemicals in mangrove ecosystems: A review. Environ. Pollut. 159, 2328–2346. doi:10.1016/j.envpol.2011.04.027
Li, J., Yu, J., Yan, C., Du, D., Liu, J., and Lu, H. (2018). Distribution correlations of cadmium to calcium, phosphorus, sodium and chloridion in mangrove Aegiceras corniculatum root tissues. Mar. Pollut. Bull. 126, 179–183. doi:10.1016/j.marpolbul.2017.10.074
Lin, Y., Fan, J., Yu, J., Jiang, S., Yan, C., and Liu, J. (2018). Root activities and arsenic translocation of Avicennia marina (Forsk.) Vierh seedlings influenced by sulfur and iron amendments. Mar. Pollut. Bull. 135, 1174–1182. doi:10.1016/j.marpolbul.2018.08.040
MacFarlane, G. R., and Burchett, M. D. (2001). Photosynthetic pigments and peroxidase activity as indicators of heavy metal stress in the grey mangrove, Avicennia marina (Forsk.) Vierh. Mar. Pollut. Bull. 42, 233–240. doi:10.1016/s0025-326x(00)00147-8
MacFarlane, G. R., and Burchett, M. D. (2002). Toxicity, growth and accumulation relationships of copper, lead and zinc in the grey mangrove Avicennia marina (Forsk.) Vierh. Mar. Environ. Res. 54, 65–84. doi:10.1016/S0141-1136(02)00095-8
MacFarlane, G. R., Koller, C. E., and Blomberg, S. P. (2007). Accumulation and partitioning of heavy metals in mangroves: A synthesis of field-based studies. Chemosphere 69, 1454–1464. doi:10.1016/j.chemosphere.2007.04.059
MacFarlane, G. R. (2002). Leaf biochemical parameters in Avicennia marina (Forsk.) Vierh as potential biomarkers of heavy metal stress in estuarine ecosystems. Mar. Pollut. Bull. 44, 244–256. doi:10.1016/S0025-326X(01)00255-7
Marchand, C., Baltzer, F., Lallier-Vergès, E., and Albéric, P. (2004). Pore-water chemistry in mangrove sediments: Relationship with species composition and developmental stages (French guiana). Mar. Geol. 208, 361–381. doi:10.1016/j.margeo.2004.04.015
Marchand, C., Disnar, J. R., Lallier-Vergès, E., and Lottier, N. (2005). Early diagenesis of carbohydrates and lignin in mangrove sediments subject to variable redox conditions (French Guiana). Geochimica Cosmochimica Acta 69, 131–142. doi:10.1016/j.gca.2004.06.016
Marchand, C., Fernandez, J.-M., Moreton, B., Landi, L., Lallier-Vergès, E., and Baltzer, F. (2012). The partitioning of transitional metals (Fe, Mn, Ni, Cr) in mangrove sediments downstream of a ferralitized ultramafic watershed (New Caledonia). Chem. Geol. 300 (301), 70–80. doi:10.1016/j.chemgeo.2012.01.018
Marchand, C., Fernandez, J.-M., and Moreton, B. (2016). Trace metal geochemistry in mangrove sediments and their transfer to mangrove plants (New Caledonia). Sci. Total Environ. 562, 216–227. doi:10.1016/j.scitotenv.2016.03.206
Marchand, C., Lallier-Vergès, E., and Allenbach, M. (2011). Redox conditions and heavy metals distribution in mangrove forests receiving effluents from shrimp farms (Teremba Bay, New Caledonia). J. Soils Sediments 11, 529–541. doi:10.1007/s11368-010-0330-3
Marchand, C., Virly, S., Buisson, D., and Duke, N. (2007). Typologies et biodiversité des mangroves de Nouvelle-Calédonie 213.
