Diversification and Germ-Line Determination Revisited: Linking Developmental Mechanism with Species Richness
- 1Department of Biology, Southeastern Louisiana University, Hammond, LA, USA
- 2School of Life Science, University of Nottingham, Nottingham, UK
Background: Explanations for asymmetric patterns of diversification continue to challenge paleontologists and neontologists with competing hypotheses within genetic-development and ecological frameworks. In 1988, a hypothesis was proposed that tied a primordial germ cell (PGC) determination mechanism to clade (phyla) diversification. Two general mechanisms for PGC determination are recognized: one is termed induced because induction signals are required for the production of primordial germ cells. The other mechanism is cell-autonomous, i.e., determinative, because the cells that develop in response to specific cytoplasmic determinants in the oocyte are pre-destined to become PGCs. We revisited the hypothesis and analyzed phyla diversity with germ cell determination mechanisms and examined sister clade asymmetry.
Results: After 25 years of additional data accumulation, the hypothesis that high levels of species diversification are associated with the induced mode is falsified, with the determinative mode revealed as associated with higher rates of diversification. The greater species numbers are significantly associated (ANOVA p > 0.003) with the determinative mode. Analysis with appropriate sister clades is unanimous in showing the clade with the determinative mode has a significantly greater number of species relative to its induced sister clade.
Conclusions: The PGC determination mechanism hypothesis explains asymmetrical species diversity and morphological disparity at the phylum level. We argue that the determinative mode of PGC determination is a constraint release that has enhanced evolvability and increased rates of speciation and morphological disparity among clades. Knowledge of the mechanism for extant theropods allows speculation that its sister clade, the Sauropodomorpha would have exhibited the induced mode.
Introduction
Asymmetric patterns of species diversification in myriad forms, (e.g., temporal, clade, sister clade, speciation rates) have been and remain of interest to paleontologists and neontologists (e.g., Stanley, 1975; Erwin et al., 1987; Gould et al., 1987; Eble, 2000; McPeek and Brown, 2007). In some cases morphological disparity is coupled with studies on species diversification (e.g., Valentine et al., 1994; Foote, 1997; Erwin, 2007). Two broad competing hypotheses have dominated attempts at explaining diversification asymmetries, one based on genetics and development and the other grounded in ecological theory. The former hypothesis essentially claims that rates of diversification are dependent on changes in the molecular developmental process and that these changes allow the production of novel body plans and morphologies (e.g., Cracraft, 1990; Davidson and Erwin, 2006) whereas the latter hypothesis states (at its simplest) that rates of diversification are controlled by available niche space (e.g., Hutchinson, 1959; Erwin et al., 1987). We agree with others who see no conflict between the two hypotheses and point out that novelty must come first and then ecological parameters determine if the novelties survive to the next generations (Gould et al., 1987; Eble, 1998; Erwin, 2007). It is the production of novelty that we address herein.
We agree in general with Davidson and Erwin (2006) with regard to how development leads to the production of novel morphology. However, the question remains unanswered as to what developmental system(s) may have been key triggers in the release of constraints on the evolutionarily conserved pathways to account for relative disparities in species richness between sister clades. Buss (1988) proposed an important hypothesis that argued for a specific trait to be correlated with levels of species diversification. In his paper, Buss (1988) revealed a pattern of species diversification that was associated with a mechanism of germ cell determination. There are essentially two general mechanisms for primordial germ cell (PGC) determination, a determinative mode, and an induced, or epigenetic, mode [Buss suggested a third mode he called multipotency (MP), but because it requires induction we lump it with the induced mode; Extavour and Akam, 2003; Johnson et al., 2003a,b]. In the determinative mode PGCs are specified cell-autonomously in the early embryo by the inheritance of germ cell determinants from the egg cytoplasm, whereas in the induced mode they are derived from unspecialized pluripotent precursor cells that require inducing signals to develop into PGCs. Buss compiled a large data set from an impressive and diverse array of literature and correlated species numbers within phyla with mode of PGC determination. He found that phyla with a determinative mode had fewer species than taxa with an induced mode. He suggested this correlation to be “a possible causal foundation for the pattern” of non-random species diversification.
At the close of his paper, Buss (1988, P. 317) posed the question “Can further tests be devised?” He noted the PGC determination mechanism for 13 metazoan phyla had not yet been described and he recognized each of these as a test of his hypothesis. He also suggested that a method to assess comparable rates of speciation would further test his idea to explain species diversification patterns. In the course of our work, especially Crother et al. (2007), we explicitly revealed the asymmetric patterns of species diversity and demonstrated in vertebrates (Evans et al., 2014) that increased rates of speciation are associated with a determinative mode.
The goal of our study is to test the hypothesis of Buss (1988) with new data and new methods. With this study, the results of Buss change and so do the conclusions and explanations. Our results also allow us to speculate on the PGC determination mechanisms of animals that cannot be measured directly, such as theropods and their sister clade, sauropods.
Methods
Traits
We identify two general mechanisms of PGC determination, determinative and induced, as opposed to three identified by Buss (1988). We lump his multipotency mechanism with the induced mode because even though the timing of determination is different, the MP mode requires induction events to program unspecialized cells into PGCs. The largest groups this change affects are Cnideria, Porifera, and Bryozoa.
Analyses
We constructed a table to mimic that of Buss (1988), with data of species numbers from Brusca and Brusca (2003) and PGC determination mode from Extavour and Akam (2003) and Crother et al. (2007). We constructed a simple bar graph of the log number (the data were log transformed to achieve normality) species/phylum of those data, also to mimic Buss (1988). We graphed phylum species richness versus PGC determination mechanism and tested the difference in the number of species associated with each mechanism using a one-way ANOVA.
