Long-term adoption of bed planted conservation agriculture based maize/cotton-wheat system enhances soil organic carbon stabilization within aggregates in the indo-gangetic plains
- 1ICAR-Indian Agricultural Research Institute, New Delhi, India
- 2Indian Grassland and Fodder Research Institute, Jhansi, Uttar Pradesh, India
- 3ICAR-Indian Institute of Maize Research, New Delhi, India
- 4School of Public Health, University of California, Berkeley, CA, United States
- 5Department of Plant Production, College of Food and Agriculture Sciences, King Saud University, Riyadh, Saudi Arabia
Sustainability of contemporary crop establishment and management practices is questioned due to soil degradation, higher carbon emission and declining soil productivity. Hence, this study was conducted to address the impacts of conservation agriculture (CA) practices like permanent broad beds (PBB), permanent narrow beds (PNB) and zero tilled flat beds (ZT) with residue retention on soil organic carbon (SOC) protection within aggregates in the Indo-Gangetic Plains (IGP). Compared to conventionally tilled (CT) plots, the total SOC content was ∼27%–33% higher in the CA plots on equivalent mass basis. The soil physical properties, such as soil aggregation and mean weight diameter were considerably improved under the CA practices. The macroaggregates were ∼41, 37% and 27% higher in the PBB with residue (PBB + R), PNB with residue (PNB + R) and ZT with residue (ZT + R) plots (CA plots), respectively, than the CT plots in the surface soil (0–15 cm). The plots under PBB + R had ∼31% higher microaggregates within macroaggregates than the CT plots (24.4 g 100 g−1) soil. An increase in SOC content by ∼72, 55% and 69% was observed in the PBB + R, PNB + R and ZT + R plots over the CT plots in microaggregates within macroaggregates (3.02 Mg ha−1). However, plots under PBB + R, PNB + R and ZT + R had only ∼11, 3% and 23% more SOC within silt + clay fraction, respectively, than CT plots (5.85 Mg ha−1). Thus, SOC stabilization within microaggregates inside macroaggregates was the major mechanism, and not the chemical stabilization within silt + clay, of C sequestration under CA. As aggregate-associated carbon is an ecosystem property that strongly affects organic carbon stabilization, water holding capacity and resistance to erosion, growing maize/cotton–wheat system under PBB + R practice is a viable option for carbon sequestration in the IGP and similar agro-ecologies.
1 Introduction
Enhancing soil physical qualities, recycling nutrients, and building and stabilizing soil structure are all made possible by increasing soil organic carbon (SOC) in agro-ecosystems (Lehmann et al., 2017). In addition to supporting soil fertility by reducing soil erosion, regulating air permeability, infiltration, and nutrient cycling, soil aggregation is crucial for soil structure and the stabilization of organic matter (Zhang et al., 2017). Moreover, it physically inhibits the accessibility of organic molecules for microorganisms, enzymes, and oxygen, thus soil aggregate stability can protect SOC from mineralization. One of the largest environmental issues and challenges on a worldwide basis is carbon loss from arable lands (Farahani et al., 2022). Several soil aggregate fractions are reported to be essential for nutrient availability, preservation, and inherent C storage (Zhang et al., 2022). In light of this, understanding the dynamics of soil organic matter (SOM) and its physical stabilization within soil aggregates under different crop management practices, such as conservation agriculture (CA), is needed (Bhattacharyya et al., 2008; Wang et al., 2013).
It is heavily emphasized that the conventional agricultural practices, such as tillage, disturbs and reduces the fraction of macroaggregates, increasing soil C losses by runoff, production of greenhouse gases, loss in fertility and loss of diversity (Babu et al., 2020). It has been demonstrated that the two most prevalent conservation management strategies viz., long-term crop residue retention and zero tillage (ZT)-improve SOC accumulation throughout various agro-eco regions in the world (Blanco-Canqui and Lal, 2008; Chalise et al., 2019; Singh et al., 2020). Numerous studies have suggested that ZT is a good way to increase SOC storage due to little or no soil disturbance, which promotes soil aggregation and shields SOC from microbial decomposition, lowers residue decomposition in comparison to regular and intensive tillage (Corbeels et al., 2016; Francaviglia et al., 2017; Virto et al., 2012; Bhattacharyya et al., 2012a; Bhattacharyya et al., 2012b). However, the benefit of CA (ZT with residue retention) is only felt over long-term (Six et al., 2004; Bhattacharyya et al., 2012a).
The primary mechanism for long-term C sequestration in agricultural soils is occluded intraaggregate particulate organic matter (iPOM-C) in soil microaggregates (Six et al., 2004). Compared to microaggregate outer surfaces or macroaggregates as a whole, microaggregates-within-macroaggregates serve as comparatively stable and segregated habitat for microorganisms (Six et al., 2002a; Mummey and Stahl, 2004). Numerous studies have demonstrated that ZT raises particulate organic matter, also known as light fraction organic matter, an unstable and transient C pool (Fabrizzi et al., 2003; Liebig et al., 2004). According to many researchers, this pool of C serves as the nucleus for the development of aggregates and is essential to aggregate stability (Cambardella and Elliott, 1993; Rees et al., 2001). It is well evidenced that soil aggregates give extra variable capacity through additive physical protection that may be altered by soil management, but soil clay plus silt fraction controls the soils’ capacity to maintain SOC (Six et al., 2002a; Denef et al., 2004). Thus, understanding C stabilization within silt + clay fraction inside aggregates as affected by CA is addressed and focused in this study.
Long-term traditional rice cultivation in the Indo-Gangetic plains (IGP) region has been linked to severe depletion of natural resources, declining factor productivity, multiple nutrient deficiencies, drying up of the groundwater supply, labor shortages, and higher cultivation costs, raising concerns about the sustainability of agriculture (Kumar et al., 2021). Rice should be replaced with crops that require less water and are grown on light-textured soil with rainwater. In this situation, maize and cotton have shown promise as viable alternative to rice during the Kharif (rainy) season. Conventional tillage with one disc ploughing and 2 ploughings in the 0–30 cm soil layer before growing each crop is a common practice in the IGP under a non-rice based cropping system. Although many reports highlighted benefits of soil conservation practices like conservation tillage in wheat and direct seeded rice, the area under such conservation tillage is less than 3 Mha (Das et al., 2014). Hence, there is a need to increase the area under CA in the IGP.
By strengthening the physical protection of C, CA slows the process of SOM breakdown and raised beds enhance the C stabilization by better aggregate distribution. Information on the long-term effects of CA on soil aggregation and carbon pools of the IGP is available (Bhattacharyya et al., 2015; Parihar et al., 2016). However, there is a lack of information regarding the long-term effects of bed planting and residue retention (a novel CA practice for non-rice based cropping system of the IGP) on SOC stabilization within aggregates, specifically within microaggregates inside macroaggregates. This research was designed to highlight the novel issue of physical and chemical stabilization of SOC as affected by long-term CA in the IGP by measuring C protection within silt + clay fractions and within microaggregates inside macroaggregates. Hence, it was hypothesized that both bed planting and residue retention alone or in combination would positively affect the C stabilization within microaggregates inside macroaggregates after 10 years of maize/cotton-wheat system in the IGP. To address this hypothesis, this study was undertaken with two objectives: i) to assess the impacts of long-term bed planting and residue management alone or in combination (CA) on soil aggregation and aggregate-associated C and ii) to evaluate the effect of long-term raised bed planting with residue retention on SOC stabilization within aggregates, specifically within microaggregates inside macroaggregates under a maize/cotton wheat system.
2 Materials and methods
2.1 Site details
A conservation agriculture (CA) experiment was started in May 2010 on an alluvial sandy clay loam soil (fine loamy, illitic, Typic Haplustept) at the research farm of the ICAR-Indian Agricultural Research Institute (IARI), New Delhi, India, with maize (Zea mays)) and wheat (Triticum aestivum) as consecutive crops in a year. In 2017, maize was replaced by cotton (Gossypium hirsutum).
The climate in this region is semi-arid, with hot summer temperatures, strong winds, and irregular rainfall patterns. The soil at the experimental site’s surface (0–15 cm) had a pH of 7.7, Walkley-Black organic C of 5.2 g kg−1, KMnO4 oxidizable N of 182.3 kg ha−1, 0.5 M NaHCO3 extractable P of 23.3 kg ha−1, and 1 N NH4OAc extractable K of 250.5 kg ha−1. The 0–15 cm soil layer contained 49.6, 23.2, and 27.2 percent sand, silt, and clay, respectively. For the 15–30 cm layer, the equivalent values were 40.4, 24.5, and 35.1 percent sand, silt, and clay, respectively; for the 30–45 cm layer, those were 42.1, 26.5, and 31.4 percent sand, silt, and clay, respectively. As a result, the 0–15, 15–30, and 30–45 cm layers of soil had sandy clay loam, clay loam, and clay loam, respectively, as their soil texture.