Marx, S. K., and McGowan, H. A. (2010). “Long-distance transport of urban and industrial metals and their incorporation into the environment: Sources, transport pathways and historical trends,” in Urban airborne particulate matter, environmental science and engineering. Editors F. Zereini, and C. L. S. Wiseman (Berlin, Heidelberg: Springer Berlin Heidelberg), 103–124. doi:10.1007/978-3-642-12278-1_6
Maurizot, P., Robineau, B., Vendé-Leclerc, M., and Cluzel, D. (2020). Chapter 1 Introduction to New Caledonia: Geology, geodynamic evolution and mineral resources. Memoirs 51, 1–12. doi:10.1144/M51-2019-33
Naidoo, G., Hiralal, T., and Naidoo, Y. (2014). Ecophysiological responses of the mangrove Avicennia marina to trace metal contamination. Flora - Morphol. Distribution, Funct. Ecol. Plants 209, 63–72. doi:10.1016/j.flora.2013.10.003
Neal, C., Jarvie, H., Rowland, P., Lawler, A., Sleep, D., and Scholefield, P. (2011). Titanium in UK rural, agricultural and urban/industrial rivers: Geogenic and anthropogenic colloidal/sub-colloidal sources and the significance of within-river retention. Sci. Total Environ. 409, 1843–1853. doi:10.1016/j.scitotenv.2010.12.021
Noël, V., Juillot, F., Morin, G., Marchand, C., Ona-Nguema, G., Viollier, E., et al. (2017). Oxidation of Ni-rich mangrove sediments after isolation from the sea (dumbea Bay, New Caledonia): Fe and Ni behavior and environmental implications. ACS Earth Space Chem. 1, 455–464. doi:10.1021/acsearthspacechem.7b00005
Noël, V., Marchand, C., Juillot, F., Ona-Nguema, G., Viollier, E., Marakovic, G., et al. (2014). EXAFS analysis of iron cycling in mangrove sediments downstream a lateritized ultramafic watershed (Vavouto Bay, New Caledonia). Geochimica Cosmochimica Acta 136, 211–228. doi:10.1016/j.gca.2014.03.019
Noël, V., Morin, G., Juillot, F., Marchand, C., Brest, J., Bargar, J. R., et al. (2015). Ni cycling in mangrove sediments from New Caledonia. Geochimica Cosmochimica Acta 169, 82–98. doi:10.1016/j.gca.2015.07.024
O’Driscoll, M., Clinton, S., Jefferson, A., Manda, A., and McMillan, S. (2010). Urbanization effects on watershed hydrology and in-stream processes in the southern United States. Water 2, 605–648. doi:10.3390/w2030605
Panagos, P., Ballabio, C., Lugato, E., Jones, A., Borrelli, P., Scarpa, S., et al. (2018). Potential sources of anthropogenic copper inputs to European agricultural soils. Sustainability 10, 2380. doi:10.3390/su10072380
Pi, N., Tam, N. F. Y., and Wong, M. H. (2011). Formation of iron plaque on mangrove roots receiving wastewater and its role in immobilization of wastewater-borne pollutants. Mar. Pollut. Bull. 63, 402–411. doi:10.1016/j.marpolbul.2011.05.036
Prasad, M. B. K., Ramanathan, A. L., Shrivastav, S. Kr., and AnshumaliSaxena, R. (2006). Metal fractionation studies in surfacial and core sediments in the Achankovil River basin in India. Environ. Monit. Assess. 121, 77–102. doi:10.1007/s10661-005-9108-2
Purnobasuki, H., Purnama, P. R., and Kobayashi, K. (2017). Morphology of four root types and anatomy of root-root junction in relation gas pathway of Avicennia Marina (Forsk) Vierh roots. Vegetos- Inter. Jour. Plnt. Rese. 30, 100. doi:10.5958/2229-4473.2017.00143.4
Purnobasuki, H., and Suzuki, M. (2005). Functional anatomy of air conducting network on the pneumatophores of a mangrove plant, Avicennia marina (Forsk.) Vierh. Asian J. Plant Sci. 4, 334–347. doi:10.3923/ajps.2005.334.347
Randhir, T. (2003). Watershed-scale effects of urbanization on sediment export: Assessment and policy. Water Resour. Res. 39. doi:10.1029/2002WR001913
Rauret, G., López-Sánchez, J. F., Sahuquillo, A., Rubio, R., Davidson, C., Ure, A., et al. (1999). Improvement of the BCR three step sequential extraction procedure prior to the certification of new sediment and soil reference materials. J. Environ. Monit. 1, 57–61. doi:10.1039/a807854h
Ray, A. K., Tripathy, S. C., Patra, S., and Sarma, V. V. (2006). Assessment of Godavari estuarine mangrove ecosystem through trace metal studies. Environ. Int. 32, 219–223. doi:10.1016/j.envint.2005.08.014
Ray, R., Mandal, S. K., González, A. G., Pokrovsky, O. S., and Jana, T. K. (2021). Storage and recycling of major and trace element in mangroves. Sci. Total Environ. 780, 146379. doi:10.1016/j.scitotenv.2021.146379
Rezaei, M., Kafaei, R., Mahmoodi, M., Sanati, A. M., Vakilabadi, D. R., Arfaeinia, H., et al. (2021). Heavy metals concentration in mangrove tissues and associated sediments and seawater from the north coast of Persian Gulf, Iran: Ecological and health risk assessment. Environ. Nanotechnol. Monit. Manag. 15, 100456. doi:10.1016/j.enmm.2021.100456
Robin, S. L., Marchand, C., Ham, B., Pattier, F., Laporte-Magoni, C., and Serres, A. (2021). Influences of species and watersheds inputs on trace metal accumulation in mangrove roots. Sci. Total Environ. 787, 147438. doi:10.1016/j.scitotenv.2021.147438
Ruiz, J.-L., Ravary, F., and Debar, L. (2020). Suivi environnemental des milieux: ZAC PANDA et DSM-année 2019, suivie environnemental sur la ZAC PANDA et DSM. Dumbéa: SECAL.
Service de la Géologie de Nouvelle-Calédonie (2016). Carte de la géologie de la Nouvelle-Calédonie. [WWW Document]. URL. Available at: https://dtsi-sgt.maps.arcgis.com/apps/webappviewer/index. html?id=da224a6ff1c24c029de4024d7ae8af26 (accessed 12 12, 21).