In addition to testing the association of PGC determination mode with the overall numbers for each phylum, we made sister group comparisons. The goal of this approach, widely regarded as a powerful tool in comparative biology (e.g., Brooks and McLennan, 1992, 2002; Barraclough et al., 1998; Isaac et al., 2003), was to see if species numbers were asymmetrical in sister groups. Some of these data were derived from Crother et al. (2007) and include Anura-Caudata, within Mollusca, Teleostei-Chondrostei (a paraphyletic group), Holometabola—Hemimetabola, and Sipuncula-Errantia + Sedentaria. As in Buss (1988), any relationship between PGC determination mode and species numbers must be obvious to suggest a causal relationship.
Results
The compiled data are listed in Table 1 and reveal that the phyla in which a determinative mechanism is present have the highest numbers of species. The bar graph (Figure 1) and dot graph (Figure 2) display the table in different ways, with both showing how species numbers differ in phyla with the different mechanisms of PGC determination. The transformed data were normally distributed (confirmed by Shapiro-Wilk and Kolmogorov-Smirnoff tests). The one-way ANOVA found the number of species based on mechanism to be significantly different (p < 0.003). There remained the possibility that Arthropoda (6.04) was an outlier and skewed the results. We performed a Grubb's Test and found that no outliers were present (p > 0.05). Mesozoa, Chaetognatha, and Onychophora exhibit a determinative mode, but had low numbers of species (83, 100, 110, respectively). Porifera (5500) and Bryozoa (4500) exhibit an induced mode but had relatively high numbers of species. Worthy of note is that Porifera and Bryozoa were categorized as MP by Buss and although the PGCs require induction, they are generated throughout the life of the organism as opposed to only once during embryonic development, as in more typical animals with an induced mode. If we removed them from the induced numbers, and instead used Buss' classification into three modes, the diversification asymmetry would be even greater, with the induced mechanism even more blatantly species-depauperate. However, even with these exceptions, the sums of the two PGC mode groups show the determinative groups to have about two orders of magnitude more species than the induced mode groups (1,223,781 to 13,195).
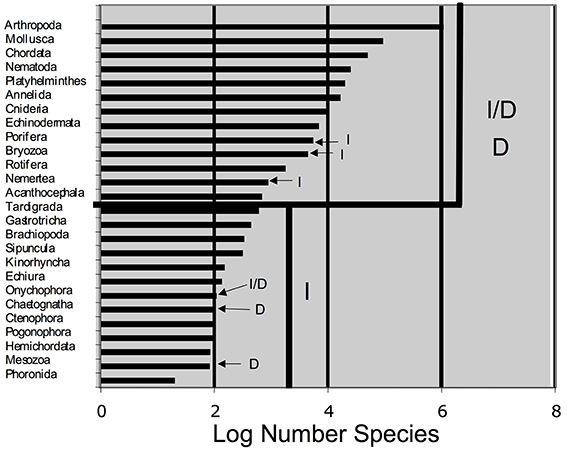
Figure 1. Log number of species for phyla whose germ cell determination mechanism is known. I, Induced mechanism; D, determinative mechanism. This graph mimics Figure 1 in Buss (1988).
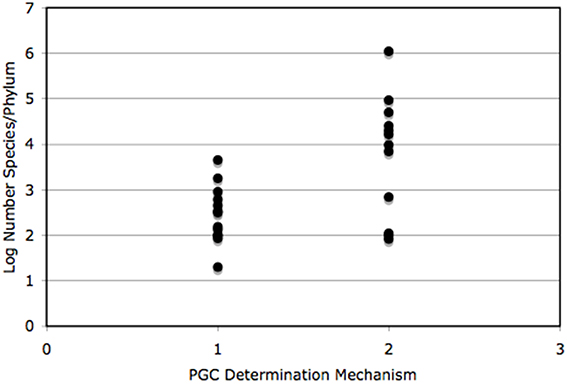
Figure 2. The log number of species is compared for PGC determination mechanism only. Number of species per group came from Table 1 and citations therein. Each dot represents the log number of species/phylum. These data were found to be statistically significantly different (see text for details). 1, induced/induced mechanism; 2, determinative mechanism.
To ascertain whether some induced mode diversity was hidden in large phyla with both modes, some of the largest phyla were broken down into smaller groups for comparison. In Mollusca, the determinative groups (Cephalopoda, Gastropoda, Bivalvia) account for 90,900 species and the induced groups (Aplacophora, Monoplacophora, Polyplacophora) account for 3195 species. In Chordata, the determinative groups Serpentes, Aves, Teleostei, and Anura alone accounted for about 43,200 species or 87% of chordate diversity. In Annelida, exemplars of Hirudinea, Oligochaeta, and Polychaeta (or Errantia and Sedentaria as in Struck et al., 2011) are all determinative (Rebscher et al., 2012) but the breakdown is complex because apparently both modes are exhibited in Clitellata (Extavour and Akam, 2003). In Arthropoda (whose diversity we underestimate by saying >1,000,000 species), the mode of determination has been established for fewer groups, but what is known among insects is instructive. Diptera, Lepidoptera, Coleoptera, and Hymenoptera account for about 771,000 species and representatives of each have been shown to exhibit a determinative mode (Ewen-Campen et al., 2012). In comparison, the insect orders Hemiptera and Orthoptera have about 98,000 species and studied species have shown an induced mode (Ewen-Campen et al., 2013). So there does not appear to be hidden diversity for the induced mode among the phyla that possess both modes.