2.2 Experiment details
The experiment was set up in three times replicated randomized block design (size: 10.0 m × 8.4 m). For the first 6 years, the cropping system was maize-wheat. It was then changed to cotton-wheat. Residue of the entire crop was completely removed in residue removal plot. Further details about the experiment can be seen in Das et al. (2014) for the cotton-wheat system and Das et al. (2018) for the maize-wheat system. Treatment details are provided in Table 1. A depiction of permanent bed is provided in the Figure 1.
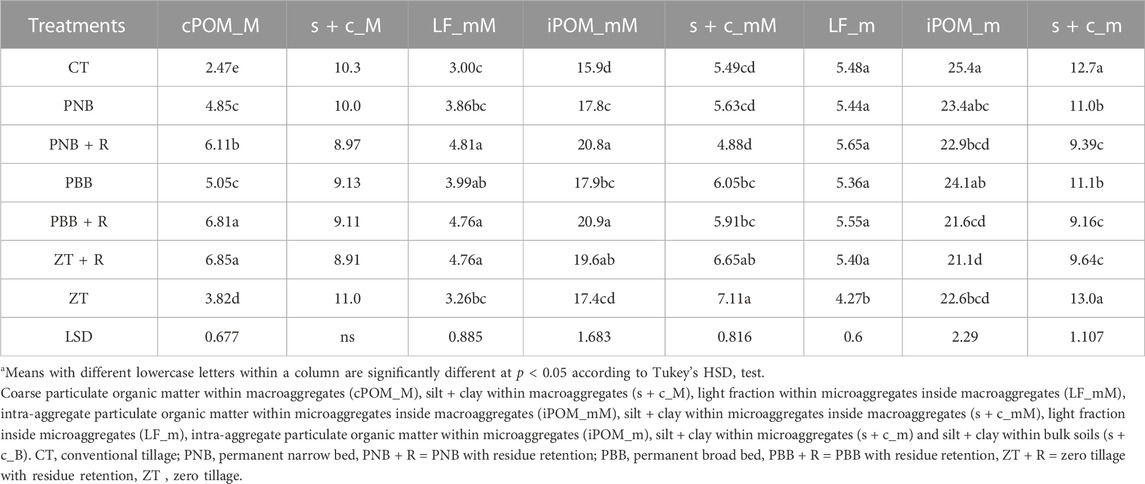
TABLE 1. Distribution (%) of density fractions of soil aggregates in the 0–15 cm layer as affected by bed planting and residue management under a maize/cotton-wheat system.
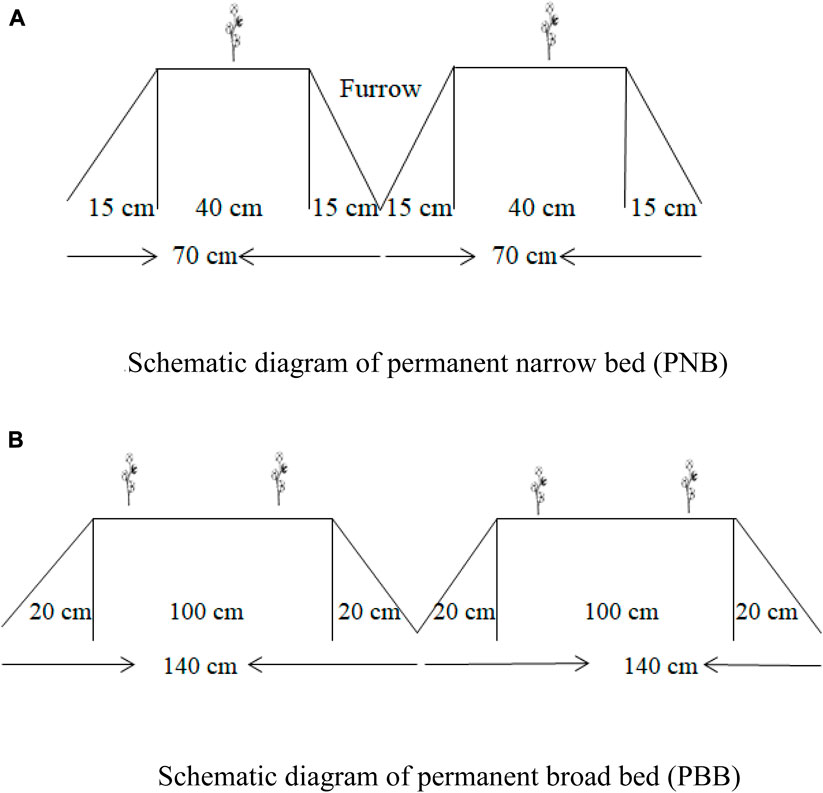
FIGURE 1. (A) Schematic diagram of permanent narrow bed (PNB). (B) Schematic diagram of permanent broad bed (PBB).
Bt cotton (cultivar MRC-7017) was manually sowed at the end of May at a spacing of 70 cm, and it was harvested in the second week of November each year. Cotton was given a common dosage of 150 kg N, 60 kg P2O5, and 40 kg K2O ha−1, of which P and K were treated as basal along with half of the N. The remaining N was added to the cotton crop in two equal applications at 30 and 60 days after sowing (DAS). In the ZT plots, glyphosate herbicide was treated at 1.0 kg ha−1 about 1 week before to the planting of both crops. At 2-3 DAS, 0.75 kg of pendimethalin was applied as a pre-emergence herbicide. Prior to this when the field was under maize-wheat cultivation, in both CT and ZT conditions, maize “HQPM 1”seeds were sowed at a spacing of 70 × 30 cm. The crop was cultivated throughout the wet season (July to October) and was harvested at the end of October each year. Both maize and wheat crops received the same application of 120 kg N, 60 kg P2O5, and 40 kg K2O ha−1. Using a turbo seeder/bed planter (in PNB, PBB, and ZT) and broadcasting (in CT), the full doses of P and K and half of the amount of N were applied as basal at the time of sowing to both crops. The rest of the dosage was top-dressed and special attention was paid to applying fertilizers along the crop rows and on the beds while leaving the furrows untouched.
2.3 Soil sampling and processing
After the cotton harvest in November 2020, triplicate soil samples were taken from soil layers at two different depths: 0–5 and 5–15 cm. Gravels and observable crop residue particles were removed during soil sampling and sample preparation. Each sample was a composite sample of seven locations for all plots. In the PNB plots, seven soil samples were collected from each replicate, 4 from beds and 3 from furrows, as the ratio of bed to furrow area was 4:3. Similarly, in the PBB plots, seven soil samples were collected from each replicate, 5 from beds and 2 from furrows, as the ratio of bed to furrow area was 5:2. The visible pieces of crop residues and gravels were removed during the time of soil sampling and at the time of sample preparation. The soil samples of 0–5 and 5–15 cm soil layers were sieved through an 8-mm sieve after air-drying and ground in a wooden pestle and mortar.
2.4 Soil bulk density
Soil bulk density (BD) was measured using a cylindrical core sampler of 5 cm internal diameter and 6 cm height (Veihmeyer and Hendrickson, 1948). The core sampler was driven into the undisturbed soil to desired depth and the soil was collected into the core. Then the edges of cores with soil samples were trimmed and kept in oven at 105°C till constant weight was achieved. Bulk density (Mg m−3) of the soil was determined as given below:
2.5 Soil aggregate separation
In order to separate soil samples’ aggregate sizes, Cambardella and Elliott’s. (1993) approach was used. In a nutshell, a 2 mm sieve was kept on top of a 100 g air-dried bulk soil sample that had been put through an 8 mm sieve. To accomplish aggregate separation, the sieving was carried out by moving the sieve up and down 3 cm in deionized water at a frequency of 25 times per minute. Four aggregate size fractions, including large macroaggregates (>2000 µm), small macroaggregates (250–2000 µm), microaggregates (53–250 µm), and silt + clay related particle (53 µm) were obtained using three sieves. At 40°C, all aggregate fractions were oven-dried to achieve consistent weight. The large and small macroaggregates were combined, since the proportions of large macroaggregates (>2 mm) recovered after the wet sieving were too small in weight. Mean weight diameter (MWD) of aggregates was estimated (Kemper and Rosenau, 1986) using the following formula:
Where, Wi, is the proportion of aggregates retained on each sieves in relation to the whole, Xi is the mean diameter of the sieve (mm).
2.6 Density fractionation
The large and small macroaggregates that were obtained through wet sieving were combined, and a 20 g sample of macroaggregates (M) was placed on a 250 µm sieve and wet sieved again. To obtain density fractions of soil aggregates, the sieving was carried out by moving the sieve up and down 3 cm in deionized water at a frequency of 30 times per minute. Coarse particulate organic matter (cPOM_M, >250 µm), microaggregates inside macroaggregates (mM; macro-aggregates passed through a 250 μm sieve and retained in a 53 μm sieve during aggregate separation, 53–250 µm), and silt + clay inside macroaggregates (s + c_M, <53 µm) were obtained.