Silva, C. A. R., Lacerda, L. D., Ovalle, A. R., and Rezende, C. E. (1998). The dynamics of heavy metals through litterfall and decomposition in a red mangrove forest. Mangroves Salt Marshes 2, 149–157. doi:10.1023/a:1009923223882
Singh, G., Ranjan, R. K., Chauhan, R., and Ramanathan, A. L. (2010). “Dissolved metal distribution in Indian mangrove ecosystem: Case studies from East Coast of India,” in Management and sustainable development of coastal zone environments. Editors A. L. Ramanathan, P. Bhattacharya, T. Dittmar, M. B. K. Prasad, and B. R. Neupane (Dordrecht: Springer Netherlands), 212–224. doi:10.1007/978-90-481-3068-9_14
Tardy, Y., and Roquin, C. (1992). “Geochemistry and evolution of lateritic landscapes,” in Weathering soils and paleosols (Amsterdam: Elsevier), 407–443.
Taylor, G. J., and Crowder, A. A. (1983). Use of the DCB technique for extraction of hydrous Iron oxides from roots of wetland plants. Am. J. Bot. 70, 1254–1257. doi:10.1002/j.1537-2197.1983.tb12474.x
Thakur, S., Singh, L., Wahid, Z. A., Siddiqui, M. F., Atnaw, S. M., and Din, M. F. M. (2016). Plant-driven removal of heavy metals from soil: Uptake, translocation, tolerance mechanism, challenges, and future perspectives. Environ. Monit. Assess. 188, 206. doi:10.1007/s10661-016-5211-9
Thakur, V. K., Thakur, M. K., Raghavan, P., and Kessler, M. R. (2014). Progress in green polymer composites from lignin for multifunctional applications: A review. ACS Sustain. Chem. Eng. 2, 1072–1092. doi:10.1021/sc500087z
Thanh-Nho, N., Marchand, C., Strady, E., Huu-Phat, N., and Nhu-Trang, T.-T. (2019). Bioaccumulation of some trace elements in tropical mangrove plants and snails (Can Gio, Vietnam). Environ. Pollut. 248, 635–645. doi:10.1016/j.envpol.2019.02.041
Thomas, N., Lucas, R., Bunting, P., Hardy, A., Rosenqvist, A., and Simard, M. (2017). Distribution and drivers of global mangrove forest change, 1996–2010. PLoS ONE 12, e0179302. doi:10.1371/journal.pone.0179302
Thornton, I. (2012). “Geochemical aspects of the distribution and forms of heavy metals in soils,” in Effect of heavy metal pollution on plants: Metals in the environment, pollution monitoring series (Springer Science & Business Media), 258.
Tremel-Schaub, A., and Feix, I. (2005). Contamination des sols : Transferts des sols vers les plantes. EDP Sciences.
Turekian, K. K., and Wedepohl, K. H. (1961). Distribution of the elements in some major units of the Earth’s crust. Geol. Soc. Am. Bull. 72, 175. doi:10.1130/0016-7606(1961)72[175:DOTEIS]2.0.CO;2
Valiela, I., Bowen, J. L., and York, J. K. (2001). Mangrove forests: One of the world’s threatened major tropical environments. BioScience 51, 807. doi:10.1641/0006-3568(2001)051[0807:mfootw]2.0.co;20807:MFOOTW]2.0.CO;2
Vracko, P., Tuomisto, J., Grad, J., and Kunseler, E. (2007). Exposure of children to chemical hazards in food. European Environment and Health Information System.
Yamaguchi, N., Ohkura, T., Takahashi, Y., Maejima, Y., and Arao, T. (2014). Arsenic distribution and speciation near rice roots influenced by Iron plaques and redox conditions of the soil matrix. Environ. Sci. Technol. 48, 1549–1556. doi:10.1021/es402739a
Keywords: mangrove forests, trace metals, urbanization, bioaccumulation, anthropogenic pressure, Avicennia marina, Rhizophora stylosa
Citation: Robin SL, Marchand C, Mathian M, Baudin F and Alfaro AC (2022) Distribution and bioaccumulation of trace metals in urban semi-arid mangrove ecosystems. Front. Environ. Sci. 10:1054554. doi: 10.3389/fenvs.2022.1054554
Received: 04 October 2022; Accepted: 20 October 2022;
Published: 09 November 2022.
Edited by:
Jun Zhou, University of Massachusetts Lowell, United StatesReviewed by:
Sadia Bibi, University of Agriculture, Faisalabad, PakistanM. Belal Hossain, Noakhali Science and Technology University, Bangladesh
Copyright © 2022 Robin, Marchand, Mathian, Baudin and Alfaro. This is an open-access article distributed under the terms of the Creative Commons Attribution License (CC BY). The use, distribution or reproduction in other forums is permitted, provided the original author(s) and the copyright owner(s) are credited and that the original publication in this journal is cited, in accordance with accepted academic practice. No use, distribution or reproduction is permitted which does not comply with these terms.
*Correspondence: Sarah Louise Robin, sarah.robin@unc.nc