The available sister clade comparisons unambiguously support the general trend from the overall group number analysis: Anura (4800 species)—Caudata (515), Teleostei (30,000)—Chondrostei (44, this is a paraphyletic group that we lumped together), Bivalvia + Gastropoda + Cephalopoda (90,900)—Aplacophora + Monoplacophora + Polyplacophora (1395). Based on Ewen-Campen et al. (2013), for the insect groups of known PGC determination mechanism, the sister clades Holometabola and Hemimetabola1 show asymmetry of 771,000 to 98,000. If we follow Struck et al. (2011) and Weigert et al. (2014), the Sipuncula (induced) is sister to the segmented worm clade Errantia + Sedentaria (determinative) revealing an asymmetry of about 320 to 13,000. All of the clades with the high values exhibit a determinative mode and the asymmetry in species numbers range from approximately double to three orders of magnitude in difference.
Discussion
We might quibble that the above results indicating that a determinative mechanism is associated with greater species numbers are an artifact of not including all the described extinct species. While we do not have an exhaustive list of extinct species numbers for all phyla, for the estimates we do have, the asymmetry remains because both sides increase in species richness approximately the same, but with still more species added to the determinative groups. For example, if we add estimates of extinct gastropods and cephalopods, that determinative clade increases by about 32,000 species and similarly echinoderms would add about 13,000 species. For induced clades, bryozoans, and brachiopods may have as many 12–15,000 extinct species each, which certainly increases the number of induced species but still falls about 15,000 species short of the added determinative species.
Research conducted since Buss (1988) proposed his hypothesis yields a strong association between a determinative mode of PGC specification and diversification, which is the opposite conclusion Buss arrived at based on the data available to him. Crother et al. (2007), in a paper on a related topic, noted the apparent falsification of Buss (1988), but we were surprised at how the overall group numbers changed based on new data. While appropriate sister group comparisons remain relatively few, the consistency in the asymmetry of apparent rates of speciation associated with PGC determination mechanisms is striking. Understanding the PGC determination mode in Nematomorpha, Siphonaptera, Anguimorpha, and elasmobranchs would add to the sample size of sister clade comparisons because the PGC determination mode in the sister groups are all known (assuming exemplars are representative of entire groups; Crother et al., 2007).
Of mechanistic importance, evidence suggests that all species of cephalochordate studied employ a determinative mode of PGC specification (Wu et al., 2011; Zhang et al., 2013; Dailey et al., 2016), indicating that the acquisition of determinative specification does not by necessity lead to enhanced speciation. Rather, as we have discussed elsewhere (Crother et al., 2007; Johnson et al., 2011), data such as these suggest that the acquisition of determinative PGC specification may facilitate speciation. Consistent with this, we previously showed that an induced mode of PGC determination is a developmental constraint and a determinative mode is a constraint release (Crother et al., 2007). More recent work demonstrated that the evolution of a determinative mode significantly increased the rates of evolution within a clade (Evans et al., 2014). While the mechanism for this remains unresolved, studies in amphibians, in which both modes of PGC determination exist, shed light on this problem.
Among extant amphibians urodeles retain basal embryological traits (Johnson et al., 2003a; Johnson and Alberio, 2015), and the induction mechanism for PGCs has been elaborated in axolotl embryos (Chatfield et al., 2014). In axolotl embryos, signals in the posterior-lateral mesoderm induce PGCs from pluripotent precursors. Importantly, this mechanism is a stochastic process that relies on homeostatic signaling within a germ cell niche, so that the disruption of signaling levels within the niche can terminate germ line development. Because this would result in an evolutionary dead end, the stochastic nature of induced PGC specification imposes a constraint on the ability of signaling systems within the early embryo to evolve. For example, in axolotls, and other vertebrates, PGCs develop within the posterior domain of the gastrulating mesoderm, a region whose development is dependent on fibroblast growth factor signaling. The abrogation of FGF signaling in axolotls terminates PGC development, concomitant with disrupted development of the posterior mesoderm, suggesting that the inductive mode of PGC specification constrains the potential for vertebrate embryos to evolve novel morphogenetic movements. Consistent with this, the formation and closure of the blastopore during embryogenesis has been conserved through the evolution of primitive to derived tetrapods (Shook and Keller, 2008), with the basic body plan primitive and conserved in basal vertebrate lineages. On this basis, we have speculated previously that the induced mode of PGC specification constrains morphological “experimentation” during development and concomitant with that are reduced rates of speciation (Johnson et al., 2003b; Crother et al., 2007).
The determinative mode, conversely, is a constraint release, which we argue increased/increases evolvability within lineages (Crother et al., 2007). In this mode, PGCs are rendered transcriptionally quiescent after specification by maternal molecules; therefore, they develop independent of the signaling regimes in neighboring somatic tissues in contrast to the induced mode. The gene regulatory network (GRN) for mesoderm in Xenopus includes expanded Nodal and Mix gene families which could not support the stochastic mode of PGC specification that is ancestral to amphibians. This provides evidence that the evolution of a determinative mode allows the GRNs that control early development to diverge from a conserved state (Swiers et al., 2010; Johnson et al., 2011; Chatfield et al., 2014). In Xenopus PGCs are determined early and then migrate to the anterior region of the embryo (Johnson et al., 2003b). With the PGCs protected, subsequent mutations in morphogenesis that lead to the production of novel morphological phenotypes would not affect or destroy the PGCs required to produce the next generation. In a way, Dixon (1994, P. 104) suggested the same thing, “…germ cells are segregated at a time and place that enables them to avoid the influence of the processes specifying adult body form.” That statement neatly summarized his (Dixon, 1994) enclave hypothesis, which he felt generally applied to all modes of PGC determination. In that respect we diverge from Dixon (1994), because we think that while determinative mechanisms offer protection of the PGCs, the induced mechanisms do not.