Using modified technique of Sohi et al. (2001), the SOC of mM was separated into light fraction (LF_mM) and heavy fraction (HF) (intra-aggregate particulate organic matter within microaggregates inside macroaggregates (iPOM_mM) and silt + clay within microaggregates inside macroaggregates (s + c_mM)). Soil sample (5 g mM) was taken in 50 mL plastic centrifuge tubes and 1.85 g cm−3 solution of NaI was added to it in 1:5 ratio. The mixture was hand shaken for 30 s and centrifuged for 1 h at 4,000 rpm. Then, the tubes were taken out and the solution was decanted and filtered on a 47 mm diameter glass microfiber filter paper (1.6 μm retention) to separate the light fraction within microaggregate inside macroaggregates (LF_mM). The weight of the LF_mM was taken after drying the previously weighed filter paper in 40°C. The heavy fraction (HF) was washed (3-4 times) with distilled water to remove the excess NaI solution. Sodium hexa-metaphosphate (SHMP) (5%) was added to HF in 1:5 ratio and shaken for 18 h. The solution was then decanted in a 53 μm sieve to separate out the intra-particulate organic matter (iPOM_mM, >53 μm) and silt + clay fraction (s + c_mM, <53 μm) of microaggregates inside macroaggregates. Similarly, for free microaggregates (m), 5 g subsample was taken in a 50 mL plastic centrifuge tubes and 1.85 g cm−3 solution of NaI was added to it in 1:5 ratio and centrifuged for 1 h at 4,000 rpm. The fractions obtained from this free microaggregate were LF_m, and HF_m (iPOM_m and s + c_m). The latter was then separated into iPOM_m and s + c_m by 5% SHMP solution. The weights of all these fractions were taken separately after drying at 40°C. The schematic representation of the whole procedure is depicted in Figure 2.
cPOM_M-Coarse particulate organic matter within macroaggregates, s + c_M-silt + clay within macroaggregates, LF_mM-light fraction within microaggregates inside macroaggregates, iPOM_mM-intra-aggregate particulate organic matter within microaggregates inside macroaggregates, s + c_mM-silt + clay within microaggregates inside macroaggregates, LF_m-light fraction inside microaggregates, iPOM_m-intra-aggregate particulate organic matter within microaggregates and s + c_m-silt + clay within microaggregates.
2.7 Total soil organic carbon analysis
Using a total organic carbon (TOC) analyzer, the total soil C of all fractions was analyzed. The aggregate fractions were pulverized and passed through a 0.2 mm filter for this purpose. Soil organic carbon content was calculated using the following formula (Lal et al., 1998):
The SOC content of each fraction was estimated in a similar manner using the proportions of each fraction.
Because these techniques frequently result in considerable changes in soil BD, which modify the amount of soil present, it is frequently argued that the above total SOC calculation is insufficient when comparing the impacts of CT and CA. We designated the initial soil mass as the equivalent soil mass (ESM) and calculated soil C stocks on an ESM basis in order to account for the soil with greater BD overestimation of the overall SOC stock relative to the soil with lesser BD (Ellert and Bettany, 1995). The ESM calculated on the basis of initial BD was 2,360 Mg ha−1 for the 0–15 cm layer.
where, Msoil is the soil mass and ESM is the equivalent soil mass.
The SOC sequestration rate was computed as
2.8 Statistical analyses
In order to test for differences between the treatment means, the data collected from the study of the soil were prepared for an analysis of variance (ANOVA).using the Gomez and Gomez and Gomez. (1984). As a post hoc mean separation test, SAS 9.3 (SAS Institute, Cary, North Carolina, United States) was employed with Tukey’s honestly significant difference test (HSD; p < 0.05). The Tukey’s technique was applied in cases where the ANOVA showed a significant difference. Microsoft Office Excel 2013 was used to create all the figures.
3 Results
3.1 Total soil organic carbon content
The different treatments had no impact on soil bulk density values in the 0–15 cm soil layer (Supplementary Figure S1). The effect of long-term CA practices was evident on the total SOC content on an equivalent mass basis. The plots under PBB + R, PNB + R and ZT + R had ∼33, 27% and 29% higher total SOC stock than CT plots, respectively, in the 0–15 cm layer (Figure 3). The residue retained plots (PBB + R, PNB + R and ZT + R) had ∼15, 11% and 10% higher total SOC content on equivalent mass basis than the respective residue removed plots (Figure 3). Interestingly, adoption of ZT also increased the total SOC content by ∼17% over the farmers’ practice (CT). Total SOC sequestration in the plots under PBB + R over CT plots was 0.42 Mg ha−1 year−1 in the 0–15 cm layer after 10 years.
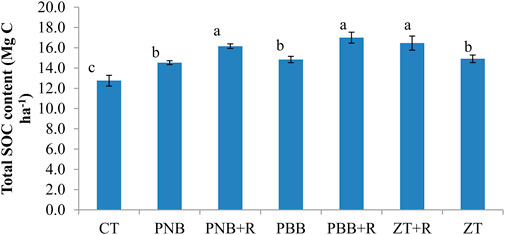
FIGURE 3. Total soil organic carbon (SOC) content (Mg C ha−1) of bulk soil in the 0–15 cm layer as affected by bed planting and residue management under a maize/cotton-wheat system CT = conventional tillage, PNB = permanent narrow bed, PNB + R = PNB with residue retention, PBB = permanent broad bed, PBB + R = PBB with residue retention, ZT + R = zero tillage with residue retention, ZT = zero tillage. Bars with different lowercase letters are significantly different according to Tukey’s HSD test at p < 0.05. The error bars are the standard deviation of the mean.
3.2 Soil aggregate distribution and mean weight diameter
As there was no huge variations in all parameters for 0–5 and 5–15 cm soil layers as affected by different CA treatments, we presented whole data for 0–15 cm soil layer (combined all results of 0–5 and 5–15 cm layers). Retention of residues enhanced the percentage of macroaggregates in the PBB + R, PNB + R, and ZT + R plots over the CT plots by ∼41, 37% and 27%, respectively (Figure 4). Due to residue retention, the proportion of macroaggregates increased by ∼21, 15, and 11% under PBB + R, PNB + R and ZT over the PBB, PNB, and ZT plots, respectively (Figure 4). The adoption of raised beds with residue retention (PBB + R and PNB + R) had ∼12% and 8% more macroaggregates in the 0–15 cm layer than ZT + R plots. Also, the adoption of long-term ZT alone improved the macroaggregate proportion compared to the CT plots by ∼14% (Figure 4). Residue retained plots had lesser microaggregates than the residue removal plots (Figure 4). Also, tillage had profound influence on microaggregate proportion as the plots under CT had ∼9% more microaggregates than ZT. Similarly the silt plus clay content in soil was significantly affected by long-term CA practice, where the higher proportion was observed in the CT plots (Figure 4).
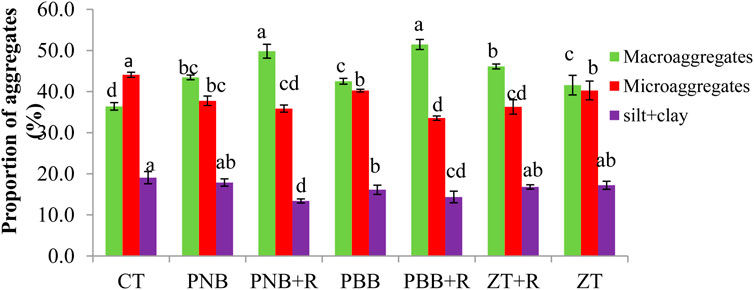
FIGURE 4. Distribution (%) of macroaggregates, microaggregates and silt + clay of bulk soil in the 0–15 cm layer as affected by long-term conservation agriculture in a maize/cotton-wheat cropping system. CT = conventional tillage, PNB = permanent narrow bed, PNB + R = PNB with residue retention, PBB = permanent broad bed, PBB + R = PBB with residue retention, ZT + R = zero tillage with residue retention, ZT = zero tillage. Bars with different lowercase letters are significantly different according to Tukey’s HSD test at p < 0.05. The error bars are the standard deviation of the mean.
The results revealed that the tillage and residue retention had major impacts on mean weight diameter (MWD) in the 0–15 cm of soil depth. An increase in MWD by ∼50, 33% and 28% was observed in the PBB + R, PNB + R and ZT + R plots over the CT plots, respectively (Figure 5). Also, plots under PBB + R, PNB + R and ZT + R plots had ∼26, 15% and 19% higher MWD than the PBB, PNB and ZT plots, respectively (Figure 5).
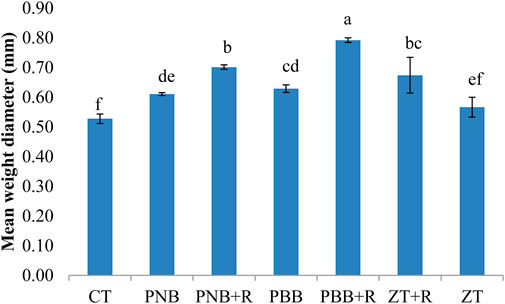
FIGURE 5. Mean weight diameter (MWD) soil in the 0–15 cm layer as affected by long-term conservation agriculture in a maize/cotton-wheat cropping system. CT = conventional tillage, PNB = permanent narrow bed, PNB + R = PNB with residue retention, PBB = permanent broad bed, PBB + R = PBB with residue retention, ZT + R = zero tillage with residue retention, ZT = zero tillage. Bars with different lowercase letters are significantly different according to Tukey’s HSD test at p < 0.05. The error bars are the standard deviation of the mean.