Having evolved a determinative mode, morphological “experimentation” during development could occur, potentially yielding increased rates of speciation. Consider as an example caudates contrasted with anurans. Salamanders, which have an induced mechanism, retain the primitive tetrapod body plan whereas frogs, which use a determinative mechanism, have radically anteriorized body plans with drastic reductions in the number of vertebrae, uniquely restructured sacral vertebrae, elongated pelvic elements, and enlarged hindlimbs. How the morphological release might work in invertebrates with radically altered body plans, such as mollusks, we do not know. However, given the demonstrated association between a determinative mode and rates of speciation, we think it is clear that early determination of PGCs in certain clades may increase evolvability.
We use the concept of evolvability in the manner of Kirschner and Gerhart (1998) and later authors as the ability of organisms to survive increased mutation loads and benefit from the increased genetic diversity through increased speciation rates. Or, as simplified by Brown (2014, P. 569) “…as the robust and abstract dispositional property of populations to evolve.” Tied to the greater genetic diversity would be the expectation of increased morphological diversity. So like Kirschner and Gerhart (1998), Cracraft (1990), and Davidson and Erwin (2006), among others, we see the production of novel phenotypes and potential key innovations as a simple mutation + development process. Evolvability is a clade level process and not acted upon by selection, but instead allows production of novel structures and phenotypes. These novelties may not be immediately apparent in their utility, but could be considered Gouldian spandrels (exaptations, Gould and Lewontin, 1979; Gould and Vrba, 1982; Gould, 2002). Thus, evolvability is a result of constraint release and is essentially an experimental evolutionary process in that each novelty is an experiment unto itself, i.e., a hopeful monster (Goldschmidt, 1940; Theißen, 2009).
Cracraft (1990) further argued that increased rates of diversification should be viewed as epiphenomena of innovations; therefore, that while key innovations may indeed be a constraint release and facilitate rate change, innovations themselves say little about causes of patterns of diversification. The pattern we see associated with the determinative mode of PGC determination is contra to Cracraft's (1990) prediction, and so we are not in general agreement with the idea that identified innovations are poor predictors of speciation patterns. For PGC determination mechanisms, innovation is strongly associated with increased rates of speciation (Crother et al., 2007; Evans et al., 2014).
In Erwin's (2007) discussion on the relationship between increased morphological disparity and increased diversification, he concluded that a concomitant increase in both would be the least likely to occur. A possible explanation would be that developmental GRNs would constrain developmental variability (Davidson and Erwin, 2006), at least at certain scales. The PGC data appear to provide evidence to support the scenario that Erwin considered least likely, because there is certainly increased morphological disparity associated with increased rates of diversification.
Davidson and Erwin (2006) suggested that conservation of body plans at the phylum level since the Cambrian is because of the evolution of “kernels” at the top of GRNs. They argue these GRNs are essentially invariant, and changes to them would be lethal. Because pluripotency is critical to any multicellular animal that goes through a unicellular phase, one might imagine that the pluripotency GRN, primarily established by the genes Oct4 and Nanog in vertebrates, would be one such kernel. And indeed Pou domain genes related to Oct4 have been shown to be involved in pluripotency in animals as distant as planarians (Önal et al., 2012) and cnidarians (Millane et al., 2011). However, the Oct4 gene was lost in frogs, teleost fish, and birds (Frankenberg and Renfree, 2013). Nanog was deleted from the frog genome about 200 million years ago (Dixon et al., 2010), and in teleosts it was rewired in a different GRN to regulate endoderm development, not pluripotency (Camp et al., 2009; Xu et al., 2012), long after the Cambrian. This was a massive change in a GRN for early development in vertebrates, which, for example, would be incompatible with development of mammals. It likely was made possible by the evolution of germ plasm, because pluripotent cells were no longer required to produce PGCs (Dixon et al., 2010; Johnson et al., 2011). Importantly, the loss of Oct4 occurred convergently in teleosts, frogs, and birds (theropods). So contra to the constrained kernels of Davidson and Erwin (2006), a vital vertebrate GRN can be changed, but presumably a compensatory component is required to relieve the constraint, in this case germ plasm. In animals with the induced mechanism, the pluripotency GRN appears to be conserved at least as far back as urodeles to mammals, and probably chondrostean fish as well (Dixon et al., 2010).
On Theropods and Sauropods
One of the extant groups of vertebrates that we did not do a sister group comparison on was Aves (about 10,000 extant species; we use Aves in the sense of Gauthier (1986), to refer to crown-group birds), even though we have data on crocodilians (23 extant species) which suggests they use the induced mode. Members of Aves, as we noted above, have the determinative mode and three orders of magnitude greater diversity than crocodilians. However, that comparison belies the fact that there are many other groups in the Archosauria between Aves and Crocodylomorpha (e.g., Gauthier, 1986; Benton, 1999). That fact would render the comparison of extant birds and extant crocodilians void of content.