3.3 Distribution of total soil organic C in aggregates
Regardless of crop residue retention in the surface layer (0–15 cm), the ZT plots had higher SOC concentration in the macroaggregates than CT after 10 years of CA practices. Maximum SOC concentration was found in the bed planted plots with residue treatments, while minimal SOC concentration was observed in the CT plots. In comparison to the CT plots, the increase in SOC concentration in the macroaggregates were ∼40, 33, and 37% in the PBB + R, PNB + R and ZT + R plots, respectively (Figure 6). Also, the plots under residue retention had ∼15, 12% and 17% more C concentrations in the macroaggregates than PBB, PNB and ZT plots (Figure 6). The adoption of ZT practice alone had also considerably improved the SOC concentration in the macroaggregates.
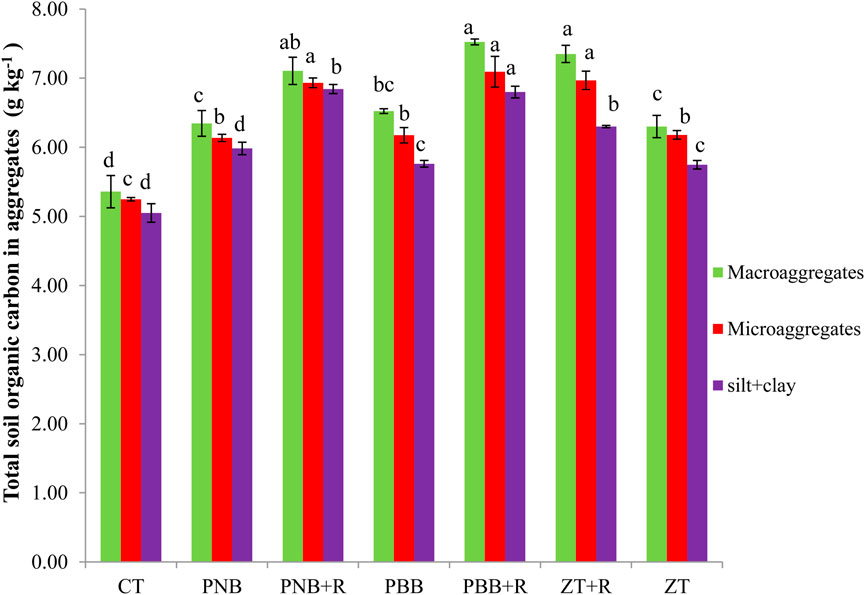
FIGURE 6. Total soil organic carbon (TSOC) concentration (g kg−1) within macroaggregates, microaggregates and silt + clay in the 0–15 cm layer as affected by long-term conservation agriculture in a maize/cotton-wheat cropping system. CT = conventional tillage, PNB = permanent narrow bed, PNB + R = PNB with residue retention, PBB = permanent broad bed, PBB + R = PBB with residue retention, ZT + R = zero tillage with residue retention, ZT = zero tillage. Bars with different lowercase letters are significantly different according to Tukey’s HSD test at p < 0.05. The error bars are the standard deviation of the mean.
Zero tillage had influence on SOC concentration, but long-term residue retention considerably increased the C concentrations in the microaggregates. In PBB + R and ZT + R plots, the SOC concentrations inside microaggregates were comparable, but were much greater than CT plots (Figure 6). Also, the residue added plots had ∼15, 13% and 13% more SOC concentrations than the respective residue removal plots and the ZT plot had ∼18% more SOC in the microaggregates than the CT plots. The SOC concentrations within silt plus clay in both soil layers were significantly impacted by the tillage and residue management. The PBB + R plots had 35% higher silt + clay associated-C concentration than that of CT plots. Significant changes in silt + clay associated-C concentration between residue retained and residue removal plots were observed (Figure 6).
3.4 Distribution of density fractions
The overall impact of tillage and residue management had a significant influence on the coarse particulate organic matter within macroaggregates (cPOM_M) proportion. On comparing to the CA plots (PBB + R, PNB + R, and ZT + R), CT plots had ∼64, 59% and 64% lesser cPOM_M fraction, respectively (Table 1). Tillage operation had noticeable impact on the light fraction inside microaggregates within macroaggregates (LF_mM). Among the residue removed plots, the raised bed (PBB and PNB) plots had higher concentration of LF_mM than ZT (flat bed plots) (Table 1). The long-term adoption of CA had significant effect on intra-aggregate particulate organic matter within microaggregates inside macroaggregates (iPOM_mM) fraction in the 0–15 cm soil depth (Table 1). The quantities of the iPOM_mM fraction in the plots under PBB + R, PNB + R and ZT + R were comparable and were ∼31, 31% and 23% higher than CT plots, respectively (Table 1). The residue removal plots had similar quantities of iPOM_mM fraction and ZT plots had ∼9% higher iPOM_mM fraction than CT plots. Interestingly, ZT plots had ∼30% higher proportion of silt plus clay within microaggregates inside macroaggregates (s + c_mM) in the 0–15 cm soil layer than CT plots (Table 1) and the ZT plots had ∼18% and 26% more s + c_mM fractions than PBB and PNB plots in the surface layer (Table 1).
The tillage management had no effect on the light fraction inside microaggregates (LF_m) fraction (Table 1). The plots under PNB + R had the highest proportion of LF_m and the value was ∼32% higher than ZT plots. The intra-aggregate particulate organic matter inside microaggregates (iPOM_m) fraction was higher by ∼12% in the CT than the ZT plots in the 0–15 cm soil layer (Table 1). The CT and ZT plots had significantly higher amount of silt plus clay within microaggregates (s + c_m) fraction in the 0–15 cm soil layer (Table 1).
3.5 SOC concentration within different density fractions
The SOC concentration within cPOM_M in the 0–15 cm soil layer was influenced by tillage operation. The adoption of ZT increased the C concentration of cPOM_M by ∼18% compared to farmers’ practice (CT plots) (Table 2). The CA plots (PBB + R, PNB + R and ZT + R) had similar C concentrations within cPOM_M. The cPOM_M concentration of PBB + R plots was ∼23% higher than PBB, of PNB + R plots was 32% higher than PNB and of ZT + R plots was 26% higher than ZT plots (Table 2). The ZT + R plots had higher s + c_M associated C in the 0–15 cm soil depth than all other treatments (Table 2). Residue retention increased the s + c_M associated C by ∼20, 9% and 29% than the respective residue removal plots (PBB + R versus PBB, PNB + R versus PNB and ZT + R versus ZT) (Table 2). Tillage management had no noticeable effect on the LF_mM associated C concentration on the surface soil, but residue management increased LF_mM associated C concentration. The PBB + R plots had highest LF_mM associated C concentration and were ∼9% higher than PNB + R plots (Table 2). The PBB + R, PNB + R and ZT + R plots had ∼19, 9% and 11% higher C concentrations in the LF_mM fraction than the corresponding residue removal plots. Long-term adoption of PBB + R increased the iPOM_mM associated C by ∼37, 28% and 40% compared to the CT treatment in the surface layer (Table 2).
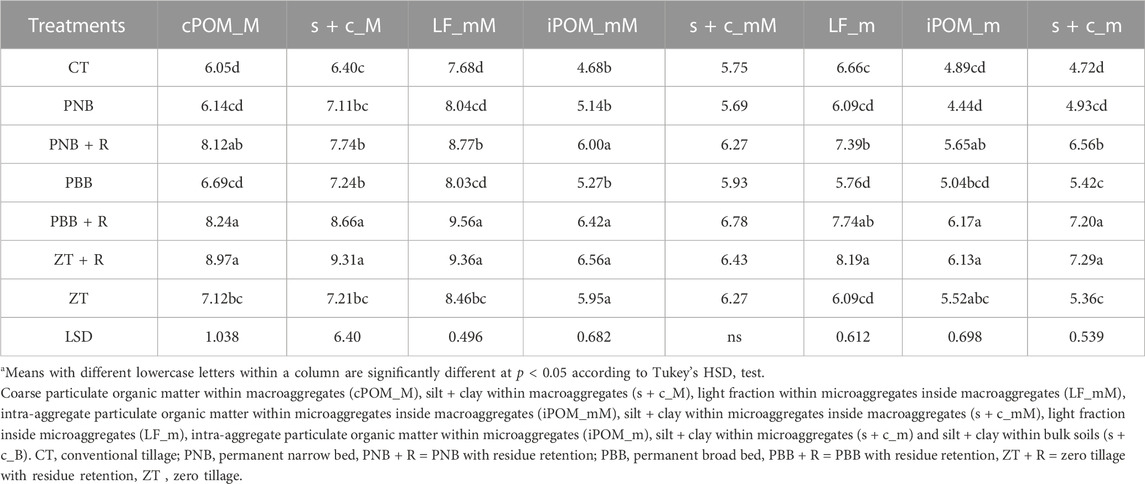
TABLE 2. Distribution (g kg−1) of SOC in density fractions of soil aggregates in the 0–15 cm layer as affected by bed planting and residue management under a maize/cotton-wheat system.