Given that Aves is nested within Avialae, which is nested within Theropoda (Padian, 2004), the proper sister group comparison is between Theropoda and its sister clade, Sauropodomorpha (Padian et al., 1999). Unfortunately, we have no evidence for the PGC determination mechanism of sauropodomorphs, but based on the associations described above we can provide reasonable speculation. Current thought is that extant birds exhibit the determinative mode of PGC determination (Tsunekawa et al., 2000). The avian body plan is obviously derived relative to primitive tetrapods, as is the body plan of theropods in general. For example, Holtz and Osmólska (2004, P. 21) noted that “Theropoda, the sister taxon to Sauropodomorpha, is the most differentiated group among saurischians” (emphasis ours). Holtz and Osmólska (2004, P. 45) further note that many of the derived novel skeletal characters are associated with the hindlimb and “enhanced bipedal locomotion.” So although bipedalism is primitive for dinosaurs, the bipedal system was further modified in theropods. If pre-avian tetrapods already possessed the determinative mode of PGC determination, posterior morphological novelty is a possibility, because the PGCs can be anteriorized and sequestered early in development.
In contrast, sauropods exhibit “morphological conservatism” (Upchurch et al., 2004, P. 259). Admittedly, it is difficult to consider animals that weighed between 50 and 100 tons and reached lengths over 30 m as morphologically conservative, but the skeletal modifications can mostly be associated with the giant size as opposed to radical rearrangement of the general quadraped phenotype (Upchurch et al., 2004). The unfortunate problem with treating sauropods as possessing a basic conservative tetrapod body plan is that sauropods are secondarily quadrapedal, derived from the saurischian bipedal condition (Romer, 1956; Galton and Upchurch, 2004). The bipedal forms in the paraphyletic Prosauropoda appeared to have been variously more or less bipedal and quadrapedal, with their stance dependent upon their behavior, although there were a couple of species interpreted as fully bipedal. So while it appears that the body plan of sauropodomorphs should not be considered conserved, and thus our claims about PGC determination and diversification asymmetry would be wrong, it is worth noting that arguably the greatest morphological diversity and disparity in sauropodomorphs is anterior, in the modified skulls and elongated necks. In groups that exhibit an induced mode of PGC determination, it would be expected that posterior regions would remain conserved (because the PGCs must be there during development to receive the proper signaling regime) whereas the anterior could develop evolutionary novelties.
Remarkably, these characterizations are consistent with the species diversity associations found in extant forms. Extant birds alone account for about 9900 species and the remainder of extinct described theropods account for about 260 species for an estimation of 10,160 species (Lee et al., 2014). The estimated number of described sauropodomorphs is about 143 (Weishampel et al., 2004). If we extrapolate from the association described above (low species diversity, basic tetrapod body plan), we propose that sauropodomorphs exhibited the conserved primitive induced PGC determination mechanism whereas theropods, extant and extinct, use/used the derived determinative mechanism. In support of our claim that the determinative mode increased rates of morphological change and speciation, Lee et al. (2014) showed that theropod evolution occurred at rates about 150 times faster than other clades.
Coda
Buss (1988) was prescient in considering PGC determination mechanism as a significant factor in explaining asymmetrical patterns of species diversification. He posed the hypothesis that because the induced mode was strongly associated with increased rates of diversification, the induced mode could be a causal factor in differing rates of species diversification. This was a natural conclusion for the data available to him. Twenty-five years of accumulated data later, the evidence indicates that the hypothesis of Buss (1988) is falsified, that instead the determinative mode is associated with increased rates of diversification. The determinative mode of PGC determination is a constraint release that has enhanced evolvability and significantly increased rates of speciation and morphological disparity among clades. The concept of release is critical. We argue that the determinative mode allows the possibility of increased rates of speciation. While other processes cause the increased rates, without the allowance it won't happen. No doubt processes such as genome duplication, gastrulation mode, lineage restriction, etc. led to phenotypic and rate changes. However, none of these events could have occurred if the germ cell lineage was not fixed, and was thus rendered resistant to such changes.
We speculated on the mechanism in sauropodomorphs and suggest that this type of speculation be carried out for other groups. In mollusks for example, if known extinct species are included the asymmetry in diversification would probably be even more dramatic than the extant lineage-only comparisons. This would add further evidence that something as simple, yet critical for lineage continuity, as PGC determination mode could account for much of the variation in diversification rates and morphological disparity we see in the history of life.
To conclude, we present a caveat and tied to the caveat, a plea. The caveat is not insignificant but is based on the data available to us. Like Buss (1988) before us, our hypothesis is constrained by the available data. Even though there is much more data than in 1988, many of the groups' PGC determination modes are based on one or few exemplars. The same is true for the sister clade comparisons; while convincing in the congruence of sister clade asymmetrical species numbers, the comparisons are few as are the exemplars for those clades. This leads to the plea. The PGC determination mode needs to be studied in more species to test our hypotheses and our hope is that workers will be intrigued enough to collect such data.
Author Contributions
BC conducted the analyses and wrote the original draft manuscript. MW and AJ contributed to versions of the draft. All authors contributed to the ideas that form the foundation of the study. All authors read and approved the final manuscript.
Conflict of Interest Statement
The authors declare that the research was conducted in the absence of any commercial or financial relationships that could be construed as a potential conflict of interest.
Acknowledgments
C. Fontenot and C. Beachy kindly helped with the statistics and T. Struck generously provided the most recent annelid systematics and species diversity. W. Font and C. Criscione provided insight on flatworms.