The plots under PBB + R, PNB + R and ZT + R had higher microaggregate-associated C concentrations than PBB, PNB and ZT plots, respectively (Table 2). The CA plots had ∼16, 11% and 23% higher LF_m associated C than CT plots. Also, these plots had ∼34, 21% and 34% higher C inside LF_m than the respective residue removal plots (Table 2). Similarly, PBB + R treatment had ∼26% and 53% higher C concentrations within iPOM_m and s + c _m than the CT plots (Table 2). The residue retention (PBB + R, PNB + R and ZT + R) significantly improved the C inside iPOM_m fraction by ∼22, 27% and 11% compared to the corresponding residue removal plots (Table 2). The s + c_m associated SOC concentrations were significantly higher in the CA plots than CT and the residue removal plots (Table 2).
3.6 SOC content within different density fractions
The long-term adoption of CA practices had a positive impact on total SOC content within aggregates. In the surface soil (0–15 cm), the CT plots had ∼74, 70% and 76% lesser cPOM_M associated C than PBB + R, PNB + R and ZT + R plots, respectively (Table 3). In case of C content of microaggregates within macroaggregates (mM), CA practices had a significant impact on improving it. The C content of LF_mM was increased by ∼101 and 97% in PBB + R and ZT + R and plots compared to farmers’ practice (CT). Also residue retained plots (ZT + R, PBB + R and PNB + R plots) had ∼43, 39% and 63% more SOC within in the LF_mM fraction compared with the respective residue removal plots (Table 3). The SOC content within iPOM_mM was ∼83, 70% and 75% higher in CA than the CT plots. Also residue retention significantly improved the SOC content and was ∼43, 40% and 24% higher than residue removal plots under ZT within iPOM_mM fraction (Table 3). The s + c_mM associated C content was ∼42% higher under ZT than CT plots (Table 3).
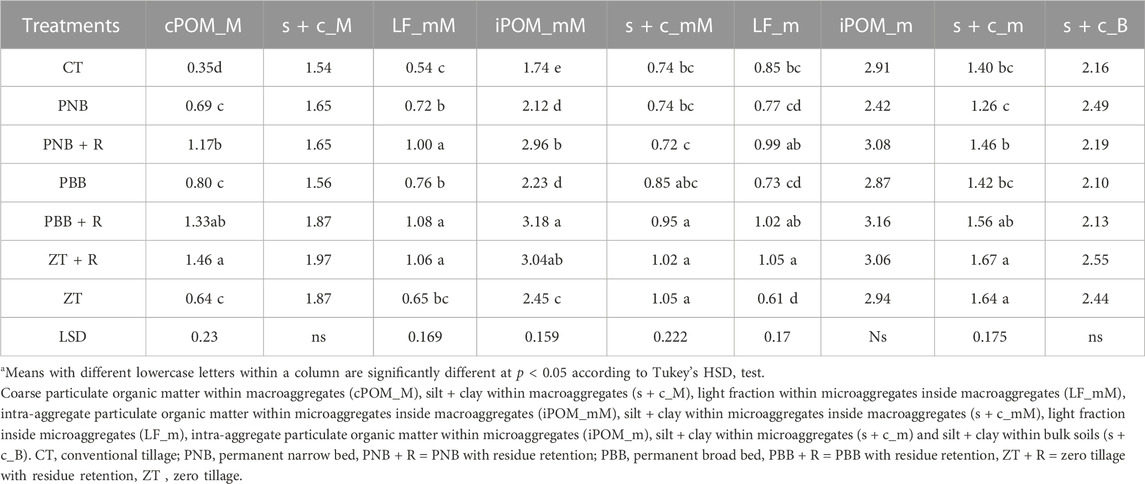
TABLE 3. Total SOC content (Mg C ha-1) in density fractions of soil aggregates in the 0–15 cm layer as affected by bed planting and residue management under a maize/cotton-wheat system.
The ZT + R, PBB + R and PNB + R treatments had ∼71, 40% and 29% more LF_m associated C contents in 0–15 cm soil layer than ZT, PBB and PNB plots, respectively (Table 3). But the effect was not significant for the iPOM_m associated C content (Table 3). Similarly tillage alone had no significant impact on SOC content in the s + c_m fraction. But, the PBB + R and PNB + R plots had ∼10 and 16% higher SOC contents than PBB and PNB plots, respectively (Table 3).
3.7 Soil organic carbon stabilization under silt + clay fraction and under microaggregate within macroaggregates
The long-term adoption of CA practices had a significant impact on total SOC content of microaggregate within macroaggregates. An increase in SOC stabilization by ∼72, 55% and 69% was observed in the PBB + R, PNB + R and ZT + R plots over the CT plots, respectively (Figure 7). Also, PBB + R, PNB + R and ZT + R plots had ∼36, 31% and 23% higher SOC stabilization than PBB, PNB and ZT plots respectively (Figure 7). Interestingly, SOC stabilization was ∼27, 19% and 38% higher in the PBB, PNB and ZT plots respectively when compared to the CT plots. The combined SOC content within the silt + clay fractions of the soil was significantly higher in the ZT + R plots among all the treatments (Figure 7). SOC stabilization was ∼23% and 20% higher under the ZT + R and PBB + R plots than CT plots (Figure 7).
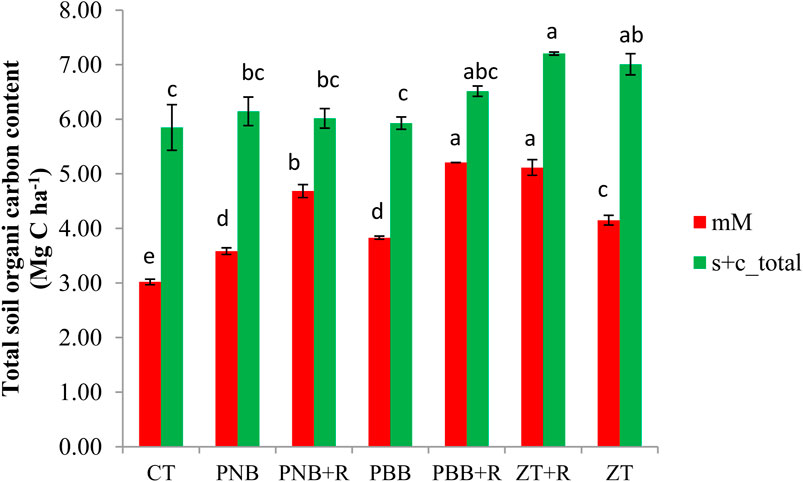
FIGURE 7. Total SOC content (Mg C ha−1) in microaggregate within macroaggregates (mM) and silt + clay fractions in the 0–15 cm layer as affected by bed planting and residue management under a maize/cotton-wheat system *Means with different lowercase letters within a column are significantly different at p < 0.05 according to Tukey’s HSD test. CT = conventional tillage, PNB = permanent narrow bed, PNB + R = PNB with residue retention, PBB = permanent broad bed, PBB + R = PBB with residue retention, ZT + R = zero tillage with residue retention, ZT = zero tillage.
4 Discussion
4.1 Total soil organic carbon content
Ten years of CA under a maize/cotton-wheat system significantly increased total SOC concentration and content on equivalent mass basis in the surface layer. By accelerating organic matter oxidation, soil tilling decreases carbon content and speeds up organic matter breakdown. Also, the crop roots stay undisturbed in the root zone as a result of CA, which may help increase the accumulation of organic carbon in root zones through the decomposition of the crop roots under the CA plots (Parihar et al., 2016). Additionally, the microclimate brought about by residue retention favors greater aggregate stability in ZT, PBB and PNB plots than CT, which also lessens the susceptibility to erosion, and this may possibly increase the rates of C sequestration in CA plots (Lal, 2015).
4.2 Soil aggregation and aggregate-associated SOC
Numerous soil particles are bound together to form secondary units during soil aggregation (Six et al., 2004). Ten years after adoption of different treatments, permanent raised beds with full residue retention were found to have more macroaggregates than traditionally tilled soils, but the conventional method had significantly more free microaggregates. Higher proportion of macroaggregates was found in CA plots than CT as macroaggregates are physically disrupted by intensive tillage methods under CT (Bhattacharyya et al., 2012b). Contrarily, the residues retained on the surface under CA plots protect the soil from raindrop impact (Six et al., 2000; Zotarelli et al., 2007). No-tillage encourages fungal growths, which aid in the production and stabilization of macroaggregates (Modak et al., 2019; Modak et al., 2020). The binding effect of root exudates and the tangling of root networks constitute the role of vegetation in sustaining soil structure (Six et al., 2004; Xiao et al., 2020). Also the results were in line with the findings of Gupta Choudhury et al. (2014) and Modak et al. (2020) who observed an increase in the quantity of macroaggregates with residue retention. By boosting microbial activity, fresh residue serves as a nucleation site for the production of new aggregates. Apart from this, the added residues upon degradation release polysaccharides, organic acids, glomalin etc. that considerably increase the aggregate proportion and stability in soil (Singh et al., 2018). The proportion of silt + clay was comparatively higher in CT plots in both soil layers and can be due to the increased tillage intensity which caused the breakdown of macroaggregates into smaller fractions and component primary particles (silt- and clay-sized fractions), as stated by Six et al. (2000) and Grandy and Robertson (2007). The more aggregate fraction in the raised bed plots than that of flat-bed plots indicates a good sign of soil binding and protection under the raised bed system. Higher MWD values under CA plots may be attributed to the higher total SOC content of the treatments. The percentage of large macroaggregates is the most crucial component to consider when assessing how management approaches affect soil aggregation, since it has a significant impact on the MWD. The results revealed that raised broad bed plots had higher macroaggregates and thus higher MWD than CT plots and the trend was in line with the previous findings (Litcher et al., 2008; Parihar et al., 2016).
Regardless of tillage practices, residue retained plots had greater total SOC concentration than CT plots. The C concentration in macroaggregates (>0.25 mm) was greater than that in microaggregates (0.25–0.053 mm), which is consistent with the hypothesis of aggregate hierarchy, according to which macroaggregates are formed from microaggregates by cementing polysaccharides generated from microorganisms and plants (Six et al., 2004). Higher macroaggregate-associated SOC concentration may be attributed to slower macroaggregate turnover rates, physical soil protection by mulching with crop residues and ZT (Bhattacharyya et al., 2012a; Modak et al., 2020). Aggregates can physically prevent SOC from decomposing by encasing it in narrower pores and thus can stabilize SOC (Six et al., 2002b). Also, ZT lessens soil disturbance and mixing, allowing SOM to accumulate (Lützow et al., 2002). The surface layers under no-tillage have considerably more microaggregate- and macroaggregate-protected C than under conventional tillage and the findings were similar to others (Liebig et al., 2004; Pinheiro et al., 2004).
4.3 Distribution of density fractions present in the soil
The addition of crop residue and below ground biomass often resulted in an increased cPOM_M fractions, as seen by the higher proportion of cPOM_M in the residue-retained plots. The cPOM_M is reportedly vulnerable to microbial attack, and as it degrades, it releases a sticky, cement-like polysaccharide that aids in the production of microaggregates inside of macroaggregates (Six et al., 1999). As a result, the percentage of microaggregates within macroaggregates (mM) fraction increased. Increased SOM under no-tillage is partially determined by the relationship between macroaggregate turnover, microaggregate formation, and C stability within microaggregates (Six et al., 2000).
The volume, stability, and turnover of the microaggregates within macroaggregates can be used to explain differences in C concentrations within the fraction of microaggregates occluded in the macroaggregates under different management systems (Denef et al., 2007). The LF is the most labile form of C and is made up of root biomass pieces that have partially decomposed (Six et al., 2000). Although the light fraction is primarily composed of plant leftovers, it also contains significant amounts of microbial and micro faunal waste, such as fungal hyphae and spores (Modak et al., 2020). The PBB + R, ZT + R and PNB + R plots had greater iPOM_mM fractions on average across the treatments, which may be attributable to better protection of these fractions within microaggregates concealed by macroaggregates and this fraction is important with respect to physical stabilization of C (Six et al., 2001). The protective environment within the macroaggregates causes the turnover of the macroaggregate-occluded-microaggregates to be slower in permanent elevated beds. Its slower turnover enables better mineral-bound C breakdown product stabilization and cPOM protection in the macroaggregate-occluded-microaggregates (Denef et al., 2007). The enhanced stability of microaggregates is likely owing to silt and clay (Bandyopadhyay et al., 2010), whereas residue-derived C significantly influenced macroaggregation in all the residue-added plots.
Tillage showed little effect on LF_m similar to LF_mM. However residue retention had significant effect on this fraction. Whether they are free or occluded within microaggregates, the iPOM m and s + c m fractions are regarded as the principal building blocks of microaggregates (Six et al., 1999). But the proportion of iPOM_m and s + c_m was not influenced by CA practices and may be due to soil texture (sandy loam) or it might take more years to show a significant effect (Modak et al., 2020).
4.4 SOC content within the density fractions
Retaining crop residues in ZT plots increased SOC content and this was in conformity with the results of Samson et al. (2020a) and Modak et al. (2020), who found that crop residue retention enhanced SOC content in the bulk soils and in macroaggregate pool. It is anticipated that increased macroaggregate associated C under CA will boost plant-derived carbohydrates, which aid in soil macroaggregation (Samson et al., 2020b). The SOC contents of LF_mM and LF_m fractions were higher in residue retained plots than CT or residue removed plots as this particular fraction is highly influenced by management practices. A sensitive indication of the effects of farming methods on soil organic matter may be found in the light fraction carbon (LFC), which is primarily made up of partially degraded organic residues (Six et al., 2002a). In contrast to other C fractions, light fraction carbon is linked to non-aggregated soil, is not easily lost during any ploughing operations, and accumulates more quickly in microbial biomass (David et al., 2006). The SOC content of iPOM_mM and iPOM_m were higher in our study under CA plots than CT plots and is in line with the findings of Six et al. (1999) where the ZT had a much greater C concentration in iPOM fractions than the CT. This was because there was less POM-C degradation, less tillage disturbance, less raindrop effect, and more microaggregates inside macroaggregates under ZT than CT.
4.5 Soil organic carbon stabilization under silt + clay fraction and under microaggregate within macroaggregates
A significant proportion of total SOC content in the density fractions was seen in silt + clay fraction which is positively correlated to stability of SOC in soils (Trigalet et al., 2014). These findings imply that during ZT and crop residue retention, the accumulation of organic C in silt + clay may help to stabilize the more labile organic C in soil, which may encourage soil macroaggregation and organic C content. Higher carbon input through residues as well as increased silt and clay specific surface area acting as sorption sites through cation bridges and ligand exchange could be the causes of the higher C associated with the “silt + clay” sized fraction in soil under CA-based management systems (Bandyopadhyay et al., 2010; Gupta Choudhury et al., 2014). As a result, the slow carbon pool is connected to SOC associated with clay and silt particles via a process of stabilizing SOC against microbial mineralization. Also the higher content among the CA plots was seen in the ZT + R plots although the total SOC content was higher in the PBB + R plots can be due to the annual disturbances caused to the beds during periodical reshaping that led to more SOC oxidation and loss to the atmosphere (Dey et al., 2020).
5 Conclusion
Total SOC sequestration in the plots under PBB + R over CT plots was 0.42 Mg ha−1 year−1 in the 0–15 cm layer after 10 years. Thus, our findings support the idea that agricultural residue retention during planting on zero tilled permanent beds (PBB) is a robust alternative to the conventional practice for maintaining SOC levels particularly under tropical conditions in Inceptisols. CA practices had improved the aggregate stability and retention of residues enhanced the percentage of macroaggregates in the PBB + R, PNB + R, and ZT + R plots over the CT plots by ∼41, 37% and 27%, respectively. This study computed SOC stabilization within physical and chemical fractions. The PBB + R plots had ∼72% and 36% more SOC stabilization inside microaggregates within macroaggregates (physical stabilization) than CT and PBB plots. Thus, residue retention is a crucial factor for physical protection of SOC. The chemical stabilization of SOC within silt + clay was lesser affected by CA than the physical protection. The difference in SOC stabilization inside microaggregates within macroaggregates (mM) under PBB + R and CT plots was 2.19 Mg ha−1 against only 0.67 Mg ha−1 for the same difference of C stabilization within silt + clay fractions. This study explicitly demonstrated that under long-term CA, the physical stabilization of SOC inside mM was the major mechanism of C sequestration in an Inceptisol of the IGP. An increase in SOC stabilization within mM and silt + clay fractions as a result of CA indicated that these soils are not C saturated. Thus PBB + R can indeed be a viable option for maize/cotton-wheat cultivation in the IGP without any impairment to the environment and enhancing carbon levels in the soil. Future studies should investigate whether PBB + R raises subsurface SOC in the IGP and similar agro-ecosystems.
Data availability statement
The original contributions presented in the study are included in the article/Supplementary Material, further inquiries can be directed to the corresponding author.
Author contributions
AJ and RB designed the research and performed analysis, data collection and wrote first draft; TD maintained the experimental field, DB, AG, KB, AD, SN, SJ, PR, AB, RC, and HE reviewed, edited approved the draft of manuscript. All authors contributed to the article and approved the submitted version.
Funding
Researchers Supporting Project number (RSP2023R118), King Saud University.
Acknowledgments
The first author acknowledges Director, ICAR-IARI for his kind support to conduct the study. The financial support by the Indian Council of Agricultural Research is duly acknowledged. The authors would like to thank Researchers Supporting Project number (RSP2023R118), King Saud University, Riyadh, Saudi Arabia.