Footnotes
1. ^In these two massive groups, two possible exceptions have been described. Schröder (2006) suggested that a Tribolium beetle was induced. Based on our reading of Schröder, we are not convinced. Vasa is localized and the PGCs are set aside in the early developing embryo, classic marks of a determinative mechanism. Lin et al. (2014) suggested that the hemimetabolous pea aphid presented a determinative mechanism. This case is more tricky. The RNA is not localized, but presumptive PGCs begin to take up vasa protein during cellularization. We think this is probably due to translational control, as recently described in sea urchins (Swartz et al., 2014), which is an alternate form of “early” PGC commitment. However, if the family Aphididae is compared with its sister family Lachnidae, there is an asymmetry of 3300 to 320 species. Perhaps the aphidids evolved a determinative mechanism which led to its rapid diversification (von Dohlen and Moran, 2000) and actually represent a case in support of our argument.
References
Barraclough, T. G., Nee, S., and Harvey, P. H. (1998). Sister-group analysis in identifying correlates of diversification. Evol. Ecol. 12, 751–754. doi: 10.1023/A:1017125317840
Benton, M. J. (1999). Scleromochlus taylori and the origin of dinosaurs and pterosaurs. Philos. Trans. R. Soc. Lond. B Biol. Sci. 354, 1423–1446. doi: 10.1098/rstb.1999.0489
Brooks, D. R., and McLennan, D. A. (1992). Phylogeny, Ecology, and Behavior: A Research Program in Comparative Biology. Chicago, IL: University Chicago Press.
Brooks, D. R., and McLennan, D. A. (2002). The Nature of Diversity: An Evolutionary Voyage of Discovery. Chicago: University Chicago Press.
Brown, R. L. (2014). What evolvability really is. Brit. J. Phil. Sci. 65, 549–572. doi: 10.1093/bjps/axt014
Camp, E., Sánchez-Sánchez, A. V., García-España, A., DeSalle, R., Odqvist, L., Enrique O'Connor, J., et al. (2009). Nanog regulates proliferation during early fish development. Stem Cells 27, 2081–2091. doi: 10.1002/stem.133
Chatfield, J., O'Reilly, M., Bachvarova, R. F., Ferjentsik, Z., Redwood, C., Walmsley, M., et al. (2014). Stochastic specification of primordial germ cells from mesoderm precursors in axolotl embryos. Development 141, 2429–2440. doi: 10.1242/dev.105346
Cracraft, J. (1990). “The origin of evolutionary novelties: pattern and process at different hierarchical levels,” in Evolutionary Innovations, ed M. H. Nitecki (Chicago, IL: University of Chicago Press), 21–44.
Crother, B. I., White, M. E., and Johnson, A. D. (2007). Inferring developmental constraint and constraint release: primordial germ cell determination mechanisms as examples. J. Theor. Biol. 248, 322–330. doi: 10.1016/j.jtbi.2007.05.035
Dailey, S. C., Planas, R. F., Espier, A. R., Garcia-Fernandez, J., and Somorjai, I. M. L. (2016). Asymmetric distribution of pl10 and bruno2, new members of a conserved core of early germline determinants in cephalochordates. Front. Ecol. Evol. 3:156. doi: 10.3389/fevo.2015.00156
Davidson, E. H., and Erwin, D. H. (2006). Gene regulatory networks and the evolution of animal body plans. Science 311, 796–800. doi: 10.1126/science.1113832
Dixon, J. E., Allegrucci, C., Redwood, C., Kump, K., Bian, Y., Chatfield, J., et al. (2010). Axolotl Nanog activity in mouse embryonic stem cells demonstrates that ground state pluripotency is conserved from urodele amphibians to mammals. Development 137, 2973–2980. doi: 10.1242/dev.049262
Dixon, K. E. (1994). Evolutionary aspects of primordial germ-cell formation. Germline Dev. 182, 92–110.
Eble, G. J. (1998). “The role of development in evolutionary radiations,” in Biodiversity Dynamics: Turnover of Populations, Taxa, and Communities, ed M. L. McKinney (New York, NY: Columbia University Press), 132–161.
Eble, G. J. (2000). Contrasting evolutionary flexibility in sister groups: disparity and diversity in Mesozoic atelostomate echinoids. Paleobiology 26, 56–79. doi: 10.1666/0094-8373(2000)026<0056:CEFISG>2.0.CO;2
Erwin, D. H. (2007). Disparity: morphological pattern and developmental context. Palaeontology 50, 57–73. doi: 10.1111/j.1475-4983.2006.00614.x
Erwin, D. H., Valentine, J. W., and Sepkoski, J. J. Jr. (1987). A comparative study of diversification events: the early Paleozoic versus the Mesozoic. Evolution 41, 1177–1186. doi: 10.2307/2409086
Evans, T., Wade, C. M., Chapman, F. A., Johnson, A. D., and Loose, M. (2014). Acquisition of germ plasm accelerates vertebrate evolution. Science 344, 200–203. doi: 10.1126/science.1249325
Ewen-Campen, B., Jones, T. E. M., and Extavour, C. G. (2013). Evidence against a germ plasm in the milkweed bug Oncopeltus fasciatus, a hemimetabolous insect. Biol. Open 2, 556–568. doi: 10.1242/bio.20134390
Ewen-Campen, B., Srouji, J. R., Schwager, E. E., and Extavour, C. G. (2012). Oskar predates the evolution of germ plasm in insects. Curr. Biol. 22, 2278–2283. doi: 10.1016/j.cub.2012.10.019
Extavour, C. G., and Akam, M. (2003). Mechanisms of germ cell specification across the metazoans: epigenesis and preformation. Development 130, 5869–5884. doi: 10.1242/dev.00804
Foote, M. (1997). The evolution of morphological diversity. Ann. Rev. Ecol. Syst. 28, 129–152. doi: 10.1146/annurev.ecolsys.28.1.129
Frankenberg, S., and Renfree, M. B. (2013). On the origin of POU5F1. BMC Biol. 11:56. doi: 10.1186/1741-7007-11-56
Galton, P. M., and Upchurch, P. (2004). “Prosauropoda,” in The Dinosauria, eds D. B. Weishampel, P. Dodson, and H. Osmólska (Berkeley: University of California Press), 232–258.
Gauthier, J. (1986). “Saurichian monophyly and the origin of birds,” in The Origin of Birds and the Evolution of Flight, Vol. 8, ed K. Padian (San Francisco, CA: Memoirs of the California Academy of Sciences), 1–55.
Gould, S. J., Gilinsky, N. L., and German, R. Z. (1987). Asymmetry of lineages and the direction of evolutionary time. Science 236, 1437–1441. doi: 10.1126/science.236.4807.1437
Gould, S. J., and Lewontin, R. C. (1979). The spandrels of San Marco and the Panglossian paradigm: a critique of the adaptationist programme. Proc. R. Soc. B Biol. Sci. 205, 581–598. doi: 10.1098/rspb.1979.0086
Gould, S. J., and Vrba, E. S. (1982). Exaptation-a missing term in the science of form. Paleobiology 8, 4–15. doi: 10.1017/S0094837300004310
Holtz, T. R. Jr., and Osmólska, H. (2004). “Saurischia,” in The Dinosauria, eds D. B. Weishampel, P. Dodson, H. Osmólska (Berkeley: University of California Press), 21–24.
Hutchinson, G. E. (1959). Homage to Santa Rosalia or why are there so many kinds of animals? Am. Nat. 93, 145–159. doi: 10.1086/282070
Isaac, N. J., Agapow, P. M., Harvey, P. H., and Purvis, A. (2003). Phylogenetically nested comparisons for testing correlates of species richness: a simulation study of continuous variables. Evolution 57, 18–26. doi: 10.1111/j.0014-3820.2003.tb00212.x
Johnson, A. D., and Alberio, R. (2015). Primordial germ cells: the first cell lineage or the last cells standing? Development 142:2730–2739. doi: 10.1242/dev.113993
Johnson, A. D., Bachvarova, R. F., Crother, B. I., White, M. E., Patient, R., Drum, M., et al. (2003a). Regulative germ cell specification in axolotl embryos: a primitive trait conserved in the mammalian lineage. Philos. Trans. R. Soc. Lond. B Biol. Sci. 358, 1371–1379. doi: 10.1098/rstb.2003.1331
Johnson, A. D., Drum, M., Bachvarova, R. F., Masi, T., White, M. E., and Crother, B. I. (2003b). Evolution of determinative germ cells in vertebrate embryos: implications for macroevolution. Evol. Devo. 5, 414–431. doi: 10.1046/j.1525-142X.2003.03048.x
Johnson, A. D., Richardson, E., Bachvarova, R. F., and Crother, B. I. (2011). Evolution of the germ line-soma relationship in vertebrate embryos. Reproduction 141, 291–300. doi: 10.1530/REP-10-0474
Kirschner, M., and Gerhart, J. (1998). Evolvability. Proc. Natl. Acad. Sci. U.S.A. 95, 8420–8427. doi: 10.1073/pnas.95.15.8420
Leclère, L., Jager, M., Barreau, C., Chang, P., Le Guyader, H., Manuel, M., et al. (2012). Maternally localized germ plasm mRNAs and germ cell/stem cell formation in the cnidarian Clytia. Devo. Biol. 364, 236–248. doi: 10.1016/j.ydbio.2012.01.018
Lee, M. S. Y., Cau, A., Naish, D., and Dyke, G. J. (2014). Sustained miniaturization and anatomical innovation in the dinosaurian ancestor of birds. Science 345, 562–566. doi: 10.1126/science.1252243
Lin, G. W., Cook, C. E., Miura, T., and Chang, C. C. (2014). Posterior localization of ApVas1 positions the preformed germ plasm in the sexual oviparous pea aphid Acyrthosiphon pisum. Evodevo 5, 1–17. doi: 10.1186/2041-9139-5-18
McPeek, M. A., and Brown, J. M. (2007). Clade age and not diversification rate explains species richness among animal taxa. Am. Nat. 169, E97–E106. doi: 10.1086/512135
Millane, R. C., Kanska, J., Duffy, D. J., Seoighe, C., Cunningham, S., Plickert, G., et al. (2011). Induced stem cell neoplasia in a cnidarian by ectopic expression of a POU domain transcription factor. Development 138, 2429–2439. doi: 10.1242/dev.064931
Önal, P., Grün, D., Adamidi, C., Rybak, A., Solana, J., Mastrobuoni, G., et al. (2012). Gene expression of pluripotency determinants is conserved between mammalian and planarian stem cells. EMBO 31, 2755–2769. doi: 10.1038/emboj.2012.110
Padian, K. (2004). “Basal avialae,” in The Dinosauria, eds D. B. Weishampel, P. Dodson, H. Osmólska (Berkeley, University of California Press), 210–231.