Conflict of interest
The authors declare that the research was conducted in the absence of any commercial or financial relationships that could be construed as a potential conflict of interest.
Publisher’s note
All claims expressed in this article are solely those of the authors and do not necessarily represent those of their affiliated organizations, or those of the publisher, the editors and the reviewers. Any product that may be evaluated in this article, or claim that may be made by its manufacturer, is not guaranteed or endorsed by the publisher.
Supplementary material
The Supplementary Material for this article can be found online at: https://www.frontiersin.org/articles/10.3389/fenvs.2023.1216242/full#supplementary-material
References
Babu, S., Singh, R., Avasthe, R. K., Yadav, G. S., Mohapatra, K. P., Selvan, T., et al. (2020). Soil carbon dynamics in Indian Himalayan intensified organic rice-based cropping sequences. Ecol. Indic. 114, 106292. doi:10.1016/j.ecolind.2020.106292
Bandyopadhyay, P. K., Saha, S., Mani, P. K., and Mandal, B. (2010). Effect of organic inputs on aggregate associated organic carbon concentration under long-term rice–wheat cropping system. Geoderma 154, 379–386. doi:10.1016/j.geoderma.2009.11.011
Bhattacharya, R., Das, T. K., Sudhishri, S., Sharma, A. R., Dudwal, B., Bhatia, A., et al. (2015). Conservation agriculture effects on soil organic carbon accumulation and crop productivity under a rice–wheat cropping system in the western Indo-Gangetic Plains. Eur. J. Agron. 70, 11–21. doi:10.1016/j.eja.2015.06.006
Bhattacharyya, R., Kundu, S., Pandey, S. C., Singh, K. P., and Gupta, H. S. (2008). Tillage and irrigation effects on crop yields and soil properties under the rice–wheat system in the Indian Himalayas. Agric. Water Manage. 95, 993–1002. doi:10.1016/j.agwat.2008.03.007
Bhattacharyya, R., Tuti, M. D., Bisht, J. K., Bhatt, J. C., and Gupta, H. S. (2012b). Conservation tillage and fertilization impact on soil aggregation and carbon pools in the Indian himalayas under an irrigated rice-wheat rotation. Soil Sci. 177, 218–228. doi:10.1097/ss.0b013e3182408f1e
Bhattacharyya, R., Tuti, M. D., Kundu, S., Bisht, J. K., and Bhatt, J. C. (2012a). Conservation tillage impacts on soil aggregation and carbon pools in a sandy clay loam soil of the Indian Himalayas. Soil Sci. Soc. Am. J. 76, 617–627. doi:10.2136/sssaj2011.0320
Blanco-Canqui, H., and Lal, R. (2008). No-Tillage and soil-profile carbon sequestration: An on-farm assessment. Soil Sci. Soc. Am. J. 72, 693–701. doi:10.2136/sssaj2007.0233
Cambardella, C. A., and Elliott, E. T. (1993). Carbon and nitrogen distribution in aggregates from cultivated and native grassland soils. Soil Sci. Soc. Am. J. 57, 1071–1076. doi:10.2136/sssaj1993.03615995005700040032x
Chalise, K. S., Singh, S., Wegner, B. R., Kumar, S., P´erez-Guti´errez, J. D., Osborne, S. L., et al. (2019). Cover crops and returning residue impact on soil organic carbon, bulk density, penetration resistance, water retention, infiltration, and soybean yield. Agron. J. 111, 99–108. doi:10.2134/agronj2018.03.0213
Corbeels, M., Marchao, R. L., Neto, M. S., Ferreira, E. G., Madari, B. E., Scopel, E., et al. (2016). Evidence of limited carbon sequestration in soils under no-tillage systems in the Cerrado of Brazil. Sci. Rep. 6, 21450. doi:10.1038/srep21450
Das, T. K., Bhattacharya, R., Sudhishri, S., Sharma, A. R., Saharawat, Y. S., Bandyopadhyay, K. K., et al. (2014). Conservation agriculture in an irrigated cotton-wheat system of the western indo-gangetic plains: Crop and water productivity and economic profitability. Field Crops Res. 158, 24–33. doi:10.1016/j.fcr.2013.12.017
Das, T. K., Saharawat, Y. S., Bhattacharyya, R., Sudhishri, S., Bandyopadhyay, K. K., Sharma, A. R., et al. (2018). Conservation agriculture effects on crop and water productivity, profitability and soil organic carbon accumulation under a maize-wheat cropping system in the North-western Indo-Gangetic Plains. Field Crops Res. 215, 222–231. doi:10.1016/j.fcr.2017.10.021
David, S., Yusmary, E., and Rafael, R. (2006). Short-term tillage practices on soil organic matter pools in a tropical Ultisol. Aust. J. Soil Res. 44, 687–693.
Denef, K., Six, J., Merckx, R., and Paustian, K. (2004). Carbon sequestration in microaggregates of no-tillage soils with different clay mineralogy. Soil Sci. Soc. Am. J. 68, 1935–1944. doi:10.2136/sssaj2004.1935
Denef, K., Zotarellia, L., Boddey, R. M., and Six, J. (2007). Microaggregate associated carbon as a diagnostic fraction for management induced changes in soil organic carbon in two Oxisols. Soil Biol. Biochem. 39, 1165–1172. doi:10.1016/j.soilbio.2006.12.024
Dey, A., Dwivedi, B. S., Bhattacharyya, R., Datta, S. P., Meena, M. C., Jat, R. K., et al. (2020). Effect of conservation agriculture on soil organic and inorganic carbon sequestration, and their lability: A study from a rice– wheat cropping system on a calcareous soil of eastern indo-gangetic plains. Soil Use Manage 47, 1–10.
Ellert, B. H., and Bettany, J. R. (1995). Calculation of organic matter and nutrients stored in soils under contrasting management regimes. Can. J. Soil Sci. 75, 529–538. doi:10.4141/cjss95-075
Fabrizzi, K. P., Morón, A., and García, F. O. (2003). Soil carbon and nitrogen organic fractions in degraded vs. non-degraded Mollisols in Argentina. Soil Sci. Soc. Am. J. 67, 1831–1841.
Farahani, E., Emami, H., and Forouhar, M. (2022). Effects of tillage systems on soil organic carbon and some soil physical properties. Land Degrad. Dev. 33, 1307–1320. doi:10.1002/ldr.4221
Francaviglia, R., Di Bene, C., Farina, R., and Salvati, L. (2017). Soil organic carbon sequestration and tillage systems in the mediterranean basin: A data mining approach. Nutr. Cycl. Agroecosyst. 107, 125–137. doi:10.1007/s10705-016-9820-z
Gomez, K. A., and Gomez, A. A. (1984). Statistical procedures for agricultural research. 2nd Edition. New York: John Wiley and Sons, 680.
Grandy, A. S., and Robertson, G. P. (2007). Land-use intensity effects on soil organic carbon accumulation rates and mechanisms. Ecosyst 10, 59–74. doi:10.1007/s10021-006-9010-y
Gupta Choudhury, S., Srivastava, S., Singh, R., Chaudhari, S. K., Sharma, D. K., Singh, S. K., et al. (2014). Tillage and residue management effects on soil aggregation, organic carbon dynamics and yield attribute in rice-wheat cropping system under reclaimed sodic soil. Soil Tillage Res. 136, 76–83. doi:10.1016/j.still.2013.10.001
Kemper, W. D., and Rosenau, R. C. (1986). “Aggregate stability and size distribution,” in Methods of soil analysis. Part 1. Physical and mineralogical methods. Editors A. Klute, G. S. Campbell, R. D. Jackson, M. M. Mortland, and D. R. Nielson (Madison, WI, USA: ASA and SSSA), 425–442.
Kumar, N., Chhokar, R. S., Meena, R. P., Kharub, A. S., Gill, S. C., Gupta, A., et al. (2021). Challenges and opportunities in productivity and sustainability of rice cultivation system: A critical review in Indian perspective. Cereal Res. Commun. 50, 573–601. doi:10.1007/s42976-021-00214-5
Lal, R., Kimble, J. M., and Follett, R. F. (1998). “Land use and soil C pools in terrestrial ecosystems. p. 1–10,” in Management of carbon sequestration in soil. Editors R. Lal, J. M. Kimble, R. F. Follet, and B. A. Stewart (Boca Raton, FL: CRC Press).