Padian, K., Hutchinson, J. R., and Holtz, T. R. Jr. (1999). Phylogenetic definitions and nomenclature of the major taxonomic categories of the carnivorous Dinosauria (Theropoda). J. Vert. Paleo. 19, 69–80. doi: 10.1080/02724634.1999.10011123
Rebscher, N., Lidke, A. K., and Ackermann, C. F. (2012). Hidden in the crowd: primordial germ cells and somatic stem cells in the mesodermal posterior growth zone of the polychaete Platynereis dumerillii are two distinct cell populations. Evodevo 3:9. doi: 10.1186/2041-9139-3-9
Schröder, R. (2006). Vasa mRNA accumulates at the posterior pole during blastoderm formation in the flour beetle Tribolium castaneum. Dev. Genes Evol. 216, 277–283. doi: 10.1007/s00427-005-0054-3
Shook, D. R., and Keller, R. (2008). Epithelial type, ingression, blastopore architecture and the evolution of chordate mesoderm morphogenesis. J. Exp. Zool. B Mol. Dev. Evol. 310, 85–110. doi: 10.1002/jez.b.21198
Smith, J. M., Cridge, A. G., and Dearden, P. K. (2010). Germ cell specification and ovary structure in the rotifer Brachionus plicatilis. Evodevo 1:5. doi: 10.1186/2041-9139-1-5
Stanley, S. M. (1975). A theory of evolution above the species level. Proc. Nat. Acad. Sci. U.S.A. 72, 646–650. doi: 10.1073/pnas.72.2.646
Struck, T. H., Paul, C., Hill, N., Hartmann, S., Hösel, C., Kube, M., et al. (2011). Phylogenomic analyses unravel annelid evolution. Nature 471, 95–98. doi: 10.1038/nature09864
Swartz, S. Z., Reich, A. M., Oulhen, N., Raz, T., Milos, P. M., Campanale, J. P., et al. (2014). Deadenylase depletion protects inherited mRNAs in primordial germ cells. Development 141, 3134–3142. doi: 10.1242/dev.110395
Swiers, G., Chen, Y. H., Johnson, A. D., and Loose, M. (2010). A conserved mechanism for vertebrate mesoderm specification in urodele amphibians and mammals. Devo. Biol. 343, 138–152. doi: 10.1016/j.ydbio.2010.04.002
Theißen, G. (2009). Saltational evolution: hopeful monsters are here to stay. Theory Biosci. 128, 43–51. doi: 10.1007/s12064-009-0058-z
Tsunekawa, N., Naito, M., Yasuhiro, S., Nishida, T., and Noce, T. (2000). Isolation of chicken vasa homolog gene and tracing the origin of primordial germ cells. Development 127, 2741–2750.
Upchurch, P., Barrett, P. M., and Dodson, P. (2004). “Sauropoda,” in The Dinosauria, eds D. B. Weishampel, P. Dodson, H. Osmólska (Berkeley, University of California Press), 259–322.
Valentine, J. W., Collins, A. G., and Meyer, C. P. (1994). Morphological complexity increase in metazoans. Paleobiology 20, 131–142.
von Dohlen, C. D., and Moran, N. A. (2000). Molecular data support a rapid radiation of aphids in the Cretaceous and multiple origins of host alternation. Biol. J. Linn. Soc. 71, 689–717. doi: 10.1111/j.1095-8312.2000.tb01286.x
Weigert, A., Helm, C., Meyer, M., Nickel, B., Arendt, D., Hausdorf, B., et al. (2014). Illuminating the base of the annelid tree using transcriptomics. Mol. Biol. Evol. 31, 1391–1401. doi: 10.1093/molbev/msu080
Weishampel, D. B., Dodson, P., and Osmólska, H. (2004). The Dinosauria. Berkeley: University of California Press.
Wu, H. R., Chen, Y. T., Su, Y. H., Luo, Y. J., Holland, L. Z., and Yu, J. K. (2011). Asymmetric localization of germline markers Vasa and Nanos during early development in the amphioxus Branchiostoma floridae. Dev. Biol. 353, 147–159. doi: 10.1016/j.ydbio.2011.02.014
Xu, C., Fan, Z. P., Müller, P., Fogley, R., DiBiase, A., Trompouki, E., et al. (2012). Nanog-like regulates endoderm formation through the Mxtx2-Nodal pathway. Devo. Cell 22, 625–638. doi: 10.1016/j.devcel.2012.01.003
Yajima, M., and Wessel, G. M. (2012). Autonomy in specification of primordial germ cells and their passive translocation in the sea urchin. Development 139, 3786–3794. doi: 10.1242/dev.082230
Keywords: primordial germ cell determination, species diversity, sister clades, morphological disparity, speciation rates, theropods, sauropods
Citation: Crother BI, White ME and Johnson AD (2016) Diversification and Germ-Line Determination Revisited: Linking Developmental Mechanism with Species Richness. Front. Ecol. Evol. 4:26. doi: 10.3389/fevo.2016.00026
Received: 02 September 2015; Accepted: 07 March 2016;
Published: 23 March 2016.
Edited by:
David Ellard Keith Ferrier, University of St. Andrews, UKReviewed by:
Ildiko M. L. Somorjai, University of St. Andrews, UKMatthias Gerberding, University of Tübingen, Germany
Copyright © 2016 Crother, White and Johnson. This is an open-access article distributed under the terms of the Creative Commons Attribution License (CC BY). The use, distribution or reproduction in other forums is permitted, provided the original author(s) or licensor are credited and that the original publication in this journal is cited, in accordance with accepted academic practice. No use, distribution or reproduction is permitted which does not comply with these terms.
*Correspondence: Brian I. Crother, bcrother@selu.edu