Lal, R. (2015). Sequestering carbon and increasing productivity by conservation agriculture. J. Soil Water Conserv. 70, 55A–62A. doi:10.2489/jswc.70.3.55a
Lehmann, A., Zheng, W., and Rillig, M. C. (2017). Soil biota contributions to soil aggregation. Nat. Ecol. Evol. 12, 1828–1835. doi:10.1038/s41559-017-0344-y
Lichter, K., Govaerts, B., Six, J., Sayre, K. D., Deckers, J., and Dendooven, L. (2008). Aggregation and C and N contents of soil organic matter fractions in a permanent raised-bed planting system in the Highlands of Central Mexico. Plant Soil 305, 237–252. doi:10.1007/s11104-008-9557-9
Liebig, M. A., Tanaka, D. L., and Wienhold, B. J. (2004). Tillage and cropping effects on soil quality indicators in the northern Great Plains. Soil Tillage Res. 78, 131–141. doi:10.1016/j.still.2004.02.002
Lützow, M. V., Leifeld, J., Kainz, M., Kögel-Knabner, I., and Munch, J. C. (2002). Indications for SOM quality in soils under different management. Geoder 105, 243–258.
Modak, K., Biswas, D. R., Ghosh, A., Pramanik, P., Das, T. K., Das, S., et al. (2020). Zero tillage and residue retention impact on soil aggregation and carbon stabilization within aggregates in subtropical India. Soil Tillage Res. 28, 104649–104655. doi:10.1016/j.still.2020.104649
Modak, K., Ghosh, A., Bhattacharyya, R., Biswas, D. R., and Das, T. K. (2019). Response of oxidative stability of aggregate-associated soil organic carbon and deep soil carbon sequestration to zero-tillage in subtropical India. Soil Tillage Res. 195, 104370. doi:10.1016/j.still.2019.104370
Mummey, D., and Stahl, P. (2004). Analysis of soil whole- and inner-microaggregate bacterial communities. Microb. Ecol. 48, 41–50. doi:10.1007/s00248-003-1000-4
Parihar, C. M., Yadav, M. R., Jat, S. L., Singh, A. K., Kumar, B., Pradhan, S., et al. (2016). Long term effect of conservation agriculture in maize rotations on total organic carbon, physical and biological properties of a sandy loam soil in north-western Indo-Gangetic Plains. Soil Tillage Res. 161, 116–128. doi:10.1016/j.still.2016.04.001
Pinheiro, F. F. M., Pereira, M. G., and Anjos, L. H. C. (2004). Aggregate distribution and SOM under different tillage systems for vegetable crops in a Red Latosol from Brazil. Soil tillage res. 77, 79–85. doi:10.1016/j.still.2003.11.005
Rees, R. M., Ball, B. C., and Campbell, C. D. (2001). “Sustaining soil organic matter,” in Sustainable management of soil organic matter. Editor R. M. Rees (Wallingford, UK: CABI Publ).
Samson, M. E., Chantigny, M. H., Vanasse, A., Menasseri-Aubry, S., and Angers, D. A. (2020a). Coarse mineral-associated organic matter is a pivotal fraction for SOM formation and is sensitive to the quality of organic inputs. Soil Biol. biochem. 149, 107935. doi:10.1016/j.soilbio.2020.107935
Samson, M. E., Chantigny, M. H., Vanasse, A., Menasseri-Aubry, S., Royer, I., and Angers, D. A. (2020b). Management practices differently affect particulate and mineral-associated organic matter and their precursors in arable soils. Soil Biol. biochem. 148, 107867. doi:10.1016/j.soilbio.2020.107867
Singh, G., Bhattacharyya, R., Das, T. K., Sharma, A. R., Ghosh, A., Das, S., et al. (2018). Crop rotation and residue management effects on soil enzyme activities, glomalin and aggregate stability under zero tillage in the Indo-Gangetic Plains. Soil Tillage Res. 184, 291–300. doi:10.1016/j.still.2018.08.006
Singh, R. K., Chaudhary, R. S., Somasundaram, J., Sinha, N. K., Mohanty, M., Hati, K. M., et al. (2020). Soil and nutrients losses under different crop covers in vertisols of Central India. J. Soils Sediments 20, 609–620. doi:10.1007/s11368-019-02437-w
Six, J., Bossuyt, H., Degryze, S., and Denef, K. (2004). A history of research on the link between (micro) aggregates, soil biota, and soil organic matter dynamics. Soil Tillage Res. 79, 7–31. doi:10.1016/j.still.2004.03.008
Six, J., Carpentier, A., van Kessel, C., Merckx, R., Harris, D., Horwath, W. R., et al. (2001). Impact of elevated CO2 on soil organic matter dynamics as related to changes in aggregate turnover and residue quality. Plant Soil 234, 27–36. doi:10.1023/a:1010504611456
Six, J., Conant, R. T., Paul, E. A., and Paustian, K. (2002a). Stabilization mechanisms of soil organic matter: Implications for C-saturation of soils. Plant Soil 241, 155–176. doi:10.1023/a:1016125726789
Six, J., Elliott, E. T., and Paustian, K. (1999). Aggregate and soil organic matter dynamics under conventional and No-tillage systems. Soil Sci. Soc. Am. J. 63, 1350–1358. doi:10.2136/sssaj1999.6351350x
Six, J., Elliott, E. T., and Paustian, K. (2000). Soil macroaggregate turnover and microaggregate formation: A mechanism for C sequestration under no-tillage agriculture. Soil Biol. biochem. 32, 2099–2103. doi:10.1016/s0038-0717(00)00179-6
Six, J., Feller, C., Denef, K., Ogle, S. M., de Moraes, J. C., and Albrecht, A. (2002b). Soil organic matter, biota and aggregation in temperate and tropical soils - effects of no-tillage. Agronomie 22, 755–775. doi:10.1051/agro:2002043
Sohi, S. P., Mahieu, N., Arah, J. R., Powlson, D. S., Madari, B., and Gaunt, J. L. (2001). A procedure for isolating soil organic matter fractions suitable for modeling. Soil Sci. Soc. Am. J. 65, 1121–1128. doi:10.2136/sssaj2001.6541121x
Trigalet, S., Van Oost, K., Roisin, C., and Wesemael, B. (2014). Carbon associated with clay and fine silt as an indicator for SOC decadal evolution under different residue management practices. Agric. Ecosyst. Environ. 19, 1–9. doi:10.1016/j.agee.2014.06.011
Veihmeyer, F. J., and Hendrickson, A. H. (1948). Soil density and root penetration. Soil Sci. 65, 487–494. doi:10.1097/00010694-194806000-00006
Virto, I., Barr, P., Burlot, A., and Chenu, C. (2012). Carbon input differences as the main factor explaining the variability in soil organic C storage in no-tilled compared to inversion tilled agrosystems. Biogeochemistry 108, 17–26. doi:10.1007/s10533-011-9600-4
Wang, Y., Liu, J., and Wang, Q. (2013). The Effects of freeze-thaw processes on soil aggregates and organic carbon. Ecol. Environ. Sci. 22, 1269–1274.
Xiao, L., Yao, K., Li, P., Liu, Y., Chang, E., Zhang, Y. i., et al. (2020). Increased soil aggregate stability is strongly correlated with root and soil properties along a gradient of secondary succession on the Loess Plateau. Ecol. Eng. 143, 105671. doi:10.1016/j.ecoleng.2019.105671
Zhang, P., Wang, Y., Xu, L., Sun, H., Li, R., and Zhou, J. (2022). Factors controlling the spatial variability of soil aggregates and associated organic carbon across a semi-humid watershed. Sci. Total Environ. 809, 151155. doi:10.1016/j.scitotenv.2021.151155
Zhang, X., Xin, X., Zhu, A., Zhang, J., and Yang, W. (2017). Effects of tillage and residue managements on organic C accumulation and soil aggregation in a sandy loam soil of the North China Plain. Catena 156, 176–183. doi:10.1016/j.catena.2017.04.012
Keywords: carbon sequestration, soil aggregation, aggregate-protected C, aggregate-associated C, silt and clay-associated C
Citation: Joseph AM, Bhattacharyya R, Biswas DR, Das TK, Bandyopadhyay KK, Dey A, Ghosh A, Roy P, Naresh Kumar S, Jat SL, Casini R, Elansary HO and Bhatia A (2023) Long-term adoption of bed planted conservation agriculture based maize/cotton-wheat system enhances soil organic carbon stabilization within aggregates in the indo-gangetic plains. Front. Environ. Sci. 11:1216242. doi: 10.3389/fenvs.2023.1216242
Received: 03 May 2023; Accepted: 29 August 2023;
Published: 14 September 2023.
Edited by:
Ilan Stavi, Dead Sea and Arava Science Center, IsraelReviewed by:
Nirmalendu Basak, Central Soil Salinity Research Institute (ICAR), IndiaAdornis Dakarai Nciizah, Agricultural Research Council of South Africa (ARC-SA), South Africa
Copyright © 2023 Joseph, Bhattacharyya, Biswas, Das, Bandyopadhyay, Dey, Ghosh, Roy, Naresh Kumar, Jat, Casini, Elansary and Bhatia. This is an open-access article distributed under the terms of the Creative Commons Attribution License (CC BY). The use, distribution or reproduction in other forums is permitted, provided the original author(s) and the copyright owner(s) are credited and that the original publication in this journal is cited, in accordance with accepted academic practice. No use, distribution or reproduction is permitted which does not comply with these terms.
*Correspondence: Ranjan Bhattacharyya, ranjanvpkas@gmail.com